- 1Department of Animal Health and Antimicrobial Strategies, National Veterinary Institute (SVA), Uppsala, Sweden
- 2Department of Microbiology, Public Health Agency of Sweden, Solna, Sweden
- 3Department of Laboratory Medicine, Karolinska Institute, Stockholm, Sweden
- 4Department of Bacteriology, Host-Pathogen Interactions and Diagnostics Development, Wageningen Bioveterinary Research, Lelystad, Netherlands
- 5Department of Microbiology, National Veterinary Institute (SVA), Uppsala, Sweden
- 6Department of Chemistry, Environment and Feed Hygiene, National Veterinary Institute (SVA), Uppsala, Sweden
Occurrence of multidrug resistant Enterobacteriaceae in livestock is of concern as they can spread to humans. A potential introduction route for these bacteria to livestock could be animal feed. We therefore wanted to identify if Escherichia spp., Enterobacter spp., Klebsiella spp., or Raoutella spp. with transferable resistance to extended spectrum cephalosporins, carbapenems or colistin could be detected in the environment at feed mills in Sweden. A second aim was to compare detected isolates to previous described isolates from humans and animals in Sweden to establish relatedness which could indicate a potential transmission between sectors and feed mills as a source for antibiotic resistant bacteria. However, no isolates with transferable resistance to extended-cephalosporins or colistin could be identified, but one isolate belonging to the Enterobacter cloacae complex was shown to be carbapenem-resistant and showing carbapenemase-activity. Based on sequencing by both short-read Illumina and long-read Oxford Nanopore MinIon technologies it was shown that this isolate was an E. asburiae carrying a blaIMI-2 gene on a 216 Kbp plasmid, designated pSB89A/IMI-2, and contained the plasmid replicons IncFII, IncFIB, and a third replicon showing highest similarity to the IncFII(Yp). In addition, the plasmid contained genes for various functions such as plasmid segregation and stability, plasmid transfer and arsenical transport, but no additional antibiotic resistance genes. This isolate and the pSB89A/IMI-2 was compared to three human clinical isolates positive for blaIMI-2 available from the Swedish antibiotic monitoring program Swedres. It was shown that one of the human isolates carried a plasmid similar with regards to gene content to the pSB89A/IMI-2 except for the plasmid transfer system, but that the order of genes was different. The pSB89A/IMI-2 did however share the same transfer system as the blaIMI-2 carrying plasmids from the other two human isolates. The pSB89A/IMI-2 was also compared to previously published plasmids carrying blaIMI-2, but no identical plasmids could be identified. However, most shared part of the plasmid transfer system and DNA replication genes, and the blaIMI-2 gene was located next the transcription regulator imiR. The IS3-family insertion element downstream of imiR in the pSB89A was also related to the IS elements in other blaIMI-carrying plasmids.
Introduction
Antibiotic resistant bacteria are one of the major threats to modern human and animal health care worldwide. Of major concern is the increasing trends of extended spectrum beta-lactamases (ESBL) and, plasmid-mediated AmpC β-lactamase (pAmpC)-producing Enterobacteriaceae and carbapenem-resistant Enterobacteriaceae (CRE) in both humans and animals (Bezabih et al., 2020; Hansen, 2021). In addition, these bacteria also occur in the environment, including wildlife, but the occurrence appears mainly to mirror the one described in human populations (Guenther et al., 2011; Atterby et al., 2017). Due to increasing trends of human infections caused by multidrug-resistant CRE, the antibiotic colistin, previously used mainly in food-producing animals, has received renewed attention as a treatment of human infections (Falagas et al., 2011). Resistance to colistin was previously thought only to be connected to chromosomal mutations, and despite the relatively extensive use in livestock settings, the proportion of colistin-resistance among Enterobacteriaceae from healthy animals appeared to remain low (Kempf et al., 2013). However, this changed in 2015 when increasing trends of colistin-resistant Enterobacteriaceae due to a transferable colistin resistance gene mcr-1 was described in China (Liu et al., 2016). Since the description of mcr-1 an additional nine mcr-genes have been described and occurrence has been shown in humans, animals, and the environment worldwide (Elbediwi et al., 2019).
The occurrence of ESBL- and pAmpC-producing Enterobacteriaceae, CRE and Enterobacteriaceae with mcr-genes in animals, primarily livestock, is of concern as animal populations can function as reservoirs for occurrence in humans, and transmissions from animals to humans have been indicated (Börjesson et al., 2016; Madec et al., 2017). The environment may also be a reservoir and dissemination routes for these resistant bacteria (EFSA, 2021). There also exists a risk that new strains of ESBL-, pAmpC-producing Enterobacteriaceae and CRE can emerge in the environment and bacteria may acquire previously unknown genes encoding antibiotic resistance in the environment. For example, the blaCTX-M encoding ESBL and blaOXA-48 encoding carbapenemase appear to be a progeny from genes in the environmental bacteria Kluyvera spp. and Shewanella spp., respectively (Humeniuk et al., 2002; Tacão et al., 2018).
Despite Sweden having a relativity low incidence of ESBL-, pAmpC-producing Enterobacteriaceae and CRE, increasing trends have been shown in human setting (Swedres-Svarm, 2020). In companion animals and livestock, except for broilers and laying hens, prevalence of ESBL and pAmpC-producing E. coli has remained low, and no carbapenemase-producing Enterobacteriaceae (CPE) has been detected (Swedres-Svarm, 2020). Today, the occurrence of ESBL/pAmpC-producing E. coli in broilers is also low but there was a high occurrence in 2010 to 2017 linked to introduction by and transmission from contaminated imported breeding stock (Nilsson et al., 2020). Occurrence of mcr-genes in Sweden appears uncommon with only a handful human cases and no cases in animals, except for mcr-9 which has been identified in clinical colistin susceptible ESBL-producing Enterobacteriaceae isolates from horses (Börjesson et al., 2020; Swedres-Svarm, 2020).
A recent EFSA report (EFSA, 2021) highlighted that feed can be contaminated by a range of resistant bacteria and is therefore a potential route for introduction of antibiotic resistant bacteria to the livestock population. Furthermore, as demonstrated by Crump et al. (2002), colonization and infections of Enterobacteriaceae in food-producing animals and outbreaks of Salmonella in humans can also be traced back to contaminated feed. Particularly imported soy products have been pointed out as high-risk (Wierup, 2017). In Sweden feed materials with high protein content, such as soya, are mainly imported from European countries, e.g., Italy or third countries like Brazil, India and China (Statistics Sweden, 2022). As ESBL-, pAmpC-producing Enterobacteriaceae, CRE and Enterobacteriaceae with mcr-genes are commonly detected in different compartments in these geographical areas, import of feed products from there might represent a potential introduction route for these bacteria to the Swedish livestock population (Sun et al., 2017; Li et al., 2019; García-Betancur et al., 2021; Lalhruaipuii et al., 2021). However, studies about the role of feed in spreading transferable resistance to extended spectrum cephalosporins, carbapenems or colistin in Enterobacteriaceae in the feed chain is lacking.
The aim of the present study was to investigate if Escherichia spp., Enterobacter spp., Klebsiella spp., or Raoutella spp. with transferable resistance to extended cephalosporins, carbapenems or colistin could be detected in the environment of feed material intake in Swedish feed mills. Any identified strains were characterized genotypically and phenotypically, and their relatedness to previously described isolates from animal and human sectors in Sweden was thereafter investigated.
Materials and methods
Feed mills and sampling
The present study included all Swedish feed mills (n = 25) producing compound livestock feed in 2019. According to the Swedish Salmonella control program, feed mills are obliged to submit weekly environmental samples collected from predetermined sample points (National Veterinary Institute (SVA), 2022). The study utilized environmental samples from the feed material intake, pit or bottom part of the elevator for feed materials at the feed mills. Dust was collected with a swab (dry or wet) or scraped off, the sampling method varied between feed mills. During the autumn of 2019, four or five environmental samples from the feed material intake were collected with about a month apart, from each of the feed mills, and included in the current study. In total 113 environmental samples were included, and all samples were anonymized for this study.
Isolation and identification of carbapenem, third-generation cephalosporin and colistin resistant Escherichia spp., Enterobacter spp., Klebsiella spp., and Raoutella spp.
From each environmental sample 25 g material was collected and then diluted 1:10 in Buffered Pepton Water (BPW). The 25 g material and BPW was mixed with a spoon and incubated at 37°C for 18–20 h as shown in Figure 1. One ml of the pre-enrichment was then diluted 1:10 with Peptone salt water, and from this dilution 10 μl was transferred to a CHROMagar™ C3GR, CHROMagar™ mSuperCARBA™ and CHROMagar™ COL-APSE plate, respectively, and the agar plates were incubated at 37°C overnight. Suspected colonies were identified based on the colony morphology as described by the manufacturer, and sub-streaked on the same selective agar again. Bacterial species identification was performed using a Bruker Biotyper MALDI-TOF MS.
Phenotypic and genotypic characterization of isolated Escherichia spp., Enterobacter spp., Klebsiella spp., or Raoutella spp.
Escherichia spp. Klebsiella spp., or Raoutella spp. isolates growing on C3GR were subjected to multiplex-PCRs detecting the gene groups: pAmpC (Pérez-Pérez and Hanson, 2002), blaCTX-M (Woodford et al., 2005), blaSHV, blaTEM and blaOXA-1 (Fang et al., 2008), and Enterobacter spp. isolates were subjected to the same PCRs except for the pAmpC PCR. Isolates belonging to the same species growing on the COL-APSE were subjected to PCR detecting the genes mcr-1 to mcr-5 (Rebelo et al., 2018). Isolates from the mSuperCARBA were tested for carbapenemase-production using the RAPIDEC® CARBA NP (bioMérieux SA).
If shown to carry transferable genes encoding ESBL, AmpC, colistin resistance or had carbapenemase activity these were checked for antibiotic susceptibility using Sensititre™ EUVSEC microdilution panels (Thermo Fischer Scientific), and any isolate with reduced susceptibility to colistin were also re-tested using MICRONAUT MIC-Strip Colistin (Merlin Diagnostika) according to the recommendations of the manufacturers. Isolates were determined as resistant based on EUCASTs Epidemiological cut-off value (ECOFF) (EUCAST, 2022).
