- 1College of Animal Science and Technology, Shihezi University, Shihezi, China
- 2School of Medicine, Shihezi University, Shihezi, China
The fermentation of grape seed meal, a non-conventional feed resource, improves its conventional nutritional composition, promotes the growth and development of livestock and fat metabolism by influencing the structure and diversity of intestinal bacteria. In this study, the nutritional components of Fermented grape seed meal (FGSM) and their effects on the growth performance, carcass quality, serum biochemistry, and intestinal bacteria of yellow feather broilers were investigated. A total of 240 male 14-day-old yellow-feathered broilers were randomly selected and divided into four groups, with three replicates of 20 chickens each. Animals were fed diets containing 0% (Group I), 2% (Group II), 4% (Group III), or 6% (Group IV) FGSM until they were 56 days old. The results showed that Acid soluble protein (ASP) and Crude protein (CP) contents increased, Acid detergent fiber (ADF) and Neutral detergent fiber (NDF) contents decreased, and free amino acid content increased in the FGSM group. The non-targeted metabolome identified 29 differential metabolites in FGSM, including organic acids, polyunsaturated fatty acids, and monosaccharides. During the entire trial period, Average daily gain (ADG) increased and Feed conversion ratio (FCR) decreased in response to dietary FGSM supplementation (p < 0.05). TP content in the serum increased and BUN content decreased in groups III and IV (p < 0.05). Simultaneously, the serum TG content in group III and the abdominal fat rate in group IV were significantly reduced (p < 0.05). The results of gut microbiota analysis showed that FGSM could significantly increase the Shannon and Simpson indices of broilers (35 days). Reducing the relative abundance of Bacteroidetes significantly altered cecal microbiota composition by increasing the relative abundance of Firmicutes (p < 0.05). By day 56, butyric acid content increased in the cecal samples from Group III (p < 0.05). In addition, Spearman’s correlation analysis revealed a strong correlation between broiler growth performance, abdominal fat percentage, SCFAs, and gut microbes. In summary, the addition of appropriate levels of FGSM to rations improved broiler growth performance and reduced fat deposition by regulating gut microbes through differential metabolites and affecting the microbiota structure and SCFA content of the gut.
Introduction
Grapes are the largest fruit crop in the world, and 80% of grape production is used for wine making (Yang and Xiao, 2013). A large amount of grape seed by-products are formed during the winemaking process that are not properly exploited and utilized. Recently, there has been increasing concern about reducing the impact of agro-industrial waste through its use as a new feed product (Câmara et al., 2020). Grape seeds have been found to be rich in fatty acids, amino acids, dietary fiber, polysaccharides and other nutrients (Zhu et al., 2015). It contains a large number of bioactive substances (such as proanthocyanidins, resveratrol and tannins, etc.) and is a feed material with good functional properties and biological activity (Chikwanha et al., 2018; Tang et al., 2018). Therefore, the development of grape seed meal resources combined with modern biotechnology not only strengthens the contribution to the implementation of circular economy, but also alleviates the current situation of feed resource shortage.
Feed biological pre-digestion techniques mainly include fermentation and enzymatic hydrolysis. Through microbial metabolic activities, feeds are enriched with more probiotics, metabolites, organic acids and other small molecules (Yang et al., 2021). After the livestock consumes the fermented feed, the number of probiotic bacteria in the feed increases and the pH value in the intestine decreases. Thus, this inhibits the growth of harmful bacteria and achieves a balance of bacteria in animal intestines (Heres et al., 2003; Chen and Yu, 2020). Consumption of fermented feed can also improve immunity (Abu-Elala et al., 2020), reduce antioxidative stress (Wu et al., 2015), increase feed intake and improve growth performance among other effects (Zhu et al., 2020). In addition, enzymatic hydrolysis was used to degrade anti- nutritional factors and increase small peptides in raw materials (Tie et al., 2020). Following feeding, animals are deficient in endogenous enzyme supplementation (Engberg et al., 2004; Alagawany et al., 2018), improving intestinal villus growth (Ritz et al., 1995; Berwanger et al., 2017) and improving nutrient uptake capacity (Córdova-Noboa et al., 2020).
Fermented Feed by Bacteria Coupled Fermentation with Enzymes has the advantages of both of these, rich in a large number of probiotics, exogenous enzymes and metabolites. Because the cecum of chickens has a large number of microorganisms and is abundant in species, they interact closely and densely with the host and ingested feed. Moreover, there is growing evidence that manipulating the composition of the gut microbiota through diet can alter the metabolic pathways and metabolite production (e.g., SCFAs) of the host, ultimately having a significant impact on the themself (Sugiharto and Ranjitkar, 2019). Therefore, according to its key functions, we hypothesized that the nutritional composition of grape seed meal would change after fermentation, producing metabolites that may have beneficial effects on broiler growth performance and intestinal health. In this study, based on untargeted metabolomics to investigate the effect of feed predigestion technology on the nutritional composition of grape seed meal, and studied the effects of different levels of FGSM added to the basal diet on the growth performance, slaughter performance, serum biochemistry, intestinal bacteria, and SCFAs content of broilers.
Materials and methods
All experimental procedures were performed in strict accordance with the guidelines and were reviewed and approved by the Institutional Animal Bioethics Committee of Shihezi University (Xinjiang, China).
Materials
Grape seed meal was provided by Western Animal Husbandry Co., Ltd., Saccharomyces cerevisiae was preserved by the Feed Biotechnology Laboratory of Shihezi University, and acid protease was purchased from Shanghai Yuanye Biotechnology Co., Ltd. Other feed ingredients, such as soybean meal and corn, were purchased from the Shihezi Farmers’ Market in Xinjiang. FGSM was fermented using Saccharomyces cerevisiae and acid protease enzymes in the Feed Biotechnology Laboratory of Shihezi University. UGSM was used as the control group (CON) without protease and probiotics were added for fermentation.
Preparation of FGSM
The fermentation process of grape seed meal was as follows: the mixture ratio of grape seed meal, corn flour, and bran was 90:5:5, and the ratio of substrate to water was 1:0.8. The mixed substrate was then autoclaved at 121°C for 15 min. After high-pressure cooling, 60 ml (bacterial solution concentration of 2.97 × 109 CFU/ml) of Saccharomyces cerevisiae liquid and 120 μg/g of acid protease (dissolved in double-distilled water before use and filtered with a 0.22 μm filter membrane for sterilization) were inoculated into each kg of fermentation substrate. Consistent mixing was performed followed by 48 h of fermentation in an incubator at 30°C. At the end of fermentation, the samples were dried in an oven at 45°C and pulverized through a 60-mesh sieve for storage. Finally, the dried samples were ground and stored at room temperature until mixture into experimental rations.
Routine nutrient testing of FGSM
Dry matter (DM), crude protein (CP), ether extract (EE), Ca, P, ASH, and acid-soluble protein (ASP) content in FGSM were determined according to the AOAC (Association of Official Analytical Chemists) (2000) method. The neutral detergent fiber (NDF) and acid detergent fiber (ADF) contents were determined using the thermostable amylase method and its residual ash method (Van Soest et al., 1991). Free amino acid profile analysis was performed according to a previously reported method (Liu et al., 2018).
Untargeted metabolomics analysis of FGSM
Metabolite extraction
100 mg of samples were weighed, precooled extract (methanol:acetonitrile:water volume ratio: 2:2:1) was added, and the solution was vortexed. Low-temperature sonication was performed on the samples for 30 min, then samples were incubated at −20°C for 10 min. Samples were centrifuged at 14,000× g for 20 min at 4°C, and the supernatant was collected for vacuum drying. During mass spectrometry, 100 μl of acetonitrile aqueous solution (acetonitrile:water volume ratio of 1:1) was added for rehydration. Samples were vortexed then centrifuged at 14,000× g for 15 min at 4°C, and the supernatant was analyzed.
Chromatographic conditions
An Agilent 1290 Infinity LC Ultra High-Performance Liquid Chromatography (UHPLC) HILIC column was used to separate samples; column temperature was 25°C; flow rate was 0.5 ml/min; mobile phase A was comprised of water +25 mm ammonium acetate +25 mm ammonia, and mobile phase B was acetonitrile with gradient elution. The procedure was as follows: 0 ~ 0.5 min, 95% B; 0.5 ~ 7 min, B varied linearly from 95 to 65%; 7 ~ 8 min, B varied linearly from 65 to 40%; 8 ~ 9 min, B was maintained at 40%; 9 ~ 9.1 min, B varied linearly from 40 to 95%; 9.1 ~ 12 min, B was maintained at 95%; the sample was placed in the autosampler at 4°C during the whole analysis. 4°C autosampler. The samples were also analyzed in random order to minimize the effect of fluctuations in the instrument signal. QC samples were inserted in the sample queue to monitor and evaluate the stability of the system and the reliability of the experimental data.
Q-TOF mass spectrometry conditions
Primary and secondary spectra of the samples were collected using an AB Triple TOF 6600 mass spectrometer. The electrospray source conditions after HILIC chromatographic separation were as follows: ion source gas 1 (Gas 1): 60, ion source gas 2 (Gas 2): 60, curtain gas (CUR): 30, source temperature: 600°C, ion capacitor voltage float (ISVF): ± 5500 V (positive and negative two modes); TOF MS scanning range: 60 ~ 1,000 m/z, product ion scanning range: 25 ~ 1,000 m/z, TOF MS scanning cumulative time 0.20 s, and product ion scanning cumulative time 0.05 s. Secondary mass spectra were acquired with information-dependent acquisition (IDA) using a high sensitivity mode, depolymerization potential (DP): ± 60 V (both positive and negative modes), collision energy within 35 ± 15 eV.
Metabolomics data processing
Raw data in Wiff format were converted to the mzXML format using the ProteoWizard software, followed by peak alignment, retention time correction, and extraction of peak areas using the XCMS software. The data obtained from XCMS extraction were first subjected to metabolite structure identification, data preprocessing, and then chemometric principles such as PCA, OPLS-DA, and multivariate statistical analysis using SIMCA 14.1 software. Metabolomics data were analyzed by Shanghai Zhongke New Life Biotechnology Co., Ltd.
