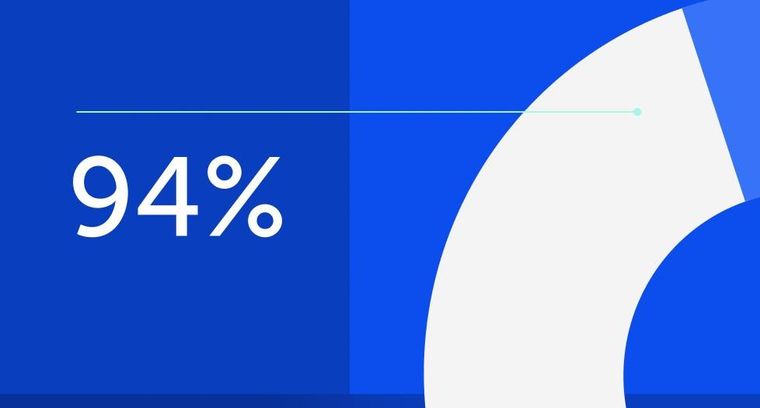
94% of researchers rate our articles as excellent or good
Learn more about the work of our research integrity team to safeguard the quality of each article we publish.
Find out more
REVIEW article
Front. Microbiol., 08 September 2022
Sec. Antimicrobials, Resistance and Chemotherapy
Volume 13 - 2022 | https://doi.org/10.3389/fmicb.2022.988728
This article is part of the Research TopicInsights in Antimicrobials, Resistance & Chemotherapy: 2021View all 14 articles
Antimicrobial resistance has become one of the greatest threats to human health, and new antibacterial treatments are urgently needed. As a tool to develop novel therapies, animal models are essential to bridge the gap between preclinical and clinical research. However, despite common usage of in vivo models that mimic clinical infection, translational challenges remain high. Standardization of in vivo models is deemed necessary to improve the robustness and reproducibility of preclinical studies and thus translational research. The European Innovative Medicines Initiative (IMI)-funded “Collaboration for prevention and treatment of MDR bacterial infections” (COMBINE) consortium, aims to develop a standardized, quality-controlled murine pneumonia model for preclinical efficacy testing of novel anti-infective candidates and to improve tools for the translation of preclinical data to the clinic. In this review of murine pneumonia model data published in the last 10 years, we present our findings of considerable variability in the protocols employed for testing the efficacy of antimicrobial compounds using this in vivo model. Based on specific inclusion criteria, fifty-three studies focusing on antimicrobial assessment against Pseudomonas aeruginosa, Klebsiella pneumoniae and Acinetobacter baumannii were reviewed in detail. The data revealed marked differences in the experimental design of the murine pneumonia models employed in the literature. Notably, several differences were observed in variables that are expected to impact the obtained results, such as the immune status of the animals, the age, infection route and sample processing, highlighting the necessity of a standardized model.
Antimicrobial resistance is recognized as one of the greatest threats to human health (World Health Organization [WHO], 2017; Morehead and Scarbrough, 2018; Murray et al., 2022). Thus, new antimicrobial therapies are urgently needed, although few are currently being developed (Hughes and Karlén, 2014; Bekeredjian-Ding, 2020; Theuretzbacher et al., 2020). Due to numerous challenges, including long research timelines and limited financial reward, most large pharmaceutical companies are no longer investing in research and development of new antibiotics. To ensure a sustainable pipeline of novel therapies, improving the efficiency and attractiveness of antibiotic drug development is crucial.