A single isolate designated SB89A was positive for carbapenemase-production and resistant to carbapenems and colistin and was therefore subjected to short-read sequencing. The isolate was cultured on horse blood agar from which bacterial material was collected and used for DNA extraction with the EZ1 DNA Tissue Kit (QIAGEN, Germany) according to the manufacturer’s protocol. DNA concentrations were determined using Qubit™ HS DNA Kit (Life Technologies) and the DNA was used for library preparation with the Illumina Nextera XT Kit (Illumina Inc) and 250-bp paired-end sequencing was performed on an Illumina MiSeq sequencer (Illumina Inc.). These reads were assembled and checked for plasmid replicons and resistance genes as described in Appendix 1, using Trimmomatic, SPAdes, Pilon, ARIBA with species checked with Kraken and rMLST, and assigned a sequence type (ST) using multi-locus sequence typing (MLST).
Plasmid characterization
The isolate SB89A which was shown to carry transferable genes encoding resistance to carbapenems was in addition to short-read sequencing also sequenced on an Oxford Nanopore MinIon, using the PCR barcoding kit SQK-LWB001 and a 9.4 MinION flowcell, producing reads with a median length of 2,686 bp. An initial hybrid assembly was performed with Unicycler (Wick et al., 2017) and annotated with Prokka (Seemann, 2014) which showed that the blaIMI-2 gene was located on a plasmid. However, this assembly did not produce a circularized sequence (details in Appendix 1). Plasmid DNA from SB89A isolate was therefore purified and transformed into a E. coli DH10B selecting for ertapenem resistance before the molecular size was determined using S1-restricted pulsed-field gel electrophoresis as previously described (Liakopoulos et al., 2016). Long-read sequencing on a MinION™ Flowcell FLO-MIN106D and using a Ligation sequencing kit SQK-LSK109 was performed for the wild-type isolate and the transformant E. coli, producing reads with a median length of 7,718 bp.
Long reads from the transformant and the previous short reads were used to create the second hybrid assembly with Flye (Kolmogorov et al., 2019) and Pilon (Walker et al., 2014). This assembly was typed with PlasmidFinder (Camacho et al., 2009; Carattoli et al., 2014) and annotated with Prokka (Seemann, 2014). Predicted protein sequences were also searched against the NCBI non-redundant protein (nr) database with blastp. The assembly was compared to previously characterized blaIMI-2 plasmids (Accession numbers KX868552, KY680213, and CP033468) and a blaIMI-3 carrying plasmid (KT780723) through sequence alignment and visualization with Mauve (Darling et al., 2004). Segments that had no similarity to these plasmids were searched against the NCBI nucleotide collection (nr/nt) database using blastn (Altschul et al., 1990). The plasmid was also analyzed with PHASTER (Arndt et al., 2016) to identify potential prophages. Analysis details including program versions and parameters are listed in Appendix 1.
Comparison to isolates from other sources in Sweden
The Public health agency of Sweden (PHAS), previously the Swedish Institute for Communicable Disease Control, has since 2007 collected and verified all Enterobacteriaceae suspected of producing carbapenemases, and since 2016 all isolates have been genome sequenced using IonTorrent™ (Swedres-Svarm, 2020). As of 2010 the National Veterinary Institute (SVA) screens all samples collected from livestock for ESBL, pAmpc and carbapenemase producing E. coli within Svarm, and Swedish veterinary laboratories are encouraged to submit presumptive ESBL, pAmpC-producing and colistin-resistant Enterobacteriaceae and CRE for verification and characterisation at SVA. From these different collections three human clinical isolates were identified to be positive for the same gene encoding carbapenemase as the SB89A isolate. The identified isolates were reassembled using CLC Assembly cell, and the assemblies used for species identification with rMLST and MLST detection. Further, the assembled contigs were aligned towards the recovered plasmid from SB89A using minimap 2 with asm10 settings (Li, 2018). The alignment in SAM-format was then sorted, converted to bam-files, and matching contigs were extracted into fasta-format, using samtools, for downstream BRIG visualization (Li et al., 2009). The whole assembly was also analyzed using Platon and BAKTA to identify blaIMI-2 in each set of contig collections as well as confirm their classification as plasmids (Schwengers et al., 2020, 2021). These three isolates were then subjected long-read sequencing on a MinION™ R9.4.1 with libraries prepared using the SQK-RKB004 kit and Rapid Barcoding Kit SQK-RBK110.96, producing reads with a median length of 5,200 bp. Reads from Nanopore sequencing were assembled using a long-read only approach, using the pipeline trycycler as described in Appendix 1. Following this, the plasmid sequences were then extracted and typed, annotated and compared to pSB89A/IMI-2 as described in “Plasmid characterization.”
Results
Collected isolates and verification of phenotype and genotype
Out of the 113 collected samples growth was recorded on 117 C3G, 4 mSuperCARBA and 93 COL-APSE agar plates. In total, 466 isolates were collected from the agar-plates, 260 from C3GR, 6 from mSuperCARBA and 200 from COL-APSE. After species identification 235 isolates belonging to the investigated species were selected for further characterization: 143 from C3GR, 1 from mSuperCARBA and 91 from COL-APSE.
The most common identified genus was Enterobacter spp. (n = 201), followed by Escherichia spp. (n = 20) and Klebsiella spp. (n = 13) as shown in Table 1. The most common typed species from all the three different types of plates were the E. cloacae complex, 139 from C3GR, 1 from mSuperCARBA and 61 from COL-APSE (Table 1).
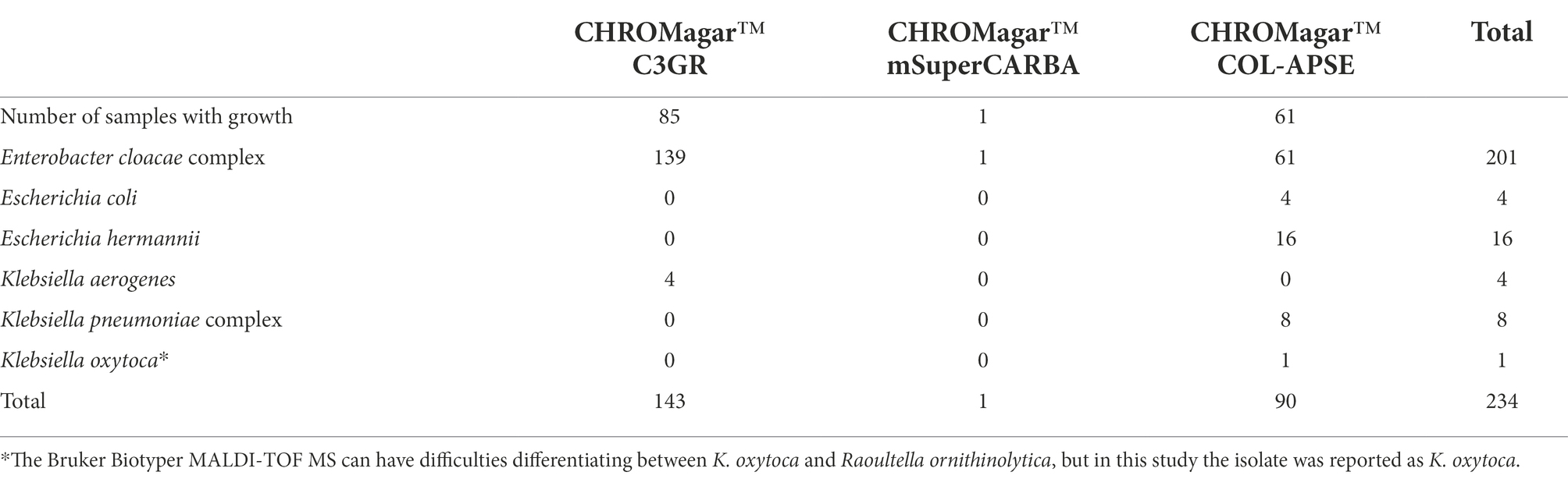
Table 1. Number of feed-mill samples with growth of investigated bacteria genera and identified bacterial species from the three agar plates.
All isolates from the C3GR and COL-APSE agar plates were negative for the investigated transferable resistance genes. The only isolate designated SB89A from the mSuperCARBA plate belonged to the E. cloacae complex and was shown to have a weak carbapenemase reaction on the CARBA-NP test. This isolate was resistant to cefepime, ertapenem, imipenem and meropenem, but was susceptible to ceftazidime and cefotaxime (Table 2). It was also resistant to colistin and was therefore re-tested using the MICRONAUT MIC-Strip Colistin and was determined to have a MIC of 64 μg/ml but skipped wells in the microdilution plate were also recorded and it was therefore retested three times, see Table 3.