Experimental design and animal management
The experiments were performed at the experimental station of the College of Animal Science and Technology, Shihezi University. 240 male yellow-feathered broilers (average initial weight: 189.7 ± 5.9 g) at 14 days were randomly divided into four groups with three replicates of 20 birds each. Every group’s basal diet was supplemented with 0% (group I), 2% (group II), 4% (group III), and 6% (group IV) FGSM. Broilers were maintained in an online flattening model with regular disinfection and cleaning. The initial temperature of the chicken house was 32°C, decreased by 2 ~ 3°C per week to 26°C, with a 24 h light cycle. The experimental period was 42 days and divided into 2 stages, with 14 to 35 days of age in stage 1 and 35 to 56 days of age in stage 2. Chickens were fed and watered freely during the experiment, and immunized according to standard immunization procedures. The chickens used in the experiment were medium-speed yellow-feathered broilers purchased from the Changji Prefecture Green Base Breeding Co. The basal diet consisted of a corn-soybean meal diet, formulated according to the Chinese agricultural industry standard “Nutritional requirements for yellow-feathered broiler chicks” (NY/T 33–2004). The test diet was crushed and turned into powder form. The composition and nutritional levels are shown in Table 1.
Assessing the growth performance of broilers
The daily feed intake of each group was recorded in detail during the experiment, and the growth performance was calculated in replicates. At days 14, 35, and 56, the broilers were weighed 12 h after fasting, and the leftovers were recovered. The average daily feed intake (ADFI), average daily gain (ADG), and feed conversion ratio (FCR) of the yellow-feathered broilers were calculated.
Sample collection for broilers
On days 35 and 56 of the experiment, six birds approaching the average weight of each group were weighed and slaughtered individually. Carcass traits, including carcass and abdominal fat, were collected, weighed, and expressed as a percentage of live weight. Blood samples were collected from euthanized birds, placed in non-heparinized tubes, and stored at −20°C until serum biochemistry analysis. Digests from the cecum were collected and divided into two subsamples, stored in RNAse and DNAse-free tubes, placed in liquid nitrogen, and stored at −80°C. One subsample was used for the analysis of intestinal bacteria, and the other was used for the determination of SCFAs.
Biochemical analysis of broiler serum
Serum biochemical parameters, including total protein (TP), albumin (ALB), blood urea nitrogen (BUN), triglycerides (TG), and total cholesterol (TC) were measured using commercially available kits (Jiancheng Bioengineering Institute, Nanjing, China).
Analysis of broilers cecal microorganism 16S rRNA
DNA was extracted from the samples using a stool panel DNA extraction kit. After passing the test by 1% agarose gel electrophoresis, the V3 – V4 region of the 16S rDNA of the samples was PCR-amplified using the universal primers 338F (5′-ACTCCTACGGGAGGCAGCAG-3′) and 806R (5′-GGACTACHVGGGTWTCTAAT-3′). The amplification program consisted of: 27 cycles (denaturation at 95°C for 30 s, annealing at 55°C for 30 s, and extension at 72°C for 30 s), and a final extension at 72°C for 10 min. Purified amplified fragments were sequenced using an Illumina MiSeq PE 2500 platform. The double-end sequencing data obtained by MiSeq sequencing removed barcodes and primer splicing, and further removed chimeras and short sequences to obtain clean tags. Under the condition of 97% similarity, the QIIME (v1.8.0) software was used for operational taxonomic unit (OTUs) clustering and species annotation. The mothur software package (version 1.31.2) was used for data processing and analysis.
Analysis of broilers cecal contents for SCFAs
To analyze SCFA, 0.30 g of the cecal contents was added to 1.5 ml of ultrapure water, vortexed for 30 s, and centrifuged at 5,000× g for 4 min. Five hundred μl of supernatant was added to a new sterile Eppendorf tube. Then, 100 μl of 25% metaphosphoric acid solution was added to the tube. The tube was vortexed for 30 s, and centrifuged at 1,500× g for 15 min through a 0.22 μm water system. The supernatant was collected into a vial for gas chromatography (Agilent 7890 B) to analyze SCFAs in the sample; the chromatographic column was DB-WAX (30 m × 0.25 mm × 0.50 μm).
Statistical analysis
One-way analysis of variance (one-way ANOVA) was performed using SPSS 22.0, and significant difference analysis was performed using Duncan’s method (p < 0.05). All data are expressed as mean ± SD. A correlation analysis was performed between growth performance, SCFAs, and intestinal microorganisms. Data were entered into SPSS version 22.0, and correlation coefficients were calculated based on Spearman correlation distances. Heat maps were constructed using ORIGIN 2021 to assess the bivariate relationships between variables.
Results
The nutritional components of FGSM
After fermentation, the contents of ASP and CP in FGSM reached 0.93 and 11.33%, respectively. It increased 365 and 24.45% with the CON group. The EE content increased to 12.31%. NDF and ADF decreased by 6.82 and 8.23%, compared with those before fermentation. The contents of Ca and P did not change significantly. The free amino acids in FGSM increased compared with CON group, except lysine. The total free amino acids were 823.56 mg/g and 903.84 mg/g in CON and FGSM group, respectively. Among them, glutamate content was the highest, accounting for 22.76 and 22.69% of the total free amino acids (Table 2).
Metabolite identification in FGSM
To reveal the changes more intuitively in metabolites after fermentation of grape seed meal, high-resolution LC–MS-based untargeted metabolomics analysis was performed. In the ESI (+) and ESI (−) modes, 240 and 169 ionic features were detected, respectively. Both the ESI positive (Figure 1A, R2X = 0.805) and ESI negative (Figure 1B, R2X = 0.820) models clearly indicated a clear separation trend between FGSM and CON, which reflects the difference in metabolites between the two sample groups. The differentially significant metabolites were identified and screened. A total of 29 differential metabolites (indexed by VIP > 3, p < 0.05) were detected in the fermented and unfermented grape seed meal samples. It mainly included 1 metabolite as a nucleic acid, 7 metabolites as lipids, 8 metabolites as carbohydrates, and 5 metabolites as organic acids. In addition, nine unclassified metabolites were identified (Table 3).
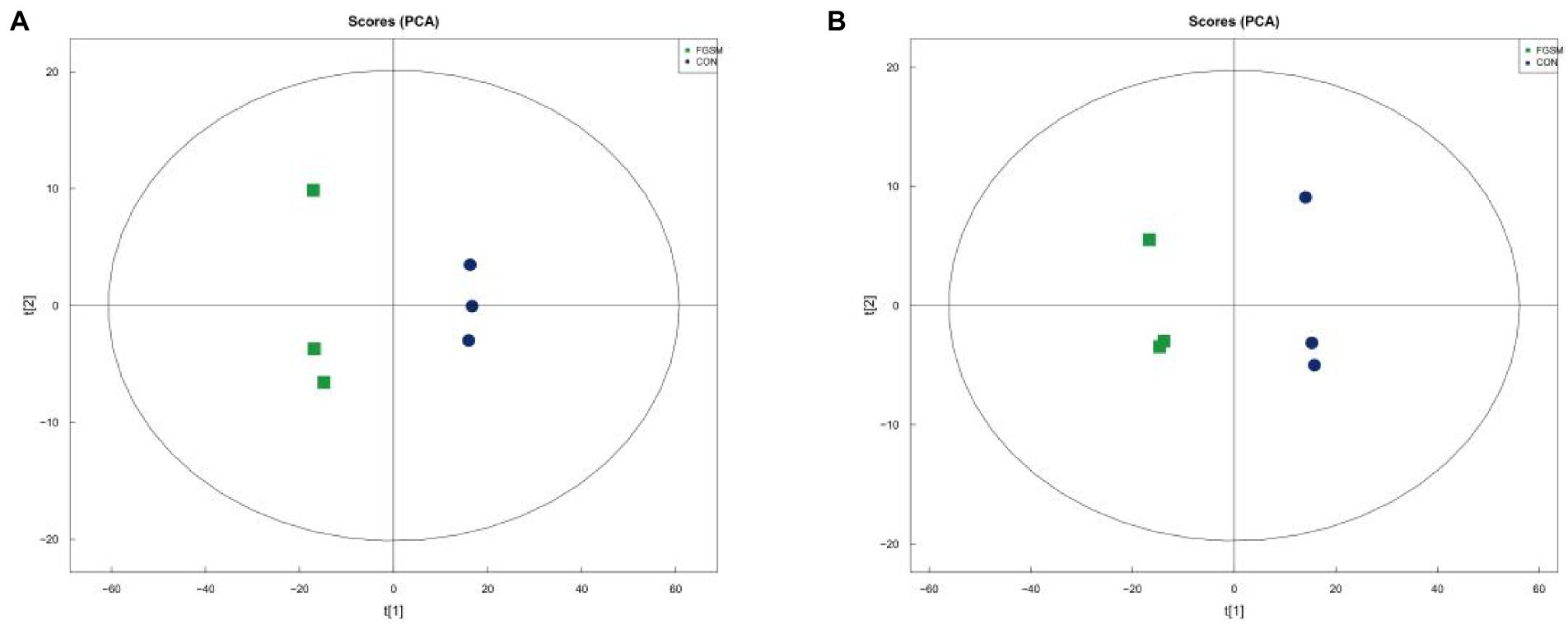
Figure 1. The principal component score plot analysis (PCA) of FGSM. (A) PCA plot in positive-ion mode; (B) PCA plot in negative-ion mode.
Analysis of differentially expressed metabolite pathways and enrichment analysis
In order to observe the significance of differences between metabolic pathways and enrichment of metabolites more intuitively, differential metabolites in the two groups of samples under positive and negative ion mode were combined in this study for enrichment analysis by KEGG pathway. The top 20 metabolic pathways with the highest significance were selected based on p values (Figure 2). The pathway enrichment analysis showed that the enriched pathways were mainly involved in Metabolic pathways. The differentially expressed metabolites detected by non-targeted metabolomics were mainly involved in ABC transporters, Biosynthesis of amino acids and Galactose metabolism. Specifically, these metabolic pathways may be critical to identify differences between FGSM and CON, whereas important differentially expressed metabolites play crucial roles in critical pathways.
Growth performance of broilers
The results showed that different levels of FGSM could increase ADG and ADFI in broilers, and different doses of FGSM had different effects on weight gain in broilers. Compared with group I, ADG was significantly increased and FCR was significantly decreased in groups III and IV (p < 0.05). FGSM had a significant effect on ADFI in broilers at the early stage of the experiment. Compared with group I, ADFI was significantly increased in group III, while ADFI was significantly decreased in group IV (p < 0.05). We found that the highest ADG and ADFI were observed in group III with 4% FGSM addition. The lowest FCR values were obtained in group IV. These data could all indicate that FGSM improved the growth performance of broilers (Table 4).
