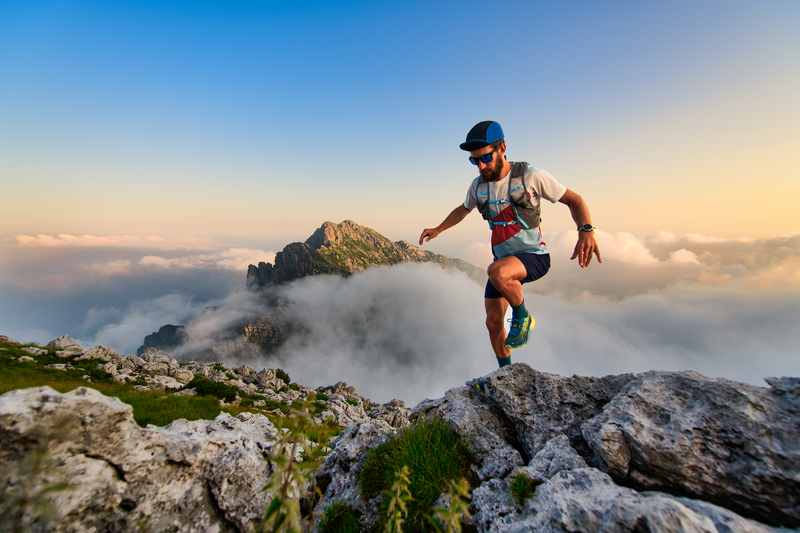
95% of researchers rate our articles as excellent or good
Learn more about the work of our research integrity team to safeguard the quality of each article we publish.
Find out more
ORIGINAL RESEARCH article
Front. Microbiol. , 16 December 2022
Sec. Microbe and Virus Interactions with Plants
Volume 13 - 2022 | https://doi.org/10.3389/fmicb.2022.992318
This article is part of the Research Topic Plant-Fungal Interactions View all 26 articles
Paridis Rhizoma is a natural medicine with strong anti-tumor and anti-inflammatory activities. Our previous research have found that Fusarium sp. C39, an endophytic fungus isolated from Dioscorea nipponica which contains the similar chemical components, significantly increased the steroidal saponins content of Paridis Rhizoma by fermentation. In this study, the inhibitory effects of fermentated Paridis Rhizoma extract (PRE) on liver cancer cells (Hepal-6), cervical cancer cells (Hela), and lung cancer cells (A549) were determined to be stronger than that of the unfermented extract. For discovering the fermentation mechanism of PRE with Fusarium sp. C39, 36 components with obviously quantitative variations were screened out by UPLC-Q/TOF-MS and 53 key genes involved in the metabolic pathways of steroidal saponins were identified by transcriptome. On the basis of comprehensively analyzing information from the metabonomics and transcriptome, it can be speculated that the increase of spirostanol saponins and nuatigenin-type saponins enhanced the inhibitory effect of fermented PRE on cancer cell proliferation. Under the action of glycosidase, glycosyltransferase, oxidoreductases, and genes involved in sterol synthesis, strain C39 achieved the synthesis of diosgenin and the alteration of configurations, sugar chain and substituent of steroidal saponins. The research suggested a microbial transformation approach to increase the resource utilization and activity of Paris polyphylla.
Paridis Rhizoma, the rhizome of the perennial herb Paris polyphylla Smith var. yunnanensis (Franch.) Hand. Mazz. (PPY) or Paris polyphylla Smith var. chinensis (Franch.) Hara (PPC), is an indispensable traditional Chinese medicine with the functions of clearing away heat, detoxifying, reducing swelling, and relieving pain. The earliest records of Paridis Rhizoma being applied traced back 2000 years ago to “Shen Nong’s Materia Medica” (China, 2020). At present, Chinese patent medicines that have been widely used clinically with Paridis Rhizoma as the main raw material include “Yunnan Baiyao,” “Chonglou Jiedu Tincture,” “Gongxuening Capsules,” and “Ji Desheng Snake Pills.” Phytochemical and pharmacological studies have shown that PPY and PPC are rich sources of steroidal saponins with significant anti-cancer, anti-inflammatory, hemostasis, immune regulation, and antioxidant effects (Tian et al., 2020; Thapa et al., 2022).
The main distribution areas of Paris polyphylla include China, India, Nepal and other countries bordering the Himalayas (Shah et al., 2012). Due to excessive development, illegal collection, open-air grazing, and other human activities, the wild resources of P. polyphylla have been severely damaged, and P. polyphylla is listed as endangered species, which seriously hinders sustainable resource acquisition (Cunningham et al., 2018; Pokhrel et al., 2019; Chauhan, 2020). At present, the expansion of artificial planting is the main measure to cope with the shortage of resources. But due to the disadvantages of the long growth cycle and low reproduction rate, it is difficult to meet the growing market demand (Yang et al., 2012). Microbial transformation is one of the promising routes to for expanding natural medicine resources. Compared with chemical synthesis, it has higher regioselectivity and stereoselectivity, mild action, high conversion rate, simple operation and low cost (Li et al., 2020). Endophytes are a special class of microorganisms, some of which are capable of producing the same biologically active compounds as the host plants or promoting the accumulation of secondary metabolites in synergy with the host plants. They are of interest as potential resources for producing and transforming natural medicines (Hardoim et al., 2015; Venieraki et al., 2017). However, these microorganisms usually do not have stable production and transformation capabilities when cultured in vitro, which limits their application (Venugopalan and Srivastava, 2015). Exploiting the function of valuable endophytes through biotechnology is currently a more useful solution (Kusari et al., 2014a,b; Shankar Naik, 2018). Therefore, exploring the regulatory mechanism of microbial production and the transformation of natural products at the molecular level is of great significance for expanding medical resources.
Fusarium sp. C39, a filamentous endophytic fungus isolated from Dioscorea nipponica that could significantly increase the total saponins content of D.nipponicae Rhizoma through fermentation (Du et al., 2013). Further research found that this strain also had similar effects on the saponin content of Paridis Rhizoma. In the liquid fermentation broth after acid hydrolysis treatment, the content of diosgenin increased by 39.00%, while the increase of pennogenin content increased by 288.64%. In this study, we investigated the inhibitory ability of fermented Paridis Rhizoma extract (PRE) on the proliferation of three cancer cell lines, compared the dynamic changes of the saponins content in PRE during fermentation process, and illustrated the differentially expressed genes (DEGs) in strain C39 that regulated the synthesis and transformation of steroidal saponins at the molecular level. The toxic activity of strain C39 on cancer cells and how strain C39 enhances the saponin content of Paridis Rhizoma were initially explored. These studies provide a theoretical basis for the further development of the ability of the strain to transform natural products.
The strain C39 was isolated from D. nipponica Makino and preserved in the Pharmaceutical Laboratory of the Heilongjiang University of Chinese Medicine. The preparation method of the culture medium is as follows: add 200 g of chopped peeled potatoes to distilled water and boil for 30 min, filter and make up the liquid to 800 ml. We added 20 g glucose and 20 g agar, which was packaged and sterilized at high pressure at 121°C for 30 min before use to obtain potato dextrose agar (PDA) medium. Then, we poured the hot medium into Petri dishes and added 35–40 ml of each. The potato dextrose broth (PDB) medium is used to prepare 100 ml seed culture and 150 ml of the drug-containing medium, which lacked the addition of agar during the same preparation process. Dispensed in conical flasks as required and sterilized.