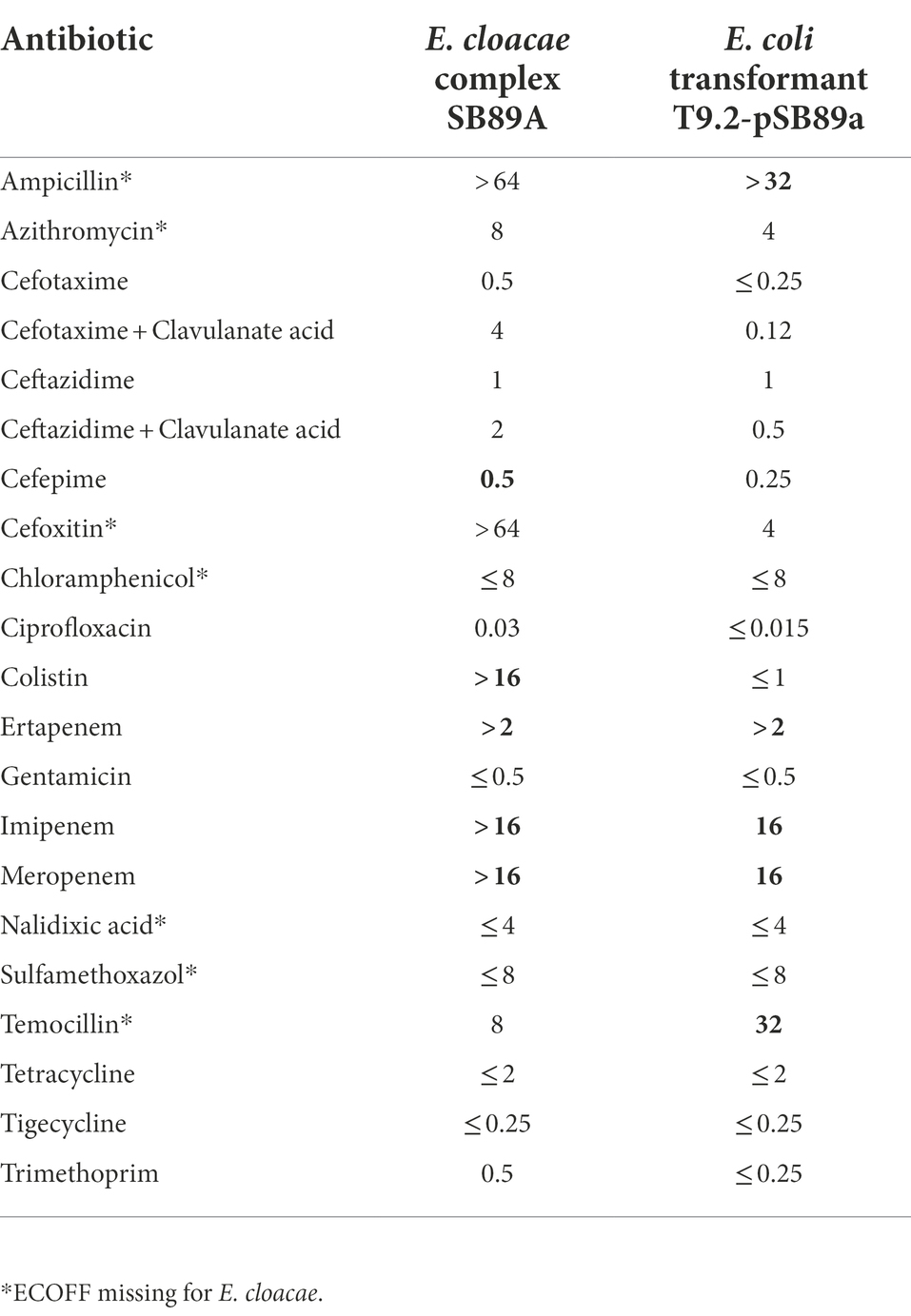
Table 2. Antibiotic Minimum inhibitory concentrations (MICs), μg/ml, for the E. cloacae complex SB89A isolate using Sensititre™ EUVSEC and EUVSEC2 panels and E. coli transformant T9.2-pSB89a using EUVSEC3 and EUVSEC2 panels. Bold MIC indicates MIC above ECOFF for E. cloace or E. coli
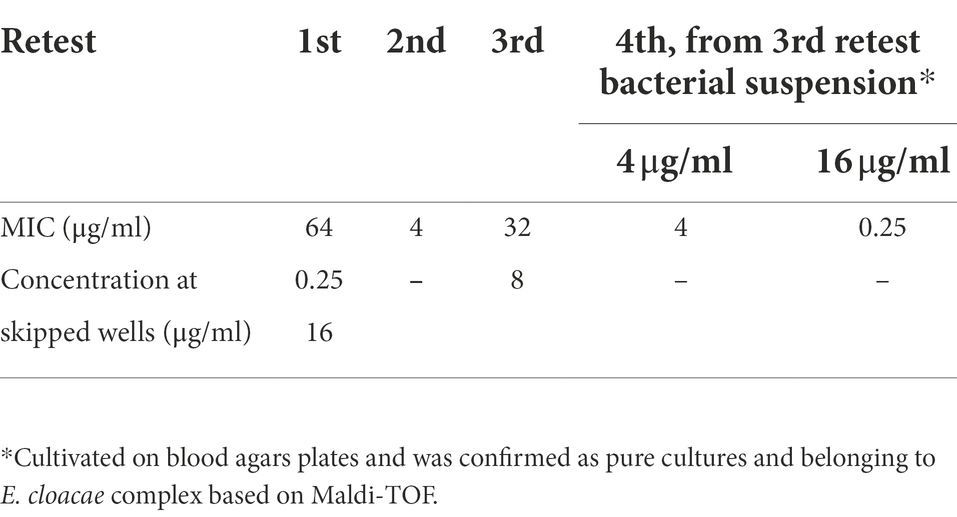
Table 3. Colistin MICs (μg/ml) for the E. cloacae complex SB89A isolate using MICRONAUT MIC-strip Colistin and skipped wells.
Genotypic characterization of the SB89A isolate
The E. cloacae complex isolate SB89A was determined to be an E. asburiae carrying the blaIMI-2 gene, which encodes a carbapenemase, on a plasmid. The isolate was also positive for the intrinsic blaACT gene, the fosA gene conferring resistance to fosfomycin, and oqxB part of the OqxAB efflux pump which has been linked to reduced susceptibility to fluoroquinolones. In the SB89A the plasmid replicons IncFIB and IncFII were detected and based on the 7-MLST scheme for E. cloacae it belonged to ST657.
Characterization of the plasmid carrying the blaIMI-2 gene
As initial hybrid assemblies of the Illumina and MinION data using different parameters resulted in potential plasmids of different sizes, PFGE of the wildtype SB89A isolate and an ertapenem-resistant E. coli transformant were therefore compared. While the SB89A isolate contained three independent plasmids, approximate 50, 150, and > 200 kb in sizes, the transformant only contained a single plasmid of approximately 200 kb in size, which validated the assembly of a 216,086 bp circular plasmid containing both IncFII(pECLA; 97.46% identity) and IncFIB (pENTAS01; 99.11% identity) replicons, here after referred to as pSB89A/IMI-2. In addition to the identified IncFII and IncFIB it contained a likely third replicon which showed 93.45% identity to IncFII(Yp). The phenotype of the E. coli transformant was confirmed using broth microdilution, showing that acquisition of the plasmid led to resistance against ertapenem, imipenem, meropenem, and ampicillin (Table 2).
On pSB89A/IMI-2 the blaIMI-2 gene was associated with the lysR-like transcription regulator imiR and several complete and partial Insertion Sequence (IS) elements (Figure 2). The plasmid also contains genes for various functions such as plasmid segregation and stability (parA, parB-like, parM, xerC, xerD, sopA, sopB, klcA), DNA replication (umuC, umuD, dinB, repA, repB, repE-like), plasmid transfer (23 tra genes and three trb genes) and arsenical transport (arsA, arsB, arsC, arsD, and arsR2). The plasmid also contained the plasmid stability system relBE/stbDE, but the parED1 toxin/antitoxin system was also identified. Furthermore, the plasmid contained the asnO genes which encodes asparagine synthetase, bioF which encodes 8-amino-7-oxononanoate synthase, and the tuaB encoding teichuronic acid synthesis a gram-positive cell wall component.
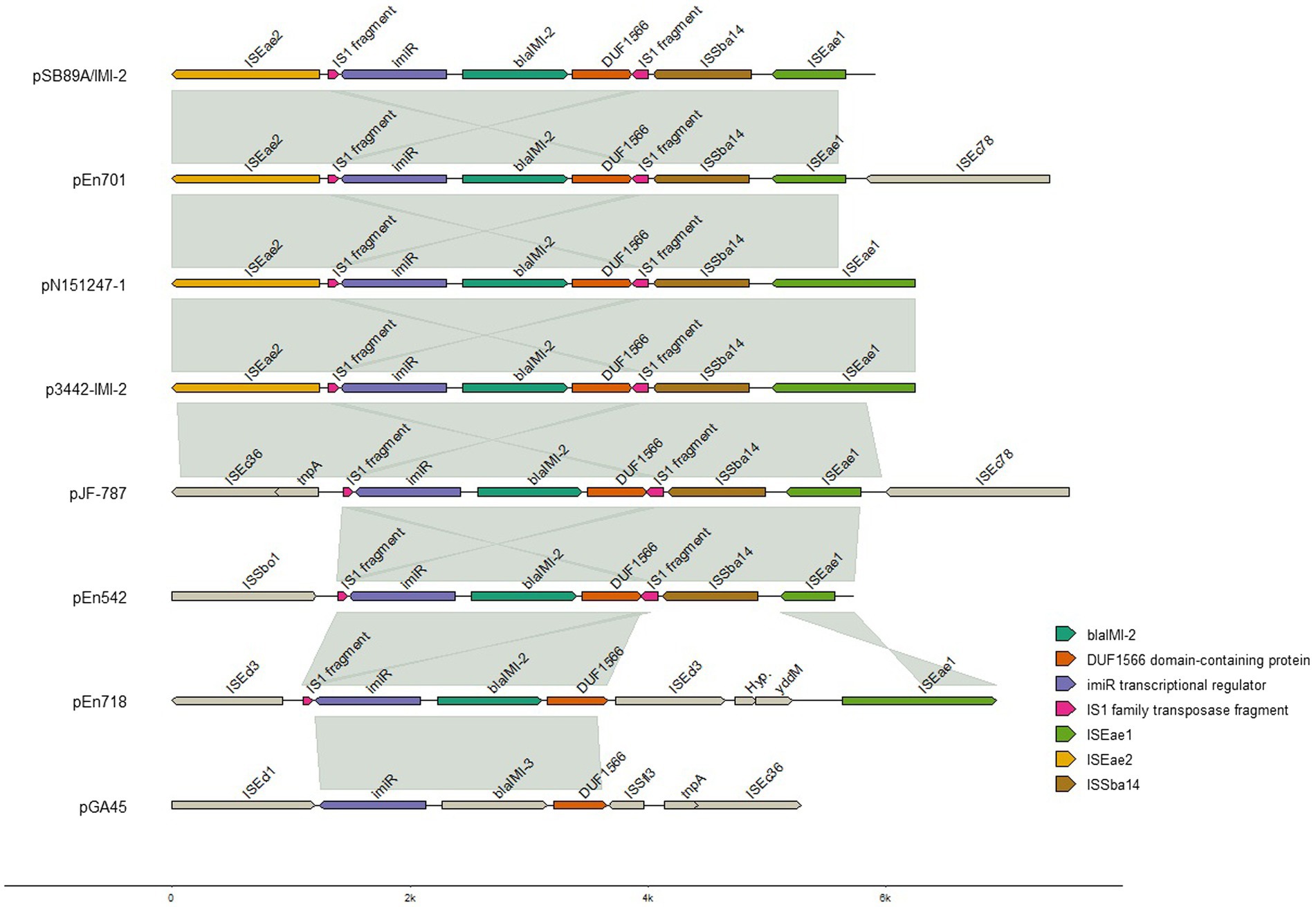
Figure 2. Alignment of blaIMI-2 genes and adjacent insertion elements. The aligned segments connected with gray lines shows 96.98–99.98% sequence identity, with the exception of p3442-IMI-2 and pJF-787 which showed 92.1% sequence identity. Figure created with genomes in R. For visualization purposes the sequences of pSB89A/IMI-2, pN151247-1 and p3442-IMI-2 have been inverted. The pGAA45 which carries a blaIMI-3 gene is also included for comparisons, as for the pSB89A/IMI-2, pN151247-1 and p3442-IMI-2 the sequence of pGAA45 have been inverted.