Carcass attributes of broilers
By day 35, compared with group I, the leg muscle rates of groups II, III, and IV were significantly increased by 5.89, 7.94, and 6.70%, respectively (p < 0.05). The abdominal fat rate in group IV was significantly lower than that in the other treatment groups (p < 0.05). By day 56, the pectoral muscle rate of Group II was significantly higher than other three groups, which were significantly increased by 15.49, 9.35, and 14.06%, respectively (p < 0.05). The abdominal fat rate in Group IV was significantly lower than that in Group I by 29.63% (p < 0.05) (Table 5).
Serum biochemistry of broilers
By day 35, The serum TP level of Group IV was significantly higher than that of Groups I and II (p < 0.05). Although Group III was higher than these, it was not significant (p > 0.05). By day 56, Groups III and IV were significantly higher than Groups I and II (p < 0.05). At 35 and 56 days, the serum TC content of group III was significantly lower than other three groups (p < 0.05), and the serum BUN levels of Group I were significantly higher than other three groups (p < 0.05). There were no significant differences in the other indicators among the groups (p > 0.05) (Table 6).
Out analysis of cecal microbial community in broilers
From the 48 samples, 6,010,963 valid reads were obtained after filtering low-quality raw tags and removing chimera sequences. An average number of 125,228 high-quality sequences per sample and an average sequence length of 500 bp for subsequent microbial richness and diversity analysis were obtained. A total of 3,285 OTUs were identified in the cecal contents of 35-day-old broilers, including 1,051 OTUs in the four groups. This accounts for 31.99% of the total OTUs (Figure 3A). In Groups I, II, III, and IV, there were 323, 412, 292, and 323 unique OTUs, respectively. In addition, a total of 2409 OTUs were identified in the cecal contents of 56 day broilers, including 777 OTUs in the four groups, accounting for 32.25% of the total OTUs (Figure 3B). There were 181, 121, 269, and 312 characteristic tables unique to groups I, II, III, and IV, respectively. The results showed that the total number of OUT decreased with the extension of the experimental period, and the number of OUT common to the four groups decreased correspondingly. This indicates that the bacterial diversity in the early experimental period was higher than that in the late experimental period.
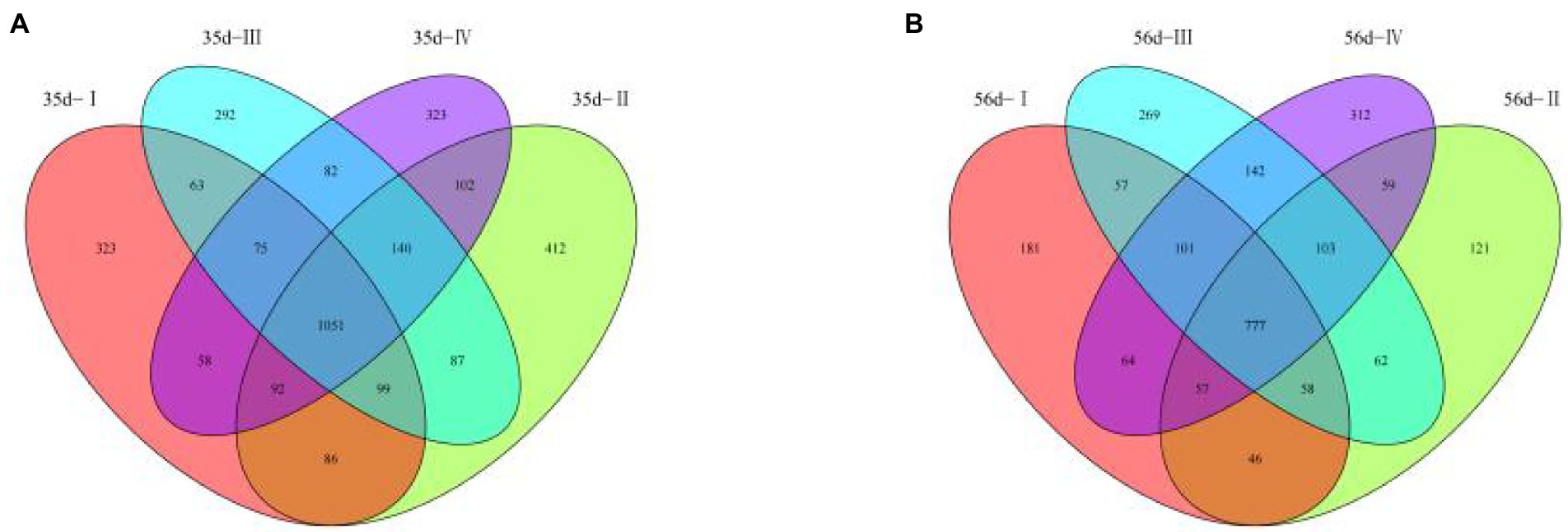
Figure 3. Analysis of Venn Diagram and Dilution Curve of Cecal Microorganism in broilers in different treatment groups. (A) Venn diagram for 35-day-old broilers; (B) Venn diagram for 56-day-old broilers.
Diversity of cecal microbiota
Alpha diversity
Based on Shannon, Simpson, Chao 1 and ACE, which represent the richness and diversity of microbial communities, alpha diversity of microbial communities in the four groups of samples was detected. By day 35, the Shannon and Simpson indices of groups III and IV were significantly higher than those of group I (p < 0.05). There was no significant difference in other indices (p > 0.05). By day 56, the observed species indices of Groups III and IV were significantly higher than that of Group I (p < 0.05); and the other indices were not significantly different (p > 0.05). As feeding time increased, the Shannon, Simpson, Chao1, ACE, observed species, and PD whole-tree indices all decreased. The results showed that the addition of FGSM to daily grains affected the abundance and diversity of the gut microbial communities (Table 7).
Beta diversity
To facilitate the observation of microbial population differences in the cecal contents between the groups, unweight-based PCoA and NMDS plots were applied to assess beta diversity. By day 35, the contribution rates of principal components 1 and 2 of PCoA were 17.7 and 7.235%, respectively (Figure 4A). By day 56, the contribution rates of principal components 1 and 2 of the PCoA were 10.21 and 8.433%, respectively (Figure 4B). The NMDS plot (Figures 4C,D) shows a clustered distribution of each sample in the four treatment groups, a small difference, similar structure of sample microbiota, and good parallelism. The results showed that the effect of adding FGSM to broiler diets in the early stage was better than that in the late stage, which would lead to a wide distribution of cecal bacteria in broilers in the early stage of the experiment.
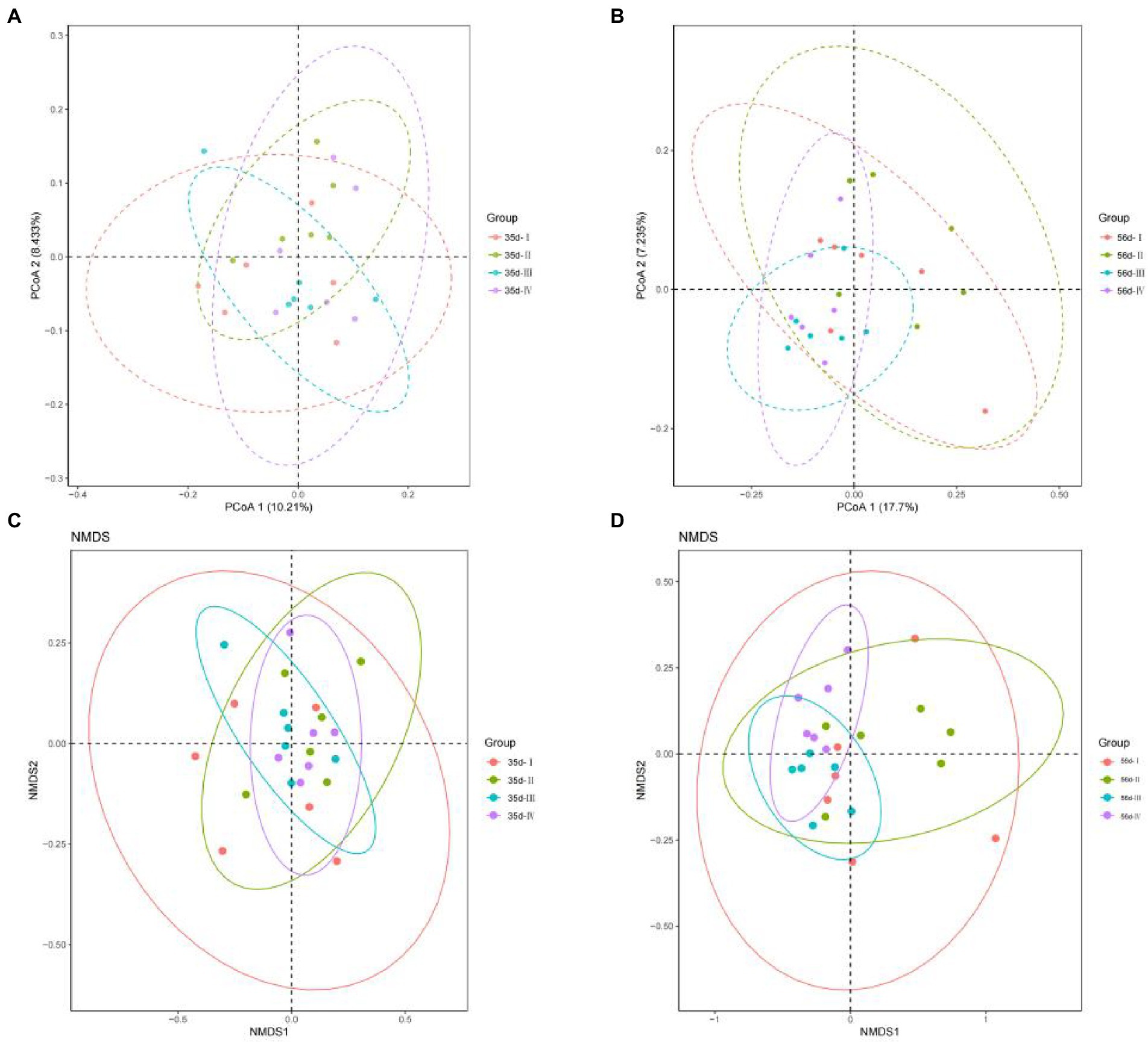
Figure 4. The cecal microbial PCoA map and NMDS map of the unweighted UniFrac distance matrix of broilers in different treatment groups. (A) 35 day-PCoA plots; (B) 56 day-PCoA plots; (C) 35 day-NMDS plots; (D) 56 day-NMDS plots.