Paridis Rhizomes were ground into powder and then sieved before use. Two grams of Paridis Rhizomes powder was added with 50 ml of 70% ethanol and reflux extract twice at 85°C for 2 h each time, combined with the filtrate and concentrated to dryness on a rotary evaporator to obtain PRE.
Strain C39 was activated on a PDA medium for 4–5 days, and a punch with a diameter of 8 mm was used to drill holes at the colony’s edge to obtain fungus cakes. Afterward, each cake was inoculated in 100 ml PDB medium for 3 days to prepare seed cultures and control samples were fermented for 0 days. Subsequently, the PRE was dissolved in 150 ml of PDB medium and sterilized. After cooling, 1.2 ml of seed solution was added to the drug-containing medium and fermented for different times of 3, 5, 7, and 9 days as the sample groups. All groups were incubated at 28°C with a rotation speed of 120 r/min in a shaker table.
As specified in Sections Preparation of Paridis Rhizoma extract and Liquid-state fermentation of PRE, 10 g of Paridis Rhizomes powder was subjected to liquid fermentation for 7 days and served as the sample group, while the unfermented raw medicinal material extract served as the control group. After the samples were concentrated to 20 ml, it was extracted three times with an equal volume of water-saturated n-butanol and the n-butanol layer was retained to prepare a lyophilized powder, which was dissolved in Dimethyl sulfoxide (DMSO) to prepare a 15 mg/ml mother solution, and stored at 4°C. The stock solution was diluted to the desired concentration with serum-free medium, followed by filtration and sterilization.
Hepal-6 liver cancer cells, Hela cervical cancer cells, and A549 lung cancer cells (Heilongjiang University of Chinese Medicine, Pharmacognosy Laboratory Storage) were cultured in the medium supplemented with 10% fetal bovine serum (FBS, Gibco, United States), 100 U/ml penicillin, and 100 μg/ml streptomycin. The medium of Hela and A549 cells is RPMI 1640 (Gibco, US), while the medium of Hepa1-6 cells is Dulbecco’s modified Eagle’s medium (DMEM, Gibco, United States), and all cells were incubated at 37°C and 5% CO2. Cells in the exponential growth phase were digested by trypsin and seeded in 96-well plates at 1 × 105 cells/mL density. After overnight incubation, cells were treated with different concentrations of the samples and incubated for another 24 h. Subsequently, the supernatant was discarded, 20 μl of 3-(4, 5-dimethylthiazol-2-yl)-2, 5-diphenyltetrazolium bromide (MTT) was added to each well, and the cells were incubated for 4 h. The supernatant was aspirated, supplemented with 100 μl of DMSO per well, and shaken for 15 min. The OD (optical density) value was measured at 490 nm using a Microplate Reader (Thermo, United States). Cell inhibition ratio (%) = [1-ASample/AControl] × 100%.
From each sample, 110 ml of culture medium was separated and concentrated, and ethanol was added to 80% concentration. After 12 h of precipitation, the filtrate was recovered under reduced pressure, and then the volume was fixed with 80% ethanol and filtered through a 0.22-μm membrane to obtain the test solution for the analysis of the changes in saponin components. For the standards, Polyphyllin I, II, VI, VII, pseudoprotodioscin, diosgenin and pennogenin were accurately weighed and prepared into a mixed reference solution with a mass concentration of 0.1 mg/ml. Metabolite profiling was conducted using a UPLC system (ACQUITY UPLC; Waters, Milford, MA, United States) and hybrid Q-TOF tandem mass spectrometry (Triple-TOF-MS; Triple TOF 5600 system; AB SCIEX, Concord, ON, Canada). Chromatographic separation was performed on an ACQUITY UPLC BEH C18 column (2.1 mm × 100 mm × 1.7 μm; Waters) using mobile phase A (0.1% formic acid in deionized water) and mobile phase B (0.1% formic acid in acetonitrile). Mobile phase B was increased linearly from 5% at 0 min to 40% at 2 min to 100% at 22 min and then held at 5% until 22.1 min. Finally, solvent B was held at 5% until 25 min. The flow rate was maintained at 0.3 ml min−1. Mass data acquisition was performed in both positive [electrospray ionization-positive (ESI+)] and negative (ESI−) modes using the following parameters: Ion spray voltage of 5.5 kV in ESI+ and −4.5 kV in ESI−; Nebulizer gas (gas 1) of 55 psi; Heater gas (gas 2) of 55 psi; Curtain gas of 35 psi; Turbo spray the temperature of 550°C; Declustering potential of 100 V in ESI+ and −100 V in ESI−. The collision energy of 35 V in ESI+ (−35 V in ESI−); Collision energy spread of 15 V. The mass spectrum scanning range was 80–1,500 Da.
To study the dynamic changes of steroidal saponins in the sample group and control group. The collected data is imported into the MarkerView (AB SCIEX, United States) software, and the error between the charge-to-mass ratio and the retention time is normalized by the Alignment&Filtering function; the processed data use the principal component analysis (PCA) method for pattern recognition and establishes the model, and the score matrix Scores and loadings are generated separately to obtain different compounds. Then, we used the established MS database of more than 200 species of plants of the genus Paris L. and the cleavage law of steroidal saponins, and used Peakview 2.0 software to pass targeted screening and non-target screening methods to infer the possible structure of the different components.
In a sterile environment, the remaining culture medium of each sample was separated and filtered with gauze, then rinsed with sterilized distilled water and removed excess water from the mycelium, immediately frozen in liquid nitrogen and stored at −80°C. The total RNA of strain C39 was extracted using TRIzol reagent (ThermoFisher, China) following the manufacturer’s protocol. The concentration of the extracted RNA samples was determined using a Nanodrop system (NanoDrop, Madison, United States), and the integrity of the RNA was examined by the RNA integrity number (RIN) using an Agilent 2,100 bioanalyzer (Agilent, Santa Clara, CA, United States). To construct the cDNA library, mRNA was purified by using Oligo (dT)-attached magnetic beads and sheared into short fragments, which served as templates for synthesizing the cDNAs using random hexamer primers. Following end-repair and adaptor ligation, the products were amplified by PCR and purified to create a cDNA library. The constructed libraries were then sequenced by using the BGIseq-500. The RNA Extraction, cDNA library construction and sequencing were performed at BGITech (Shenzhen, China).
The sequencing data of the adaptor, the low-quality reads, and the unknown bases were filtered with SOAPnuke (v1.4.0), and then clean reads were obtained and stored in FASTQ format for downstream analyses. HISAT2 (V2.1.0; Kim et al., 2015) and Bowtie2 (V2.2.5; Langmead and Salzberg, 2012) were used to map clean readings to the reference genome and align them with the reference coding gene set, respectively, and then the expression level of gene was calculated by RSEM (V1.2.8; Li and Dewey, 2011).
Differential expression analysis was performed using the DESeq2 (Love et al., 2014) with a Q value ≤ 0.05. To take an insight into the change of phenotype, GO1 and KEGG2 enrichment analysis of annotated different expressed genes was performed by Phyper3 based on Hypergeometric test. The significant levels of terms and pathways were corrected by Q value with a rigorous threshold (Q value ≤ 0.05) by Bonferroni. To visualize the results of GO enrichment of DEGs, Cytoscape version 3.6.1 with BiNGO plugin version 3.0.3 (Maere et al., 2005) were used to construct significantly enriched biological networks and output the results as graphs. To predict the functional interaction of proteins, DIAMOND (Buchfink et al., 2021) was used to align the genes to the STRING database, and the network relationship with a score value of ≥300 was screened into Cytoscape to visualize the PPI network, and the cytoHubba plugin was used to screen hub genes to construct a sub-network.
