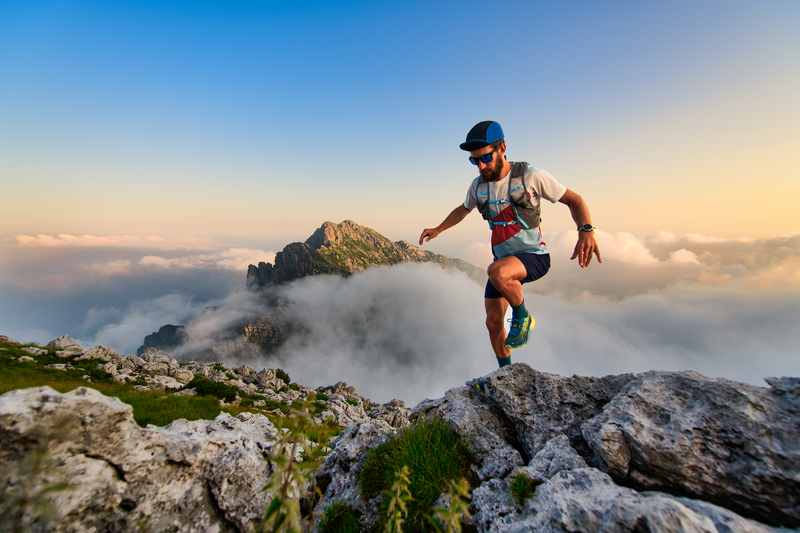
95% of researchers rate our articles as excellent or good
Learn more about the work of our research integrity team to safeguard the quality of each article we publish.
Find out more
REVIEW article
Front. Microbiol. , 20 October 2022
Sec. Infectious Agents and Disease
Volume 13 - 2022 | https://doi.org/10.3389/fmicb.2022.991678
This article is part of the Research Topic Insights in Infectious Agents and Disease: 2021 View all 37 articles
Over the last decades, research regarding innate immune responses has gained increasing importance. A growing body of evidence supports the notion that the innate arm of the immune system could show memory traits. Such traits are thought to be conserved throughout evolution and provide a survival advantage. Several models are available to study these mechanisms. Among them, we find the fruit fly, Drosophila melanogaster. This non-mammalian model has been widely used for innate immune research since it naturally lacks an adaptive response. Here, we aim to review the latest advances in the study of the memory mechanisms of the innate immune response using this animal model.
The immune system can be generally divided into an innate and an adaptive arm (Medzhitov and Janeway, 2000). For long, the dogma in immunology has been that the innate immune response is rapid and non-specific, while the adaptive immune response is slower, but antigen-specific and led to long-term immunological memory. However, it is unlikely for a crucial trait like immune memory to be restricted to the adaptive arm of the immune response when more than 95% of species do not rely on this immune system and many studies have provided pieces of evidence that some vaccines and infections protect against secondary exposure in a specific or unspecific way (Hirano et al., 2011; Conrath et al., 2015; Reimer-Michalski and Conrath, 2016). Up to date, several models have been proposed for studying innate immune memory, such as plants, vertebrates, and invertebrates (Netea et al., 2011, 2020).
The fruit fly Drosophila melanogaster has been recognized as an outstanding model to study host-pathogen interactions and immunity (Lemaitre and Hoffmann, 2007; Buchon et al., 2014; Galenza and Foley, 2019; Aromolaran et al., 2021). Over the years, several infection models have been evaluated in the fruit fly, so a deep understanding of the molecular mechanisms taking place in the host after infection has been gained [Extensively reviewed lately in Younes et al. (2020) and Michael Harnish et al. (2021)].
In this review, we have first compiled the latest insights regarding the host’s immune response to consecutive challenges and the subsequent acquisition of innate immune memory. Secondly, we outlined the relevant characteristics that make of D. melanogaster a good model for understanding innate immune memory and the methods available to assess it both orally and systematically.
An increasing number of evidences that infections or exposure to microbial-derived compounds can induce not only specific protection against reinfection but also non-specific protection against a subsequent challenge with another pathogen have been described in mice models (Di Luzio and Williams, 1978; Krahenbuhl et al., 1981; Muñoz et al., 2010; Marakalala et al., 2013; Ribes et al., 2014). It has also been described that vaccination with bacille Calmette-Guerin (BCG) conferred protection against a range of infectious diseases in both mice (Van’t Wout et al., 1992; Kaufmann et al., 2018; Covián et al., 2019; Witschkowski et al., 2020) and humans (Garly et al., 2003; Aaby et al., 2011; Biering-Sørensen et al., 2017; Rieckmann et al., 2017; Arts et al., 2018; Walk et al., 2019) as well as induced antitumoral effects through the activation of innate immune cells like monocytes and macrophages (Villumsen et al., 2009; Stewart and Levine, 2011; Buffen et al., 2014; Redelman-Sidi et al., 2014). The non-specificity of this protection, the shortened and reversible protective effect (Nankabirwa et al., 2015; Netea et al., 2016; Dominguez-Andres and Netea, 2019) and the evidence that plants and invertebrates, which only rely on innate immunity, also showed greater protection against reinfections (Dimopoulos et al., 2002; Moret and Siva-Jothy, 2003; Rodrigues et al., 2010; Norouzitallab et al., 2016; Reimer-Michalski and Conrath, 2016; Melillo et al., 2018; Lanz-Mendoza and Contreras-Garduño, 2022) revealed that immunological adaptation may also occur on the innate immunity.
The nomenclature regarding the innate immune memory acquisition process is still controversial. We describe below the basic concepts used in this field and how they have evolved as a result of deepening their study (also listed in Box 1). The concept of “trained immunity” was first proposed by Netea et al. (2011), being referred as a long-lasting functional reprogramming of innate immune cells after exposure to a microorganism or an inflammatory stimulus that lasts over time and leads to an altered response toward a second challenge. Nevertheless, this reprogramming has also been seen in epithelial stem cells, showing faster mobilization and higher induction of interferon-stimulated genes during secondary challenges (Naik et al., 2017, 2018). The first exposure of the host to the challenge is defined by the authors in the field as priming (Cooper and Eleftherianos, 2017; Sheehan et al., 2020). As perfectly detailed in the review by Bindu et al. (2022), the innate immune reprogramming happening after priming is given via epigenetic and metabolic modifications of trained cells which can lead either to an increase or a decrease in immunity (Boraschi and Italiani, 2018). The mechanisms of this reprogramming are not completely understood yet, though some evidence supports the existence of multiple regulators (Ghisletti et al., 2010; Smale et al., 2014; Fanucchi et al., 2019; Natoli and Ostuni, 2019). In addition, new studies have also suggested changes in DNA methylation patterns and changes in cellular metabolism as a mediator of trained immunity (Donohoe and Bultman, 2012; Cheng et al., 2014; Arts et al., 2016; Verma et al., 2017; Das et al., 2019; Penkov et al., 2019).
BOX 1. Innate immune memory related terms.
RNA interference (RNAi) was recently put in light as an important immune priming mechanism which confers an adaptive antiviral response (Tassetto et al., 2017). This mechanism is based on a post-transcriptional gene regulation mechanism by which small interfering RNAs (siRNAS) induce the sequence-specific degradation of homologous messenger RNA (mRNA) sustaining the antiviral effect along time after first exposure (Meng and Lu, 2017). In addition to the cell-autonomous immunity conferred to the infected cell, this antiviral RNAi signals have shown its ability to spread systematically conferring innate immune memory (Tassetto et al., 2017). This antiviral mechanism and its priming capacity have been reported in vertebrates, invertebrates and plants, although the spreading mechanisms differ between taxa (Meng and Lu, 2017; Tassetto et al., 2017; Cooper and Van Raamsdonk, 2018; Gourbal et al., 2018; Javdat and Tamara, 2020).
Despite this, the term trained immunity is also used by various authors to refer to the immune phenotype the cells obtain after a primary challenge which allows them to react quicker and stronger to secondary one, thus producing an increase in the immune response to a stimulus (Netea et al., 2020); instead, other authors suggest more accurate terms which describe this phenomenon such as “non-specific acquired resistance,” “potentiation,” or “trained potentiation” (Boraschi and Italiani, 2018). On the other hand, although this reprogramming of innate immunity to an inflammatory profile provides a great advantage in host defense, it may also be detrimental in the context of chronic inflammatory diseases. As compensatory mechanism it has also been shown the opposite reaction, being the priming-induced downregulation, which results in a reduced response after a secondary stimulus (Cooper and Eleftherianos, 2017; Boraschi and Italiani, 2018; Uribe-Querol and Rosales, 2020). Authors will be referring as “tolerance” or “trained tolerance” to this also considered innate memory phenomenon (Boraschi and Italiani, 2018). Tolerance is also induced and maintained by epigenetic changes. Analysis of this mechanism revealed that lipopolysaccharides (LPS) administration could induce alterations in the chromatin that silenced pro-inflammatory genes but not antimicrobial effector genes (Seeley and Ghosh, 2017; Hajishengallis et al., 2019; Lajqi et al., 2021; Figure 1).
Figure 1. Changes after the induction of innate immune memory. Priming is characterized by an activation of gene expression in innate immune cells that is sustained over time and does not return to basal levels before the second challenge. Often the response to the second stimulus is synergistic with the first one. In trained immunity the gene expressions levels return to basal levels when the first stimulus is removed, but epigenetic changes persist favoring a faster and higher immune response in subsequent infections. Finally, tolerance is the opposite to trained immunity, where after activation by a first stimulus and returning to basal levels the immune response is reduced in subsequent challenges. The flat dotted line represents the basal activation levels of the innate immune response in absence of infection. Adapted from Divangahi et al. (2020) and Netea et al. (2020).
Whether adaptation of innate immune cells will enhance or reduce the immune response after a second challenge will depend on the dose and the duration of the first stimulus as well as on the kind of stimuli which not necessarily has to be the same source in both challenges (Divangahi et al., 2020). Based on the functional status of these cells before the second challenge, Divangahi et al. (2020) published a review with an updated definition of the different adaptive programs a cell can incorporate. In addition to trained immunity and tolerance, in which the immune genes expression return to basal levels after the first stimulus is removed and the response after the second challenge is increased or decreased, respectively, these authors also define differentiation and priming as two adaptive programs affecting a cell innate immune response. The change of an immature cell into its mature counterpart is defined as cell differentiation and often comes together with morphological changes; although differentiation can occur during homeostatic conditions, differentiated cells can be trained as well after infection or vaccination (Lavin et al., 2014). On the other hand, when the first stimulus changes the state of the innate immune cells and the active gene expression does not return to basal levels before the second challenge it is named “priming,” and often the response to the second stimulus is synergistic with the first one (Divangahi et al., 2020; Figure 1).