Variation in cecal microbiota composition
To understand the variation in abundance levels within the cecal samples, we constructed bar graphs of the relative abundance of species. Based on the results of species annotation, we selected the top 10 most abundant species in each sample and grouped them at each taxonomic level (phylum, class, order, family, and genus) to generate column cumulative plots of relative abundances of species, allowing the visualization of relative abundances of species in each sample and their proportions at different taxonomic levels.
At the phylum level, p_Bacteroidetes, p_Firmicutes, and p_Synergistetes were the dominant bacteria, with average abundances of 58.98, 34.87 and 3.49%, respectively. At 35 days, the relative abundance of p_Bacteroidetes in Groups III and IV was significantly reduced (p < 0.05), and the relative abundance of p_Firmicutes was significantly increased (p < 0.05) compared with Group I. The relative abundances of p_Actinobacteria and p_Cyanobacteria in Group III, were significantly higher than those in Group I (p < 0.05; Figure 5A). At 56 days, the relative abundances of p_Actinobacteria and p_Firmicutes in Group IV increased significantly (p < 0.05), and the relative abundance of p_Bacteroidetes decreased significantly (p < 0.05) compared with Group I. The relative abundances of other phyla were not significantly different among the groups (p > 0.05).
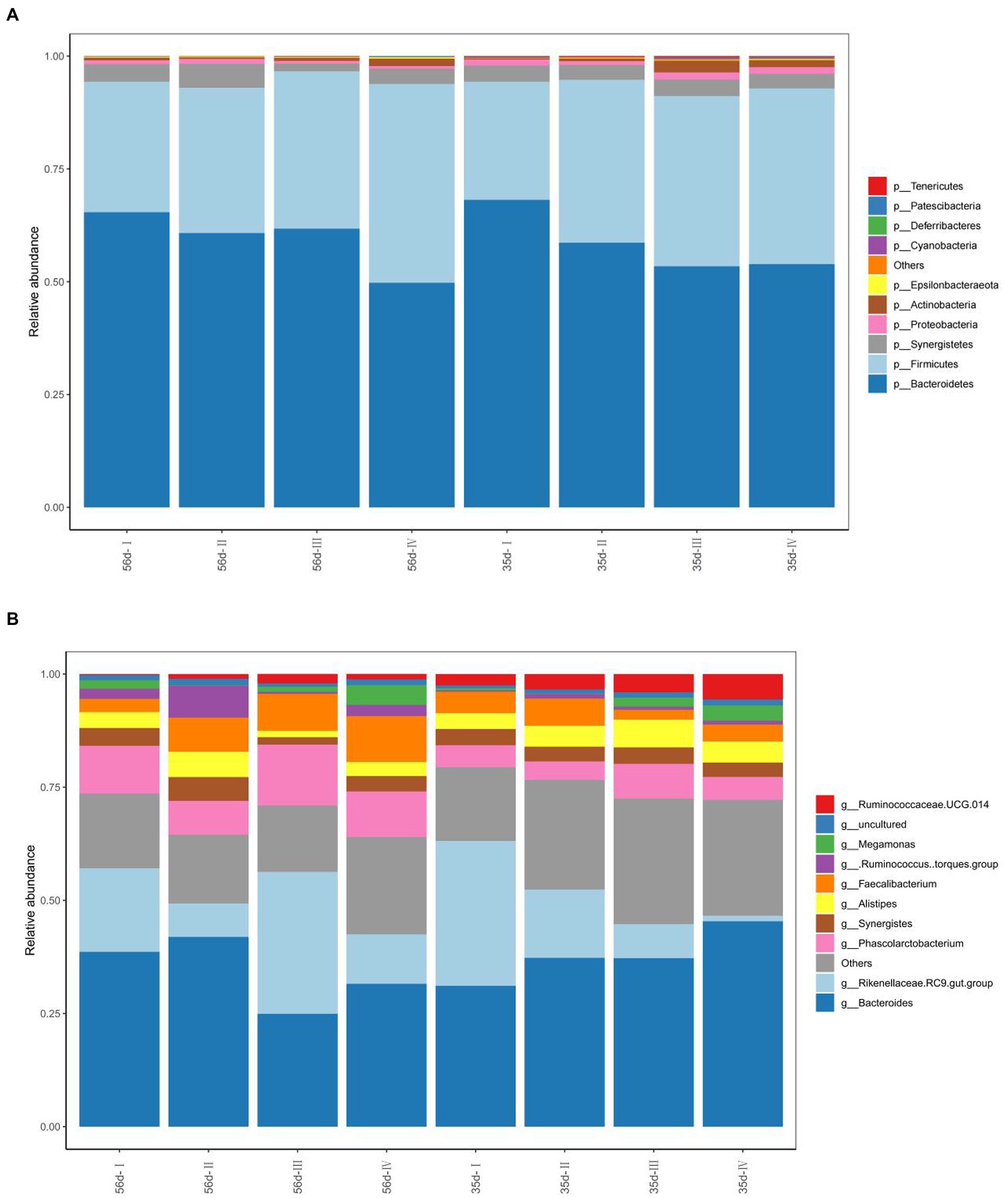
Figure 5. The composition of the cecal microflora of broilers in different treatment groups. (A) The phylum level composition of the microflora; (B) The genus level composition of the microflora.
In addition, the top ten abundant microbial groups at the genus level were obtained through analysis of bacterial composition. They are g_Bacteroides, g_Rikenellaceae RC9 gut group and g_Alitipes of Bacteroides, g_[Ruminococcus] torques group, g_Phascolarctobacterium, g_Faecalibacterium, g_Ruminococcaceae UCG-014 of p_Firmicutes, g_Synergistes, g_Megamonas, uncultured, and others (Figure 5B). The dominant bacterial groups are g_Bacteroides, g_Rikenellaceae RC9 gut group, and others. At day 35, the relative abundance of g_[Ruminococcus] torques group in Groups II and IV increased significantly (p < 0.05). The relative abundance of other was significantly increased in Groups II, III, and IV, which were supplemented with FGSM (p < 0.05). At 56 days, the relative abundance of g_Alistipes in Group II was higher than that in the other test groups.
Overview of LEfSe analysis of gut microbiota
Biomarker species with different statistical differences were observed by LEfSe (Linear discriminant analysis Effect Size). Species that met the screening criteria were identified (|LDAscore| > 2), and differential species were colored according to the most abundant group in which the species were located.
At 35 days, 21 species showed significant differences in abundance between groups, of which Group III showed the highest abundance (12 spieces). The relative abundances of g_Paraprevotella and g_Flavobacterium were higher in Group I. The dominant bacteria in Group II were g_Ruminococcus_torques group and g_Flavonifractor. The species with significant differences in Group III were p_Actinobacteria, g_Ruminiclostridium 5, g_Eubacterium hallii group, g_Lachnoclo -stridium, g_Enorma, and g_Parabacteroides. In Group IV, g_Megamonas and g_Fournierella were abundant (Figures 6A,C).
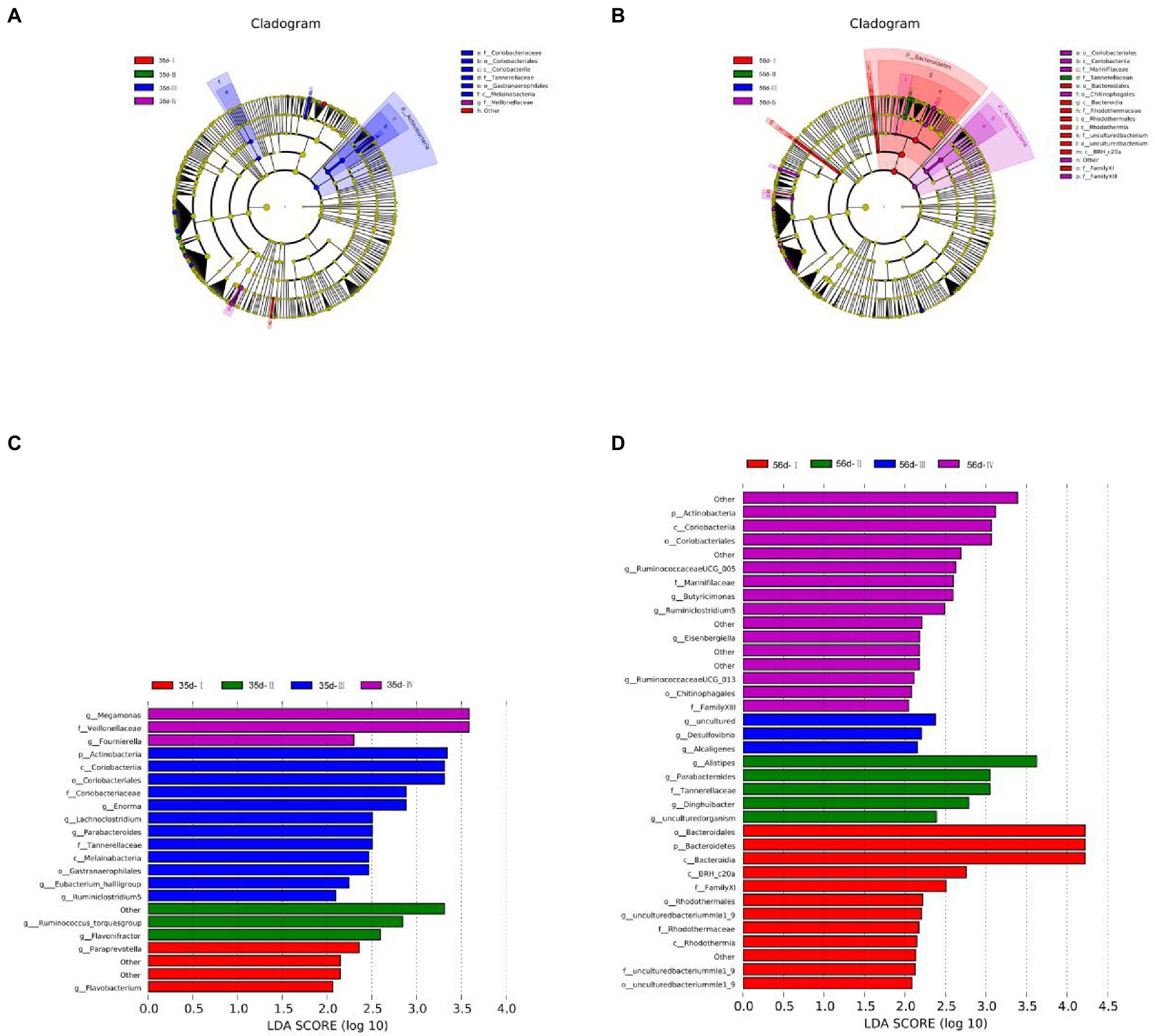
Figure 6. Differential microbial LEfSe analysis and LDA score chart of the cecum of broilers in different treatment groups. (A) LEfSe analysis of 35-day-old broilers; (B) LEfSe analysis of 56-day-old broilers; (C) LDA score chart of 35-day-old broilers; (D) LDA score chart of 56-day-old broilers. LEfSe analysis was used to determine the phylogenetic characteristics of specific bacterial taxa and major bacteria in three different groups. Biomarker taxa are highlighted by colored circles and shaded areas. The diameter of each circle is related to the abundance of taxa in the community.