The reliability of RNA-seq data was validated by qRT-PCR using the AriaMx Real-Time PCR System (Agilent, United States). Primer sequences were designed based on mRNA by Sangon Biotech (Sangon, China) and Takara Bio Inc. (Takara, Japan) using Primer 5 in the laboratory and are shown in Supplementary Table S1. First-strand cDNA was synthesized from the total RNA using PrimeScript™ RT Reagent Kit with gDNA Eraser and then TB Green ® Premix Ex Taq™ П (Takara, Japan) was used for qRT-PCR. The total reaction mixture volume for each reaction was 20 μl, and the amplification program was as follows: 95°C for 30 s, followed by 40 cycles of 95°C for 5 s, and 60°C for 30 s. The tubulin beta (TUBB) (GME8911_g) was selected as the housekeeping gene for the normalization, and three technical replicates were performed for each sample. The relative expression levels for each gene were calculated using the 2−△△Ct method.
PRE effectively reduced the viability of three cancer cells (Figure 1). The IC50 values of the control group were 234 μg/ml in Hepa1-6 cells, 97 μg/ml in Hela cells and 315 μg/ml in A549 cells, while the values of the sample group decreased by 29.48, 39.17, and 32.06%, respectively.
Figure 1. Inhibitory effects of fermentation products on proliferation of different cancer cells. (A) Hepa1-6 cells (B) Hela cells and (C) A549 cells. Values are means ± SD. The asterisk above the line chart denoted statistical significance (*p < 0.05, ** p < 0.01, and ns p > 0.05).
To assist in determinating steroidal saponins in PRE, a database containing the reported compound of the plants of Paris L. was first constructed (Zhao et al., 2009; Qin et al., 2012, 2016; Wu et al., 2012a,b; Ling et al., 2015), and according to the configuration of C-25 and the state of the F ring, the main steroidal saponins were classified into four types: isospirostane-type (25R), spirostane-type (25S), nuatigenin-type, and furostane-type. Among them, isospirostane-type saponins occupy a major composition, mainly including diosgenin-type and pennogenin-type saponins (Figure 2). Based on the relevant reports on the fragmentation mechanism of steroidal saponins of the Paris polyphylla, the characteristic fragmentation patterns were summarized, and a strategy for characterizing the structure of steroidal saponins was proposed and further verified by five reference standards, including two diosgenin-type saponins, two pennogenin-type saponins, and one furostane-type saponins (Figure 3). Generally, the molecular mass and formula could be obtained from the [M + H]+, [M + Na]+ [M + HCOO]− and [M-H]− ions.
Figure 3. Strategy used for characterizing steroidal saponins in Paridis Rhizoma by HPLC-Q/TOF-MS. (A) Chromatographic behavior of steroidal saponins with different configurations. (B) BPC chromatograms of UPLC-MS for reference standards at ESI−.
The metabolome data of fermentation samples at days 3, 5, 7, and 9, as well as control samples were collected by UPLC-Q/TOF-MS, and the base peak chromatograms (BPC) in the positive mode were shown in Figures 4A,B. Combined with the method of principal component analysis (PCA), the data were pattern recognized, and a model was established to produce scores plot and loadings plot, respectively (Figures 4C,D). The scores plot showed that the six groups of samples were divided into 4 four types, and the control group differed significantly from the fermentation group. However, no distinction occurred between 3 days and 5 days of fermentation and between 7 days and 9 days of fermentation. In the loadings plot, the distance between each point and the origin represented the contribution to the typing. The ions represented by the farther away points were the focus of the analysis, and thus a total of 36 labeled metabolites including seven furostane-type saponins (peaks 1, 3–8), 23 spirostanol saponins (peaks 2, 13–34), four nuatigenin-type saponins (peaks 9–12) and two glycosides (Peaks 35, 36), were screened out as the identification components (Figure 5; Table 1).
Figure 4. The screening of differential saponins during liquid fermentation of PRE by strain C39 at ESI+. (A) BPC chromatograms of UPLC-MS for sample group and (B) seed culture solution group. (C) PCA scores plot and (D) loadings plot of all groups.
As the fermentation time increased, the content of these labeled steroidal saponins showed different changing trends. As a whole, the content of total saponins was first decreasing, then increasing, and then decreasing, and reached the maximum in 5 days of fermentation (Figure 6A). For furostane-type saponins, the content showed an obvious continuous decreasing trend (Figure 6B), while the content of spirostanol saponins generally showed the tendency of first increasing and then decreasing. The content of nuatigenin-type saponins displayed a trend of first decreasing and then increasing (Figure 6C). For diosgenin-type saponins, the content of peaks 26 and 31–34 reached the maximum at 5 days of fermentation, while the peaks 24, 25, 27, 28, 29, and 30 reached the maximum content in fermentation to 7 days (Figure 6D). Among them, the content of peaks 27, 28, 30, 31, and 34 decreased significantly after 3 days of fermentation. According to Figure 6E, pennogenin-type saponins and some saponins with hydroxyl substituents on diosgenin have similar cleavage rules, including peaks 13–15 18, 19, 21, and 23. The content of peaks 19 and 21 reached the maximum at 3 days of fermentation, while the peaks 13, 14, 15, and 23 reached the maximum content at 5 days, and peak 18 showed a continuous upward trend. Peaks 16, 17, 20, and 22 are saponins substituted with carbonyl groups on diosgenin. Peaks 16, 20, and 22 reached the maximum at 3 days of fermentation and peak 17 showed a continuous upward trend. Peak 2 was diosgenin with a hydroxyl group attached to the C23 position, and its content decreased significantly after 3 days of fermentation. In addition, three glycosides were screened. By comparing with the standards, it was determined that peak 35 was pennogenin and the peak 36 was diosgenin, both of which increased first and then decreased (Figure 6A). In the seed culture solution, diosgenin was determined. However, the content of these components varied greatly among parallel samples.
Figure 6. Variation trend of the response values of the selected components with the fermentation time. (A) Total saponins and sapogenin. (B) Furostane-type saponins. (C) Nuatigenin-type saponins. (D) Diosgenin-type saponins. (E) Pennogenin-type and other spiosteroidal saponins. Values are means ± SD. The asterisk above the bar chart denoted statistical significance (*p < 0.05, **p < 0.01, and ns p > 0.05).
A total of 25 mycelial samples at all fermentation time points (0, 3, 5, 7, and 9 days) were tested using the DNBSEQ platform, and each sample produced 6.42Gb data on average. The average comparison rate of the sample comparison genome was 93.78%; the total number of genes detected was 13,481, of which 13,407 were known genes and 74 new genes were predicted; a total of 5,271 new transcripts were detected, of which 3,161 belonged to new alternative splicing subtypes of known protein-coding genes, 74 belong to new protein-coding gene transcripts, and the remaining 2036 belong to long-chain non-coding RNAs. The number of DEGs is shown in Figure 7.
Figure 7. Statistics of the number of DEGs. (A) The number of up-down regulated DEGs. (B) Venn diagram of DEGs of D0-vs-D3, D3-vs-D5, D5-vs-D7, and D7-vs-D9.