PHASTER also identified a complete prophage (NC_049919) as part of the pSB89A/IMI-2 which spanned a region including the blaIMI-2 and the tehA gene which confers tellurite resistance (Figure 3). The pSB89A did not carry any additional genes encoding antibiotic resistance.
When comparing the pSB89A/IMI-2 with published blaIMI-2 plasmids it was shown that they shared the type IV secretion system for plasmid transfer as well as the composition of imiR and IS element genes surrounding blaIMI-2 (Table 4; Figures 2, 3).The ISEae2 element downstream of imiR is an IS3-family insertion element similar to the ISEc36 element described in other IMI-carrying plasmids (Dang et al., 2016). Upstream of the imiR and blaIMI-2 genes is a DUF1566 domain that codes for a protein of unknown function, and truncated sequences of transposases ISSba14 and ISEae1 which are also present in most of the compared plasmids (Figure 2). In two of the plasmids, pN151247-1 and p3442-IMI-2, the ISEae1 sequence appears to be mostly complete (Figure 2). There are also small fragments of an IS1 family transposase present near blaIMI-2 in all compared plasmids. The pSB89A/IMI-2 plasmid also shared the DNA replication genes umuC and umuD with three of the compared plasmids. However, there are several sequence segments not shared among these plasmids including one containing an arsenical transport system, repA, repB plasmid segregation genes and IS elements flanked by Tn7 transposons and one containing another arsenical transport system flanked by Tn3 transposons. However, some of these sequence segments showed similarity to p48880_VIM_4 (CP059418), a mcr-9 and blaVIM-4 carrying IncHI2 plasmid (Bitar et al., 2020). When comparing the pSB89A/IMI-2 with a plasmid carrying the blaIMI-3 (pGA45) it was shown that it also had the DUF1566 domain-containing protein but shared none of the IS elements in the imiR – blaIMI region with pSB89A/IMI-2 (Figure 2). It did however have an ISEc36 IS-element present in the pJF-787 and highly similar in sequence to ISEae2.
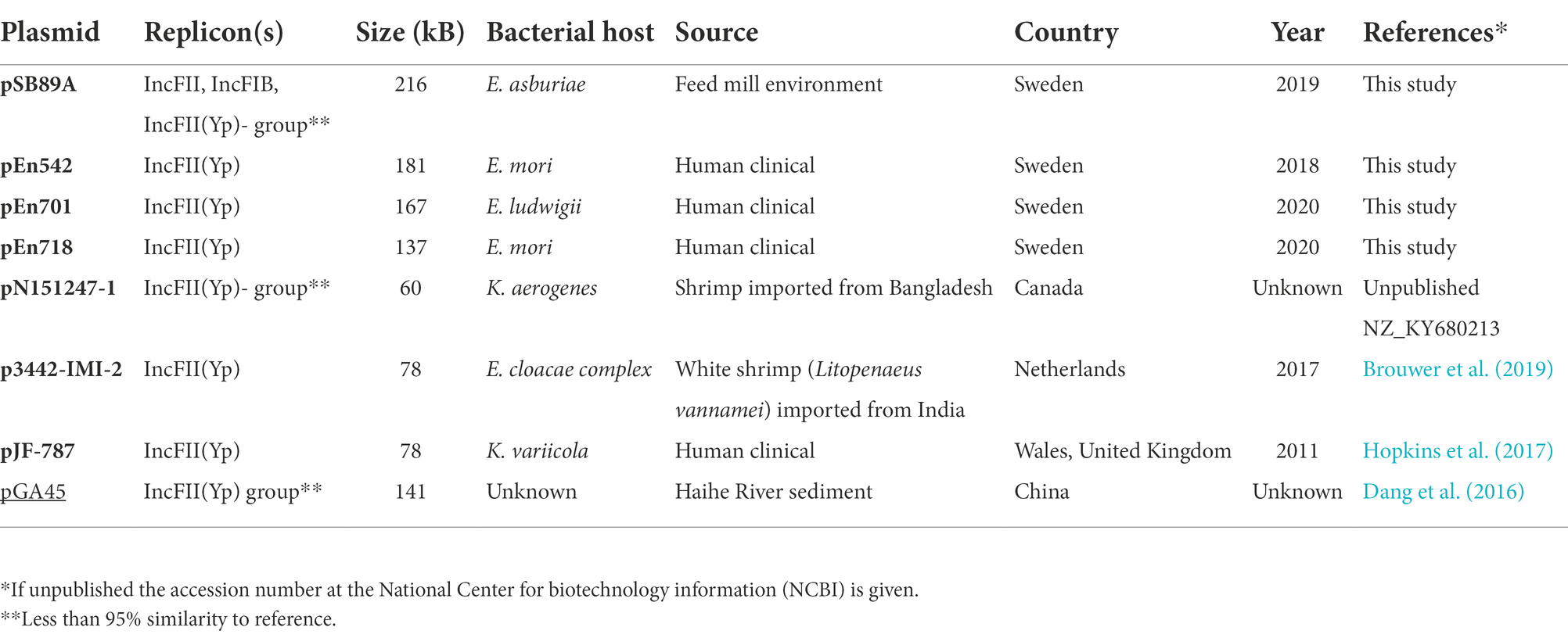
Table 4. Overview on plasmids sequenced in the current study and previously published plasmids containing blaIMI-2 (bold) and blaIMI-3 (underlined).
Genotypic comparison of SB89A and pSB89A/IMI-2 with human clinical isolates
Out of the 830 Enterobacteriaceae verified to carry a gene encoding carbapenemases at PHAS from 2015 to 2020 only seven isolates were identified to carry a gene belonging to the blaIMI group, and out of these only three isolates were identified to carry the blaIMI-2 gene. The first human clinical isolate carrying a blaIMI-2 was identified in 2018 and was an E. mori (Isolate ID En542) and the remaining two isolates were identified in 2020 and being an E. ludwigii (En701) and an E. mori (En718). All three isolates from humans carried the blaIMI-2 gene on an IncFII(Yp) plasmid of sizes 181,051 bp, 167,344 bp and 137,148 bp, respectively (Table 4). These were compared to pSB89A/IMI-2 (Figures 2, 3). In addition to blaIMI-2, all plasmids shared the imiR gene except for the plasmid in En701 (pEn701) they were overall dissimilar. However, the plasmids identified in En542 (pEn542) and En718 (pEn718) did share the same transfer system as pSB89A/IMI-2, see Figure 3. The pEn701 was highly similar in gene content and it contained both the relBE/stbDE and parED1 toxins/antitoxins, but the content was arranged in a different order compared to the pSB89A/IMI-2. The IS elements surrounding blaIMI-2 in pEn701 were identical to pSB89A/IMI-2 with the exception that pEn701 also contains the ISEc78 (Figure 2). The other two showed less similarity with the pEn542 in only sharing the upstream IS elements with the pEn718 only sharing the closest sequences and an inverted ISEae1. The pEn701 did also belong to a different replicon type IncFII(Yp) and the plasmid transfer system was dissimilar. A putative prophage spanning the area including blaIMI-2 was identified on the pEn701 which shared the blaIMI-2 region and prophages specific genes with the prophage identified in the pSB89A/IMI-2 plasmid, but regions before and after the blaIMI-2 region were dissimilar (Figure 3).