At 56 days, 35 species showed significant differences in abundance between groups, with 12 in Group I, 5 in Group II, 3 in Group III, and 16 in Group IV. The relative abundances of p_Bacteroidetes and g_uncultured bacteriummlel_9 in Group I were relatively high. The dominant genera in Group II were g_Alistipes, g_Parabacteroides, g_Dinghuibacter, and g_uncultured organism. The species with significant differences in Group III were g_Desulfovibrio, g_Alcaligenes, and g_uncultured. The relative abundances of p_Actinobacteria, g_Ruminococcaceae -UCG005, g_Butyricimbergiella, g_Eisenbergiella, g_Ruminiclostridium 5, and g_Ruminococcaceae UCG_013 were high in Group IV (Figures 6B,D).
SCFAs of cecal contents
Acetic acid, propionic acid, butyric acid, and total acid concentrations did not differ among groups II, III, and IV supplemented with FGSM throughout the test period (p > 0.05), but their levels were lower than those of group I. In addition, the yield of Butyric acid was increased in group III by day 56 (p < 0.05) (Table 8).
Spearman correlation analysis
Finally, we assessed the relationships between growth performance, abdominal fat, gut microbes, and SCFAs using Spearman’s correlation analysis. As shown in Figure 7A, at 35 days, FCR of broilers was positively correlated with total acid content and p_Deferribacteres, and ADG was positively correlated with p_Actinobacteria while showing the opposite trend with FCR. Meanwhile, FCR also showed a negative correlation with g_[Ruminococcus] torques group.
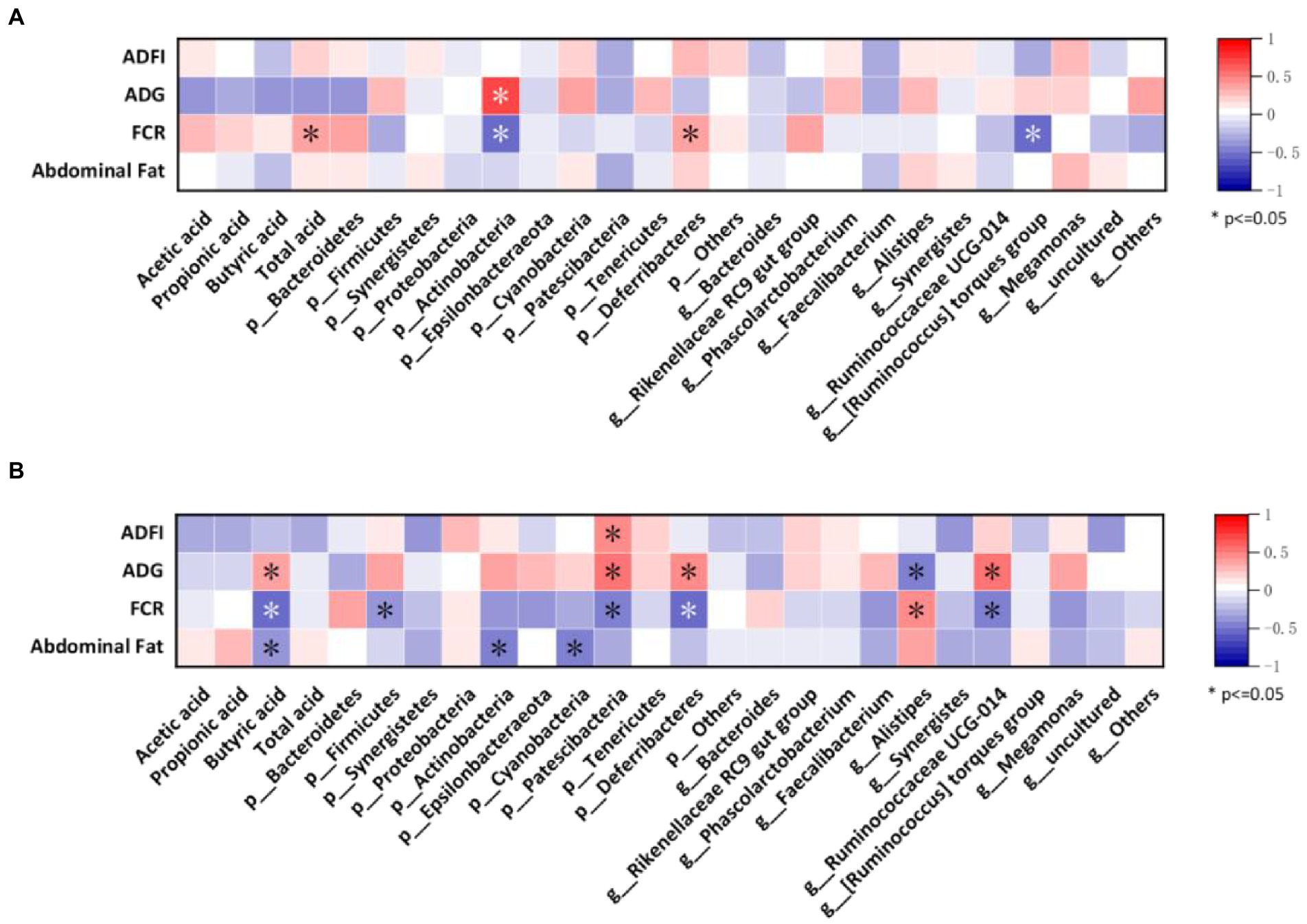
Figure 7. Correlation between the most abundant taxa of the cecal microbiota with growth performance and SCFAs of broilers. (A) Heatmap of samples from 35-day-old broilers; (B) Heatmap of samples from 56-day-old-broilers.
Figure 7B shows the correlation between growth performance, abdominal fat with gut microbes, and SCFAs in broilers at 56 days. ADFI was found to be positively correlated with the p_Patescibacteria. Additionally, ADG was positively correlated with butyric acid, p_Patescibacteria, p_Deferribacteres, and g_Ruminococcaceae UCG-014 and negatively correlated with g_Alistipes. FCR showed a negative correlation with butyric acid, p_Firmicutes, p_Patescibacteria, p_Deferribacteres, and g_Ruminococcaceae UCG-014 and a positive correlation with g_Alistipes. Moreover, abdominal fat was negatively correlated with butyric acid, p_Actinobacteria, and p_Cyanaceae.
Discussion
Improving feed nutrient content to increase broiler feed intake
Fermented grape seed meal by Bacteria coupled fermentation with enzymes has advantages over the individual processes of probiotic fermentation and protease enzymatic hydrolysis. Acid protease can improve the nutritional quality of grape seed meal. By directly increasing the number of proteases, the reaction rate of protease hydrolysis is increased. The macromolecular protein in feed material is decomposed into peptides and free amino acid, so as to improve the conversion rate of feed in animal organism. When fermenting grape seed meal with Saccharomyces cerevisiae, the acid-soluble protein content increases for the following reasons: yeast cells contain a large number of proteins and amino acids. And, yeast can produce protease during the fermentation process. As fermentation proceeds, nutrient-active substances such as organic acids (tartaric, malic, and citric acid) are produced during yeast growth and reproduction. They involved in enzymatic reactions through the tricarboxylic acid cycle, allowing proteases to break down large molecules of proteins at a faster rate. Also, microorganisms can convert non-protein nitrogen into protein nitrogen, which can be absorbed and used by livestock (Tchorbanov and Lazarova, 1988; Zhang, 2012; Wu, 2018). Moreover, the contents of NDF and ADF were reduced. The main reason was that during the fermentation process, the microorganisms produced cellulases that could decompose cellulose, hemicellulose and lignin during growth and reproduction, which reduced the fiber content in the FGSM (Rodríguez Muela et al., 2017). Simultaneously, organic acids produced by S. cerevisiae during fermentation could improve feed palatability. The relative concentration of monosaccharides used as sweeteners in feed such as D-mannose and L-fucose increased in FGSM. It has a unique volatile aroma of grapes, fragrant taste, and good palatability. Therefore, the fermented grape seed meal by Bacteria coupled fermentation with enzymes can effectively improve the nutritional composition of feed ingredients and improve animal feed intake.
FGSM promotes broiler growth and improves carcass quality
In this study, the addition of FGSM significantly increased broiler growth performance. The growth performance of animals is influenced by muscle proteins, which constitute the net balance of protein synthesis and degradation. Amino acids are basic components of proteins and play roles in regulating nutrient transport, gut microbiota, and antioxidant responses (Mott et al., 2008). In the intestine, proteins are broken down into small peptides and free amino acids by enzymes and transported through different nutrient transporters (Wu et al., 2014). By untargeted metabolomics analysis, we found that the relative concentration of adenosine was lower in FGSM. Adenosine plays an important role in cellular metabolism by acting as an intermediate in ATP synthesis. Studies have shown that phosphoribose in yeast cells is degraded into a mixture of adenosine, adenine, ribose, single nucleotide, and polynucleotides by an enzymatic system. Then Adenosine and other substances are phosphorylated through the pathway of glycolysis to produce ATP, which then binds to the protein hydrolysis region of ABC transporters, causing ATP to be hydrolyzed. The energy generated by metabolism changes the conformation of transporters, resulting in transporters transporting various sugars, amino acids, proteins and other cellular metabolites to the extracellular space (Guerrero and Mullet, 1986). It means that the content of small peptides and free amino acids increases through this metabolic pathway. This is supported by the results of the free amino acid content in FGSM. Therefore, enhanced intestinal uptake of amino acids in broilers fed diets supplemented with FGSM would favor protein deposition and ultimately promote growth.