Animal models are essential to bridge the translational gap between preclinical and clinical research (Denayer et al., 2014; Friberg, 2021). They provide an infection environment and anatomical barriers that are difficult to reproduce in vitro, and they can be very useful in predicting potentially efficacious dosing regimens (Bulitta et al., 2019; Tängdén et al., 2020). Several different mammalian species have been used to model human pneumonia including piglets (Li Bassi et al., 2014), rodents (Mizgerd and Skerrett, 2008), non-human primates (Kraft et al., 2014), sheep (Malachowa et al., 2019), and rabbits (Nguyen et al., 2021). Although these models have proven helpful in studies of disease mechanisms and in antibiotic testing, murine models have been the preferred choice in investigational new drug applications for the treatment of bacterial pneumonia (Waack et al., 2020). Despite anatomical and physiological differences, the immune system of mice mimics that of humans and pathology of murine pneumonia resemble features of human pneumonia (Mizgerd and Skerrett, 2008; Metersky and Waterer, 2020). However, the observed pathology in mice strongly depends on pathogen-specific features of virulence, route of infection, infectious dose and other factors such as animal genetic background (Mizgerd and Skerrett, 2008; Bielen et al., 2017; Dietert et al., 2017). The features and measurements of experimental acute lung injury in animals depend on the experimental question to be addressed and it has been discussed elsewhere (Matute-Bello et al., 2011). The advantages of using murine models include ease of handling and cost effectiveness. Standardization of the mouse pneumonia model is deemed necessary to improve the robustness and reproducibility in preclinical studies and therefore improve translational research (Peers et al., 2012; Begley and Ioannidis, 2015). In order to improve the reproducibility of results and to facilitate comparisons between studies, it is important to report any data that could potentially influence the outcome. Despite the development of specific guidelines such as TOP (Transparency and Openness Promotion; Nosek et al., 2015), ARRIVE (Animal Research: Reporting of In Vivo Experiments; Kilkenny et al., 2010; Percie du Sert et al., 2020) or PREPARE (Planning Research and Experimental Procedures on Animals: Recommendations for Excellence; Smith et al., 2018), there are still considerable gaps and discrepancies in the experimental information reported in the scientific literature. Establishing a standard method that includes key information can help researchers to navigate through essential variables and ensure that described study protocols are both complete and adequately detailed as well as reported in a consistent and standardized manner. The use of standardized animal model avoids the time-consuming process of developing in vivo protocols and reduces the variability of the results. Therefore, it adheres to the 3R principle, reducing the number of animals required in preclinical studies. In addition, the development of a standardized murine pneumonia model validated with at least one reference compound will enable antibiotic benchmarking and serve as a quality control mechanism of the results obtained between laboratories.
The European Innovative Medicines Initiative (IMI) Antimicrobial Resistance (AMR) Accelerator was created with the main goal of advancing the development of new medicines to treat or prevent resistant bacterial infections worldwide. Within the AMR Accelerator, the “Collaboration for prevention and treatment of MDR bacterial infections” (COMBINE) consortium aims to develop a standardized, quality-controlled murine pneumonia model for preclinical efficacy testing of novel anti-infective candidates and to improve tools for the translation of preclinical data to the clinic. Success in translational medicine heavily depends on the selected animal models and the experimental set up of the animal model (Hooijmans and Ritskes-Hoitinga, 2013; Denayer et al., 2014). In addition, the success of characterizing pharmacokinetics and pharmacodynamic (PK/PD) targets in animal models relies largely on host and microbial study design features and the ability to control variance (Andes and Craig, 2002; Andes and Lepak, 2017; Bulitta et al., 2019). Although recommendations for in vivo PK/PD studies have been published (Andes and Lepak, 2017; Bulitta et al., 2019), there is still a need for globally harmonized preclinical models.
This focused literature review aims to describe the variability in study methods and experimental protocols for the mouse lung infection model used to test antimicrobial efficacy. This is an essential preliminary step to advance the development of standardized preclinical animal models. Our review focused on murine lung infection models of the most relevant MDR Gram-negative pathogens, Pseudomonas aeruginosa, Klebsiella pneumoniae and Acinetobacter baumannii, used in proof-of-concept and/or primary pharmacology studies for small molecule antibiotics. The findings were further shared and discussed by a panel of experts at an online workshop organized by the COMBINE consortium. The resulting recommendations for standard design parameters are presented in the following joint article: “Expert Workshop Summary: Advancing toward a standardized murine model to evaluate treatments for AMR lung infections” and they will provide the basis for the development of a harmonized and bench-marked murine lung PK/PD model.
Established protocols for murine pneumonia models were collected from industrial, academic, and governmental institutions. A total of sixteen protocols from ten different institutions were reviewed and compared to create a list of parameters that varied between protocols. Furthermore, a scientific literature search was performed to investigate the variability of mouse pneumonia model protocols in published studies. Parameters from the established institutional protocols were excluded from the data analysis of the literature findings.