In the early stage, our research group has completed the genome sequencing of the C39 strain; all raw sequencing data were deposited in the NCBI database with the accession numbers JAGFMC000000000, and used to annotate the functional information of the transcripts (Ding et al., 2022).
Gene Ontology (GO) assignments were used to classify the functions of the DEGs, and the assigned DEGs were divided into three functional categories: molecular functions (MF), cellular components (CC), and biological processes (BP), which were further divided into more detailed subcategories (Figure 8A; Supplementary Figure S1). The number of DEGs of D0-vs-D3, D3-vs-D5, D5-vs-D7 and D7-vs-D9 involved in molecular function (MF) was the largest, followed by cellular_component (CC) and biological_process (BP). In the MF category, “catalytic activity” was the most abundant category, followed by “binding.” “transporter activity” and “transcription regulator activity” were similar. In the CC category, “cellular anatomical entities” occupied the largest proportion, and followed by “intracellular” and “protein-containing complexes.” In the BP category, the most abundant GO terms were “metabolic process” and “cellular process,” followed by “biological regulation” and “localization.” The GO enrichment analysis classified the DEGs according to the GO annotation results. D0-vs-D3, D3-vs-D5, D5-vs-D7, and D7-vs-D9 were, respectively, assigned 3,033, 2,918, 1888, and 569 GO terms, which contained 3,890, 3,297, 1,244, and 146 DEGs. The GO enrichment of DEGs is shown in Figure 8B; Supplementary Figure S2.
Figure 8. GO analysis of DEGs during the fermentation of D0-vs-D3. (A) The GO terms analysis and (B) GO enrichment analysis of top 20 with the smallest Q value.
According to the results of KEGG, a total of 2,594, 2,162, 809, and 116 DEGs could be aligned to the KEGG pathways teams in D0-vs-D3, D3-vs-D5, D5-vs-D7, and D7-vs-D9, respectively. The DEGs involved in KEGG pathway teams were divided into five categories: “Cellular processes,” “Environmental information processing,” “Genetic information processing,” “Metabolism,” and “Organism systems” (Figure 9A; Supplementary Figure S3). The KEGG pathway enrichment of DEGs is shown in Figure 9B; Supplementary Figure S4. Among them, DEGs were significantly concentrated in “Metabolism.” Under this group, the most relevant pathways to the synthesis of steroidal saponins were “Steroid biosynthesis” and “Terpenoid backbone biosynthesis,” which belong to the teams “Lipid metabolism” and “Metabolism of terpenoids and polyketides.” In addition, “Glycan biosynthesis and metabolism” and “Carbohydrate metabolism” were also related to this process. A protein–protein interaction (PPI) network was constructed utilizing DEGs included in these teams, and Cytoscape-cytoHubba was used to screen out the top 50 hub genes and construct a sub-network (Figure 10). The proteins encoding genes glucose-6-phosphate isomerase (GPI), phosphoglucomutase (pgm), enolase (ENO), 15-cisphytoene synthase / lycopene beta-cyclase (AL2), squalene monooxygenase (ERG1; SQLE), isopentenyl-diphosphate Delta-isomerase (IDI), sterol 14alpha-demethylase (CYP51), sterol-4alph-acarboxylate 3-dehydrogenase (decarboxylating) (ERG26; NSDHL), delta14-sterol reductase (ERG24; FK), and malate dehydrogenase (oxaloacetate-decarboxulating) (NADP+) (maeB) may play an important role in increasing saponin content of Paridis Rhizoma by strain C39. Among all KEGG pathway terms, “Pentose phosphate pathway,” “RNA degradation,” “Ribosome,” and “mRNA surveillance pathway” were considered as significantly differential pathways (Q < 0.05).
Figure 9. KEGG analysis of DEGs during the fermentation of D0-vs-D3. (A) The KEGG pathway terms analysis and (B) KEGG pathway enrichment analysis of top 20 with the smallest Q value.
Combined with the relevant annotations of the two databases, about 48 DEGs that may be involved in the synthesis and transformation of steroidal saponins were selected (Supplementary Table S2). The synthesis of steroidal saponins can be divided into three stages: sterol synthesis, aglycon synthesis, and saponin synthesis (Chen et al., 2021). In plants, sterol synthesis first relies on the MVA and MEP pathways to synthesize FPP. The screened DEGs showed that strain C39 only relied on the MVA pathway for sterol synthesis, and the DEGs in this process included acetyl-CoA C-acetyltransferase (AACT), Mevalonate kinase (MVK), hydroxymethylglutaryl-CoA reductase (NADPH, HMGCR), phosphomevalonate kinase (mvaK2), and isopentenyl-diphosphate Delta-isomerase (IDI). Then, the obtained FPP synthesizes lanosterol by farnesyl-diphosphate farnesyltransferase (FDFT1), ERG1, and lanosterol synthase (ERG7). Finally, through CYP51 ERG24/FK, methylsterol monooxygenase (ERG25), ERG26, 3-keto steroid reductase (ERG27), cholestenol Delta-isomerase (EPB; HYD1), sterol 24-C-methyltransferase (ERG6; SMT1), C-8 sterol isomerase (ERG2), Delta7-sterol 5-desaturase (ERG3; STE1), sterol 22-desaturase (ERG5), Delta24(24(1))-sterol reductase (ERG4) and Delta24-sterol reductase (DWF1) to synthesize ergosterol, cholesterol or plant sterols. The above-related genes were differentially expressed during the fermentation process. The expression levels of CYP51, ERG2 and ERG25 were the largest in the control group, and the expression levels of other selected DEGs showed a trend of first increase and then decrease or continuous increase on the whole. The expression levels of all DEGs are shown in Figures 11, 12.
Figure 11. DEGs expression heatmap related to the synthesis and transformation of steroidal saponins by strain C39. The color from blue to red indicated log2 (FPKM +1) from low to high.
Figure 12. The expression trends of genes related to steroidal saponin synthesis pathway in fermentation on days 3(T), 5(F), 7(S), and 9(N).
The synthesis of saponins also requires the participation of various cytochrome P450s, glycosyltransferases, glycosidases and oxidoreductases. After several steps of cytochrome P450s, sterols are catalyzed into sapogenins, then under the synthesis and modification of glycosyltransferases, glycosidases and some oxidoreductases, saponins with different configurations are produced. By analyzing related genes whose expression levels were significantly increased at D3 or D5, glucosyltransferase, glucosidase, galactosidase and oxidoreductase that may be involved in the synthesis and conversion of steroidal saponins were screened out.
To validate the reliability of the RNA-Seq data, eight DEGs that may be involved in the promotion and transformation of steroidal saponins were selected for the quantitative real-time PCR (qRT-PCR) analysis. As shown in Figure 13, all the selected genes were differentially expressed under different fermentation days, showing close similarity gene expression trends to RNA-Seq results.
Figure 13. The expression profiles of qRT-PCR and RNA-seq of eight selected genes. The left vertical axis represents the relative expression of the gene based on qRT-PCR. The right vertical axis represents the expression level of the gene based on qRT-PCR. Values are means ± SD. The asterisk above the bar chart denoted statistical significance (*p < 0.05, **p < 0.01, and ns p > 0.05).