Upon infection, there are two major strategies to fight disease: resistance and tolerance. Relating these two types of host’s responses to the adaptive programs exposed by Divangahi et al. (2020) trained immunity and priming would relate to an increased host resistance to reinfection, and tolerance would refer to a tolerogenic host response to reinfection. In this regard, resistance strategies will aim at killing the pathogen or inhibit its proliferation (Boraschi and Italiani, 2018). However, mounting an immune response is energetically costly, leading to a reduction in nutrient storage, growth, and reproduction in the organism. This suggests a strong link between immunity and host fitness (Stearns, 1989; Schwenke et al., 2016). The health status of a host does not always depend on the number of pathogens it can bear. Instead, it depends on the ability to reduce the effects of the damage and stress (Chow and Kagan, 2018). Tolerance strategies will reduce the negative effect on fitness caused by the infection, but will not have an impact on pathogen fitness (Howick and Lazzaro, 2017). Since the mechanisms of disease tolerance do not reduce pathogen load, they should promote the transmission of the infectious agent in the population. Some pathogens like Salmonella enterica typhimurium induce tolerance to increase their spread. However, throughout evolution, reducing infections, tolerance and resistance mechanisms became highly interconnected (Howick and Lazzaro, 2017). For example, by increasing host fitness, tolerance responses will aim at reducing tissue damage and thus, allowing the resistance mechanisms to work more potently (Martins et al., 2019). Besides, by increasing host fitness, tolerance mechanisms give time to induce resistance strategies to eliminate the pathogen (Chow and Kagan, 2018; Sheehan et al., 2020). Disease tolerance can also be important in cases where the immune response is the one generating the damage, for example, in the case of sepsis (Chow and Kagan, 2018). In some situations, the activation of one pathway can contribute to tolerance and resistance mechanisms. Autophagy, for example, can reduce pathogen burden, thus contributing to disease resistance. However, in other contexts, it can contribute to disease tolerance by reducing endothelial barrier destruction (Chow and Kagan, 2018). Evidence suggests that different organs have different tolerance capacities since they can be more susceptible to damage or have a higher tissue replication rate, allowing a higher reparation rate. Moreover, the consequences of tissue damage can vary according to the tissue. For instance, damages in the endothelium can compromise vascular integrity and lead to ischemia and tissue necrosis, whereas skin damage may not be life-threatening (Medzhitov et al., 2012).
The use of D. melanogaster as a model has provided a huge insight into the mechanisms of action of the innate immunity, as insects rely solely on this type of response thus avoiding the variability that adaptive mechanisms imply. In addition, D. melanogaster presents a high degree of conserved features with vertebrates including immune cascades, signal transduction pathways, and transcriptional regulators (Younes et al., 2020), as well as a significant amount of well-conserved homologs of disease-causing genes in humans (Bier, 2005). These facts make the fruit fly a good model for studying innate immune responses and gene functionality in basic research.
As in vertebrates, D. melanogaster immune system is also divided into humoral and cellular responses. The main mode of action of the humoral response in D. melanogaster is the production of antimicrobial peptides (AMPs). After a systemic infection, AMPs are released into the hemolymph where they persist for several days and can protect the flies against a second exposure to the pathogen (Boman et al., 1972). AMPs can act synergically or be highly specific depending on the pathogen (Imler and Bulet, 2005; Hanson et al., 2019, 2022; Hanson and Lemaitre, 2020). This response is mediated by the fat body, which is the equivalent of the liver in mammals and represents the main immune-responsive organ in the fly (Lemaitre and Hoffmann, 2007). Barrier epithelial cells are also able to secrete AMPs and reactive oxygen species (ROS) in response to a localized infection (Tzou et al., 2000). Besides this, lower levels of AMPs are also expressed from the hemocytes, muscles, Malpighian tubules, and neuronal tissues (Charroux and Royet, 2009; Badinloo et al., 2018). Three main signaling pathways have been described to play a role in the regulation of immune genes induced after infection. The Toll and the immune deficiency (Imd) pathways regulate the majority of immune genes, including the production of AMPs. The Toll pathway is activated in response to the detection of lysine (Lys)-type peptidoglycan of Gram-positive bacteria, and β-glucans of yeasts and fungi, as well as through the sensing of danger signals, like microbial proteases, or abnormal cell death. On the other hand, the Imd pathway is activated through the detection of diaminopimelic acid (DAP)-type peptidoglycan from Gram-negative and certain Gram-positive bacteria (Buchon et al., 2014; Figure 2). The study done by Kutzer and Armitage (2016) revealed that the Imd and Toll pathways have different kinetics since the AMPs triggered by those pathways were observed at different times after infection. An infection of flies with a gram-negative bacterium such as E. coli that triggered the Imd pathway showed the highest AMP concentration after 6 h. In contrast, when challenged with a gram-positive bacterium like Lactococcus lactis, thus triggering the Toll pathway, the peak of AMPs was observed 24 h post-infection. These differences in immune pathways contribute to the differences in the clearance of different pathogens (Kutzer and Armitage, 2016). In addition, a different pattern of activation is also observed between males and females (Duneau D. F. et al., 2017; Belmonte et al., 2020). Finally, Drosophila also has a complete yet more simple JAK/STAT signaling pathway, which is involved in diverse biological processes, including early and late development, innate immunity, germ-cell adhesion, and inhibition of apoptosis (De Gregorio et al., 2001; Boutros et al., 2002). This pathway also contributes to the immune response by inducing the transcription of thioester-containing protein genes and Turandot stress genes, which, both play a role in Drosophila defense against pathogens (Lagueux et al., 2000; Agaisse et al., 2003; Dostálová et al., 2017). In addition, a communication axis between the humoral pathway Imd and JAK/STAT which controls fly antiviral immune response has been recently described (Shen et al., 2022).
Figure 2. Immune recognition of microorganisms in Drosophila. The two major pathways that sense bacteria and fungi in fruit flies are the Toll pathway (left) and the immune deficiency (Imd) pathway (right). Both pathways function in the fat body for the production of antimicrobial peptides (AMP) by activating the expression of NF-κB-like factors, which are highly conserved among species. In addition, the Imd pathway also functions in the epithelial surfaces. The Imd pathway is activated when DAP-type peptidoglycan from gram-negative bacteria, and some gram-positive, binds to Peptidoglycan Recognition Proteins (PGRPs), and this activation leads to the generation of AMP and synthesis of Duox enzyme for the production of reactive oxygen species (ROS). Gram-positive bacteria contain lys-type peptidoglycan, which is recognized by PGRP-SA and Gram Negative Bacteria Protein (GNBP) 1, and GNBP3 binds to β-glucans of yeasts and fungi, leading to the activation of the Toll pathway. This pathway can also be triggered by danger signals like proteases or abnormal cell death that activates the protease Persephone (Psh). In all cases, the activation of the Toll pathway triggers a proteolytic cascade that activates the protease Spätzle- processing enzyme (SPE). This protein will cleave Spätzle (Spz). As a result of the activation of the Toll pathway, the transcription factors Dorsal-related immunity factor (Dif) or Dorsal (Dl) will translocate to the nucleus, thus inducing the expression of AMP genes like drosomycin. Similarly, the activation of the Imd pathway induces the nuclear translocation of the transcription factor Relish (Rel) and induction of the expression of AMP genes, such as diptericin. The generation of ROS is induced by the activation of Duox in the presence of uracil. This is caused by the activation of a G protein-coupled receptor (GPCR), which promotes the release of calcium from the endoplasmic reticulum. This signaling pathway, together with the activation of the Imd pathway, contributes to the expression of the Duox enzyme during infection. Atf2, activating transcription factor 2; Dredd, death-related ced-3/Nedd2-like caspase; Fadd, FAS-associated death domain ortholog; Gαq, G protein αq-subunit; IKK, inhibitor of NF-κB kinase; MKK3, MAPK kinase 3; modSP, modular serine protease; Tak1, TGFβ-activated kinase 1. Adapted from Buchon et al. (2014) and Younes et al. (2020).
Cell-mediated innate immunity of Drosophila comprises of immune blood cells, which can be found freely circulating in the hemolymph or associated with diverse tissues. These cells, which are collectively called hemocytes, can be differentiated according to their morphology and immunological functions in plasmatocytes, lamellocytes and crystal cells (Lanot et al., 2001; Hartenstein, 2006; Hultmark and Andó, 2022). Recently, a new class of cells have been identified in Drosophila, the primocytes, whose functions are not fully elucidated, but they are thought to regulate the larval hematopoietic organ which, in turn, controls the hematopoiesis of other hemocytes (Hultmark and Andó, 2022). In addition, the presence and proportion of these cells may vary depending on the developmental stage the animal (Honti et al., 2014). Phagocytosis represents a fundamental process of the innate immune response and in the maintenance of tissue homeostasis. In D. melanogaster this process is mainly performed by plasmatocytes, which represent up to 90% of the total circulating hemocytes (Wang et al., 2013) and appear to perform the functions of vertebrates’ macrophages and neutrophils (Stramer et al., 2005). Melanization is another predominant immune response in insects based on the production and release of melanin around intruding microorganisms (Christensen et al., 2005). This response is involved in wound healing and hemolymph coagulation, and is mediated by the crystal cells (Ashida and Brey, 1995; Söderhäll and Cerenius, 1998; Tang, 2009; Leitão et al., 2020; Younes et al., 2020). Lastly, lamellocytes are differentiated from plasmatocytes when the cell-mediated innate immune response is induced upon infection with parasitoid wasp, wounding or artificially by genetic induction, and are in charge of the encapsulation of foreign bodies that are too large to be phagocytosed, as well as melanization (Kounatidis and Ligoxygakis, 2012; Honti et al., 2014; Dudzic et al., 2015; Vlisidou and Wood, 2015; Leitão et al., 2020).
In Drosophila, the mechanisms by which a septic infection causes a systemic response controlled by the fat body have been well-characterized, even though these types of infections are rare. However, although the so-called natural infections take place constantly, the epithelial immune response remains less characterized. It is known that this local response is mainly regulated by the Imd-pathway (Onfelt Tingvall et al., 2001) and is shaped against different microbes via the JAK/STAT and the JNK signaling pathways (Wagner et al., 2009). In 2013, a microarray analysis performed by Gendrin et al. (2013) showed that the induction of Imd-dependant genes varies substantially among tissues with only very few “universal genes” being expressed in the fat body, the gut, and the trachea (Gendrin et al., 2013). This set of genes includes mainly AMPs and pathway components.
Specifically in the gut, local immunity includes physical and chemical barriers as well as a cellular response (Buchon et al., 2013). ROS are induced by two enzymes: dual oxidase (Duox) is stimulated through pathogen-derived uracil and peptidoglycan (Lee et al., 2013) and NADPH oxidase (Nox) is induced by microbiota-derivate lactate (Iatsenko et al., 2018). In the gut, Duox-derived ROS are mainly involved in immune response and repair tissue damage, while Nox-derived ROS regulate epithelial renewal (Iatsenko et al., 2018). However, excessive production of ROS is ultimately toxic to the host and induces epithelial cell death and early ageing, thus Duox expression is tightly regulated through the p38 mitogen-activated protein kinase–activating transcriptional factor 2 (p38 MAPK–Atf2) pathway (Ha et al., 2009b). The uracil secreted by some pathogenic bacteria activates the phospholipase Cβ (PLCβ), which in turn activates the p38MAPK-Atf2 pathway. The PLCβ pathway also promotes the release of Ca2+ from the endoplasmic reticulum to bind and activate Duox (Ha et al., 2009a). In response to commensals, the levels of both uracil and peptidoglycan are lower and the concentration of cytosolic Ca2+ is reduced, thus Duox activity is kept minimal (Ha et al., 2009b).