Study selection followed SYstematic Review Center for Laboratory animal Experimentation (SYRCLE) guidelines (Leenaars et al., 2012). The search strategy consisted of the identification and definition of three search components: mouse model, pneumonia caused by P. aeruginosa, K. pneumoniae and/or A. baumannii, and drug therapy. A total of 25 Mesh terms and 13 free text terms limited to the title and abstract were used for a literature search in PubMed (Supplementary Table 1). The studies selection process is summarized in Figure 1. A total of 601 preliminary studies were retrieved, of which 358 studies were published within the last decade in the English language. Of these, 192 publications were excluded following a title and abstract review due to not being primary studies, the disease of interest (murine pneumonia model), or not being focused on the desired intervention; thus, 166 publications were considered to be initially relevant. Following the exclusion of additional studies that focused on interventions or therapies other than small molecule antibiotics (monoclonal antibodies, bacteriophages, metal chelators, plant extracts, Lactobacillus, etc.), 53 studies remained (López-Rojas et al., 2011; Pachón-Ibáñez et al., 2011; Docobo-Pérez et al., 2012; Tang et al., 2012; Wang et al., 2012; Yamada et al., 2012, 2013a,b; He et al., 2013; Hirsch et al., 2013; Jacqueline et al., 2013; Louie et al., 2013, 2015; Harada et al., 2014; Hengzhuang et al., 2014; Yokoyama et al., 2014; Bowers et al., 2015; Cheah et al., 2015; Berkhout et al., 2016; Brunetti et al., 2016; Cigana et al., 2016; Lepak and Andes, 2016; Mardirossian et al., 2016; McCaughey et al., 2016; Parra Millán et al., 2016; Thabit et al., 2016; Yang et al., 2016; Kaku et al., 2017a,b; Li Y. T. et al., 2017, Li Y. et al., 2017; Lin et al., 2017a,b, 2018; Oshima et al., 2017; Sakoulas et al., 2017; Zhou J. et al., 2017, Zhou Y. F. et al., 2017; Avery et al., 2018; Chen et al., 2018; de Paula et al., 2018; Geller et al., 2018; Lou et al., 2018; Monogue et al., 2018; Kirby et al., 2019; Ku et al., 2019; Nakamura et al., 2019; Ren et al., 2019; Sanderink et al., 2019; Zhao et al., 2019; Johnson et al., 2020; Tan et al., 2020; Ma X. L. et al., 2020; Supplementary Table 2). Murine model variables were extracted from these articles to generate a data set for further analyses of experimental conditions. The data set contained studies from 14 different countries published from 2011 to 2020 (Supplementary Table 2).
Pseudomonas aeruginosa, Klebsiella pneumoniae and Acinetobacter baumannii are among the most common and difficult to treat opportunistic pathogens in nosocomial infections such as ventilator-associated pneumonia in immunocompromised patients (Ma Y. et al., 2020). We observed that antibiotic efficacy was most commonly evaluated against P. aeruginosa with 22 of the 53 studies reviewed including this pathogen. This pathogen is commonly involved in pneumonia of cystic fibrosis patients (Oliver et al., 2000). Although antimicrobial agents may be efficacious against more than one of these Gram-negative pathogens (Paterson et al., 2020), only a few of the published studies included the in vivo efficacy against two or three of the pathogens in separate experiments (termed “combination of bacteria,” Table 1 and Figure 2). Evaluation of antibacterial monotherapy was the most common study objective in the studies reviewed, although studies with A. baumannii focused mostly on the evaluation of a combination of therapies (18.9% of studies). Despite the increased attention given to drug delivery methods (Li et al., 2019), few studies have focused on the evaluation of alternative routes of drug administration (Table 1). This consisted mainly of aerosolization or liposomes for pulmonary administration of colistin and polymyxin B (He et al., 2013; Li Y. et al., 2017; Lin et al., 2017a,b, 2018). P. aeruginosa was the pathogen of choice for these investigations (7.5% of all studies, Table 1).
Figure 2. Circular dendrogram representing hierarchically structured variables. The area of the nodes represents the number of studies. Hierarchy from inside to outside: bacteria, mouse strain (Inb.: Inbred, Out.: Outbred), mouse immune status (NT: neutropenic, IC: Immunocompetent), study main readout (S: mice survival, B: bacterial load) and infection route (IT: intratracheal, IN: intranasal, ORP: oropharyngeal, IB: intrabronchial, AE: aerosolization).