In the early part of the trial, the breast and leg muscle rates increased in broilers that were fed FGSM, but there was no significant difference between the full clearance rate and half clearance rate. This suggests that broiler muscle protein metabolism had the potential to change (Lee et al., 2009). Rodas et al. (1995) found that enhanced synthesis of metabolic proteins can be observed when TP levels increase. The results of this study showed that the addition of 6% FGSM could significantly increase the TP content in the serum of broilers and increase the ALB content. This indicates that FGSM could improve serum TP and ALB concentrations and enhance protein metabolism in broilers. Dhanalakshmi et al. (2007) showed that good nutritional status maintained high levels of total serum protein and albumin, with increased levels of both indicating high metabolic activity. This is similar to (Feng et al., 2007), which demonstrate the positive effect of fermented feed on the metabolic capacity of broilers. BUN is one of the protein metabolites, and its content reflects the status between protein metabolism level and amino acid balance in animals to some extent (Scott et al., 1982). When serum BUN levels are reduced, amino acid catabolism decreases and nitrogen deposition in the body increases, indicating enhanced protein metabolism (Sugiharto et al., 2020). Therefore, adding FGSM to the diet plays an important role in promoting the absorption and utilization of protein in broilers.
At the same time, we found that FCR gradually decreased as the amount of FGSM added increased. In particular, the FCR of group IV was significantly lower than that of the remaining three groups, indicating that the addition of FGSM to the diets had better economic benefits. One study shown that the addition of peas fermented with probiotics or treated with complex enzymes to broiler diets reduces FCR throughout the experimental period (Goodarzi et al., 2017). In another study, the addition of fermented corn gluten flour to daily food improved ADG in broilers (Yan et al., 2018). This is more reflective of the improved nutritional composition of the feed after fermentation or enzymatic digestion, which has the effect of promoting animal growth.
FGSM regulates lipid metabolism in broilers
Fat deposition in broilers is dominated by three areas: subcutaneous fat, abdominal fat, and intramuscular fat, with abdominal fat receiving more attention than other adipose tissues. Abdominal fat becomes visible at 7 days of age in broilers (Kim and Voy, 2021), indicating a rapid growth rate. The number of adipocytes gradually increases with age and broiler fat cells increase in size as they absorb and store fatty acids (Bai et al., 2015). Because of ease in dissection and weighing, abdominal fat percentage is widely used to assess the total lipid content in broilers. In the group fed with 6% FGSM, there was a significant reduction in abdominal fat percentage and serum TG levels, suggesting that FGSM regulates body lipid metabolism (Yasar and Yegen, 2017).
After fermentation, the number of probiotic bacteria in FGSM increased. Homma and Shinohara (2004) indicated that probiotics can inhibit lipid synthesis and promote fatty acid catabolism, thereby inhibiting abdominal fat deposition. TC and TG are important components of blood fat, and their levels reflect the absorption and metabolism of fat in the body. Kim and Voy (2021) reported that abdominal fat is the main site of TG storage in mature broilers. When the weight of abdominal fat in broilers decreased, the TG content stored in abdominal fat also decreased and the TG content in the serum increased. Our study found that the addition of appropriate FGSM concentrations resulted in a decrease in serum TG levels. Ooi and Liong (2010) reported that some probiotics are effective at lower levels, whereas others require higher amounts to exert cholesterol-lowering effects. Cholesterol adsorbed in the cell wall of probiotics, or the cells of the bacterium can absorb cholesterol and allow cholesterol removal, thus promoting fat metabolism (Milićević et al., 2014).
The oils in grape seed meal are rich in fatty acids (FAs). Compared with unsaturated fatty acids, saturated fatty acids are more stable. However, they can be converted from saturated fats (palmitoleic and stearic acids) to unsaturated fatty acids (oleic, linolenic, and free fatty acids) by microorganisms with a range of active proteins, such as dehydrogenases and lengthening enzymes (Rhead et al., 1971). The unsaturated fatty acids present in FGSM reduce fat accumulation (Riera-Heredia et al., 2020). Polyunsaturated fatty acids (PUFAs) are classified as n-3 or n-6 depending on the position of the first double bond relative to the methyl end of the molecule. At the cellular level, Fleckenstein-Elsen et al. (2016) found that n-3 PUFAs inhibit lipid production and attenuate lipid accumulation in adipocytes. In contrast, n-6 PUFAs tended to be pro-lipogenic (Massiera et al., 2003). According to the results of non-targeted metabolomics, α-linolenic acid belonging to n-3 PUFAs was upregulated and linoleic acid belonging to n-6 PUFAs was downregulated, resulting in the inhibition of abdominal fat deposition.
FGSM alters the composition of broilers intestinal bacteria
Intestinal microorganisms play important roles in maintaining animal health, growth, feed absorption, and utilization (Zhang et al., 2018). However, its composition and structure are constrained by various factors such as feed, age, and feeding method (Carrasco et al., 2019). Among these, feed has the greatest effect on intestinal bacteria, which changes their structure and quantity. The health of animals is affected by the production of specific metabolites through biological reactions (Doré and Blottière, 2015). Therefore, changes in feed nutrient composition are very important for the regulation of intestinal bacteria. We found that organic acids, monosaccharides, and unsaturated fatty acids, which are differential metabolites produced by FGSM, have some regulatory effects on broiler gut microbiota.
Our study showed that dietary supplementation of FGSM altered the composition of the cecal microbial community and increased cecal microbial diversity in broilers. At phylum level, Bacteroides, Firmicutes, Synergistetes, and Proteobacteria were the four dominant groups in broiler cecal microorganisms. Similar to the results of Ma et al. (2018), but the third dominant phylum was altered. Synergistetes is a newly discovered genus of bacteria that is widely found in anaerobic environments such as the animal intestines and soil (Jumas-Bilak et al., 2009). As a class of Gram-negative, obligate anaerobic bacteria, most of them can grow at room temperature and neutrally and have the ability to degrade amino acids. Simultaneously, Synergistetes caused changes in the dominant flora in the gut of broilers, which was responsible for the changes in the structure of the gut community.
When the diet contains high levels of free amino acids, unsaturated fatty acids, and monosaccharides, intestinal bacteria in the body have a higher proportion of Firmicutes and a lower proportion of Bacteroidetes (De Filippo et al., 2010). Both have a direct relationship in improving the digestion and absorption of nutrients and energy metabolism in animals (Jumpertz et al., 2011), both induce fat deposition in animals. However, Firmicutes more effectively extract energy from food, thereby promoting weight gain. Additionally, most studies support an increased (F/B) ratio in obese individuals (Crovesy et al., 2020). In this study, the (F/B) ratio was <1 in all four test groups, and the (F/B) ratio also increased correspondingly with the increase of FGSM addition, but the deposition of abdominal fat decreased. In a Ukrainian study, individuals with an F/B ratio ≥1 were 23% more likely to be overweight than those with an F/B ratio <1 (Koliada et al., 2017). Therefore, an increase in the (F/B) ratio cannot be used as the sole basis for obesity, and the specific ratio has to be discussed. Bervoets et al. (2013) reported that a higher (F/B) indicates a relative abundance of energy-metabolizing microorganisms in the gut microbiota. Similarly, an increased F/B ratio is often associated with growth-promoting performance (Salaheen et al., 2017). In this study, the addition of different concentrations of FGSM to the diet did not change the status of Bacteroidetes as the dominant bacterial group. However, with increasing FGSM levels, the relative abundance of Bacteroidetes decreased and Firmicutes increased. The reduction in abdominal fat percentage, increase in daily weight gain, and reduction in FCR in broilers provided more evidence of efficient energy utilization.
Actinobacteria are key players in the maintenance of the intestinal barrier and consist of three major families of anaerobic bacteria (Bifidobacteria, Propionibacterium, and Corynebacterium) and one family of aerobic bacteria (Streptomyces). Among these, Bifidobacteria are considered important probiotics in Actinobacteria. Studies have reported that most antimicrobials are composed of bioactive substances produced by Actinobacteria and show good antibacterial properties in controlling pathogenic microorganisms (Gomes et al., 2017). The results of the non-targeted metabolome showed an increase in the relative concentrations of sugar metabolites such as D-mannose and L-amylose. When animals consume diets containing monosaccharides, they benefit from increased abundance of Bifidobacteria and colonization of the intestinal tract, thereby improving broiler intestinal health (Dev et al., 2020). Previous studies have shown that Actinobacteria can utilize complex carbohydrates and contribute to improved growth performance and increased feed conversion efficiency in broilers (Courtin et al., 2008). Also, the relative abundance of Bifidobacteria has been correlated with fat deposition. In a study comparing fecal samples from lean and obese individuals, it was found that the abundance of Bifidobacteria differed between the two, their relative abundance showed a negative correlation with the percentage of body fat (Teixeira et al., 2013).
To further investigate the effect of FGSM on gut microbiota diversity in yellow-feathered broilers, we analyzed differences at the genus level. Among the four groups of samples, Bacteroides and Rikenellaceae RC9 gut group under p_Bacteroides were the dominant genera. This genus help the host to break down polysaccharides, generate volatile fatty acids and butyrate. In this way, it improves the host immunity and facilitating the regulation of the intestinal immune system (Zhang et al., 2019). In contrast, [Ruminococcus] torques group, Phascolarcto -bacterium, Faecalibacterium, and Ruminococcaceae UCG-014 under p_Firmicutes (Louis and Flint, 2017) which can increase the concentration of SCFAs in the intestine. Macfarlane and Macfarlane (2012) pointed out that the content of SCFAs in the gut is closely related to the composition and abundance of gut microbiota. So FGSM increases the concentration of SCFAs in the gut. The main sources of SCFAs in the gut of animals are bacterial metabolites produced by anaerobic fermentation of carbohydrates that are not easily digested (Garron and Henrissat, 2019). It not only acts as an energy source for host cells and gut microbiota, but also improves host lipid and glucose metabolism. Moreover, the production of acetic acid and butyric acid by gut microbiota can cause an increase in broiler feed intake (Chen et al., 2022). Therefore, the pH in the intestine of broilers fed with FGSM decreased, which inhibited the growth of harmful microorganisms. Thereby improving the structure of the intestinal flora and enhancing growth performance, further exerting a potential promotional effect on intestinal health.
In summary, the nutritional composition of grape seeds changed after fermentation, and the concentrations of free amino acids, unsaturated fatty acids, monosaccharides, and organic acids increased. Adding 4% ~ 6% FGSM to broiler diets promotes broiler growth and regulates fat metabolism by altering gut microbiota structure.
Data availability statement
The datasets presented in this study can be found in online repositories. The names of the repository/repositories and accession number(s) can be found at: https://www.ncbi.nlm.nih.gov/, PRJNA858635.