The use of microbial fermentation to synergize, attenuate, or transform medicinal plants for better clinical treatment has a long history. Relying on the microorganism characteristics of high efficiency, strong specificity, economy, and environmental protection, the production and transformation of natural products by microorganisms has become an important way to expand natural medicinal resources, increase the yield of medicinally active ingredients, and find new medicinal substances (Venisetty and Ciddi, 2003; Pham et al., 2019). For example, a variety of pentacyclic triterpenoids were biotransformed into new derivatives by Nocardia sp. NRRL 5646 through esterification, methyl transfer, backbone rearrangement, and hydroxylation reactions (Cheng et al., 2004; Zhang et al., 2005; Qian et al., 2009). The main saponins of ginsenosides Rb1, Rb2, Re, and Rg1 in ginseng that is not easy to be directly utilized in the human body were converted by microorganisms into rare saponins with small molecules such as ginsenoside CK, which possess higher bioavailability and better pharmacological activities (Geraldi, 2020). The yield and purity of resveratrol – a precious anti-cancer and antioxidant phenolic substance – are low in natural plants. Using co-immobilized edible Aspergillus niger and Yeast, the conversion rate of polydatin can reach 97%, which is significantly higher than that of the control group (Jin et al., 2013). Currently, the main use of steroidal saponins is as the raw material of steroid hormone drugs, but the production process will cause serious environmental pollution (Cheng et al., 2009). Microbial transformation can replace acid hydrolysis to produce diosgenin with high efficiency and environmental protection (Chen et al., 2018). At the same time, microbial biotechnologies play a significant role in the pharmaceutical steroid industry (Donova, 2018). The pharmacological effects of steroidal saponins are closely related to structural characteristics such as sugar chains, glycosyl types, and substituents (Wang et al., 2010; Prawat et al., 2016). Spirostanol saponins have stronger cytotoxicity than furostane-type saponins (Zhao et al., 2009). For spirostanol saponins, diosgenin-type saponins have stronger anti-cancer effects on various cancers, while pennogenin-type saponins show more excellent hemostatic effects (Huang et al., 2007; Fu et al., 2008; Yan et al., 2009). Nuatigenin-type saponins are rare in Paris polyphylla, and some show cytotoxicity to human cancer cell lines (HepG2 and HEK293; Qin et al., 2016). Pharmacological studies showed that the fermentation sample had significantly stronger inhibitory effects on the proliferation of Hepal-6 liver cancer cells, Hela cervical cancer cells, and A549 lung cancer cells than its raw medicinal material sample. This proves that strain C39 significantly increased the saponin content and enhanced the anti-cancer effect. There are few studies on the microbial synthesis and promotion of steroidal saponins in Paris polyphylla, especially related to the transformation mechanism.
The saponin contents of the four configurations showed different trends during the fermentation process. The content of furostane-type saponins linked to glucose at the C26 position shows an obvious downward trend, while spirostanol saponins increase first. Studies have shown that furostane-type saponins can be converted to spirostanol saponins by breaking the C26 bond and cyclization chain (Nakayasu et al., 2015) suggesting that the biotransformation of furostanol-type saponins to spirostanol saponins occurs under the action of strain C39. Chaetomium olivaceum and Gibberella fujikuroi are two reported microorganisms with the character of biotransforming pseudoprotodioscin (Dong et al., 2016; Hu et al., 2018). This is the first report of increasing the content of diosgenin-type saponins and pennogenin-type in Paris polyphylla by microbial transformation, which promoted the biosynthesis of spirosteroidal saponins with stronger pharmacological activity. Diosgenin can be converted into nuatigenone by microbial biotransformation (Wang et al., 2007). In this study, the content of nuatigenin-type saponins linked to glucose at C26 showed a significant downward trend after 3 days of fermentation and then gradually increased, so it is speculated that this type of saponins may be the intermediates in the biotransformation process. After the cyclization of furostanol saponins, the chemical bond at the C26 position is broken and converted into spirosterol saponins or other types of saponins through isomerization. Peak 2 is a diosgenin-type saponin, which shows an unexpected and obvious decreasing trend. Structurally, it is linked to a glucose saponin at the C23 position, so it is speculated that hydrolysis of glucose at the C23 position may have occurred. The above transformations all depended on the glucose hydrolysis of strain C39. The transformation of microorganisms into natural medicines can also be achieved through other redox reactions. Among the spirosteroidal saponins that have been found, the saponins containing carbonyl or hydroxyl groups show a trend of first decreasing and then increasing or continuing to increase. It is speculated that the diosgenin-type saponins are hydroxylated or carbonylated. This effect was also verified in the study of the mechanism of biotransformation of Dioscorea nipponica by strain C39 (Huang et al., 2022). The ability of strain C39 to promote the synthesis and transformation of steroidal saponins in Paris polyphylla improved the accumulation of effective substances, supplemented the steroidal saponin synthesis pathway, and improved the utilization of Paris polyphylla.
To further explain the mechanism of the transformation of steroidal saponins by strain C39, the mycelia of different fermentation times were used for transcriptome analysis. Through GO enrichment analysis, GO terms related to oxidoreductase activity showed a strong correlation. According to the KEGG database, the “Pentose phosphate pathway,” “RNA degradation,” “Ribosome,” and “mRNA surveillance pathway” showed significant enrichment. Hub genes GPI, pgm, ENO, AL2, ERG1, IDI, CYP51, ERG26, ERG24, and maeB, which were involved in “Steroid biosynthesis,” “Glycolysis / Gluconeogenesis,” “Carbon metabolism,” “Terpenoid backbone biosynthesis,” and “Carotenoid biosynthesis,” were considered to be at the key positions in the process of strain C39 increasing the content of steroidal saponins. The sterol required in the synthesis of steroidal saponins is cholesterol or sitosterol, but in fungi, ergosterol occupies a major position, and only certain microorganisms have been found to produce cholesterol or phytosterol (Grandmougin-Ferjani et al., 1999; Wang et al., 2012; Torres et al., 2017). Moreover, most of the genes required for the sterol synthesis pathway of microorganisms are the isoenzymes with those in phytosterols. Studies have shown that the synthesis of cholesterol and diosgenin has been achieved using the sterol synthesis pathway in yeast (Souza et al., 2011; Cheng et al., 2020). The sterol synthesis pathway of strain C39 may be the key pathway for sapogenin synthesis. By screening the expression of related genes in this pathway. None of the related genes on the MEP pathway showed differential expression. However, the expression levels of most genes in the MVA pathway and other processes increased significantly in 3 or 5 days of fermentation, which further verifies this conjecture. The transformation of natural medicines by microorganisms is catalyzed by characteristic enzymes that constantly exhibit substrate promiscuity (Copley, 2015). Designing an in vitro synthesis pathway by combining the inherent promiscuous enzyme of protein engineering and metabolic engineering is one of the important solutions to improve the in vitro production yield of natural active products. Glycosyltransferase is the key enzyme in converting sapogenin to saponin and the enrichment of saponin species. A glycosyltransferase cloned from Bacillus subtilis introduces a glucose at the C-6 position of ginsenoside Rh1 to obtain a potential active saponin (Luo et al., 2015). Sterol glycosyltransferase uses cholesterol, sitosterol, and ergosterol as sugar receptors to synthesize the corresponding sterol glycosides. The sterol glycosyltransferase UGT51 isolated from Saccharomyces cerevisiae was used to construct a yeast engineering strain to achieve the maximum titer production of ginsenoside Rh2 synthesized by protopanaxadiol in vitro (Zhuang et al., 2017). The conversion of furostanol saponins to spirostanol saponins and the deglycosylation of spirostanol saponins are all completed under glycosidases. The glycosidase PGase-1 isolated and purified from Aspergillus oryzae can hydrolyze the terminal 26-O-β-D glucopyranoside and 3-O-(1 → 4)-α-L-rhamnopyranoside (Liu et al., 2013). The β-glucosidase AfG obtained from Aspergillus fumigatus has strong thermal stability and can realize the hydrolysis of 3-O-glucopyranoside of various diosgenin-type saponins (Lei et al., 2012). Through transcriptome analysis, seven glycosyltransferases, 11 glycosidases and one oxidoreductase with unknown function were screened that may promote the synthesis of steroidal saponins. These enzymes have potential value for synthesizing or converting of steroidal saponins in vitro. The functions of these genes need to be further verified. The inferred transformation pathway of strain C39 is shown in Figure 14.