Several studies have proved that an interorgan communication occurs in D. melanogaster by proving that local intestinal infections are able to induce a systemic response in the fat body (Basset et al., 2000; Foley and O’Farrell, 2003; Wu et al., 2012). The simplest explanation would be that when an ingested pathogen is able to break the intestinal epithelial barrier and enter into the hemolymph, circulating immune cells phagocyte them and activate a systemic immune response in the fat body, as observed for the bacteria-derived peptidoglycan (PGN) (Charroux et al., 2018). However, some studies revealed Ecc15 is not detectable in the hemolymph of larvae after ingestion, thus proving that it is unlikely that the bacteria directly interact with the hemocytes in the hemolymph (Basset et al., 2000; Foley and O’Farrell, 2003). Although the specific mechanisms involved have not been extensively explored yet, it has been shown that gut-derived ROS might interact with circulating hemocytes and regulate diptericin production in the fat body cells (Amcheslavsky and Ip, 2012). Other studies also showed a correlation between gut-expressed PGRP-LE with the levels of AMPs expression in the fat body (Paredes et al., 2011; Bosco-Drayon et al., 2012). Finally, a cross-talk between muscles and immunity via JAK/STAT activation has also been described (Yang and Hultmark, 2016). This interorgan communication mechanisms have been recently extensively reviewed (Liu and Jin, 2017; Figure 3).
Figure 3. Schematic representation of the interorgan communication during infection in Drosophila melanogaster. In response to ingested pathogens, these microorganisms might either break the gut epithelium themselves or release peptidoglycan (PGN) from their cell walls into the hemolymph. The enterocytes (ECs) are also able to release reactive oxygen species (ROS) in response to tissue damage. These circulating signals are sensed by the hemocytes located close to the gut epithelium. Hemocyte-expressed Decapentaplegic (Dpp) and Unpaireds (Upds) during early stages of the infection activate the intestinal stem cells (ISCs) proliferation to cope with tissue damage. Hemocytes-derived Upds also induce accumulation of the transcription factor dFOXO in the fat body via JAK/STAT pathway. Activation of dFOXO induces expression of AMPs and a systemic metabolic dysregulation that mobilizes energy resourced toward phagocytic cells. Finally, infection-induced Upd3 in enterocytes activate the JAK/STAT signaling pathway in visceral muscles (VM), which in turns promote ISCs proliferation, as well as activation of the close-located hemocytes.
Several methods have been developed that aided in the understanding of the processes related to innate immune memory in the D. melanogaster model. According to the research question and the experimental design, different kinds of infection models could be used. For inducing a systemic infection there are several methods available; the most used ones are needle pricking and injection pumping or microinjection (Troha and Buchon, 2019). Needle pricking involves dipping a needle into a solution containing the pathogen, which is later used to prick anesthetized flies. Inoculation can be done in the abdomen or thorax, yet minimal damage should be generated. Since a wound is produced, infection is first local and later disseminates systemically, allowing to assess both types of infections. This type of inoculation is quick; hence this method can be used for the infection of large amounts of flies. However, a limitation of this method is that the infection dose can be variable.
In addition, to be able to observe dissemination and systemic infection, very small number of bacteria should be inoculated by needle pricking to minimize the effect of the local infection (Apidianakis and Rahme, 2009). Therefore, some authors agree that needle pricking would be most appropriate injection method for highly virulent bacteria such as Pseudomonas aeruginosa and Staphylococcus aureus (Apidianakis and Rahme, 2009; Lee et al., 2018). In case a more precise dose is needed, it is suggested to use the microinjection method. Here, a needle is charged with a specific volume of pathogen solution, and using a pumped injector, the solution is introduced in the abdomen or thorax, entering directly into the haemolymph, thus generating a systemic infection. Although more reproducible, this method is slower and requires specific equipment. Assessing the circumstances, an oral infection could be desirable. Unlike the injecting methods, feeding results in local infection primarily in the gut epithelium (Lee et al., 2018). However, it should be noted that in oral infections the infective dose is also a limitation, as it is variable and difficult to monitor (Troha and Buchon, 2019).
In order to assess innate immune memory acquisition, flies have to be primed by exposing them to either a sublethal dose of a pathogen, a heat-killed pathogen or a pathogen-derived material previously to the second challenge (lethal infection) (Sheehan et al., 2020). Priming can also be performed both orally and systematically, making sure in the last one that both challenges are done one on each side of the fly body (Kutzer et al., 2019). The protective effect is then assessed by comparing survival and pathogen loads between primed and non-primed flies in response to the second challenge.
Other methods to assess the immunological mechanisms behind the innate immune memory acquisition in D. melanogaster, include analysis of gene expression of AMPs by RT-qPCR, RNAi, or RNA-seq; protein levels quantitation, phagocytic or melanization activities and release of ROS upon infection (reviewed in Troha and Buchon, 2019). Measuring expression levels of AMPs together with survival could bring surprising results, as it has been observed by several authors that on certain occasions, there were no changes in survival after oral administration of non-pathogenic bacteria, yet levels of AMPs were increased (Patrnogic et al., 2018). In addition, on a genetic level, the process of innate immune memory is thought to be associated with epigenetic changes in the chromatin. To evaluate such changes, methyl marks in histone H3 can be measured, as well as the concentration of enzymes associated with chromatin remodeling, like histone lysine methyltransferases (Bonnet et al., 2019).
Last but not least, several studies have taken advantage of the multiple genetic-editing tools available in the Drosophila model to assess the mechanisms of innate immune acquisition (Prakash and Khan, 2022). Most studies have used Toll- and Imd-impaired mutants to validate the role of these key pathways in the defense against subsequent infections (Apidianakis et al., 2005; Pham et al., 2007; Aymeric et al., 2010; Christofi and Apidianakis, 2013; Wen et al., 2019; Cabrera et al., 2022). Other studies have used fly lines unable to mount a proper cellular immune response (Christofi and Apidianakis, 2013; Chakrabarti and Visweswariah, 2020), as well as ROS-deficient individuals (Chakrabarti and Visweswariah, 2020). In addition, this model also offers the possibility to study wild populations to assess whether host environment accounted for the difference in resistance patterns observed after infection with the same pathogen (Corby-Harris and Promislow, 2008).
Few studies have been performed in D. melanogaster addressing this topic (Table 1). Pham et al. (2007) found that the first exposure to a non-lethal dose of Streptococcus pneumoniae or to the heat-killed bacteria conferred protection against a second exposure to lethal doses of the same pathogen. This response was found to last the rest of the fly’s life, to be specific and to be mediated by the Toll pathway together with phagocytes, but not the Imd pathway or AMPs (Pham et al., 2007). They also showed that a wide range of heat-killed bacteria, including S. typhimurium, Listeria monocytogenes, and Mycobacterium marinum, which are known to be potent immune activators, did not confer protection to subsequent infections neither with the same pathogen nor with S. pneumoniae (Pham et al., 2007). Similar studies were performed with P. aeruginosa. Priming flies with the avirulent P. aeruginosa strain, CF5, revealed that both Toll and Imd pathways and phagocytosis were necessary for the induction of protection against subsequent infections with the more virulent strain PA14 and that heat-killed CF5 did confer protection although this was shorter (Apidianakis et al., 2005; Christofi and Apidianakis, 2013).
Some authors have evaluated the protection conferred by exposure of D. melanogaster to an avirulent Escherichia coli against subsequent infection in a short period of time; interestingly, protection on both occasions is driven by the presence of AMPs from the Imd signaling pathway in fly hemolymph prior to challenge (Apidianakis et al., 2005; Aymeric et al., 2010). More recent studies have gone deeper into the mechanisms of these innate immune adaptations. Chakrabarti and Visweswariah defined the ROS production and accumulation in hemocytes after an injury as the key regulators for the induction of the Toll pathway, which in turn confers protection to subsequent infections with Enterococcus faecalis (Chakrabarti and Visweswariah, 2020). Nevertheless, this protection conceived by the wound lasted a maximum of 5 days. On the other hand, when Drosophila was primed with a low dose of E. faecalis, the protection against the second infection with the same pathogen was observed to las for at least 7 days (Cabrera et al., 2022). More insights on the innate immune memory acquisition were described by Tassetto et al. (2017) when D. melanogaster was systemically infected with Sindbis virus (SINV). They found that the hemocytes acquired immunological memory in the form of stable virus-derived complementary DNA and that they were able to systemically disseminate it through RNAi-containing exosomes.
Lack of protection after priming has also been documented. For example, systemic injection of killed Lactococcus lactis, Pseudomonas entomophila, or Providencia burhodogranariea did not confer the expected protection against subsequent homologous infections (Kutzer et al., 2019; Acuña Hidalgo and Armitage, 2022); just as systemic infections with a low dose of Drosophila C Virus did not protect against subsequent challenge with a lethal one (Longdon et al., 2013). Considering all of the above, protection in D. melanogaster is achieved differently depending on the priming agent. This protection seems to be correlated with the immunity pathways activated by this priming species that allows protection, specific or not, against the challenge (Pham et al., 2007; Aymeric et al., 2010; Christofi and Apidianakis, 2013).
Drosophila melanogaster and mammalian intestines are similar both in structure and function, but D. melanogaster has a more simple microbiota composed of only 2–30 bacterial species (Wong et al., 2011). However, as seen in mammals, the microbiota can also influence the immune response and shape intestinal function and development in insects (Ryu et al., 2008; Liu et al., 2017; Capo et al., 2019).
Previous research done in which non-pathogenic or heat-killed bacteria were administrated by food to different insects showed, in certain cases, an increased level of AMPs or increased survival. For example, pre-exposure with non-pathogenic E. coli can protect larvae of tobacco hornworm Manduca sexta from a Photorhabdus luminescens infection (Eleftherianos et al., 2006). Moreover, feeding the larvae of cabbage looper Trichoplusia ni with non-pathogenic E. coli and Micrococcus luteus has shown to increase the antibacterial activity (Freitak et al., 2007). These studies serve as a background to establish that microorganisms ingested with the diet, even if non-pathogenic, can trigger an immune response that could reduce the risk of severe disease from other infections.
In D. melanogaster oral induction of immune memory has also been assessed (Table 1). Contrary to what happens systematically, a transstadial assay in which larvae were exposed to DCV showed an increase in the tolerance to infections with the same virus in adulthood. This protection was shown to be species-specific as well as RNAi pathway dependent (Mondotte et al., 2018). Specificity of protection was also tested by Wen et al. (2019) by oral priming of D. melanogaster with heat-killed bacteria. This study evidenced an heterogeneous protection gram-positive/gram-negative-specific (Wen et al., 2019). In addition, this study also found differences among the levels of AMPs expression between males and females. Females showed higher levels of AMPs after short-term oral priming with killed bacteria, which translated to higher protection against subsequent infection. On the other hand, further research has proven that a previous encounter with non-pathogenic bacteria on the diet failed to protect fruit flies from an infection with entomopathogenic bacteria (Patrnogic et al., 2018). To delve into the mechanisms of fly immunity, priming of flies through diet has also been carried out by administering either suppressors or inducers of the immune response, Juvenile Hormone (JH) or cyclic dinucleotide (CDN), respectively (Schwenke and Lazzaro, 2017; Segrist et al., 2021). Results of these studies showed the impact of the hormonal pathway in D. melanogaster in the regulation of the immune response (Schwenke and Lazzaro, 2017), as well as the possibility of bacterial-derived CDNs to induce immunity in microbiota-deficient flies (Segrist et al., 2021).