Ethics statement
The animal study was reviewed and approved by Animal Ethics Committee of the First Affiliated Hospital of Shihezi University School of Medicine. Written informed consent was obtained from the owners for the participation of their animals in this study.
Author contributions
SN and CN carried out the experimental design of the study. SN, MY, XZ, and HW contributed to the experimental implementation. SN, MY, HW, and JL contributed to the sampling for this study. SN, MY, and CN contributed to data analysis. SN, MY, XZ, JN, CC, WZ, and CN contributed to the writing of the article. All authors contributed to the article and approved the submitted version.
Funding
This work was supported by the Science and Technology Innovation Talent Program of Xinjiang Production and Construction Corps (project no. 2020CB023) and the Young Innovation Talent Cultivation Program of Shihezi University (project no. CXRC201807).
Conflict of interest
The authors declare that the research was conducted in the absence of any commercial or financial relationships that could be construed as a potential conflict of interest.
Publisher’s note
All claims expressed in this article are solely those of the authors and do not necessarily represent those of their affiliated organizations, or those of the publisher, the editors and the reviewers. Any product that may be evaluated in this article, or claim that may be made by its manufacturer, is not guaranteed or endorsed by the publisher.
References
Abu-Elala, N. M., Younis, N. A., AbuBakr, H. O., Ragaa, N. M., Borges, L. L., and Bonato, M. A. (2020). Influence of dietary fermented Saccharomyces cerevisiae on growth performance, oxidative stress parameters, and immune response of cultured Oreochromis niloticus. Fish Physiol. Biochem. 46, 533–545. doi: 10.1007/s10695-019-00711-9
Alagawany, M., Elnesr, S. S., and Farag, M. R. (2018). The role of exogenous enzymes in promoting growth and improving nutrient digestibility in poultry. Iran. J. Vet. Res. 19, 157–164.
AOAC (Association of Official Analytical Chemists) (2000). Official Methods of Analysis of Official Analytical Chemists International.17th Edn. Arlington,VA: Association of Official Analytical Chemists.
Bai, S., Wang, G., Zhang, W., Zhang, S., Rice, B. B., Cline, M. A., et al. (2015). Broiler chicken adipose tissue dynamics during the first two weeks post-hatch. Comp. Biochem. Physiol. A Mol. Integr. Physiol. 189, 115–123. doi: 10.1016/j.cbpa.2015.08.002
Bervoets, L., Van Hoorenbeeck, K., Kortleven, I., Van Noten, C., Hens, N., Vael, C., et al. (2013). Differences in gut microbiota composition between obese and lean children: a cross-sectional study. Gut. Pathog. 5:10. doi: 10.1186/1757-4749-5-10
Berwanger, E., Nunes, R. V., Pasquetti, T. J., Murakami, A. E., de Oliveira, T. M., Bayerle, D. F., et al. (2017). Sunflower cake with or without enzymatic complex for broiler chickens feeding. Asian-Australas J. Anim. Sci. 30, 410–416. doi: 10.5713/ajas.15.0644
Câmara, J. S., Lourenço, S., Silva, C., Lopes, A., Andrade, C., and Perestrelo, R. (2020). Exploring the potential of wine industry by-products as source of additives to improve the quality of aquafeed. Microchem. J. 155, 104758–104710. doi: 10.1016/j.microc.2020.104758
Carrasco, J. M. D., Casanova, N. A., and Fernández Miyakawa, M. E. (2019). Microbiota, gut health and chicken productivity: what is the connection? Microorganisms 7:374. doi: 10.3390/microorganisms7100374
Chen, K., Hu, M., Tang, M., Gao, C., Wang, H., Man, S., et al. (2022). Oligosaccharide and short-chain fatty acid: a double-edged sword in obese mice by regulating food intake and fat synthesis. Food Res. Int. 159:111619. doi: 10.1016/j.foodres.2022.111619
Chen, Y. C., and Yu, Y. H. (2020). Bacillus licheniformis-fermented products improve growth performance and the fecal microbiota community in broilers. Poult. Sci. 99, 1432–1443. doi: 10.1016/j.psj.2019.10.061
Chikwanha, O. C., Emiliano, R., Opara, U. L., Fawole, O. A., Setati, M. E., Voster, M., et al. (2018). Impact of dehydration on retention of bioactive profile and biological activities of different grape (Vitis vinifera L.) pomace varieties. Anim. Feed Sci. Technol. 244, 116–127. doi: 10.1016/j.anifeedsci.2018.08.006
Córdova-Noboa, H. A., Oviedo-Rondón, E. O., Ortiz, A., Matta, Y., Hoyos, S., Buitrago, G. D., et al. (2020). Corn drying temperature, particle size, and amylase supplementation influence growth performance, digestive tract development, and nutrient utilization of broilers. Poult. Sci. 99, 5681–5696. doi: 10.1016/j.psj.2020.07.010
Courtin, C. M., Broekaert, W. F., Swennen, K., Lescroart, O., Onagbesan, O., Buyse, J., et al. (2008). Dietary inclusion of wheat bran arabinoxylooligosaccharides induces beneficial nutritional effects in chickens. Cereal Chem. J. 85, 607–613. doi: 10.1094/CCHEM-85-5-0607
Crovesy, L., Masterson, D., and Rosado, E. L. (2020). Profile of the gut microbiota of adults with obesity: a systematic review. Eur. J. Clin. Nutr. 74, 1251–1262. doi: 10.1038/s41430-020-0607-6
De Filippo, C., Cavalieri, D., Di Paola, M., Ramazzotti, M., Poullet, J. B., Massart, S., et al. (2010). Impact of diet in shaping gut microbiota revealed by a comparative study in children from Europe and rural Africa. Proc. Natl. Acad. Sci. U. S. A. 107, 14691–14696. doi: 10.1073/pnas.1005963107
Dev, K., Akbar Mir, N., Biswas, A., Kannoujia, J., Begum, J., and Kant, R. (2020). Dietary Mannan-oligosaccharides potentiate the beneficial effects of Bifidobacterium bifidum in broiler chicken. Lett. Appl. Microbiol. 71, 520–530. doi: 10.1111/lam.13360
Dhanalakshmi, S., Devi, R. S., Srikumar, R., Manikandan, S., and Thangaraj, R. (2007). Protective effect of Triphala on cold stress-induced behavioral and biochemical abnormalities in rats. Yakugaku Zasshi 127, 1863–1867. doi: 10.1248/yakushi.127.1863
Doré, J., and Blottière, H. (2015). The influence of diet on the gut microbiota and its consequences for health. Curr. Opin. Biotechnol. 32, 195–199. doi: 10.1016/j.copbio.2015.01.002
Engberg, R. M., Hedemann, M. S., Steenfeldt, S., and Jensen, B. B. (2004). Influence of whole wheat and xylanase on broiler performance and microbial composition and activity in the digestive tract. Poult. Sci. 83, 925–938. doi: 10.1093/ps/83.6.925
Feng, J., Liu, X., Xu, Z. R., Liu, Y. Y., and Lu, Y. P. (2007). Effects of aspergillus oryzae 3.042 fermented soybean meal on growth performance and plasma biochemical parameters in broilers. Anim. Feed Sci. Technol. 134, 235–242. doi: 10.1016/j.anifeedsci.2006.08.018
Fleckenstein-Elsen, M., Dinnies, D., Jelenik, T., Roden, M., Romacho, T., and Eckel, J. (2016). Eicosapentaenoic acid and arachidonic acid differentially regulate adipogenesis, acquisition of a brite phenotype and mitochondrial function in primary human adipocytes. Mol. Nutr. Food Res. 60, 2065–2075. doi: 10.1002/mnfr.201500892
Garron, M. L., and Henrissat, B. (2019). The continuing expansion of CAZymes and their families. Curr. Opin. Chem. Biol. 53, 82–87. doi: 10.1016/j.cbpa.2019.08.004
Gomes, K. M., Duarte, R. S., and de Freire Bastos, M. D. C. (2017). Lantibiotics produced by Actinobacteria and their potential applications (a review). Microbiology 163, 109–121. doi: 10.1099/mic.0.000397
Goodarzi, B. F., Senz, M., Kozłowski, K., Boros, D., Wisniewska, M., Rose, D., et al. (2017). The effects of fermentation and enzymatic treatment of pea on nutrient digestibility and growth performance of broilers. Animal 11, 1698–1707. doi: 10.1017/S1751731117000787
Guerrero, F., and Mullet, J. E. (1986). Increased abscisic acid biosynthesis during plant dehydration requires transcription. Plant Physiol. 80, 588–591. doi: 10.1104/pp.80.2.588
Heres, L., Engel, B., van Knapen, F., de Jong, M. C., Wagenaar, J. A., and Urlings, H. A. (2003). Fermented liquid feed reduces susceptibility of broilers for Salmonella enteritidis. Poult. Sci. 82, 603–611. doi: 10.1093/ps/82.4.603
Homma, H., and Shinohara, T. (2004). Effects of probiotic Bacillus cereus toyoi on abdominal fat accumulation in the Japanese quail (Coturnix japonica). Anim. Sci. J. 75, 37–41. doi: 10.1111/j.1740-0929.2004.00152.x
Jumas-Bilak, E., Roudière, L., and Marchandin, H. (2009). Description of ‘Synergistetes’ phyl. nov. and emended description of the phylum ‘Deferribacteres’ and of the family Syntrophomonadaceae, phylum ‘Firmicutes’. Int. J. Syst. Evol. Microbiol. 59, 1028–1035. doi: 10.1099/ijs.0.006718-0
Jumpertz, R., Le, D. S., Turnbaugh, P. J., Trinidad, C., Bogardus, C., Gordon, J. I., et al. (2011). Energy-balance studies reveal associations between gut microbes, caloric load, and nutrient absorption in humans. Am. J. Clin. Nutr. 94, 58–65. doi: 10.3945/ajcn.110.010132
Kim, M., and Voy, B. H. (2021). Fighting fat with fat: n-3 polyunsaturated fatty acids and adipose deposition in broiler chickens. Front. Physiol. 12:755317. doi: 10.3389/fphys.2021.755317
Koliada, A., Syzenko, G., Moseiko, V., Budovska, L., Puchkov, K., Perederiy, V., et al. (2017). Association between body mass index and Firmicutes/Bacteroidetes ratio in an adult Ukrainian population. BMC Microbiol. 17:120. doi: 10.1186/s12866-017-1027-1
Lee, S. D., Kim, H. Y., Jung, H. J., Ji, S. Y., Chowdappa, R., Ha, J. H., et al. (2009). The effect of fermented apple diet supplementation on the growth performance and meat quality in finishing pigs. Anim. Sci. J. 80, 79–84. doi: 10.1111/j.1740-0929.2008.00598.x
Liu, J., Yue, S., Yang, Z., Feng, W., Meng, X., Wang, A., et al. (2018). Oral hydroxysafflor yellow A reduces obesity in mice by modulating the gut microbiota and serum metabolism. Pharmacol. Res. 134, 40–50. doi: 10.1016/j.phrs.2018.05.012
Louis, P., and Flint, H. J. (2017). Formation of propionate and butyrate by the human colonic microbiota. Environ. Microbiol. 19, 29–41. doi: 10.1111/1462-2920.13589
Ma, Y., Wang, W., Zhang, H., Wang, J., Zhang, W., Gao, J., et al. (2018). Supplemental Bacillus subtilis DSM 32315 manipulates intestinal structure and microbial composition in broiler chickens. Sci. Rep. 8:15358. doi: 10.1038/s41598-018-33762-8
Macfarlane, G. T., and Macfarlane, S. (2012). Bacteria, colonic fermentation, and gastrointestinal health. J. AOAC Int. 95, 50–60. doi: 10.5740/jaoacint.SGE_Macfarlane
Massiera, F., Saint-Marc, P., Seydoux, J., Murata, T., Kobayashi, T., Narumiya, S., et al. (2003). Arachidonic acid and prostacyclin signaling promote adipose tissue development: a human health concern? J. Lipid Res. 44, 271–279. doi: 10.1194/jlr.M200346-JLR200
Milićević, D., Vranić, D., Mašić, Z., Parunović, N., Trbović, D., Nedeljković-Trailović, J., et al. (2014). The role of total fats, saturated/unsaturated fatty acids and cholesterol content in chicken meat as cardiovascular risk factors. Lipids Health Dis. 13:42. doi: 10.1186/1476-511X-13-42
Mott, C. R., Siegel, P. B., Webb, K. E. Jr., and Wong, E. A. (2008). Gene expression of nutrient transporters in the small intestine of chickens from lines divergently selected for high or low juvenile body weight. Poult. Sci. 87, 2215–2224. doi: 10.3382/ps.2008-00101
Ooi, L. G., and Liong, M. T. (2010). Cholesterol-lowering effects of probiotics and prebiotics: a review of in vivo and in vitro findings. Int. J. Mol. Sci. 11, 2499–2522. doi: 10.3390/ijms11062499
Rhead, M. M., Eglinton, G., Draffan, G. H., and England, P. J. (1971). Conversion of oleic acid to saturated fatty acids in Severn estuary sediments. Nature 232, 327–330.