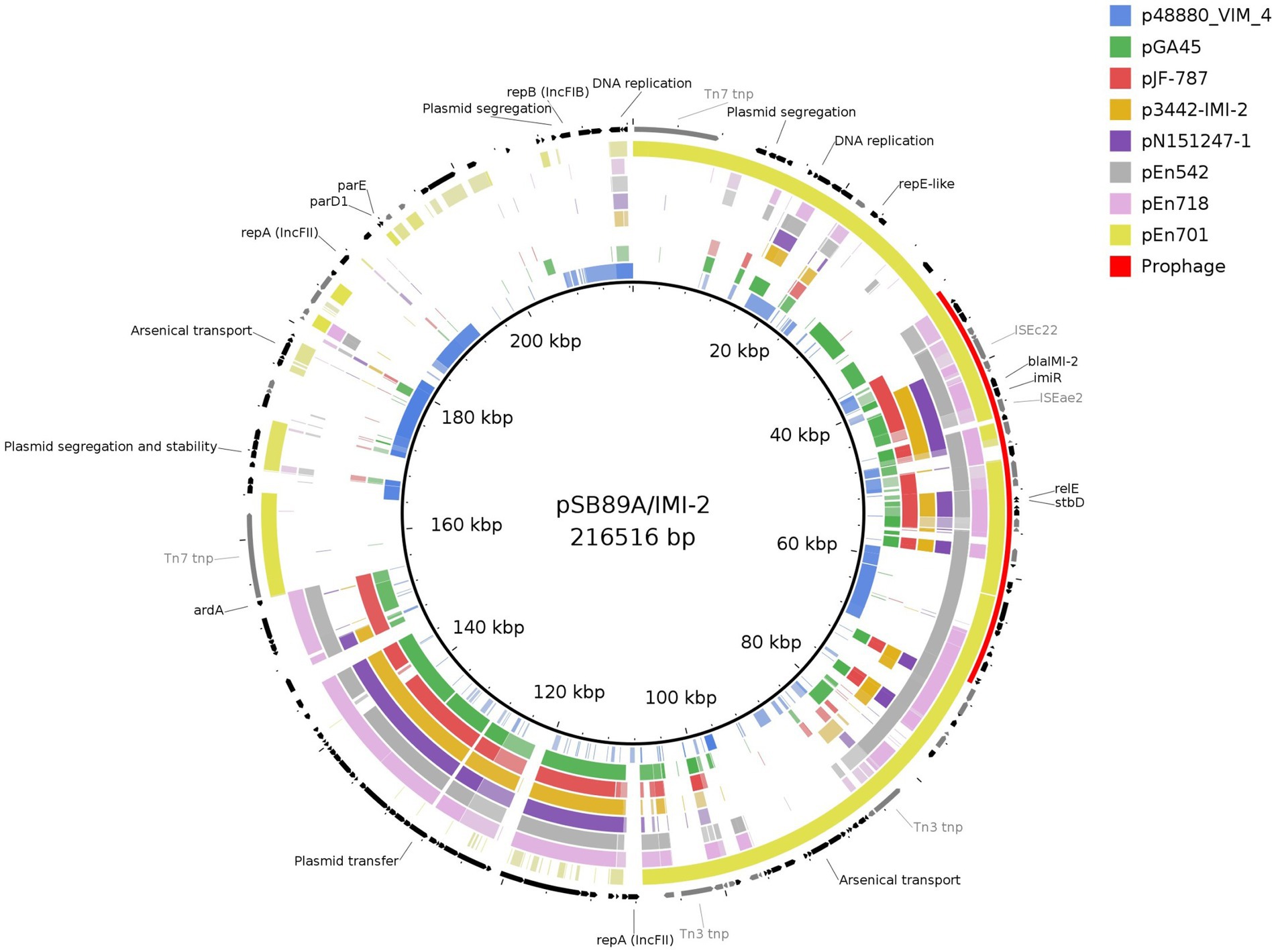
Figure 3. Comparison of pSBA89A/IMI-2 to three blaIMI-2 carrying plasmids from human clinical samples sequenced in this study, and to previously published plasmids of which three contains blaIMI-2 (KX868552, CP033468, and KY680213), one containing blaIMI-3 (KT780723), and one negative for blaIMI-genes (CP059418). Genes are shown in black and IS elements in gray. Figure created with BRIG.
Discussion
The current study showed that Enterobacteriaceae with transferable resistance to extended cephalosporins, carbapenems and colistin are uncommon in the environment of Swedish feed mills, with only a single E. asburiae carrying the blaIMI-2 gene isolated. However, there was growth observed of the investigated genera on the plates selective for extended-spectrum cephalosporin resistance (C3GR) and for colistin resistance (COL-APSE). This was not unexpected on the C3GR agar as it is designed not to inhibit growth of AmpC beta-lactamase producing strains and that the investigated genera can all carry intrinsic genes encoding beta-lactamase activity which can facilitate growth. In fact, the most commonly detected genus among the false-negatives were Enterobacter spp. (Table 1) which all carry different AmpC intrinsically (Jacoby, 2009). Furthermore, it was not also unanticipated to identify false-negative growth on the COL-APSE either, as Enterobacter spp. also can show reduced susceptibility to colistin, which was also observed for the E. asburiae carrying the blaIMI-2 gene isolated in the current study (Landman et al., 2013; Band et al., 2016; Hong et al., 2018). Resistance to colistin and extended-spectrum cephalosporin among the investigated genera might also been influenced by chromosomal mutations which were not investigated. In addition, the false-negative growth might have been influenced by growth by bacteria (data not shown) commonly occurring in the environment, which are intrinsically resistant to the investigated antibiotics (Cantón, 2009; Cox and Weight, 2013; Gogry et al., 2021).
This is to our knowledge the first time a carbapenem resistant Enterobacteriaceae has been described in a feed mill and the first description of such a bacterium in an environment linked to animals in Sweden. When reviewing the available literature, Enterobacteriaceae with the blaIMI gene group appear uncommon both among humans and domesticated animals globally, and the same is seen in Sweden where only seven human cases so far has been reported (Swedres-Svarm, 2020; Bonnin et al., 2021). Nevertheless, the blaIMI-1 was described already in 1996 in two E. cloacae from patients in US, with the oldest isolate being from 1984, and since then, 21 additional variants have been described (Rasmussen et al., 1996; Bonnin et al., 2021; National Library of Medicine (NIH), 2021). As in this study, most previous studies have detected the blaIMI genes in isolates mainly belonging to the E. cloacae complex (Bonnin et al., 2021). In addition, most descriptions are related to the outer environment and not clinical isolates, and primarily from or connected to aqueous environments. In fact, the blaIMI-2 was also described in 1996 in an E. asburiae isolated from a US river and, as in this study, the gene was located on a transferable plasmid (Aubron et al., 2005). Three additional blaIMI-2 E. asburiae isolates from three distant rivers were identified in the study, and since then, the blaIMI-2 gene has been described in E. cloacae complex isolates from, Spanish river sediments and vannamei white shrimp (Litopenaeus vannamei) originating from India (Piedra-Carrasco et al., 2017; Brouwer et al., 2019). Additionally, human clinical cases with E. cloaace complex carrying the blaIMI-2 have been reported from Czech Republic (Rotova et al., 2017), China (Yun-Song et al., 2006), Austria (Hartl et al., 2019) and South Africa (Gqunta et al., 2015). In the case report from Austria it was also reported that the infection was developed after a thermal bath visit. In addition, a recent French study also described a case related to a near-drowning in a river (Laurens et al., 2018). That study was also able to isolate E. asburiae with a blaIMI-2 from the river water in which the near-drowning had occurred and showed that these isolates were closely related to the isolates from the patient. The blaIMI-2 in E. cloacae complex appears therefore mainly linked, directly or indirectly, to environmental samples and appears uncommon in clinical settings. Thus, it is likely that the environment is the natural reservoir for these bacteria and the mobile elements carrying the blaIMI-2. In our study the blaIMI-2 isolate from the feed mill sample was compared with two E. mori and one E. ludwigii carrying blaIMI-2 from human clinical cases, but there was no information available about any environmental connection for these patients. However, as blaIMI-2 is located on transferable plasmids there is still a risk that this gene could be transferred to other species, or strains of E. cloacae, more prone to cause infections and spread in clinical settings, although this appears to happen very infrequently and the reason for this remains to be further elucidated. Plasmids with blaIMI-2 have nonetheless been identified in clinical E. coli isolates in Spain and China, in Klebsiella variicola in the United Kingdom and in Pseudomonas aeruginosa in Spain (Rojo-Bezares et al., 2012; Hopkins et al., 2017; Zhang et al., 2017; Cabrera et al., 2022).
In the current study the blaIMI-2 was located on plasmids belonging to the IncFII-family in all four investigated isolates. This mirrors the results of earlier published studies where the blaIMI-2 appears exclusively linked to IncFII plasmids, primarily IncFII(Yp), including those where the blaIMI-2 has been identified in isolates belonging to the E. cloacae complex species and in other Enterobacteriaceae (Rojo-Bezares et al., 2012; Hopkins et al., 2017; Piedra-Carrasco et al., 2017; Zhang et al., 2017; Brouwer et al., 2019). We therefore compared the pSB89A/IMI-2 plasmid identified in this study to earlier published plasmids and although it was shown that they shared some similar traits, the IncFII-plasmids varied greatly in both gene content and size (Figures 2, 3; Table 4). The same was true when comparing the pSB89A/IMI-2 to the plasmids identified in the three Swedish human clinical isolates. In contrast to the other plasmids the pSB89A/IMI-2 also contained multiple IncF-replicons, was the largest in size and it also contained sequence segments which showed similarity to an IncHI2 plasmid p48880_VIM-4 carrying a mcr-9 and blaVIM-4 (Figure 3). However, the ISEae1, or a truncated version of it, was identified on all investigated blaIMI-2 plasmids in the blaIMI-2-imiR region and all had the toxin/antitoxins relBE/stbDE (Figures 2, 3). It was also shown that all four plasmids sequenced in this study had a variety of different genes which could possibly give the isolates fitness advantages in different environmental settings such as genes encoding amino acid productions, arsenic and metal resistance, but any function by these genes needs to be further investigated. The pSB89A/IMI-2 and the pEn701 showed the greatest similarity with most overlapping genes including that both carried the parED1 toxin/antitoxin, in addition to the relBE/stbDE (Figures 2, 3). These two plasmids also had an almost identical blaIMI-2-imiR region with an identical ISEae2 identified, thus indicating a potential common origin (Figure 2). Of the previously published plasmids two also had highly similar blaIMI-2-imiR regions to pSB89A/IMI-2 and pEn701, with the difference being primarily in the ISEae1 (Figure 2). Interestingly, both of these plasmids originated from South-Asia and were isolated from shrimps (Table 4). However, no information was available if the patient or the positive feed mill had any contact with South Asia. The blaIMI-2-imiR regions in the remaining plasmids showed higher dissimilarity and did not carry the ISEae2 (Figure 2). The pGA45 and the blaIMI-2 plasmid pJF-787 did have an ISEc36 which is related to the ISEae2 present in most other blaIMI-2 plasmids, with the exception of the pEn542 and pEn718. So it is possible that the ISEae2 family could play a role in the transposition of blaIMI genes, and the study describing the pJF-787 also suggested that ISEc36 could have an essential role in the dissemination of blaIMI genes (Dang et al., 2016). ISEae1was not present in pGA45 which is present in all blaIMI-2 plasmids included in the current study, so this insertion sequence likely plays an essential role in understanding the dissemination of the blaIMI-2. Unfortunately, not much appears known about this IS except its connection to the E. cloace complex and that it appears to be first detected in this complex.1 In Sweden, ISEae1 has also been identified in connection with a pathogenicity island in a group B Salmonella enterica isolated from porpoise (Phocoena phocoena) (Sandholt et al., 2021).