As another memory mechanism, Madhwal et al. (2020) showed that the systemic elevation of GABA levels neuronally upon olfactory stimulation of D. melanogaster (via oral), specifically promoted pre-differentiation of lamellocytes and thus, a more efficient cellular response when challenged with Leptopilina boulardi parasitoid wasp. Moreover, the protection of D. melanogaster by oral priming has not only been proven against subsequent infections. Jacqueline et al. (2020) showed that previous exposure to Pectobacterium carotovorum protected flies with a cancer-inducing genotype; results evidenced the importance of both Diptericin and Drosomycin in tumor cell death, and thus, in tumor regression. All these findings suggest that protection provided by an oral exposure to a particular microorganism or antigenic agent is possible in D. melanogaster. However, further studies on the mechanisms of immunity are needed in search of more consistent evidence of immunological memory.
It has also been described in insects that innate immune memory can pass down from primed parent individuals to the offspring (Dhinaut et al., 2018; Vilcinskas, 2021). This kind of memory is currently named as Transgenerational Immune Priming (TgIP) and has been studied in a wide range of insects, although only a few used the Drosophila model. However, the exact mechanism is not yet fully understood, as well as how many generations it lasts (Sheehan et al., 2020). One of the possible mechanisms is the transgenerational transfer of microbial elicitors, in which bacteria or bacterial fragments ingested by female individuals are translocated to their eggs following the same route as bacterial symbionts, thus providing the offspring with the capacity to mount a more specific innate immune response against these bacteria (Herren et al., 2013; Freitak et al., 2014; Knorr et al., 2015). However, some studies also provide evidences of paternal TgIP in insects which are thought to be induced by epigenetic modifications transferred to the germ cells and transmitted to the offspring (Soubry et al., 2014; Zenk et al., 2017).
Using the D. melanogaster model, Bozler et al. (2020) showed that increased maternal Peptidoglycan Recognition Protein LB (PGRP-LB) expression levels after exposure to parasitic wasp correlated with a more successful immune response to the parasite in the offspring mainly mediated by a rapid cellular activation. Same implication of the cellular immune response in TgIP was observed in flies primed with another parasitic wasp species (Leitão et al., 2020). Mondotte et al. (2020) also described an antiviral transgenerational immune priming in both D. melanogaster and the mosquito Aedes aegypti after parental priming with different single stranded RNA viruses with specificity in the protection of the progeny for several generations. On the contrary, it has also been seen how the offspring inherited indirect costs associated with the immune response to the infection of the progenitors, having shorter lifespans (Linder and Promislow, 2009).
Once flies are primed, measurement of tolerance and resistance to the subsequent infection is needed to assess the acquisition of innate immune memory. In this regard, different theoretical models have been used along time (Råberg et al., 2008; Lefèvre et al., 2011; Gupta and Vale, 2017). The application of these models is not absolute and aspects such as the experimental model used, its lifespan, or the possibility of carrying out a certain test along the course of infection, will determine which model is the most appropriate at each moment. In the literature, resistance is defined as the inverse of the pathogen concentration (number of parasites per host or per unit tissue); when all the other variables are equal, a lower Y-intercept means the host is more resistant. Tolerance, on the contrary, is usually defined as the slope of a linear regression model when plotting host fitness against infection intensity in 2- dimensional health-by-microbe space; the flatter the slope, the higher the tolerance (Råberg et al., 2008). If the slope varies among groups, such that the fitness of some hosts declines faster with increasing inoculation doses, this means there is variation in tolerance among hosts types (Råberg et al., 2008; Ayres and Schneider, 2012).
In ecology and evolutionary biology, this description of how specific individuals respond to different environmental conditions is known as the “reaction norm” and considers the different pathogen burdens that can be applied to infections (Råberg et al., 2007). Depending on the animal model, host fitness can be measured differently. In mammals, the most often measured effects of infection on host health are anemia and weight loss and they are plotted against the peak pathogen density (Råberg et al., 2008). In insects, counting the Bacillary Load Upon Death (BLUD, in case of bacterial infections) is interesting as dead caused by disease is correlated with the ability of the pathogen to proliferate and the ability of the host to react against that infection. Therefore, as explained, the maximal bacterial load that flies can cope with before death represents a measure of host disease tolerance (Duneau D. et al., 2017). Moreover, the Set Point Bacterial Load (SPBL), meaning measuring the colony forming units (CFUs) at different time points, is also a useful observation to dissect the mechanism taking place throughout the course of infection. Studies done with D. melanogaster aim to measure bacillary load against inoculation dose as a measure of resistance (Corby-Harris et al., 2007; Kutzer et al., 2019; Wen et al., 2019), and both host’s health or fecundity as the host-fitness parameter against inoculation dose to measure tolerance (Kutzer et al., 2019).
When using this linear model, four different patterns could be observed. A low survival with low pathogen load could explain the metabolic costs associated with mounting an immune response. When a high mortality is associated with a high bacterial load, it might be due to the high costs of fighting a virulent microorganism. High survival rates associated with low pathogen loads suggest that a robust immune response is present, and the host is resistant to the infection. In contrast, a high survival with high bacterial loads suggests a tolerant phenotype in which the host can survive longer since it does not need to use sources to clear the infection (Corby-Harris et al., 2007; Kutzer and Armitage, 2016; Duneau D. et al., 2017; Box 2). In contrast to the linear models, Gupta and Vale proposed a 4-parameter non-linear model in D. melanogaster to estimate disease tolerance with more detail (Gupta and Vale, 2017). While linear approaches bring light to the rate at which hosts lose health, non-linear approaches are useful to clarify the dose that causes this lost in health or the severity of the infection (Gupta and Vale, 2017).
BOX 2. Patterns associated with an infection according to linear models.
Over the years, scientists have used the D. melanogaster model to study innate immunity. However, the mechanisms behind the induction of innate immune memory in this host model are still not fully elucidated. Previous research has shown that a former encounter of D. melanogaster with heat-killed, not pathogenic bacteria or low dose of pathogens could confer protection from future infections with pathogenic microorganisms (Pham et al., 2007; Cooper and Eleftherianos, 2017; Patrnogic et al., 2018).
Interestingly, this mechanism cannot be generalized, so certain parameters have to be standardized first to have a better comparison of the results. Susceptibility to infection in flies can vary according to age, sex, genetics, feeding and physiological and environmental factors, such as temperature, resources, humidity, time of the day or light; or even according to the presence of other infections or single-nucleotide polymorphisms (SNPs) that could confer greater vulnerability to certain pathogens (Merkling and Van Rij, 2015; Duneau D. F. et al., 2017; Kutzer et al., 2019; Troha and Buchon, 2019). However, the advantage of this model is that experiments can be repeated easily and in an affordable manner. Such a quantity of factors regulating fly immune response explains why not every challenge with a non-lethal dose of a pathogen induces protection from further encounters, and why the duration of this protection is variable (Hillyer, 2016; Hajishengallis et al., 2019), although it was suggested that it might pass to the progeny (Mondotte et al., 2020). The differences on the kinetics of the different immune pathways that are activated upon infection, as well as the sexual dimorphism that exists when mounting an immune response, also influence the acquisition of innate immune memory. In addition, a different pattern of activation is observed between sexes (Duneau D. F. et al., 2017; Belmonte et al., 2020), which translate with different protection levels against a second exposure to the pathogen (Wen et al., 2019).
As tolerance and resistance confer protection to a host at different levels when infected, several models to measure their acquisition have been described (Råberg et al., 2008; Lefèvre et al., 2011; Gupta and Vale, 2017). Nevertheless, the choice of the model will depend such as the nature of the host or the answers we want to obtain. Recent research on this topic pointed out that tolerance and resistance mechanisms can have a differential importance throughout the course of the infection. For example, during the early phases of Listeria monocytogenes infection in mice, resistance mechanisms were predominant, whereas tolerance was more important during the last stages. This also points out the fact that both mechanisms are interconnected (Kutzer et al., 2019). Examples of immune priming that could protect insects from future infections were seen when the larvae of wood tiger moth Parasemia plantaginis received a diet containing a low dose of Serratia marcescens or non-pathogenic E. coli. Larvae primed with S. marcescens were later protected from an otherwise lethal injection of the same pathogen. This, however, was not the case of larvae primed with E. coli. This study suggests that pathogen recognition at the midgut level can be important for conferring systemic immunity (Mikonranta et al., 2014). Moreover, other examples include the oral administration of peptidoglycans from the cell wall of P. aeruginosa, or heat-killed P. aeruginosa conferred protection against a following infection with P. aeruginosa in silkworms. However, silkworms receiving heat-killed S. aureus did not show an increased survival after infection with P. aeruginosa (Miyashita et al., 2015). This review underlines the lack of standardized method for assessing the type of protection provided by priming the host with different stimuli, as each author focused on different parameters, such as survival, pathogen load or gene expression. However, the assessment of all three parameters would be of interest in order to define the mechanism behind innate immune memory.
Immune priming in D. melanogaster has been studied on several occasions. And, although the results verify the great variability that the fly presents in terms of protection, they allow us to obtain an increasingly precise overall view of the mechanisms behind trained immunity. Most of the studies conclude that a certain specificity between the priming pathogen, the innate immune response triggered by the host and the protection that this provides against subsequent infections. This specificity has been observed at species level (Pham et al., 2007) and sequence-specific in viruses (Mondotte et al., 2020). Fewer studies have also shown less specific protection e.g., between Gram-negative/Gram-positive species (Wen et al., 2019). Nevertheless, in those studies evidencing protection against subsequent infections is not easy to discriminate if this protection is due to innate immune memory acquisition or due to the fact that the immunity is still stimulated in the flies at the time of the second challenge (Chambers et al., 2019). Further studies which test maximum times in between priming and challenge, as well as the maximum duration of protection would help to elucidate this. In addition, it would be interesting to perform more priming tests in this animal model to further elucidate the specificity of protection and the ability to transmit this protection to offspring. In addition, oral priming prior to the second challenge has been poorly studied in this experimental model. A more exhaustive study of the subject would be interesting in order to screen protective components against infection with the possibility of further translation to other animal models. The use of Drosophila to assess oral priming might be very useful considering the possibility to study local adaptation of wild-caught strains, which are in constant contact with different pathogen-rich environments (Corby-Harris and Promislow, 2008).
Bearing all of the above in mind, it is still a challenge to decipher innate immune memory mechanisms induced after infection with a pathogen and further research is needed. As selecting the ideal model is crucial for future developments, non-mammalian models, especially D. melanogaster, are a good alternative to deep into its study.
All authors listed have made a substantial, direct, and intellectual contribution to the work, and approved it for publication.
This research was funded by “La Caixa” Foundation (ID 100010434), under agreement LCF/PR/GN16/10290002, Spanish Government-FEDER Funds through PI17/01511 grant, and the “CIBER Enfermedades Respiratorias” Network (CIBERES). RK, registered in the EMJMD LIVE (Erasmus + Mundus Joint Master Degree Leading International Vaccinology Education), co-funded by the EACEA (Education, Audiovisual and Culture Executive Agency, award 2018-1484) of the European commission, receives a scholarship from the EACEA.