Riera-Heredia, N., Lutfi, E., Sánchez-Moya, A., Gutiérrez, J., Capilla, E., and Navarro, I. (2020). Short-term responses to fatty acids on lipid metabolism and adipogenesis in rainbow trout (Oncorhynchus mykiss). Int. J. Mol. Sci. 21:1623. doi: 10.3390/ijms21051623
Ritz, C. W., Hulet, R. M., Self, B. B., and Denbow, D. M. (1995). Growth and intestinal morphology of male turkeys as influenced by dietary supplementation of amylase and xylanase. Poult. Sci. 74, 1329–1334. doi: 10.3382/ps.0741329
Rodas, B. Z., Sohn, K. S., Maxwell, C. V., and Spicer, L. J. (1995). Plasma protein for pigs weaned at 19 to 24 days of age: effect on performance and plasma insulin-like growth factor I, growth hormone, insulin, and glucose concentrations. J. Anim. Sci. 73, 3657–3665. doi: 10.2527/1995.73123657x
Rodríguez Muela, C., Rodríguez Ramírez, H. E., Díaz Plascencia, D., Bocourt Salabarría, R., and Arzola Álvarez, C. (2017). Concentration of acid detergent fiber, neutral detergent fiber and lignin during solid state fermentation of apple (Malus domestica) derivates. Cuban J. Agric. Sci. 51, 47–60.
Salaheen, S., Kim, S. W., Haley, B. J., Van Kessel, J. A. S., and Biswas, D. (2017). Alternative growth promoters modulate broiler gut microbiome and enhance body weight gain. Front. Microbiol. 8:2088. doi: 10.3389/fmicb.2017.02088
Scott, M. L, Nesheim, M. C, and Young, R. J. (1982). Nutrition of the Chicken [M]. New York: Scott and Associates.
Sugiharto, S., and Ranjitkar, S. (2019). Recent advances in fermented feeds towards improved broiler chicken performance, gastrointestinal tract microecology and immune responses: a review. Anim. Nutr. 5, 1–10. doi: 10.1016/j.aninu.2018.11.001
Sugiharto, S., Yudiarti, T., Isroli, I., Widiastuti, E., Wahyuni, H. I., and Sartono, T. A. (2020). Growth performance, haematological responses, intestinal microbiology and carcass traits of broiler chickens fed finisher diets containing two-stage fermented banana peel meal. Trop. Anim. Health Prod. 52, 1425–1433. doi: 10.1007/s11250-019-02147-y
Tang, G. Y., Zhao, C. N., Liu, Q., Feng, X. L., Xu, X. Y., Cao, S. Y., et al. (2018). Potential of grape wastes as a natural source of bioactive compounds. Molecules 23:2598. doi: 10.3390/molecules23102598
Tchorbanov, B., and Lazarova, G. (1988). Evaluation of protein hydrolysates using the fermentation activity of immobilized yeast cells. Biotechnol. Appl. Biochem. 10, 301–304. doi: 10.1111/j.1470-8744.1988.tb00021.x
Teixeira, T. F. S., Grześkowiak, L. M., Salminen, S., Laitinen, K., Bressan, J., and Gouveia, P. M. C. (2013). Faecal levels of bifidobacterium and Clostridium coccoides but not plasma lipopolysaccharide are inversely related to insulin and HOMA index in women. Clin. Nutr. 32, 1017–1022. doi: 10.1016/j.clnu.2013.02.008
Tie, Y., Li, L., Liu, J., Liu, C., Fu, J., Xiao, X., et al. (2020). Two-step biological approach for treatment of rapeseed meal. J. Food Sci. 85, 340–348. doi: 10.1111/1750-3841.15011
Van Soest, P. J., Robertson, J. B., and Lewis, B. A. (1991). Methods for dietary fiber, neutral detergent fiber, and nonstarch polysaccharides in relation to animal nutrition. J. Dairy Sci. 74, 3583–3597. doi: 10.3168/jds.S0022-0302(91)78551-2
Wu, ZK. (2018). Process optimization of solid-state fermentation of rapeseed meal with multi-strains and its feed value evaluation for broilers[D]. Master’s thesis, Beijing: Chinese Academy of Agricultural Sciences.
Wu, G., Bazer, F. W., Dai, Z., Li, D., Wang, J., and Wu, Z. (2014). Amino acid nutrition in animals: protein synthesis and beyond. Annu. Rev. Anim. Biosci. 2, 387–417. doi: 10.1146/annurev-animal-022513-114113
Wu, Q. J., Wang, Z. B., Wang, G. Y., Li, Y. X., and Qi, Y. X. (2015). Effects of feed supplemented with fermented pine needles (Pinus ponderosa) on growth performance and antioxidant status in broilers. Poult. Sci. 94, 1138–1144. doi: 10.3382/ps/pev013
Yan, W., Liu, X., Long, J., Wen, Q., and Zheng, Y. (2018). Effects of fermented corn gluten meal on growth performance, serum parameters, intestinal morphology and immunity performance of three-yellow broilers. Can. J. Anim. Sci. 99. doi: 10.1016/j.livsci.2019.103866
Yang, J., and Xiao, Y. Y. (2013). Grape phytochemicals and associated health benefits. Crit. Rev. Food Sci. Nutr. 53, 1202–1225. doi: 10.1080/10408398.2012.692408
Yang, L., Zeng, X., and Qiao, S. (2021). Advances in research on solid-state fermented feed and its utilization: the pioneer of private customization for intestinal microorganisms. Anim. Nutr. 7, 905–916. doi: 10.1016/j.aninu.2021.06.002
Yasar, S., and Yegen, K. M. (2017). Yeast fermented additive enhances broiler growth. Rev. Bras. Zootecn. 46, 814–820. doi: 10.1590/s1806-92902017001000004
Zhang, K. (2012). Study on the production of protein feed with bacterial enzymes and solid-state fermentation of miscellaneous meal[D]. Master’s thesis, Wuhan: Wuhan Institute of Technology.
Zhang, W., Ma, C., Xie, P., Zhu, Q., Wang, X., Yin, Y., et al. (2019). Gut microbiota of newborn piglets with intrauterine growth restriction have lower diversity and different taxonomic abundances. J. Appl. Microbiol. 127, 354–369. doi: 10.1111/jam.14304
Zhang, L., Wu, W., Lee, Y. K., Xie, J., and Zhang, H. (2018). Spatial heterogeneity and co-occurrence of mucosal and luminal microbiome across swine intestinal tract. Front. Microbiol. 26:48. doi: 10.3389/fmicb.2018.00048
Zhu, F., Du, B., Zheng, L., and Li, J. (2015). Advance on the bioactivity and potential applications of dietary fibre from grape pomace. Food Chem. 186, 207–212. doi: 10.1016/j.foodchem.2014.07.057
Keywords: fermented grape seed meal, yellow feather broilers, gut microbiota, growth performance, abdominal fat deposition
Citation: Nan S, Yao M, Zhang X, Wang H, Li J, Niu J, Chen C, Zhang W and Nie C (2022) Fermented grape seed meal promotes broiler growth and reduces abdominal fat deposition through intestinal microorganisms. Front. Microbiol. 13:994033. doi: 10.3389/fmicb.2022.994033
Edited by:
Junhua Liu, Nanjing Agricultural University, ChinaCopyright © 2022 Nan, Yao, Zhang, Wang, Li, Niu, Chen, Zhang and Nie. This is an open-access article distributed under the terms of the Creative Commons Attribution License (CC BY). The use, distribution or reproduction in other forums is permitted, provided the original author(s) and the copyright owner(s) are credited and that the original publication in this journal is cited, in accordance with accepted academic practice. No use, distribution or reproduction is permitted which does not comply with these terms.
*Correspondence: Cunxi Nie, bmllY3VueGlAc2luYS5jb20=
†These authors have contributed equally to this work