The blaIMI-2-imiR region in pSB89A/IMI-2 and pEn701 was also located on a putative prophage. The same prophage was also identified in this study in the plasmid, p3442-IMI-2, isolated in Netherlands from an Indian vannamei white shrimp (Brouwer et al., 2019). Interestingly, similar prophages have previously been linked with the Shiga toxin Stx1 in E. coli (Ogura et al., 2015). In addition, the tehA gene which previously has been identified in the chromosome of E. coli was also found on the prophages in this study (Taylor et al., 1994; Turner et al., 1997). These results can indicate that prophages may play a role in the spread of blaIMI genes in the natural environment. Although not extensively studied it has been shown that transduction can play a role in the dissemination of genes encoding resistance (Colavecchio et al., 2017; Kondo et al., 2021; Wendling et al., 2021). In the case of STEC/EHECs prophages play an important role in the transfer and expression of the stx-genes, but they also transfer other virulence factors, and have been described to increases fitness of the host (Bondy-Denomy and Davidson, 2014; Wendling et al., 2021). Despite the blaIMI-2 gene being both connected to prophages and transferable plasmids spread to other bacterial species than the E. cloacae complex isolates appears infrequent, as previously discussed. The exact reason for the limited transmission beyond environmental E. cloacae complex strains of the blaIMI-2 gene and its corresponding IncFII-plasmids needs to be elucidated. However, as most other narrow-hosts IncF-plasmids they are likely dependent on both host-encoded and self-encoded factors for replication and the toxin/antitoxin systems also likely influences the limited dissemination (Kopotsa et al., 2019; Fraikin et al., 2020).
In addition to being a CRE due to the blaIMI-2 the E. asburiae isolate from the feed mill also displayed a hetero-phenotype for colistin. The isolate was shown on several retests being sometimes resistant and sometimes susceptible to colistin, with a skip-well phenotype also being observed (Table 3). However, this sort of hetero-phenotype has previously been described in E. cloacae complex isolates and is likely related to a subpopulation with an increased expression of arnB and eptA (Landman et al., 2013; Band et al., 2016; Hong et al., 2018). These two genes are responsible for the modification of lipid A, the molecular target of polymyxins, and were also detected in the chromosome of the SB89A isolate. As both arnB and eptA are located on the chromosome and are not transferable, this phenotype was therefore not further investigated in the current study.
Even though uncommon, the present study shows that the CRE occurs in the environment of feed mills and therefore feed materials may be a potential source for introduction of CREs to livestock and humans. The impact of antibiotic resistant bacteria in feed materials is likely reduced by the heat treatment that most Swedish compound feed for livestock is subjected to. However, it has been described that heat treatment performed on feed does not eliminate all bacteria (Cox et al., 1983) and occurrence of antibiotic resistant Enterobacteriaceae has been described in processed feed (da Costa et al., 2007; Fraiture et al., 2021). Since feed cannot be excluded as a route of introduction it ought to be considered as a material to screen for antibiotic resistant bacteria.
Conclusion
Screening of the environmental samples from the feed material intake at Swedish feed mills revealed that transferable resistance to extended spectrum cephalosporins, carbapenems or colistin has a very low prevalence. A single carbapenemase producing E. asburiae was isolated during the study and was shown to encode a blaIMI-2 carbapenemase gene on an IncFII plasmid that had similarities, but no direct molecular epidemiological links, to blaIMI-2 encoding plasmids from Swedish human clinical and global clinical and environmental isolates could be established. Thus, this and other studies indicate that the environment may be a reservoir for the blaIMI-2 gene and that this gene is present on a diverse set of IncF-plasmids.
Data availability statement
The datasets presented in this study can be found in online repositories. The names of the repository/repositories and accession number(s) can be found at: https://www.ebi.ac.uk/ena, PRJEB53023.
Author contributions
SB, JoE, and LE conceptualized the study with all authors contributing to the final design. SB, MB, JoE, and LE secured funding for the project. SB, MB, and JeE performed the laboratory work, with SB, MB, EÖ, and OK performing the bioinformatic analyses. SB, MB, EÖ, OK, and LE were responsible for the data curation. SB wrote the first draft of the manuscript after which all authors contributed text. SB and LE finalised the manuscript. All authors contributed to the article and approved the submitted version.
Funding
This work was conducted in the framework of the Full Force project, supported by funding from the European Union’s Horizon 2020 Research and Innovation program under grant agreement no 773830: One Health European Joint Programme. In addition, internal funds were used at the National Veterinary Institute (SVA) in Sweden.
Acknowledgments
Joakim Skarin, Tobias Lilja, Magnus Thelander, Boel Harbom, Mattias Haukland, Karin Westmo, and Arie Kant are acknowledged for their assistance on the project.
Conflict of interest
The authors declare that the research was conducted in the absence of any commercial or financial relationships that could be construed as a potential conflict of interest.
Publisher’s note
All claims expressed in this article are solely those of the authors and do not necessarily represent those of their affiliated organizations, or those of the publisher, the editors and the reviewers. Any product that may be evaluated in this article, or claim that may be made by its manufacturer, is not guaranteed or endorsed by the publisher.
Supplementary material
The Supplementary material for this article can be found online at: https://www.frontiersin.org/articles/10.3389/fmicb.2022.993454/full#supplementary-material
Footnotes
References
Altschul, S. F., Gish, W., Miller, W., Myers, E. W., and Lipman, D. J. (1990). Basic local alignment search tool. J. Mol. Biol. 215, 403–410. doi: 10.1016/S0022-2836(05)80360-2
Arndt, D., Grant, J. R., Marcu, A., Sajed, T., Pon, A., Liang, Y., et al. (2016). PHASTER: a better, faster version of the PHAST phage search tool. Nucleic Acids Res. 44, W16–W21. doi: 10.1093/nar/gkw387
Atterby, C., Börjesson, S., Ny, S., Järhult, J. D., Byfors, S., and Bonnedahl, J. (2017). ESBL-producing Escherichia coli in Swedish gulls—a case of environmental pollution from humans? PLoS One 12:e0190380. doi: 10.1371/journal.pone.0190380
Aubron, C., Poirel, L., Ash, R. J., and Nordmann, P. (2005). Carbapenemase-producing Enterobacteriaceae, U.S. rivers. Emerg. Infect. Dis. 11, 260–264. doi: 10.3201/eid1102.030684
Band, V. I., Crispell, E. K., Napier, B. A., Herrera, C. M., Tharp, G. K., Vavikolanu, K., et al. (2016). Antibiotic failure mediated by a resistant subpopulation in Enterobacter cloacae. Nat. Microbiol. 1:16053. doi: 10.1038/nmicrobiol.2016.53
Bezabih, Y. M., Sabiiti, W., Alamneh, E., Bezabih, A., Peterson, G. M., Bezabhe, W. M., et al. (2020). The global prevalence and trend of human intestinal carriage of ESBL-producing Escherichia coli in the community. J. Antimicrob. Chemother. 76, 22–29. doi: 10.1093/jac/dkaa399
Bitar, I., Papagiannitsis, C. C., Kraftova, L., Chudejova, K., Mattioni, M. V., and Hrabak, J. (2020). Detection of five mcr-9-carrying Enterobacterales isolates in four Czech hospitals. mSphere 5:e01008-20. doi: 10.1128/mSphere.01008-20
Bondy-Denomy, J., and Davidson, A. R. (2014). When a virus is not a parasite: the beneficial effects of prophages on bacterial fitness. J. Microbiol. 52, 235–242. doi: 10.1007/s12275-014-4083-3
Bonnin, R. A., Jousset, A. B., Emeraud, C., Oueslati, S., Dortet, L., and Naas, T. (2021). Genetic diversity, biochemical properties, and detection methods of minor Carbapenemases in Enterobacterales. Front. Med. 7:616490. doi: 10.3389/fmed.2020.616490
Börjesson, S., Greko, C., Myrenås, M., Landén, A., Nilsson, O., and Pedersen, K. (2020). A link between the newly described colistin resistance gene mcr-9 and clinical Enterobacteriaceae isolates carrying blaSHV-12 from horses in Sweden. J. Glob. Antimicrob. Resist. 20, 285–289. doi: 10.1016/j.jgar.2019.08.007
Börjesson, S., Ny, S., Egervärn, M., Bergström, J., Rosengren, Å., Englund, S., et al. (2016). Limited dissemination of extended-Spectrum β-lactamase– and plasmid-encoded AmpC–producing Escherichia coli from food and farm animals, Sweden. Emerg. Infect. Dis. J. 22, 634–640. doi: 10.3201/eid2204.151142
Brouwer, M. S. M., Tehrani Kamaleddin, H. M. E., Rapallini, M., Geurts, Y., Kant, A., Harders, F., et al. (2019). Novel Carbapenemases FLC-1 and IMI-2 encoded by an Enterobacter cloacae Complex isolated from food products. Antimicrob. Agents Chemother. 63, e02338–e02318. doi: 10.1128/AAC.02338-18
Cabrera, R., Fernández-Barat, F., Vázquez, N., Alcaraz-Serrano, V., Bueno-Freire, L., Amaro, R., et al. (2022). Resistance mechanisms and molecular epidemiology of Pseudomonas aeruginosa strains from patients with bronchiectasis. J. Antimicrob. Chemother. 77, 1600–1610. doi: 10.1093/jac/dkac084