The authors declare that the research was conducted in the absence of any commercial or financial relationships that could be construed as a potential conflict of interest.
All claims expressed in this article are solely those of the authors and do not necessarily represent those of their affiliated organizations, or those of the publisher, the editors and the reviewers. Any product that may be evaluated in this article, or claim that may be made by its manufacturer, is not guaranteed or endorsed by the publisher.
Aaby, P., Roth, A., Ravn, H., Napirna, B. M., Rodrigues, A., Lisse, I. M., et al. (2011). Randomized trial of BCG vaccination at birth to low-birth-weight children: Beneficial nonspecific effects in the neonatal period? J. Infect. Dis. 204, 245–252.
Acuña Hidalgo, B., and Armitage, S. A. O. (2022). Host Resistance to Bacterial Infection Varies Over Time, but Is Not Affected by a Previous Exposure to the Same Pathogen. Front. Physiol. 13:860875. doi: 10.3389/fphys.2022.860875
Agaisse, H., Petersen, U., Boutros, M., Mathey-Prevot, B., and Perrimon, N. (2003). Signaling role of hemocytes in Drosophila JAK/STAT-dependent response to septic injury. Dev. Cell. 5, 441–450. doi: 10.1016/s1534-5807(03)00244-2
Amcheslavsky, A., and Ip, Y. T. (2012). Be a Good Neighbor: Organ-to-Organ Communication during the Innate Immune Response. Cell Host Microbe 11, 323–324. doi: 10.1016/j.chom.2012.04.003
Apidianakis, Y., and Rahme, L. G. (2009). Drosophila melanogaster as a model host for studying Pseudomonas aeruginosa infection. Nat. Protoc. 4, 1285–1294.
Apidianakis, Y., Mindrinos, M., Xiao, W., Lau, G., Baldini, R., Davis, R., et al. (2005). Profiling early infection responses: Pseudomonas aeruginosa eludes host defenses by suppressing antimicrobial peptide gene expression. Proc. Natl. Acad. Sci. U. S. A. 102, 2573–2578. doi: 10.1073/pnas.0409588102
Aromolaran, O., Beder, T., Adedeji, E., Ajamma, Y., Oyelade, J., Adebiyi, E., et al. (2021). Predicting host dependency factors of pathogens in Drosophila melanogaster using machine learning. Comput. Struct. Biotechnol. J. 19, 4581–4592. doi: 10.1016/j.csbj.2021.08.010
Arts, R. J. W., Moorlag, S. J. C. F. M., Novakovic, B., Li, Y., Wang, S. Y., Oosting, M., et al. (2018). BCG Vaccination Protects against Experimental Viral Infection in Humans through the Induction of Cytokines Associated with Trained Immunity. Cell Host Microbe 23, 89–100.e5.
Arts, R., Carvalho, A., La Rocca, C., Palma, C., Rodrigues, F., Silvestre, R., et al. (2016). Immunometabolic Pathways in BCG-Induced Trained Immunity. Cell Rep. 17, 2562–2571.
Ashida, M., and Brey, P. (1995). Role of the integument in insect defense: Pro-phenol oxidase cascade in the cuticular matrix. Proc. Natl. Acad. Sci. U. S. A. 92, 10698–10702. doi: 10.1073/pnas.92.23.10698
Aymeric, J. L., Givaudan, A., and Duvic, B. (2010). IMD pathway is involved in the interaction of Drosophila melanogaster with the entomopathogenic bacteria, Xenorhabdus nematophila and Photorhabdus luminescens. Mol. Immunol. 47, 2342–2348. doi: 10.1016/j.molimm.2010.05.012
Badinloo, M., Nguyen, E., Suh, W., Alzahrani, F., Castellanos, J., Klichko, V. I., et al. (2018). Overexpression of antimicrobial peptides contributes to aging through cytotoxic effects in Drosophila tissues. Arch. Insect. Biochem. Physiol. 98:e21464. doi: 10.1002/arch.21464
Basset, A., Khush, R. S., Braun, A., Gardan, L., Boccard, F., Hoffmann, J. A., et al. (2000). The phytopathogenic bacteria Erwinia carotovora infects Drosophila and activates an immune response. Proc. Natl. Acad. Sci. U. S. A. 97, 3376–3381. doi: 10.1073/pnas.97.7.3376
Belmonte, R. L., Corbally, M. K., Duneau, D. F., and Regan, J. C. (2020). Sexual Dimorphisms in Innate Immunity and Responses to Infection in Drosophila melanogaster. Front. Immunol. 10:3075. doi: 10.3389/fimmu.2019.03075
Bier, E. (2005). Drosophila, the golden bug, emerges as a tool for human genetics. Nat. Rev. Genet. 6, 9–23. doi: 10.1038/nrg1503
Biering-Sørensen, S., Aaby, P., Lund, N., Monteiro, I., Jensen, K. J., Eriksen, H. B., et al. (2017). Early BCG-Denmark and Neonatal Mortality Among Infants Weighing. Clin. Infect. Dis. 65, 1183–1190. doi: 10.1093/cid/cix525
Bindu, S., Dandapat, S., Manikandan, R., Dinesh, M., Subbaiyan, A., Mani, P., et al. (2022). Prophylactic and therapeutic insights into trained immunity: A renewed concept of innate immune memory. Hum. Vaccine Immunother. 18:2040238. doi: 10.1080/21645515.2022.2040238
Boman, H., Nilsson, I., and Rasmuson, B. (1972). Inducible Antibacterial Defence System in Drosophila. Nature 237, 232–235.
Bonnet, J., Lindeboom, R. G. H., Pokrovsky, D., Stricker, G., Çelik, M. H., Rupp, R. A. W., et al. (2019). Quantification of Proteins and Histone Marks in Drosophila Embryos Reveals Stoichiometric Relationships Impacting Chromatin Regulation. Dev. Cell. 51, 632–644.e6. doi: 10.1016/j.devcel.2019.09.011
Boraschi, D., and Italiani, P. (2018). Innate immune memory: Time for adopting a correct terminology. Front. Immunol. 9:799. doi: 10.3389/fimmu.2018.00799
Bosco-Drayon, V., Poidevin, M., Boneca, I. G., Narbonne-Reveau, K., Royet, J., and Charroux, B. (2012). Peptidoglycan sensing by the receptor PGRP-LE in the Drosophila gut induces immune responses to infectious bacteria and tolerance to microbiota. Cell Host Microbe 12, 153–165. doi: 10.1016/j.chom.2012.06.002
Boutros, M., Agaisse, H., and Perrimon, N. (2002). Sequential activation of signaling pathways during innate immune responses in Drosophila. Dev. Cell. 3, 711–722. doi: 10.1016/s1534-5807(02)00325-8
Bozler, J., Kacsoh, B., and Bosco, G. (2020). Maternal Priming of Offspring Immune System in Drosophila. G3 10, 165–175. doi: 10.1534/g3.119.400852
Buchon, N., Broderick, N., and Lemaitre, B. (2013). Gut homeostasis in a microbial world: Insights from Drosophila melanogaster. Nat. Rev. Microbiol. 11, 615–626. doi: 10.1038/nrmicro3074
Buchon, N., Silverman, N., and Cherry, S. (2014). Immunity in Drosophila melanogaster — from microbial recognition to whole- organism physiology. Nat. Rev. Immunol. 14:796. doi: 10.1038/nri3763
Buffen, K., Oosting, M., Quintin, J., Ng, A., Kleinnijenhuis, J., Kumar, V., et al. (2014). Autophagy Controls BCG-Induced Trained Immunity and the Response to Intravesical BCG Therapy for Bladder Cancer. PLoS Pathog. 10:e1004485. doi: 10.1371/journal.ppat.1004485
Cabrera, K., Hoard, D. S., Martinez, D. I., and Wunderlich, Z. (2022). Drosophila immune priming to Enterococcus faecalis relies on immune tolerance rather than resistance. bioRxiv [Preprint]. doi: 10.1101/2022.07.20.500468v1
Capo, F., Wilson, A., and Di Cara, F. (2019). The Intestine of Drosophila melanogaster: An Emerging Versatile Model System to Study Intestinal Epithelial Homeostasis and Host-Microbial Interactions in Humans. Microorganisms 7:336. doi: 10.3390/microorganisms7090336
Chakrabarti, S., and Visweswariah, S. S. (2020). Intramacrophage ROS Primes the Innate Immune System via JAK/STAT and Toll Activation. Cell Rep. 33:108368. doi: 10.1016/j.celrep.2020.108368
Chambers, M. C., Jacobson, E., Khalil, S., and Lazzaro, B. P. (2019). Consequences of chronic bacterial infection in Drosophila melanogaster. PLoS One 14:e0224440. doi: 10.1371/journal.pone.0224440
Charroux, B., and Royet, J. (2009). Elimination of plasmatocytes by targeted apoptosis reveals their role in multiple aspects of the Drosophila immune response. Proc. Natl. Acad. Sci. U. S. A. 106, 9797–9802. doi: 10.1073/pnas.0903971106
Charroux, B., Capo, F., Kurz, C. L., Peslier, S., Chaduli, D., Viallat-lieutaud, A., et al. (2018). Cytosolic and Secreted Peptidoglycan-Degrading Enzymes in Drosophila Respectively Control Local and Systemic Immune Responses to Microbiota. Cell Host Microbe 23, 215–228.e4. doi: 10.1016/j.chom.2017.12.007
Cheng, S., Quintin, J., Cramer, R., Shepardson, K., Saeed, S., Kumar, V., et al. (2014). mTOR- and HIF-1α-mediated aerobic glycolysis as metabolic basis for trained immunity. Science 45:1250684. doi: 10.1126/science.1250684
Chow, J., and Kagan, J. C. (2018). The Fly Way of Antiviral Resistance and Disease Tolerance. Adv. Immunol. 140, 59–93. doi: 10.1016/bs.ai.2018.08.002
Christensen, B. M., Li, J., Chen, C. C., and Nappi, A. J. (2005). Melanization immune responses in mosquito vectors. Trends Parasitol. 21, 192–199.
Christofi, T., and Apidianakis, Y. (2013). Drosophila immune priming against Pseudomonas aeruginosa is short-lasting and depends on cellular and humoral immunity. F1000Research 2:76. doi: 10.12688/f1000research.2-76.v1
Conrath, U., Beckers, G., Langenbach, C., and Jaskiewicz, M. (2015). Priming for enhanced defense. Annu. Rev. Phytopathol. 53, 97–119.
Cooper, D., and Eleftherianos, I. (2017). Memory and specificity in the insect immune system: Current perspectives and future challenges. Front. Immunol. 8:539. doi: 10.3389/fimmu.2017.00539
Cooper, J. F., and Van Raamsdonk, J. M. (2018). Modeling Parkinson’s Disease in C. elegans. J. Parkinsons Dis. 8, 17–32.
Corby-Harris, V., and Promislow, D. E. L. (2008). Host ecology shapes geographical variation for resistance to bacterial infection in Drosophila melanogaster. J. Anim. Ecol. 77, 768–776. doi: 10.1111/j.1365-2656.2008.01399.x
Corby-Harris, V., Habel, K. E., Ali, F. G., and Promislow, D. E. L. (2007). Alternative measures of response to Pseudomonas aeruginosa infection in Drosophila melanogaster. J. Evol. Biol. 20, 526–533.