There are no firm requirements for the number of bacterial strains to be included in preclinical studies for the evaluation of novel antimicrobials in vivo. However, regulatory guidance (European Medicines Agency, 2016) and scientific recommendations (Bulik et al., 2017; Bulitta et al., 2019) suggest to include at least four strains of each target pathogen species for establishing PK/PD targets. Ideally, these should include a reference strain and be representative of contemporary, relevant resistance profiles and mechanisms (European Medicines Agency, 2016; Bulik et al., 2017; Bulitta et al., 2019). We observed that most of the studies included only one or two bacterial strains, especially those focusing on P. aeruginosa (35.8% of all studies). Less than one third of the studies tested three or more strains of the same species (Table 1). The value of testing several strains is that it accounts for genetic and biological variation within the target species which may affect strain fitness and susceptibility and therefore the overall efficacy assessment of the investigated drug (Andes and Lepak, 2017).
The source of the bacterial strain was reported in 67.9% of reviewed studies (Table 1). Private clinical isolates were the most common source of bacteria for A. baumannii and P. aeruginosa. The lack of globally accessible reference strains with corresponding in vivo benchmark data may partially explain why some studies used a strain obtained from another researcher (Table 1). Therefore, it would be highly recommended to deposit in vivo pathogenic strains (and associated data) in biorepositories to make them accessible to other researchers.
Another variable expected to impact bacterial fitness and infectivity is the preparation of the bacterial inoculum. However, the details related to the inoculum preparation procedure were rarely included. Only 28.3% of studies reported the bacterial growth stage at the time of infection. Of these, a fresh bacterial subculture in logarithmic phase of growth was generally employed for the infection and the use of frozen stocks was limited (Table 1). Some virulence factors are differentially expressed between logarithmic and stationary phase. Their expression often increases in stationary phase, when the cell density is high and bacteria are subjected to higher biological stress (Carter et al., 2007; Bravo et al., 2008; Choi et al., 2011). P. aeruginosa quorum sensing signal increases at late stationary phase (Choi et al., 2011). In Salmonella and Shigella, the production of the lipopolysaccharide long-chain O-antigen increases in the late exponential and stationary growth phases, which affects serum resistance (Carter et al., 2007; Bravo et al., 2008). Despite this, bacteria in logarithmic phase are overwhelmingly employed for in vivo infection for a number of reasons. First, the ability of logarithmic phase bacteria to survive and establish a robust infection in the lung is consistently reproducible. Second, logarithmic phase bacteria can be more accurately quantified in the inoculum. It is technically challenging if not impossible to produce a stationary phase culture containing no dead cells. The effects, of employing bacterial cultures in different growth stages or bacteria cultured on liquid vs solid media is currently unknown. Bacterial growth stage is rarely reported; and very few studies reported the specific stage of the log phase (Table 1). However, to be able to increase the reproducibility of the preclinical studies, it is recommended to describe the culture conditions precisely and occasionally to monitor the expression of virulence factors.
The selection of mouse strain is a choice that should be carefully considered. Outbred mice were used more frequently than inbred mice. Swiss Webster mice were the most common outbred stock, followed by ICR mice. With regard to inbred mice, C57BL/6 and BALB/c mice were used most frequently (Table 2). The preference for inbred vs. outbred mice varied depending on the bacterial species under investigation. Studies performed with P. aeruginosa mainly used outbred mice, while for A. baumannii inbred mice slightly predominated (Figure 2). When working with K. pneumoniae, inbred and outbred mice were used to a similar extent (Figure 2). Outbred mice are generally selected for PK/PD studies in murine lung and thigh models (Andes and Lepak, 2017; Bulitta et al., 2019). It has been described that Pseudomonas infection led to higher mortality in BALB/c mice (classified as a Th2 responder strain) compared to C3H/HeN mice (Th1 responders; Moser et al., 1997) and C57BL/6 mice are more susceptible to Klebsiella infection of the lung than are 129/Sv mice (Schurr et al., 2005). Inbred mice are presumed to be more uniform (thus decreasing the number of animals needed to detect a specific response) and more repeatable (a result of being genetically defined and less prone to genetic change; Festing, 2014). However, to date there is no evidence of greater trait stability in inbred mice. This suggests that the advantages of inbred mice may not be as great as previously supposed and that the use of outbred mice in biomedical research may provide an important advantage in reaching conclusions that are generalizable across conditions and populations (Tuttle et al., 2018).