Camacho, C., Coulouris, G., Avagyan, V., Ma, N., Papadopoulos, J., Bealer, K., et al. (2009). BLAST+: architecture and applications. BMC Bioinform. 10:421. doi: 10.1186/1471-2105-10-421
Cantón, R. (2009). Antibiotic resistance genes from the environment: a perspective through newly identified antibiotic resistance mechanisms in the clinical setting. Clin. Microbiol. Infect. 15, 20–25. doi: 10.1111/j.1469-0691.2008.02679.x
Carattoli, A., Zankari, E., García-Fernández, A., Larsen, M. V., Lund, O., Villa, L., et al. (2014). In Silico detection and typing of plasmids using plasmid finder and plasmid multilocus sequence typing. Antimicrob. Agents Chemother. 58, 3895–3903. doi: 10.1128/AAC.02412-14
Colavecchio, A., Cadieux, B., Lo, A., and Goodridge, L. D. (2017). Bacteriophages contribute to the spread of antibiotic resistance genes among foodborne pathogens of the Enterobacteriaceae Family–a review. Front. Microbiol. 8:1108. doi: 10.3389/fmicb.2017.01108
Cox, N. A., Bailey, J. S., Thomson, J. E., and Juven, B. J. (1983). Salmonella and other Enterobacteriaceae found in commercial poultry feed. Poult. Sci. 62, 2169–2175. doi: 10.3382/ps.0622169
Cox, G., and Weight, G. D. (2013). Intrinsic antibiotic resistance: mechanisms, origins, challenges and solutions. Int. J. Med. Microbiol. 303, 287–292. doi: 10.1016/j.ijmm.2013.02.009
Crump, J. A., Griffin, P. M., and Angulo, F. J. (2002). Bacterial contamination of animal feed and its relationship to human foodborne illness. Clin. Infect. Dis. 35, 859–865. doi: 10.1086/342885
da Costa, P. M., Oliveira, M., Bica, A., Vaz-Pires, P., and Bernardo, F. (2007). Antimicrobial resistance in Enterococcus spp. and Escherichia coli isolated from poultry feed and feed ingredients. Vet. Microbiol. 120, 122–131. doi: 10.1016/j.vetmic.2006.10.005
Dang, B., Mao, D., and Luo, Y. (2016). Complete nucleotide sequence of pGA45, a 140,698-bp Inc FIIY plasmid encoding Bla IMI-3-mediated Carbapenem resistance, from river sediment. Front. Microbiol. 7:188. doi: 10.3389/fmicb.2016.00188
Darling, A. C. E., Mau, B., Blattner, F. R., and Perna, N. T. (2004). Mauve: multiple alignment of conserved genomic sequence with rearrangements. Genome Res. 14, 1394–1403. doi: 10.1101/gr.2289704
EFSA (2021). Role played by the environment in the emergence and spread of antimicrobial resistance (AMR) through the food chain. EFSA J. 19:e06651. doi: 10.2903/j.efsa.2021.6651
Elbediwi, M., Li, Y., Paudyal, N., Pan, H., Li, X., Xie, S., et al. (2019). Global burden of Colistin-resistant bacteria: mobilized Colistin resistance genes study (1980–2018). Microorganisms 7:461. doi: 10.3390/microorganisms7100461
European Committee on Antimicrobial Susceptibility Testing (EUCAST) (2022). Data From the EUCAST MIC Distribution Website [online]. Sweden: European Committee on Antimicrobial Susceptibility Testing.
Falagas, M. E., Karageorgopoulos, D. E., and Nordmann, P. (2011). Therapeutic options for infections with Enterobacteriaceae producing carbapenem-hydrolyzing enzymes. Future Microbiol. 6, 653–666. doi: 10.2217/fmb.11.49
Fang, H., Ataker, F., Hedin, G., and Dornbusch, K. (2008). Molecular epidemiology of extended-Spectrum β-lactamases among Escherichia coli isolates collected in a Swedish hospital and its associated health care facilities from 2001 to 2006. J. Clin. Microbiol. 46, 707–712. doi: 10.1128/JCM.01943-07
Fraikin, N., Goormaghtigh, F., Van Melderen, L., and Margolin, W. (2020). Type II toxin-antitoxin systems: evolution and revolutions. J. Bacteriol. 202, e00763–e00719. doi: 10.1128/JB.00763-19
Fraiture, M.-A., Joly, L., Vandermassen, E., Delvoye, M., Van Geel, D., Michelet, J.-Y., et al. (2021). Retrospective survey of unauthorized genetically modified bacteria harbouring antimicrobial resistance genes in feed additive vitamin B2 commercialized in Belgium: challenges and solutions. Food Control 119:107476. doi: 10.1016/j.foodcont.2020.107476
García-Betancur, J. C., Appel, T. M., Esparza, G., Gales, A. C., Levy-Hara, G., Cornistein, W., et al. (2021). Update on the epidemiology of carbapenemases in Latin America and the Caribbean. Expert Rev. Anti-Infect. Ther. 19, 197–213. doi: 10.1080/14787210.2020.1813023
Gogry, D. A., Siddiqui, M. R., Sultan, I., and Haq, Q. M. R. (2021). Current update on intrinsic and acquired Colistin resistance mechanisms in bacteria. Front. Med. 8:677720. doi: 10.3389/fmed.2021.677720
Gqunta, K., van Wyk, J., Ekermans, P., Bamford, C., Moodley, C., and Govender, S. (2015). First report of an IMI-2 carbapenemase-producing Enterobacter asburiae clinical isolate in South Africa. South. Afr. J. Infect. Dis. 30, 34–35. doi: 10.1080/23120053.2015.1103963
Guenther, S., Ewers, C., and Wieler, L. (2011). Extended-Spectrum Beta-lactamases producing E. coli in wildlife, yet another form of environmental pollution? Front. Microbiol. 2:246. doi: 10.3389/fmicb.2011.00246
Hansen, G. T. (2021). Continuous evolution: perspective on the epidemiology of Carbapenemase resistance among Enterobacterales and other gram-negative bacteria. Infect. Dis. Ther. 10, 75–92. doi: 10.1007/s40121-020-00395-2
Hartl, R., Kerschner, H., Gattringer, R., Lepuschitz, S., Allerberger, F., Sorschag, S., et al. (2019). Whole-genome analysis of a human Enterobacter mori isolate carrying a Bla IMI-2 Carbapenemase in Austria. Microb. Drug Resist. 25, 94–96. doi: 10.1089/mdr.2018.0098
Hong, Y.-K., Lee, J.-Y., and Ko, K. S. (2018). Colistin resistance in Enterobacter spp. isolates in Korea. J. Microbiol. 56, 435–440. doi: 10.1007/s12275-018-7449-0
Hopkins, K. L., Findlay, J., Doumith, M., Mather, B., Meunier, D., D’Arcy, S., et al. (2017). IMI-2 carbapenemase in a clinical Klebsiella variicola isolated in the UK. J. Antimicrob. Chemother. 72, 2129–2131. doi: 10.1093/jac/dkx103
Humeniuk, C., Arlet, G., Gautier, V., Grimont, P., Labia, R., and Philippon, A. (2002). β-Lactamases of Kluyvera ascorbata, probable progenitors of some plasmid-encoded CTX-M types. Antimicrob. Agents Chemother. 46, 3045–3049. doi: 10.1128/AAC.46.9.3045-3049.2002
Jacoby, G. A. (2009). AmpC beta-lactamases. Clin. Microbiol. Rev. 22, 161–182. doi: 10.1128/CMR.00036-08
Kempf, I., Fleury, M. A., Drider, D., Bruneau, M., Sanders, P., Chauvin, C., et al. (2013). What do we know about resistance to colistin in Enterobacteriaceae in avian and pig production in Europe? Int. J. Antimicrob. Agents 42, 379–383. doi: 10.1016/j.ijantimicag.2013.06.012
Kolmogorov, M., Yuan, J., Lin, Y., and Pevzner, P. A. (2019). Assembly of long, error-prone reads using repeat graphs. Nat. Biotechnol. 37, 540–546. doi: 10.1038/s41587-019-0072-8
Kondo, K., Kawano, M., Sugai, M., and Castanheira, M. (2021). Distribution of antimicrobial resistance and virulence genes within the Prophage-associated regions in nosocomial pathogens. mSphere 6, e00452–e00421. doi: 10.1128/mSphere.00452-21
Kopotsa, K., Osei Sekyere, J., and Mbelle, N. M. (2019). Plasmid evolution in carbapenemase-producing Enterobacteriaceae: a review. Ann. N. Y. Acad. Sci. 1457, 61–91. doi: 10.1111/nyas.14223
Lalhruaipuii, K., Dutta, T. K., Roychoudhury, P., Chakraborty, S., Subudhi, P. K., Samanta, I., et al. (2021). Multidrug-resistant extended-Spectrum β-lactamase-producing Escherichia coli Pathotypes in north eastern region of India: backyard small ruminants-human-water interface. Microb. Drug Resist. 27, 1664–1671. doi: 10.1089/mdr.2020.0365
Landman, D., Salamera, J., and Quale, J. (2013). Irreproducible and Uninterpretable Polymyxin B MICs for Enterobacter cloacae and Enterobacter aerogenes. J. Clin. Microbiol. 51, 4106–4111. doi: 10.1128/JCM.02129-13
Laurens, C., Jean-Pierre, H., Licznar-Fajardo, P., Hantova, S., Godreuil, S., Martinez, O., et al. (2018). Transmission of IMI-2 carbapenemase-producing Enterobacteriaceae from river water to human. J. Glob. Antimicrob. Resist. 15, 88–92. doi: 10.1016/j.jgar.2018.06.022
Li, H. (2018). Minimap2: pairwise alignment for nucleotide sequences. Bioinformatics 34, 3094–3100. doi: 10.1093/bioinformatics/bty191
Li, J., Bi, Z., Ma, S., Chen, B., Cai, C., He, J., et al. (2019). Inter-host transmission of Carbapenemase-producing Escherichia coli among humans and backyard animals. Environ. Health Perspect. 127:107009. doi: 10.1289/EHP5251
Li, H., Handsaker, B., Wysoker, A., Fennell, T., Ruan, J., Homer, N., et al. (2009). The sequence alignment/map format and SAMtools. Bioinformatics 25, 2078–2079. doi: 10.1093/bioinformatics/btp352
Liakopoulos, A., Geurts, Y., Dierikx, C. M., Brouwer, M. S., Kant, A., Wit, B., et al. (2009). Extended-spectrum cephalosporin-resistant Salmonella enterica serovar Heidelberg strains, the Netherlands. Emerg. Infect. Dis. 22, 1257–1261. doi: 10.3201/eid2207.151377
Liu, Y.-Y., Wang, Y., Walsh, T. R., Yi, L.-X., Zhang, R., Spencer, J., et al. (2016). Emergence of plasmid-mediated colistin resistance mechanism MCR-1 in animals and human beings in China: a microbiological and molecular biological study. Lancet Infect. Dis. 16, 161–168. doi: 10.1016/S1473-3099(15)00424-7
Madec, J. Y., Haenni, M., Nordmann, P., and Poirel, L. (2017). Extended-spectrum β-lactamase/AmpC-and carbapenemase-producing Enterobacteriaceae in animals: a threat for humans? Clin. Microbiol. Infect. 23, 826–833. doi: 10.1016/j.cmi.2017.01.013
National Library of Medicine (NIH) (2021). Reference gene catalog [online]. Available at: https://www.ncbi.nlm.nih.gov/pathogens/refgene. (Accessed December 12, 2021).