Covián, C., Fernández-Fierro, A., Retamal-Díaz, A., Díaz, F. E., Vasquez, A. E., Lay, M. K., et al. (2019). BCG-Induced Cross-Protection and Development of Trained Immunity: Implication for Vaccine Design. Front. Immunol. 10:2806. doi: 10.3389/fimmu.2019.02806
Das, J., Verma, D., Gustafsson, M., and Lerm, M. (2019). Identification of DNA methylation patterns predisposing for an efficient response to BCG vaccination in healthy BCG-naïve subjects. Epigenetics 14, 589–601. doi: 10.1080/15592294.2019.1603963
De Gregorio, E., Spellman, P., Rubin, G., and Lemaitre, B. (2001). Genome-wide analysis of the Drosophila immune response by using oligonucleotide microarrays. Proc. Natl. Acad. Sci. U. S. A. 98, 12590–12595.
Dhinaut, J., Chogne, M., and Moret, Y. (2018). Immune priming specificity within and across generations reveals the range of pathogens affecting evolution of immunity in an insect. J. Anim. Ecol. 87, 448–463. doi: 10.1111/1365-2656.12661
Di Luzio, N. R., and Williams, D. L. (1978). Protective effect of glucan against systemic Staphylococcus aureus septicemia in normal and leukemic mice. Infect. Immun. 20:804. doi: 10.1128/iai.20.3.804-810.1978
Dimopoulos, G., Christophides, G. K., Meister, S., Schultz, J., White, K. P., Barillas-Mury, C., et al. (2002). Genome expression analysis of Anopheles gambiae: Responses to injury, bacterial challenge, and malaria infection. Proc. Natl. Acad. Sci. U. S. A. 99, 8814–8819. doi: 10.1073/pnas.092274999
Divangahi, M., Aaby, P., Khader, S. A., Barreiro, L. B., Bekkering, S., Chavakis, T., et al. (2020). Trained immunity, tolerance, priming and differentiation: Distinct immunological processes. Nat. Immunol. 22, 2–6.
Dominguez-Andres, J., and Netea, M. G. (2019). Long-term reprogramming of the innate immune system. J. Leukoc. Biol. 105, 329–338.
Donohoe, D., and Bultman, S. (2012). Metaboloepigenetics: Interrelationships between energy metabolism and epigenetic control of gene expression. J. Cell Physiol. 227, 3169–3177. doi: 10.1002/jcp.24054
Dostálová, A., Rommelaere, S., Poidevin, M., and Lemaitre, B. (2017). Thioester-containing proteins regulate the Toll pathway and play a role in Drosophila defence against microbial pathogens and parasitoid wasps. BMC Biol. 15:79. doi: 10.1186/s12915-017-0408-0
Dudzic, J. P., Kondo, S., Ueda, R., Bergman, C. M., and Lemaitre, B. (2015). Drosophila innate immunity: Regional and functional specialization of prophenoloxidases. BMC Biol. 13:81. doi: 10.1186/s12915-015-0193-6
Duneau, D. F., Kondolf, H. C., Im, J. H., Ortiz, G. A., Chow, C., Fox, M. A., et al. (2017). The Toll pathway underlies host sexual dimorphism in resistance to both Gram-negative and Gram-positive bacteria in mated Drosophila. BMC Biol. 15:124. doi: 10.1186/s12915-017-0466-3
Duneau, D., Ferdy, J. B., Revah, J., Kondolf, H., Ortiz, G. A., Lazzaro, B. P., et al. (2017). Stochastic variation in the initial phase of bacterial infection predicts the probability of survival in D. melanogaster. Elife 6:e28298. doi: 10.7554/eLife.28298
Eleftherianos, I., Marokhazi, J., Millichap, P. J., Hodgkinson, A. J., Sriboonlert, A., ffrench-Constant, R. H., et al. (2006). Prior infection of Manduca sexta with non-pathogenic Escherichia coli elicits immunity to pathogenic Photorhabdus luminescens: Roles of immune-related proteins shown by RNA interference. Insect. Biochem. Mol. Biol. 36, 517–525. doi: 10.1016/j.ibmb.2006.04.001
Fanucchi, S., Fok, E., Dalla, E., Shibayama, Y., Börner, K., Chang, E., et al. (2019). Immune genes are primed for robust transcription by proximal long noncoding RNAs located in nuclear compartments. Nat. Genet. 51, 138–150.
Foley, E., and O’Farrell, P. H. (2003). Nitric oxide contributes to induction of innate immune responses to gram-negative bacteria in Drosophila. Genes Dev. 17, 115–125. doi: 10.1101/gad.1018503
Freitak, D., Schmidtberg, H., Dickel, F., Lochnit, G., Vogel, H., and Vilcinskas, A. (2014). The maternal transfer of bacteria can mediate trans-generational immune priming in insects. Virulence 5, 547–554.
Freitak, D., Wheat, C. W., Heckel, D. G., and Vogel, H. (2007). Immune system responses and fitness costs associated with consumption of bacteria in larvae of Trichoplusia ni. BMC Biol. 5:56. doi: 10.1186/1741-7007-5-56
Galenza, A., and Foley, E. (2019). Immunometabolism: Insights from the Drosophila model. Dev. Comp. Immunol. 94, 22–34.
Garly, M. L., Martins, C. L., Balé, C., Baldé, M. A., Hedegaard, K. L., Gustafson, P., et al. (2003). BCG scar and positive tuberculin reaction associated with reduced child mortality in West Africa. A non-specific beneficial effect of BCG? Vaccine 21, 2782–2790. doi: 10.1016/s0264-410x(03)00181-6
Gendrin, M., Zaidman-Rémy, A., Broderick, N. A., Paredes, J., Poidevin, M., Roussel, A., et al. (2013). Functional Analysis of PGRP-LA in Drosophila Immunity. PLoS One 8:e69742. doi: 10.1371/journal.pone.0069742
Ghisletti, S., Barozzi, I., Mietton, F., Polletti, S., De Santa, F., Venturini, E., et al. (2010). Identification and characterization of enhancers controlling the inflammatory gene expression program in macrophages. Immunity 32, 317–328.
Gourbal, B., Pinaud, S., Beckers, G., Van Der Meer, J., Conrath, U., and Netea, M. (2018). Innate immune memory: An evolutionary perspective. Immunol. Rev. 283, 21–40.
Gupta, V., and Vale, P. F. (2017). Nonlinear disease tolerance curves reveal distinct components of host responses to viral infection. R. Soc. Open Sci. 4:170342. doi: 10.1098/rsos.170342
Ha, E., Lee, K., Seo, Y., Kim, S., Lim, J., Oh, B., et al. (2009b). Coordination of multiple dual oxidase-regulatory pathways in responses to commensal and infectious microbes in Drosophila gut. Nat. Immunol. 10, 949–957. doi: 10.1038/ni.1765
Ha, E., Lee, K., Park, S., Kim, S., Nam, H., Lee, H., et al. (2009a). Regulation of DUOX by the Galphaq-phospholipase Cbeta-Ca2+ pathway in Drosophila gut immunity. Dev. Cell. 16, 386–397. doi: 10.1016/j.devcel.2008.12.015
Hajishengallis, G., Li, X., Mitroulis, I., and Chavakis, T. (2019). Trained Innate Immunity and Its Implications for Mucosal Immunity and Inflammation. Adv. Exp. Med. Biol. 1197, 11–26.
Hanson, M. A., and Lemaitre, B. (2020). New insights on Drosophila antimicrobial peptide function in host defense and beyond. Curr. Opin. Immunol. 62, 22–30. doi: 10.1016/j.coi.2019.11.008
Hanson, M. A., Dostálová, A., Ceroni, C., Poidevin, M., Kondo, S., and Lemaitre, B. (2019). Synergy and remarkable specificity of antimicrobial peptides in vivo using a systematic knockout approach. Elife 8:e44341.
Hanson, M. A., Kondo, S., and Lemaitre, B. (2022). Drosophila immunity: The Drosocin gene encodes two host defence peptides with pathogen-specific roles. bioRxiv [Preprint]. doi: 10.1101/2022.04.21.489012v1
Hartenstein, V. (2006). Blood Cells and Blood Cell Development in the Animal Kingdom. Annu. Rev. Cell. Dev. Biol. 22, 677–712.
Herren, J. K., Paredes, J. C., Schüpfer, F., and Lemaitre, B. (2013). Vertical transmission of a Drosophila endosymbiont via cooption of the yolk transport and internalization machinery. Mbio 4, 1–8. doi: 10.1128/mBio.00532-12
Hirano, M., Das, S., Guo, P., and Cooper, M. D. (2011). The evolution of adaptive immunity in vertebrates. Adv. Immunol. 109, 125–157.
Honti, V., Csordás, G., Kurucz, É., Márkus, R., and Andó, I. (2014). The cell-mediated immunity of Drosophila melanogaster: Hemocyte lineages, immune compartments, microanatomy and regulation. Dev. Comp. Immunol. 42, 47–56. doi: 10.1016/j.dci.2013.06.005
Howick, V. M., and Lazzaro, B. P. (2017). The genetic architecture of defence as resistance to and tolerance of bacterial infection in Drosophila melanogaster. Mol. Ecol. 26, 1533–1546. doi: 10.1111/mec.14017
Hultmark, D., and Andó, I. (2022). Hematopoietic plasticity mapped in Drosophila and other insects. Elife 11:e78906. doi: 10.7554/eLife.78906
Iatsenko, I., Boquete, J., and Lemaitre, B. (2018). Microbiota-Derived Lactate Activates Production of Reactive Oxygen Species by the Intestinal NADPH Oxidase Nox and Shortens Drosophila Lifespan. Immunity 49, 929–942.e5. doi: 10.1016/j.immuni.2018.09.017
Imler, J. L., and Bulet, P. (2005). Antimicrobial peptides in Drosophila: Structures, activities and gene regulation. Chem. Immunol. Allergy 86, 1–21.