In conclusion, this study explored the cytotoxic activity of PRE fermentation products and conducted a preliminary study on how strain C39 increases the steroidal saponins content of Paridis Rhizoma. While strain C39 increased the saponin content, the inhibition of cancer cell proliferation was also enhanced, especially in cervical cancer. Under the action of glycosidase, glycosyltransferase, and oxidoreductase, the configuration and substituent of steroidal saponins were changed. Strain C39 could synthesize diosgenin, and the sterol synthesis pathways were closely related to this process. Further, the synthesized diosgenin may be synthesized into steroidal saponins. The DEGs were significantly enriched in the “Pentose phosphate pathway,” “RNA degradation,” “Ribosome,” and “mRNA surveil-lance pathway,” which means that these four pathways were significantly affected and played a prominent role. Through PPI, hub genes GPI, pgm, ENO, AL2, ERG1, IDI, CYP51, ERG26, ERG24, and maeB were implicated in directly affecting the steroidal saponins content of strain C39. Understanding the synthetic pathway and key genes is the basis for the in vitro synthesis of natural products, and exploring the transformation mechanism promoted the in vitro synthesis of steroidal saponins in Paridis Rhizoma. In subsequent studies, the functions and properties of key genes in strain C39 involved in regulating steroidal saponin synthesis and transformation will be deeply explored and applied in practice.
The datasets presented in this study can be found in online repositories. The names of the repository/repositories and accession number(s) can be found at: https://www.ncbi.nlm.nih.gov/, PRJNA850155.
YC, XD, and DY conceptualized and designed the experiment. YC performed a study of the transformation mechanism of strain C39 to increase saponin content, analyzed the data, and drafted the manuscript. JH and NH assisted in the analysis of the chemical constituent structures. MZ conducted cancer cell cytotoxicity experiments. XD and DY supervised and edited the manuscript. All authors contributed to the article and approved the submitted version.
This research was funded by National Natural Science Foundation of China, grant number 81872967.
All authors would like to acknowledge the HACMS staff members for directing the acquisition of UPLC-Q/TOF-MS data.
The authors declare that the research was conducted in the absence of any commercial or financial relationships that could be construed as a potential conflict of interest.
All claims expressed in this article are solely those of the authors and do not necessarily represent those of their affiliated organizations, or those of the publisher, the editors and the reviewers. Any product that may be evaluated in this article, or claim that may be made by its manufacturer, is not guaranteed or endorsed by the publisher.
The Supplementary material for this article can be found online at: https://www.frontiersin.org/articles/10.3389/fmicb.2022.992318/full#supplementary-material
Buchfink, B., Reuter, K., and Drost, H. G. (2021). Sensitive protein alignments at tree-of-life scale using DIAMOND. Nat. Methods. 18, 366–368. doi: 10.1038/s41592-021-01101-x
Chauhan, H.K. (2020). The IUCN red list of threatened species 2020: E.T175617476A176257430. [Online].
Chen, Y., Dong, Y., Chi, Y. L., He, Q., Wu, H., and Ren, Y. (2018). Eco-friendly microbial production of diosgenin from saponins in Dioscorea zingiberensis tubers in the presence of Aspergillus awamori. Steroids. 136, 40–46. doi: 10.1016/j.steroids.2018.05.005
Chen, Y. Y., Wu, J. K., Yu, D., and Du, X. W. (2021). Advances in steroidal saponins biosynthesis. Planta. 254:91. doi: 10.1007/s00425-021-03732-y
Cheng, J., Chen, J., Liu, X. N., Li, X. C., and Ma, Y. H. (2020). The origin and evolution of diosgenin biosynthetic pathway in yam. Plant Commun. 2:100079. doi: 10.1016/j.xplc.2020.100079
Cheng, Z. H., Yu, B. Y., Cordell, G. A., and Qiu, S. X. (2004). Biotransformation of quinovic acid glycosides by microbes: direct conversion of the ursane to the oleanane triterpene skeleton by Nocardia sp. NRRL 5646. Org. Lett. 6:3163. doi: 10.1021/ol048787b
Cheng, P., Zhao, H. Z., Zhao, B., and Ni, J. R. (2009). Pilot treatment of wastewater from Dioscorea zingiberensis C.H. Wright production by anaerobic digestion combined with a biological aerated filter. Bioresour. Technol. 100, 2918–2925. doi: 10.1016/j.biortech.2009.01.054
China., E.B.o.t.P.o.t.P.s.R.o (2020). Pharmacopoeia of the People’s Republic of China. Beijing: China Medical Science Press.
Copley, S. D. (2015). An evolutionary biochemist's perspective on promiscuity. Trends Biochem. Sci. 40, 72–78. doi: 10.1016/j.tibs.2014.12.004
Cunningham, A. B., Brinckmann, J. A., Bi, Y. F., Pei, S. J., Schippmann, U., and Luo, P. (2018). Paris in the spring: a review of the trade, conservation and opportunities in the shift from wild harvest to cultivation of Paris polyphylla (Trilliaceae). J. Ethnopharmacol. 222, 208–216. doi: 10.1016/j.jep.2018.04.048
Ding, C. H., Yu, D., Chen, Y. Y., Zhu, J. Y., Wu, J. K., Du, X. W., et al. (2022). Analysis of the mechanism of saponin biotransformation in Dioscoreae nipponicae rhizoma by the endophytic fungus Fusarium sp. C39 using whole-genome sequencing. J. Basic Microbiol. 62, 623–633. doi: 10.1002/jobm.202100664
Dong, X. R., Gao, Z. H., Hu, H. X., Gao, R. R., and Sun, D. (2016). Microbial transformation of Pseudoprotodioscin by Chaetomium olivaceum. J. Mol. Catal. B Enzym. 130, 88–95. doi: 10.1016/j.molcatb.2016.05.001
Donova, M. (2018). Microbiotechnologies for steroid production. Microbiol. Aust. 39, 126–129. doi: 10.1071/MA18039
Du, X. W., Mou, J. C., Meng, F. J., Liu, Y., Li, B., Yu, D., et al. (2013). Increase of diosgenin in Dioscoreae Nippponicae Rhizoma with solid state fermentation of endophytic fungi. Drugs Clin. 28, 179–181.