The sex of experimental animals is known to impact host-pathogen interactions (García-Gómez et al., 2013). We observed that the vast majority of the studies were carried out in female mice regardless of the bacteria used for infection (Table 2). Interestingly, only one study used both female and male mice in separate experiments with different readouts (Kirby et al., 2019; Table 2). Female C57BL/6J mice have been shown to be more susceptible to A. baumannii lung infection than their male counterparts (Pires et al., 2020). However, an increased susceptibility of male C3HeB/FeJ mice has been reported in the oral aspiration pneumonia model (Luna et al., 2019). In humans, women with cystic fibrosis and P. aeruginosa infection have worse outcomes than men (Demko et al., 1995), which has been partly attributed to estrogen effects (Vidaillac et al., 2020). In the case of K. pneumoniae infection, female mice have showed higher survival rates than males although exposure to ozone reversed the trend and resulted in female mice surviving less than males (Mikerov et al., 2011). The sex of the test animal can also have implications in drug PK/PD (Soldin and Mattison, 2009; Madla et al., 2021). Therefore, the selection of male or female mice in an antimicrobial efficacy study is a variable that should be carefully considered and taken into account when interpreting the results.
The age of mice used in the study could substantially affect the immune response and thus the infection outcome (Cai et al., 2016; Jackson et al., 2017). Older mice have a more mature immune response. It has been shown in mice that B-cells have an immature phenotype until 4 weeks of age (Ghia et al., 1998) and T-cell responses mature around 8 weeks of age (Holladay and Smialowicz, 2000). In addition, drug metabolism by the liver is affected by the age of mice and has a critical impact on systemically administered compounds (Pibiri et al., 2015). This variable is also related to the weight of the animal and in some cases this parameter is reported instead of the age. For data analysis, we transformed the weight of the mice (if it was the only data provided) to age in weeks according to the vendor’s growth data provided for the mouse strain of interest. The age of mice ranged from 4 to 10 weeks and animals with an average age of 6 weeks were most common. Outbred mice tended to be younger than inbred mice, and no inbred mice younger than 6 weeks were used (Table 2). Although outbred mice grow faster than inbred mice, their immune system may not be fully developed at the selected age (Ghia et al., 1998; Holladay and Smialowicz, 2000). This may be less of a problem for studies using outbred animals, as they are often rendered neutropenic or used at older ages in immunocompetent models. Several guidelines such as ARRIVE have strongly encouraged reporting the age of animals used in an experiment (Kilkenny et al., 2010; Percie du Sert et al., 2020). However, it is not clear if the age reported in the studies refers to the age of an animal upon arrival at the facility (before the acclimatization period), the age when the experimental infection is performed or the age when the first intervention in the animals is executed (i.e., cyclophosphamide treatment). This could lead to even greater variation in age than immediately apparent. The host microbiota correlates with animal age and affects host response and lung infection resistance (McMahan et al., 2022). The mouse microbiome varies based on a number of factors, including the animal’s origin, nutrition, housing, bedding, and care during infancy (Ericsson and Franklin, 2021). Around 6 weeks of age, the lung’s microbial diversity significantly increases and is maintained throughout time (Singh et al., 2017). Researchers should make an effort to minimize the effects of this factor on the host’s susceptibility to infection in order to improve the reproducibility of the studies.
The number of animals per group should be selected based on a power analysis considering expected data dispersion (Festing, 2018; Bulitta et al., 2019). We observed broad variation in the number of animals included per group. In studies with bacterial burden as the main endpoint, a range of two to fifteen animals was used, with four to six animals per group in most of the studies. In studies with survival as the study endpoint, the most common group size was 7–10 animals per group, reflecting that survival data typically present higher variability than bacterial burden (Table 2).