Jacqueline, C., Parvy, J. P., Rollin, M., Lou Faugère, D., Renaud, F., Missé, D., et al. (2020). The role of innate immunity in the protection conferred by a bacterial infection against cancer: Study of an invertebrate model. Sci. Rep. 10, 1–8. doi: 10.1038/s41598-020-66813-0
Javdat, M., and Tamara, A. (2020). RNA Interference: Antiviral Defense Mechanism and Immune Memory. Adv. Appl. Physiol. 5, 24–29. doi: 10.1146/annurev-ento-033020-090410
Kaufmann, E., Sanz, J., Dunn, J. L., Khan, N., Mendonça, L. E., Pacis, A., et al. (2018). BCG Educates Hematopoietic Stem Cells to Generate Protective Innate Immunity against Tuberculosis. Cell 172, 176–190.e19. doi: 10.1016/j.cell.2017.12.031
Knorr, E., Schmidtberg, H., Arslan, D., Bingsohn, L., and Vilcinskas, A. (2015). Translocation of bacteria from the gut to the eggs triggers maternal transgenerational immune priming in Tribolium castaneum. Biol. Lett. 11:20150885. doi: 10.1098/rsbl.2015.0885
Kounatidis, I., and Ligoxygakis, P. (2012). Drosophila as a model system to unravel the layers of innate immunity to infection. Open Biol. 2:120075. doi: 10.1098/rsob.120075
Krahenbuhl, J. L., Sharma, S. D., Ferraresi, R. W., and Remington, J. S. (1981). Effects of muramyl dipeptide treatment on resistance to infection with Toxoplasma gondii in mice. Infect. Immun. 31:716. doi: 10.1128/iai.31.2.716-722.1981
Kutzer, M. A. M., and Armitage, S. A. O. (2016). The effect of diet and time after bacterial infection on fecundity, resistance, and tolerance in Drosophila melanogaster. Ecol. Evol. 6, 4229–4242. doi: 10.1002/ece3.2185
Kutzer, M. A. M., Kurtz, J., and Armitage, S. A. O. (2019). A multi-faceted approach testing the effects of previous bacterial exposure on resistance and tolerance. J. Anim. Ecol. 88, 566–578. doi: 10.1111/1365-2656.12953
Lagueux, M., Perrodou, E., Levashina, E., Capovilla, M., and Hoffmann, J. (2000). Constitutive expression of a complement-like protein in toll and JAK gain-of-function mutants of Drosophila. Proc. Natl. Acad. Sci. U. S. A. 97, 11427–11432. doi: 10.1073/pnas.97.21.11427
Lajqi, T., Braun, M., Kranig, S. A., Frommhold, D., Pöschl, J., and Hudalla, H. (2021). LPS Induces Opposing Memory-like Inflammatory Responses in Mouse Bone Marrow Neutrophils. Int. J. Mol. Sci. 22:9803. doi: 10.3390/ijms22189803
Lanot, R., Zachary, D., Holder, F., and Meister, M. (2001). Postembryonic hematopoiesis in Drosophila. Dev. Biol. 230, 243–257.
Lanz-Mendoza, H., and Contreras-Garduño, J. (2022). Innate immune memory in invertebrates: Concept and potential mechanisms. Dev. Comp. Immunol. 127:104285.
Lavin, Y., Winter, D., Blecher-Gonen, R., David, E., Keren-Shaul, H., Merad, M., et al. (2014). Tissue-Resident Macrophage Enhancer Landscapes Are Shaped by the Local Microenvironment. Cell 159, 1312–1326. doi: 10.1016/j.cell.2014.11.018
Lee, K. A., Kim, S. H., Kim, E. K., Ha, E. M., You, H., Kim, B., et al. (2013). Bacterial-Derived Uracil as a Modulator of Mucosal Immunity and Gut-Microbe Homeostasis in Drosophila. Cell 153, 797–811. doi: 10.1016/j.cell.2013.04.009
Lee, Y. J., Jang, H. J., Chung, I. Y., and Cho, Y. H. (2018). Drosophila melanogaster as a polymicrobial infection model for Pseudomonas aeruginosa and Staphylococcus aureus. J. Microbiol. 56, 534–541.
Lefèvre, T., Williams, A. J., and De Roode, J. C. (2011). Genetic variation in resistance, but not tolerance, to a protozoan parasite in the monarch butterfly. Proc. R. Soc. B Biol. Sci. 278:751.
Leitão, A. B., Arunkumar, R., Day, J. P., Geldman, E. M., Morin-Poulard, I., Crozatier, M., et al. (2020). Constitutive activation of cellular immunity underlies the evolution of resistance to infection in Drosophila. Elife 24:9. doi: 10.7554/eLife.59095
Lemaitre, B., and Hoffmann, J. (2007). The host defense of Drosophila melanogaster. Annu. Rev. Immunol. 25, 697–743.
Linder, J. E., and Promislow, D. E. L. (2009). Cross-generational fitness effects of infection in Drosophila melanogaster. Fly 3, 143–150. doi: 10.4161/fly.8051
Liu, Q., and Jin, L. H. (2017). Organ-to-Organ Communication: A Drosophila Gastrointestinal Tract Perspective. Front. Cell. Dev. Biol. 5:29. doi: 10.3389/fcell.2017.00029
Liu, X., Hodgson, J. J., and Buchon, N. (2017). Drosophila as a model for homeostatic, antibacterial, and antiviral mechanisms in the gut. PLoS Pathog. 13:e1006277. doi: 10.1371/journal.ppat.1006277
Longdon, B., Cao, C., Martinez, J., and Jiggins, F. M. (2013). Previous Exposure to an RNA Virus Does Not Protect against Subsequent Infection in Drosophila melanogaster. PLoS One 8:e73833. doi: 10.1371/journal.pone.0073833
Madhwal, S., Shin, M., Kapoor, A., Goyal, M., Joshi, M. K., Rehman, P. M. U., et al. (2020). Metabolic control of cellular immune-competency by odors in Drosophila. Elife 9, 1–93. doi: 10.7554/eLife.60376
Marakalala, M. J., Williams, D. L., Hoving, J. C., Engstad, R., Netea, M. G., and Brown, G. D. (2013). Dectin-1 plays a redundant role in the immunomodulatory activities of β-glucan-rich ligands in vivo. Microbes Infect. 15:511. doi: 10.1016/j.micinf.2013.03.002
Martins, R., Carlos, A. R., Braza, F., Thompson, J. A., Bastos-Amador, P., Ramos, S., et al. (2019). Disease Tolerance as an Inherent Component of Immunity. Annu. Rev. Immunol. 37, 405–437.
Medzhitov, R., and Janeway, C. (2000). Innate immune recognition: Mechanisms and pathways. Immunol. Rev. 173, 89–97.
Medzhitov, R., Schneider, D. S., and Soares, M. P. (2012). Disease tolerance as a defense strategy. Science 335, 936–941.
Melillo, D., Marino, R., Italiani, P., and Boraschi, D. (2018). Innate Immune Memory in Invertebrate Metazoans: A Critical Appraisal. Front. Immunol. 9:1915. doi: 10.3389/fimmu.2018.01915
Meng, Z., and Lu, M. (2017). RNA interference-induced innate immunity, off-target effect, or immune adjuvant? Front. Immunol. 8:331. doi: 10.3389/fimmu.2017.00331
Merkling, S. H., and Van Rij, R. P. (2015). Analysis of resistance and tolerance to virus infection in Drosophila. Nat. Protoc. 10, 1084–1097.
Michael Harnish, J., Link, N., and Yamamoto, S. (2021). Drosophila as a Model for Infectious Diseases. Int. J. Mol. Sci. 22, 1–42.
Mikonranta, L., Mappes, J., Kaukoniitty, M., and Freitak, D. (2014). Insect immunity: Oral exposure to a bacterial pathogen elicits free radical response and protects from a recurring infection. Front. Zool. 11:23. doi: 10.1186/1742-9994-11-23
Miyashita, A., Takahashi, S., Ishii, K., Sekimizu, K., and Kaito, C. (2015). Primed Immune Responses Triggered by Ingested Bacteria Lead to Systemic Infection Tolerance in Silkworms. PLoS One 10:e0130486. doi: 10.1371/journal.pone.0130486
Mondotte, J. A., Gausson, V., Frangeul, L., Blanc, H., Lambrechts, L., and Saleh, M.-C. (2018). Immune priming and clearance of orally acquired RNA viruses in Drosophila. Nat. Microbiol. 3, 1394–1403. doi: 10.1038/s41564-018-0265-9
Mondotte, J. A., Gausson, V., Frangeul, L., Suzuki, Y., Vazeille, M., Mongelli, V., et al. (2020). Evidence For Long-Lasting Transgenerational Antiviral Immunity in Insects. Cell Rep. 33:108506. doi: 10.1016/j.celrep.2020.108506
Moret, Y., and Siva-Jothy, M. T. (2003). Adaptive innate immunity? Responsive-mode prophylaxis in the mealworm beetle, Tenebrio molitor. Proc. R. Soc. B Biol. Sci. 270:2475. doi: 10.1098/rspb.2003.2511
Muñoz, N., Van Maele, L., Marqués, J. M., Rial, A., Sirard, J. C., and Chabalgoity, J. A. (2010). Mucosal administration of flagellin protects mice from Streptococcus pneumoniae lung infection. Infect Immun. 78, 4226–4233. doi: 10.1128/IAI.00224-10
Naik, S., Larsen, S. B., Gomez, N. C., Alaverdyan, K., Sendoel, A., Yuan, S., et al. (2017). Inflammatory memory sensitizes skin epithelial stem cells to tissue damage. Nature 550, 475–480.
Naik, S., Larsen, S., Cowley, C., and Fuchs, E. (2018). Two to Tango: Dialog between Immunity and Stem Cells in Health and Disease. Cell 175, 908–920. doi: 10.1016/j.cell.2018.08.071
Nankabirwa, V., Tumwine, J. K., Mugaba, P. M., Tylleskär, T., Sommerfelt, H., Van De Perre, P., et al. (2015). Child survival and BCG vaccination: A community based prospective cohort study in Uganda. BMC Public Health 15:175. doi: 10.1186/s12889-015-1497-8
Natoli, G., and Ostuni, R. (2019). Adaptation and memory in immune responses. Nat. Immunol. 20, 783–792.
Netea, M. G., Domínguez-Andrés, J., Barreiro, L. B., Chavakis, T., Divangahi, M., Fuchs, E., et al. (2020). Defining trained immunity and its role in health and disease. Nat. Rev. Immunol. 20, 375–388.
Netea, M. G., Joosten, L. A. B., Latz, E., Mills, K. H. G., Natoli, G., Stunnenberg, H. G., et al. (2016). Trained immunity: A program of innate immune memory in health and disease. Science 352:427.
Netea, M. G., Quintin, J., and Van Der Meer, J. W. M. (2011). Trained immunity: A memory for innate host defense. Cell Host Microbe 9, 355–361.
Norouzitallab, P., Baruah, K., Biswas, P., Vanrompay, D., and Bossier, P. (2016). Probing the phenomenon of trained immunity in invertebrates during a transgenerational study, using brine shrimp Artemia as a model system. Sci. Rep. 6:21166. doi: 10.1038/srep21166
Onfelt Tingvall, T., Roos, E., and Engström, Y. (2001). The imd gene is required for local Cecropin expression in Drosophila barrier epithelia. EMBO Rep. 2, 239–243. doi: 10.1093/embo-reports/kve048
Paredes, J. C., Welchman, D. P., Poidevin, M., and Lemaitre, B. (2011). Negative regulation by amidase PGRPs shapes the Drosophila antibacterial response and protects the fly from innocuous infection. Immunity 35, 770–779. doi: 10.1016/j.immuni.2011.09.018
Patrnogic, J., Castillo, J. C., Shokal, U., Yadav, S., Kenney, E., Heryanto, C., et al. (2018). Pre-exposure to non-pathogenic bacteria does not protect Drosophila against the entomopathogenic bacterium Photorhabdus. PLoS One 13:e0205256. doi: 10.1371/journal.pone.0205256
Penkov, S., Mitroulis, I., Hajishengallis, G., and Chavakis, T. (2019). Immunometabolic Crosstalk: An Ancestral Principle of Trained Immunity? Trends Immunol. 40, 1–11. doi: 10.1016/j.it.2018.11.002
Pham, L. N., Dionne, M. S., Shirasu-Hiza, M., and Schneider, D. S. (2007). A Specific Primed Immune Response in Drosophila Is Dependent on Phagocytes. PLoS Pathog. 3:e26. doi: 10.1371/journal.ppat.0030026
Prakash, A., and Khan, I. (2022). Why do insects evolve immune priming? A search for crossroads. Dev. Comp. Immunol. 126:104246. doi: 10.1016/j.dci.2021.104246
Råberg, L., Graham, A. L., and Read, A. F. (2008). Decomposing health: Tolerance and resistance to parasites in animals. Philos. Trans. R. Soc. B Biol. Sci. 364, 37–49. doi: 10.1098/rstb.2008.0184
Råberg, L., Sim, D., and Read, A. F. (2007). Disentangling Genetic Variation for Resistance and Tolerance to Infectious Diseases in Animals. Science 318, 812–814. doi: 10.1126/science.1148526
Redelman-Sidi, G., Glickman, M. S., and Bochner, B. H. (2014). The mechanism of action of BCG therapy for bladder cancer–a current perspective. Nat. Rev. Urol. 11, 153–162.