Fu, Y. L., Yu, Z. Y., Tang, X. M., Zhao, Y., Yuan, X. L., Wang, S., et al. (2008). Pennogenin glycosides with a spirostanol structure are strong platelet agonists: structural requirement for activity and mode of platelet agonist synergism. J. Thromb. Haemost. 6, 524–533. doi: 10.1111/j.1538-7836.2007.02881.x
Geraldi, A. (2020). Advances in the production of minor ginsenosides using microorganisms and their enzymes. BIO Integ. 1, 15–24. doi: 10.15212/bioi-2020-0007
Grandmougin-Ferjani, A., Dalpé, Y., Hartmann, M.-A., Laruelle, F., and Sancholle, M. (1999). Sterol distribution in arbuscular mycorrhizal fungi. Phytochemistry. 50, 1027–1031. doi: 10.1016/S0031-9422(98)00636-0
Hardoim, P. R., van Overbeek, L. S., Berg, G., Pirttilä, A. M., Compant, S., Campisano, A., et al. (2015). The hidden world within plants: ecological and evolutionary considerations for defining functioning of microbial Endophytes. Microbiol. Mol. Biol. Rev. 79, 293–320. doi: 10.1128/MMBR.00050-14
Hu, H. X., Gao, R. R., Gao, Z. H., Qiao, Y., Dong, X. R., Ding, G., et al. (2018). Microbial transformation of pseudoprotodioscin by Gibberella fujikuroi. J. Asian Nat. Prod. Res. 20, 1–9. doi: 10.1080/10286020.2018.1468438
Huang, Y., Cui, L. J., Zhan, W. H., Dou, Y. H., Wang, Y. L., Wang, Q., et al. (2007). Separation and identification of steroidal compounds with cytotoxic activity against human gastric cancer cell lines in vitro from the rhizomes of Paris polyphylla var. chinensis. Chem. Nat. Compd. 43, 672–677. doi: 10.1007/s10600-007-0225-8
Huang, N. N., Yu, D., Huo, J. H., Wu, J. K., Chen, Y. Y., Du, X. W., et al. (2022). Study of Saponin components after biotransformation of Dioscorea nipponica by Endophytic fungi C39. J. Anal. Method. Chem. 2022:2943177. doi: 10.1155/2022/2943177
Jin, S., Luo, M., Wang, W., Zhao, C. J., Gu, C. B., Li, C. Y., et al. (2013). Biotransformation of polydatin to resveratrol in Polygonum cuspidatum roots by highly immobilized edible Aspergillus Niger and yeast. Bioresour. Technol. 136, 766–770. doi: 10.1016/j.biortech.2013.03.027
Kim, D., Langmead, B., and Salzberg, S. L. (2015). HISAT: a fast spliced aligner with low memory requirements. Nat. Methods. 12, 357–360. doi: 10.1038/nmeth.3317
Kusari, S., Singh, S., and Jayabaskaran, C. (2014a). Biotechnological potential of plant-associated endophytic fungi: hope versus hype. Trends Biotechnol. 32, 297–303. doi: 10.1016/j.tibtech.2014.03.009
Kusari, S., Singh, S., and Jayabaskaran, C. (2014b). Rethinking production of Taxol® (paclitaxel) using endophyte biotechnology. Trends Biotechnol. 32, 304–311. doi: 10.1016/j.tibtech.2014.03.011
Langmead, B., and Salzberg, S. (2012). Fast gapped-read alignment with bowtie 2. Nat. Methods. 9, 357–359. doi: 10.1038/nmeth.1923
Lei, J., Niu, H., Li, T. H., and Huang, W. (2012). A novel β-glucosidase from Aspergillus fumigates releases diosgenin from spirostanosides of Dioscorea zingiberensis CH Wright (DZW). World J. Microbiol. Biotechnol. 28, 1309–1314. doi: 10.1007/s11274-011-0907-z
Li, B., and Dewey, C. N. (2011). RSEM: accurate transcript quantification from RNA-Seq data with or without a reference genome. BMC Bioinform. 12, 323–323. doi: 10.1186/1471-2105-12-323
Li, Y., Zhou, J., He, X. D., Shao, D. X., Zhu, Z., and J., and Lin, Y.M., (2020). Application of microbial transformation in the research of modern traditional Chinese medicine. Chin. J. Antibiot. 45, 418–422. doi: 10.3969/j.issn.1001-8689.2020.05.003
Ling, Y., Fu, Z., Zhang, Q., Xu, L. L., and Liao, L. (2015). Identification and structural elucidation of steroidal saponins from the root of Paris polyphylla by HPLC-ESI-QTOF-MS/MS. Nat. Prod. Res. 29, 1798–1803. doi: 10.1080/14786419.2015.1007137
Liu, T. Q., Yu, H. S., Liu, C. Y., Wang, Y. H., Tang, M. Q., Yuan, X. D., et al. (2013). Protodioscin-glycosidase-1 hydrolyzing 26-O-β-D-glucoside and 3-O-(1 → 4)-α-L-rhamnoside of steroidal saponins from Aspergillus oryzae. Appl. Microbiol. Biotechnol. 97, 10035–10043. doi: 10.1007/s00253-013-4791-3
Love, M. I., Huber, W., and Anders, S. (2014). Moderated estimation of fold change and dispersion for RNA-seq data with DESeq2. Genome Biol. 15:550. doi: 10.1186/s13059-014-0550-8
Luo, S. L., Dang, L. Z., Zhang, K., Liang, L., and Li, G. (2015). Cloning and heterologous expression of UDP-glycosyltransferase genes from Bacillus subtilis and its application in the glycosylation of ginsenoside Rh1. Lett. Appl. Microbiol. 60, 72–78. doi: 10.1111/lam.12339
Maere, S., Heymans, K., and Kuiper, M. (2005). BiNGO: a Cytoscape plugin to assess overrepresentation of gene ontology categories in biological networks. Bioinformatics. 21, 3448–3449. doi: 10.1093/bioinformatics/bti551
Nakayasu, M., Kawasaki, T., Lee, H.J., Sugimoto, Y., and Mizutani, M.. (2015). Identification of furostanol glycoside 26-O-β-glucosidase involved in steroidal saponin biosynthesis from Dioscorea esculenta. Plant Biotechnology. 32(4):299–308. doi: 10.5511/plantbiotechnology.15.1023b
Pham, J. V., Yilma, M. A., Feliz, A., Majid, M. T., Maffetone, N., Walker, J. R., et al. (2019). A review of the microbial production of bioactive natural products and biologics. Front. Microbiol. 10:1404. doi: 10.3389/fmicb.2019.01404
Pokhrel, G., Upadhyaya, A., and Thapa, M. S. (2019). Threats and conservation of Paris polyphylla: vulnerable medicinal Plant in Panchase Protected Forest, Nepal. For. J. Int. For. Nepal 16, 14–30. doi: 10.3126/forestry.v16i0.28351
Prawat, H., Mahidol, C., Kaweetripob, W., Intachote, P., Pisutjaroenpong, S., and Ruchirawat, S. (2016). Cytotoxic steroidal glycosides from the whole Plant of Calamus acanthophyllus. Planta Med. 82, 1117–1121. doi: 10.1055/s-0042-106972
Qian, L. W., Zhang, J., Liu, J., Hua,, Yu, B., and Yang, (2009). Direct microbial-catalyzed asymmetric α-hydroxylation of betulonic acid by Nocardia sp. NRRL 5646. Tetrahedron Lett. 50, 2193–2195. doi: 10.1016/j.tetlet.2009.02.137
Qin, X. J., Sun, D. J., Ni, W., Chen, C. X., Hua, Y., He, L., et al. (2012). Steroidal saponins with antimicrobial activity from stems and leaves of Paris polyphylla var. yunnanensis. Steroids. 77, 1242–1248. doi: 10.1016/j.steroids.2012.07.007
Qin, X. J., Yu, M. Y., Ni, W., Yan, H., Chen, C. X., Cheng, Y. C., et al. (2016). Steroidal saponins from stems and leaves of Paris polyphylla var. yunnanensis. Phytochemistry. 121, 20–29. doi: 10.1016/j.phytochem.2015.10.008
Shah, S. A., Mazumder, P., and Duttachoudhury, M. (2012). Medicinal properties of paris polyphylla smith:a review. J. Herb. Med. Toxicol. 6, 27–33.