National Veterinary Institute (SVA) (2022). Available at [Online]: www.sva.se/en/our-topics/feed-safety/general-facts-about-salmonella/salmonella-control-surveillance/. (Accessed August 23, 2022).
Nilsson, O., Börjesson, S., Landén, A., Greko, C., and Bengtsson, B. (2020). Decreased detection of ESBL-or pAmpC-producing Escherichia coli in broiler breeders imported into Sweden. Acta Vet. Scand. 62:33. doi: 10.1186/s13028-020-00532-4
Ogura, Y., Mondal, S. I., Islam, M. R., Mako, T., Arisawa, K., Katsura, K., et al. (2015). The Shiga toxin 2 production level in enterohemorrhagic Escherichia coli O157:H7 is correlated with the subtypes of toxin-encoding phage. Sci. Rep. 5:16663. doi: 10.1038/srep16663
Pérez-Pérez, F. J., and Hanson, N. D. (2002). Detection of plasmid-mediated AmpC β-lactamase genes in clinical isolates by using multiplex PCR. J. Clin. Microbiol. 40, 2153–2162. doi: 10.1128/JCM.40.6.2153-2162.2002
Piedra-Carrasco, N., Fàbrega, A., Calero-Cáceres, W., Cornejo-Sánchez, T., Brown-Jaque, M., Mir-Cros, A., et al. (2017). Carbapenemase-producing enterobacteriaceae recovered from a Spanish river ecosystem. PLoS One 12:e0175246. doi: 10.1371/journal.pone.0175246
Rasmussen, B. A., Bush, K., Keeney, D., Yang, Y., Hare, R., O'Gara, C., et al. (1996). Characterization of IMI-1 beta-lactamase, a class a carbapenem-hydrolyzing enzyme from Enterobacter cloacae. Antimicrob. Agents Chemother. 40, 2080–2086. doi: 10.1128/AAC.40.9.2080
Rebelo, A. R., Bortolaia, V., Kjeldgaard, J. S., Pedersen, S. K., Leekitcharoenphon, P., Hansen, I. M., et al. (2018). Multiplex PCR for detection of plasmid-mediated colistin resistance determinants, mcr-1, mcr-2, mcr-3, mcr-4 and mcr-5 for surveillance purposes. Eur. Secur. 23, 17–00672. doi: 10.2807/1560-7917.ES.2018.23.6.17-00672
Rojo-Bezares, B., Martín, C., López, M., Torres, C., and Sáenz, Y. (2012). First detection of blaIMI-2 gene in a clinical Escherichia coli strain. Antimicrob. Agents Chemother. 56, 1146–1147. doi: 10.1128/AAC.05478-11
Rotova, V., Papagiannitsis, C. C., Chudejova, K., Medvecky, M., Skalova, A., Adamkova, V., et al. (2017). First description of the emergence of Enterobacter asburiae producing IMI-2 carbapenemase in the Czech Republic. J. Glob. Antimicrob. Resist. 11, 98–99. doi: 10.1016/j.jgar.2017.10.001
Sandholt, A. K. S., Neimanis, A., Roos, A., Eriksson, J., and Söderlund, R. (2021). Genomic signatures of host adaptation in group B salmonella enterica ST416/ST417 from harbour porpoises. BMC Vet. Res. 52:134. doi: 10.1186/s13567-021-01001-0
Schwengers, O., Barth, P., Falgenhauer, L., Hain, T., Chakraborty, T., and Goesmann, A. (2020). Platon: identification and characterization of bacterial plasmid contigs in short-read draft assemblies exploiting protein sequence-based replicon distribution scores. Microbial. Genomics 6. doi: 10.1099/mgen.0.000398
Schwengers, O., Jelonek, L., Dieckmann, M. A., Beyvers, S., Blom, J., and Goesmann, A. (2021). Bakta: rapid and standardized annotation of bacterial genomes via alignment-free sequence identification. Microb. Genom. 7. doi: 10.1099/mgen.0.000685
Seemann, T. (2014). Prokka: rapid prokaryotic genome annotation. Bioinformatics 30, 2068–2069. doi: 10.1093/bioinformatics/btu153
Statistics Sweden (2022). Foreign trade – Exports and imports of goods [online]. Available at: www.scb.se (Accessed March 7, 2022).
Sun, P., Bi, Z., Nilsson, M., Zheng, B., Berglund, B., Stålsby Lundborg, C., et al. (2017). Occurrence of blaKPC-2, blaCTX-M, and mcr-1 in Enterobacteriaceae from well water in rural China. Antimicrob. Agents Chemother. 61, e02569–e02516. doi: 10.1128/AAC.02569-16
Swedres-Svarm (Ed). (2020). Sales of Antibiotics and Occurrence of Antibiotic Resistance in Sweden. Swedres-Svarm: Solna/Uppsala.
Tacão, M., Araújo, S., Vendas, M., Alves, A., and Henriques, I. (2018). Shewanella species as the origin of Bla(OXA-48) genes: insights into gene diversity, associated phenotypes and possible transfer mechanisms. Int. J. Antimicrob. Agents 51, 340–348. doi: 10.1016/j.ijantimicag.2017.05.014
Taylor, D. E., Hou, Y., Turner, R. J., and Weiner, J. H. (1994). Location of a potassium tellurite resistance operon (tehA tehB) within the terminus of Escherichia coli K-12. J. Bacteriol. 176, 2740–2742. doi: 10.1128/jb.176.9.2740-2742.1994
Turner, R. J., Taylor, D. E., and Weiner, J. H. (1997). Expression of Escherichia coli TehA gives resistance to antiseptics and disinfectants similar to that conferred by multidrug resistance efflux pumps. Antimicrob. Agents Chemother. 41, 440–444. doi: 10.1128/aac.41.2.440
Walker, B. J., Abeel, T., Shea, T., Priest, M., Abouelliel, A., Sakthikumar, S., et al. (2014). Pilon: an integrated tool for comprehensive microbial variant detection and genome assembly improvement. PLoS One 9:e112963. doi: 10.1371/journal.pone.0112963
Wendling, C. C., Refardt, D., and Hall, A. R. (2021). Fitness benefits to bacteria of carrying prophages and prophage-encoded antibiotic-resistance genes peak in different environments. Evolution 75, 515–528. doi: 10.1111/evo.14153
Wick, R. R., Judd, L. M., Gorrie, C. L., and Holt, K. E. (2017). Unicycler: resolving bacterial genome assemblies from short and long sequencing reads. PLoS Comput. Biol. 13:e1005595. doi: 10.1371/journal.pcbi.1005595
Wierup, M. (2017). “Production of soybean-derived feed material free from salmonella contamination: an essential food safety challenge” in Soybean-the Basis of Yield Biomass and Productivity. ed. M. Kasai (London: INTECH), 187–213.
Woodford, N., Fagan, E. J., and Ellington, M. J. (2005). Multiplex PCR for rapid detection of genes encoding CTX-M extended-spectrum β-lactamases. J. Antimicrob. Chemother. 57, 154–155. doi: 10.1093/jac/dki412
Yun-Song, Y., Xiao-Xing, D., Zhi-Hui, Z., Ya-Gang, C., and Lan-Juan, L. (2006). First isolation of blaIMI-2 in an Enterobacter cloacae clinical isolate from China. Antimicrob. Agents Chemother. 50, 1610–1611. doi: 10.1128/AAC.50.4.1610-1611.2006
Keywords: antimicrobial resistance, plasmid, carbapenem resistance, blaIMI-2, environment, Enterobacter cloacae complex, clinical isolates
Citation: Börjesson S, Brouwer MSM, Östlund E, Eriksson J, Elving J, Karlsson Lindsjö O and Engblom LI (2022) Detection of an IMI-2 carbapenemase-producing Enterobacter asburiae at a Swedish feed mill. Front. Microbiol. 13:993454. doi: 10.3389/fmicb.2022.993454
Edited by:
Yi-Tsung Lin, Taipei Veterans General Hospital, TaiwanReviewed by:
Milena Dropa, University of São Paulo, BrazilMahmoud Mohamed Fayez, Veterinary Serum and Vaccine Research Institute, Egypt
Copyright © 2022 Börjesson, Brouwer, Östlund, Eriksson, Elving, Karlsson Lindsjö and Engblom. This is an open-access article distributed under the terms of the Creative Commons Attribution License (CC BY). The use, distribution or reproduction in other forums is permitted, provided the original author(s) and the copyright owner(s) are credited and that the original publication in this journal is cited, in accordance with accepted academic practice. No use, distribution or reproduction is permitted which does not comply with these terms.
*Correspondence: Stefan Börjesson, c3RlZmFuLmJvcmplc3NvbkBmb2xraGFsc29teW5kaWdoZXRlbi5zZQ==
†These authors have contributed equally to this work and share second authorship