Reimer-Michalski, E. M., and Conrath, U. (2016). Innate immune memory in plants. Semin. Immunol. 28, 319–327.
Ribes, S., Meister, T., Ott, M., Redlich, S., Janova, H., Hanisch, U. K., et al. (2014). Intraperitoneal prophylaxis with CpG oligodeoxynucleotides protects neutropenic mice against intracerebral Escherichia coli K1 infection. J. Neuroinflammation 11, 1–11. doi: 10.1186/1742-2094-11-14
Rieckmann, A., Villumsen, M., Sørup, S., Haugaard, L. K., Ravn, H., Roth, A., et al. (2017). Vaccinations against smallpox and tuberculosis are associated with better long-term survival: A Danish case-cohort study 1971–2010. Int. J. Epidemiol. 46:695. doi: 10.1093/ije/dyw120
Rodrigues, J., Brayner, F. A., Alves, L. C., Dixit, R., and Barillas-Mury, C. (2010). Hemocyte Differentiation Mediates Innate Immune Memory in Anopheles gambiae Mosquitoes. Science 329:1353. doi: 10.1126/science.1190689
Ryu, J. H., Kim, S. H., Lee, H. Y., Bai, J. Y., Nam, Y. D., Bae, J. W., et al. (2008). Innate Immune Homeostasis by the Homeobox Gene Caudal and Commensal-Gut Mutualism in Drosophila. Science 319, 777–782. doi: 10.1126/science.1149357
Schwenke, R. A., and Lazzaro, B. P. (2017). Juvenile Hormone Suppresses Resistance to Infection in Mated Female Drosophila melanogaster. Curr. Biol. 27, 596–601. doi: 10.1016/j.cub.2017.01.004
Schwenke, R. A., Lazzaro, B. P., and Wolfner, M. F. (2016). Reproduction–Immunity Trade-Offs in Insects. Annu. Rev. Entomol. 61, 239–256.
Seeley, J. J., and Ghosh, S. (2017). Molecular mechanisms of innate memory and tolerance to LPS. J. Leukoc. Biol. 101, 107–119.
Segrist, E., Dittmar, M., Gold, B., and Cherry, S. (2021). Orally acquired cyclic dinucleotides drive dSTING-dependent antiviral immunity in enterocytes. Cell. Rep. 37:110150. doi: 10.1016/j.celrep.2021.110150
Sheehan, G., Farrell, G., and Kavanagh, K. (2020). Immune priming: The secret weapon of the insect world. Virulence 11, 238–246. doi: 10.1080/21505594.2020.1731137
Shen, R., Zheng, K., Zhou, Y., Chi, X., Pan, H., Wu, C., et al. (2022). A dRASSF-STRIPAK-Imd-JAK/STAT axis controls antiviral immune response in Drosophila. Cell. Rep. 40:111143. doi: 10.1016/j.celrep.2022.111143
Smale, S., Tarakhovsky, A., and Natoli, G. (2014). Chromatin contributions to the regulation of innate immunity. Annu. Rev. Immunol. 32, 489–511. doi: 10.1146/annurev-immunol-031210-101303
Söderhäll, K., and Cerenius, L. (1998). Role of the prophenoloxidase-activating system in invertebrate immunity. Curr. Opin. Immunol. 10, 23–28.
Soubry, A., Hoyo, C., Jirtle, R. L., and Murphy, S. K. (2014). A paternal environmental legacy: Evidence for epigenetic inheritance through the male germ line. Bioessays 36, 359–371.
Stewart, J. H., and Levine, E. A. (2011). Role of Bacillus Calmette-Guérin in the treatment of advanced melanoma. Expert Rev. Anticancer Ther. 11, 1671–1676.
Stramer, B., Wood, W., Galko, M. J., Redd, M. J., Jacinto, A., Parkhurst, S. M., et al. (2005). Live imaging of wound inflammation in Drosophila embryos reveals key roles for small GTPases during in vivo cell migration. J. Cell. Biol. 168, 567–573. doi: 10.1083/jcb.200405120
Tang, H. (2009). Regulation and function of the melanization reaction in Drosophila. Fly 3, 105–111. doi: 10.4161/fly.3.1.7747
Tassetto, M., Kunitomi, M., and Andino, R. (2017). Circulating Immune Cells Mediate a Systemic RNAi-Based Adaptive Antiviral Response in Drosophila. Cell 169, 314–325.e13. doi: 10.1016/j.cell.2017.03.033
Troha, K., and Buchon, N. (2019). Methods for the study of innate immunity in Drosophila melanogaster. Wiley Interdiscip. Rev. Dev. Biol. 8:e344.
Tzou, P., Ohresser, S., Ferrandon, D., Capovilla, M., Reichhart, J., Lemaitre, B., et al. (2000). Tissue-specific inducible expression of antimicrobial peptide genes in Drosophila surface epithelia. Immunity 13, 737–748.
Uribe-Querol, E., and Rosales, C. (2020). Phagocytosis: Our Current Understanding of a Universal Biological Process. Front. Immunol. 11:1066. doi: 10.3389/fimmu.2020.01066
Van’t Wout, J. W., Poell, R., and Vam Furth, R. (1992). The role of BCG/PPD-activated macrophages in resistance against systemic candidiasis in mice. Scand. J. Immunol. 36, 713–720. doi: 10.1111/j.1365-3083.1992.tb03132.x
Verma, D., Parasa, V., Raffetseder, J., Martis, M., Mehta, R., Netea, M., et al. (2017). Anti-mycobacterial activity correlates with altered DNA methylation pattern in immune cells from BCG-vaccinated subjects. Sci. Rep. 7:12305. doi: 10.1038/s41598-017-12110-2
Vilcinskas, A. (2021). Mechanisms of transgenerational immune priming in insects. Dev. Comp. Immunol. 124:104205.
Villumsen, M., Sørup, S., Jess, T., Ravn, H., Relander, T., Baker, J. L., et al. (2009). Risk of lymphoma and leukaemia after bacille Calmette-Guérin and smallpox vaccination: A Danish case-cohort study. Vaccine 27, 6950–6958. doi: 10.1016/j.vaccine.2009.08.103
Vlisidou, I., and Wood, W. (2015). Drosophila blood cells and their role in immune responses. FEBS J. 282, 1368–1382.
Wagner, C., Isermann, K., and Roeder, T. (2009). Infection induces a survival program and local remodeling in the airway epithelium of the fly. FASEB J. 23, 2045–2054. doi: 10.1096/fj.08-114223
Walk, J., de Bree, L. C. J., Graumans, W., Stoter, R., van Gemert, G. J., van de Vegte-Bolmer, M., et al. (2019). Outcomes of controlled human malaria infection after BCG vaccination. Nat. Commun. 10, 1–8.
Wang, L., Kounatidis, I., and Ligoxygakis, P. (2013). Drosophila as a model to study the role of blood cells in inflammation, innate immunity and cancer. Front. Cell. Infect. Microbiol. 3:113. doi: 10.3389/fcimb.2013.00113
Wen, Y., He, Z., Xu, T., Jiao, Y., Liu, X., Wang, Y. F., et al. (2019). Ingestion of killed bacteria activates antimicrobial peptide genes in Drosophila melanogaster and protects flies from septic infection. Dev. Comp. Immunol. 95, 10–18. doi: 10.1016/j.dci.2019.02.001
Witschkowski, J., Behrends, J., Frank, R., Eggers, L., von Borstel, L., Hertz, D., et al. (2020). BCG Provides Short-Term Protection from Experimental Cerebral Malaria in Mice. Vaccines 8, 1–15. doi: 10.3390/vaccines8040745
Wong, C. N. A., Ng, P., and Douglas, A. E. (2011). Low-diversity bacterial community in the gut of the fruitfly Drosophila melanogaster. Environ. Microbiol. 13, 1889–1900. doi: 10.1111/j.1462-2920.2011.02511.x
Wu, S. C., Liao, C. W., Pan, R. L., and Juang, J. L. (2012). Infection-induced intestinal oxidative stress triggers organ-to-organ immunological communication in Drosophila. Cell Host Microbe 11, 410–417. doi: 10.1016/j.chom.2012.03.004
Yang, H., and Hultmark, D. (2016). Tissue communication in a systemic immune response of Drosophila. Fly 10:115. doi: 10.1080/19336934.2016.1182269
Younes, S., Al-Sulaiti, A., Nasser, E. A. A., Najjar, H., and Kamareddine, L. (2020). Drosophila as a Model Organism in Host–Pathogen Interaction Studies. Front. Cell. Infect. Microbiol. 10:214. doi: 10.3389/fcimb.2020.00214
Keywords: Drosophila melanogaster, innate immune memory, trained immunity, tolerance, resistance, infection
Citation: Arch M, Vidal M, Koiffman R, Melkie ST and Cardona P-J (2022) Drosophila melanogaster as a model to study innate immune memory. Front. Microbiol. 13:991678. doi: 10.3389/fmicb.2022.991678
Received: 11 July 2022; Accepted: 03 October 2022;
Published: 20 October 2022.
Edited by:
Axel Cloeckaert, Institut National de Recherche pour l’Agriculture, l’Alimentation et l’Environnement (INRAE), FranceReviewed by:
Sofia J. Araújo, University of Barcelona, SpainCopyright © 2022 Arch, Vidal, Koiffman, Melkie and Cardona. This is an open-access article distributed under the terms of the Creative Commons Attribution License (CC BY). The use, distribution or reproduction in other forums is permitted, provided the original author(s) and the copyright owner(s) are credited and that the original publication in this journal is cited, in accordance with accepted academic practice. No use, distribution or reproduction is permitted which does not comply with these terms.
*Correspondence: Pere-Joan Cardona, cGouY2FyZG9uYUBnbWFpbC5jb20=
†These authors have contributed equally to this work
Disclaimer: All claims expressed in this article are solely those of the authors and do not necessarily represent those of their affiliated organizations, or those of the publisher, the editors and the reviewers. Any product that may be evaluated in this article or claim that may be made by its manufacturer is not guaranteed or endorsed by the publisher.
Research integrity at Frontiers
Learn more about the work of our research integrity team to safeguard the quality of each article we publish.