Shankar Naik, B. (2018). Developments in taxol production through endophytic fungal biotechnology: a review. Orient Pharm Exp Med, (12), 1–13. doi: 10.1007/s13596-018-0352-8
Souza, C. M., Schwabe, T. M., Pichler, H., Ploier, B., Leitner, E., Guan, X. L., et al. (2011). A stable yeast strain efficiently producing cholesterol instead of ergosterol is functional for tryptophan uptake, but not weak organic acid, resistance. Metab. Eng. 13, 555–569. doi: 10.1016/j.ymben.2011.06.006
Thapa, C. B., Paudel, M. R., Bhattarai, H. D., Pant, K. K., Devkota, H. P., Adhikari, Y. P., et al. (2022). Bioactive secondary metabolites in Paris polyphylla Sm. and their biological activities: a review. Heliyon 8:e08982. doi: 10.1016/j.heliyon.2022.e08982
Tian, Y., Gong, G. Y., Ma, L. L., Wang, Z. Q., Song, D., and Fang, M. Y. (2020). Anti-cancer effects of Polyphyllin I: an update in 5 years. Chem. Biol. Interact. 316:108936. doi: 10.1016/j.cbi.2019.108936
Torres, S., Cajas, D., Palfner, G., Astuya, A., Aballay, A., Pérez, C., et al. (2017). Steroidal composition and cytotoxic activity from fruiting body of Cortinarius xiphidipus. Nat. Prod. Res. 31, 473–476. doi: 10.1080/14786419.2016.1185717
Venieraki, A., Dimou, M., and Katinakis, P. (2017). Endophytic fungi residing in medicinal plants have the ability to produce the same or similar pharmacologically active secondary metabolites as their hosts. Nephron Clin. Pract. 10, 51–66. doi: 10.1515/hppj-2017-0006
Venisetty, R. K., and Ciddi, V. (2003). Application of microbial biotransformation for the new drug discovery using natural drugs as substrates. Curr. Pharm. Biotechnol. 4, 153–167. doi: 10.2174/1389201033489847
Venugopalan, A., and Srivastava, S. (2015). Endophytes as in vitro production platforms of high value plant secondary metabolites. Biotechnol. Adv. 33, 873–887. doi: 10.1016/j.biotechadv.2015.07.004
Wang, Y. Z., Feng, B., Huang, H. Z., Kang, L. P., Cong, Y., Zhou, W. B., et al. (2010). Glucosylation of steroidal saponins by cyclodextrin glucanotransferase. Planta Med. 76, 1724–1731. doi: 10.1055/s-0030-1249938
Wang, F. Q., Li, B., Wang, Y., Zhang, C. G., and Wei, D. Z. (2007). Biotransformation of diosgenin to nuatigenin-type steroid by a newly isolated strain, Streptomyces virginiae IBL14. Appl. Microbiol. Biotechnol. 77, 771–777. doi: 10.1007/s00253-007-1216-1
Wang, L. W., Xu, B. G., Wang, J. Y., Su, Z. Z., Lin, F. C., Zhang, C. L., et al. (2012). Bioactive metabolites from Phoma species, an endophytic fungus from the Chinese medicinal plant Arisaema erubescens. Appl. Microbiol. Biotechnol. 93, 1231–1239. doi: 10.1007/s00253-011-3472-3
Wu, X., Wang, L., Wang, H., Dai, Y., Ye, W. C., and Li, Y. L. (2012b). Steroidal saponins from Paris polyphylla var. yunnanensis. Phytochemistry. 81, 133–143. doi: 10.1016/j.phytochem.2012.05.034
Wu, X., Wang, L., Wang, G. C., Wang, H., Dai, Y., Ye, W. C., et al. (2012a). New steroidal saponins and sterol glycosides from Paris polyphylla var. yunnanensis. Planta Med. 78, 1667–1675. doi: 10.1055/s-0032-1315239
Yan, L. L., Zhang, Y. J., Gao, W. Y., Man, S. L., and Wang, Y. (2009). In vitro and in vivo anticancer activity of steroid saponins of Paris polyphylla var. yunnanensis. Exp. Oncol. 31, 27–32.
Yang, B., Li, S. P., Yan, S. W., Wang, X., Li, L. Y., Dong, Z. Y., et al. (2012). Study on resource status and sustainable utilization of Paris polyphylla Smith var. yunnanensis. J. Chin. Med. Mater. 035, 1698–1700. doi: 10.13863/j.issn1001-4454.2012.10.007
Zhang, J., Cheng, Z. H., Yu, B. Y., Cordellb, G. A., and Qiu, S. X. (2005). Novel biotransformation of pentacylic triterpenoid acids by Nocardia sp. NRRL 5646. Tetrahedron Lett. 46, 2337–2340. doi: 10.1016/j.tetlet.2005.01.155
Zhao, Y., Kang, L. P., Liu, Y. X., Liang, Y. G., Tan, D. W., Yu, Z. Y., et al. (2009). Steroidal saponins from the rhizome of Paris polyphylla and their cytotoxic activities. Planta Med. 75, 356–363. doi: 10.1055/s-0028-1088380
Keywords: Paridis Rhizoma, strain C39, steroidal saponins, cytotoxicity, UPLC-Q/TOF-MS, transcriptome
Citation: Chen Y, Yu D, Huo J, Huang N, Zhang M and Du X (2022) Studies on biotransformation mechanism of Fusarium sp. C39 to enhance saponin content of Paridis Rhizoma. Front. Microbiol. 13:992318. doi: 10.3389/fmicb.2022.992318
Received: 12 July 2022; Accepted: 18 November 2022;
Published: 16 December 2022.
Edited by:
Samantha Chandranath Karunarathna, Qujing Normal University, ChinaReviewed by:
Suhail Asad, Yunnan Agricultural University, ChinaCopyright © 2022 Chen, Yu, Huo, Huang, Zhang and Du. This is an open-access article distributed under the terms of the Creative Commons Attribution License (CC BY). The use, distribution or reproduction in other forums is permitted, provided the original author(s) and the copyright owner(s) are credited and that the original publication in this journal is cited, in accordance with accepted academic practice. No use, distribution or reproduction is permitted which does not comply with these terms.
*Correspondence: Xiaowei Du, eGlhb3dlaWR1QGhvdG1haWwuY29t
Disclaimer: All claims expressed in this article are solely those of the authors and do not necessarily represent those of their affiliated organizations, or those of the publisher, the editors and the reviewers. Any product that may be evaluated in this article or claim that may be made by its manufacturer is not guaranteed or endorsed by the publisher.
Research integrity at Frontiers
Learn more about the work of our research integrity team to safeguard the quality of each article we publish.