Induced neutropenia is a variable that can have a significant impact on the study outcomes (Andes and Craig, 2002; Andes and Lepak, 2017). In general, a higher PK/PD index magnitude is required in neutropenic animals although it varies among drug classes and bacterial species (Andes and Craig, 2002; Andes and Lepak, 2017). We observed that neutropenic mice were used more often than immunocompetent mice (Table 3). Immunocompetent mice were preferred when working with inbred mouse strains, while the use of neutropenic animals prevails when outbred mice were used. This confirms the results of a previous literature review that evaluated different animal models for antibiotic efficacy testing, which found that neutropenic models slightly predominate over immunocompetent ones (Waack et al., 2020). Our analysis showed that the immune status of the mice varied by the study outcome measured. Survival was most frequently evaluated in immunocompetent animals. P. aeruginosa studies employed mostly immunocompetent lung models whereas A. baumannii and K. pneumoniae infections were mostly conducted in neutropenic models (Figure 2). Neutropenic animals were found to have increased bacterial growth over the study period in untreated animals (Table 4). Despite a common misconception, use of immunocompromised mice is not generally intended to mimic any particular patient population (Zhao et al., 2016; Andes and Lepak, 2017). Neutropenia promotes better growth of bacteria in mice, thus minimizing spontaneous resolution of infection (which complicates interpretation of treatment effects) and enabling more strains to be studied in mice than otherwise might be possible.
Neutropenia can be induced by different methods, including the use of drugs such as cyclophosphamide or vinblastine and neutrophil depleting antibodies (Stackowicz et al., 2020). Cyclophosphamide has a relative low cost and produce depletion of hematopoietic stem cells associated with an almost complete disappearance of blood neutrophils as early as 3–4 days after injection (Van’t Wout et al., 1989; Zuluaga et al., 2006). Cyclophosphamide has the greatest effect on neutrophil numbers, but also markedly reduces numbers of circulating monocytes, B and T cells (Van’t Wout et al., 1989; Zuluaga et al., 2006). Cyclophosphamide was the only methodology employed in the reviewed studies to render animals neutropenic. More than half of the studies with immunocompromised mice used a protocol of administering cyclophosphamide 4 days (150 mg/kg) and one day (100 mg/kg) before infection. The remaining studies used slight variations, such as increasing the administered dose or varying the days of administration.
The route of infection has been shown to impact host-pathogen interactions (Martins et al., 2013). In the reviewed studies, intratracheal (IT) and intranasal (IN) routes of infection were the most common, followed by aerosolization, the intrabronchial route and oropharyngeal infection route. Infections with A. baumannii and P. aeruginosa were mostly achieved through IT bacterial inoculation, while K. pneumoniae infection protocols mostly used IN bacterial administration (Figure 2). Moreover, we observed that the selection of the infection route was related to the immune status of the animal. IN infection was mostly employed when working with neutropenic animals, while for immunocompetent animals, IT infection was most common (Table 3). The predominance of the IN route when using neutropenic animals and the IT when employing immunocompetent animals was also observed in a previous report that summarized studies of antibiotic efficacy against seven gram-negative and two gram-positive pneumonia-causing bacterial species (Waack et al., 2020). This variation may reflect the challenge of establishing lung infections in immunocompetent mice, where a more direct inoculation method such as IT route can lead to a greater success. In our reviewed studies using three gram-negative bacteria, we observed a higher percentage of immunocompetent animals infected via IT route than previously reported using a higher number of pneumonia-producing bacteria (17), suggesting that the infection with these three selected gram-negative bacteria may be more difficult to achieve.
The infection volume is closely related to both the employed route of infection and the infectious dose. The administered volume ranged from 10 to 70 μl and most of the studies used a volume of 50 μl (Table 3). The lowest volume of 10 μl was only used for IT bacterial delivery (He et al., 2013; Hirsch et al., 2013).
It has previously been shown in a murine model of tularemia that intranasal instillation of a volume of 10 μl routinely resulted in infection of the upper airways but failed to initiate infection of the pulmonary compartment. For efficient delivery of the bacteria into the lungs, a dose volume of 50 μl or more was required (Miller et al., 2012). Similarly, studies with the azo dye Evans blue have revealed that intranasal administration of a dye volume of 40–50 μl, in comparison to 10–20 μl, led to increased dye retention in the lungs (Visweswaraiah et al., 2002; Smith et al., 2019). These data suggest that infecting volumes of at least 50 μl IN are preferred for establishing robust pulmonary infection.
Before selecting bacterial strains and infectious dose to perform antimicrobial efficacy studies, bacterial pathogenicity studies in mice are required. The infectious inoculum varied greatly between the reviewed studies. Most indicated that 7–8 log10 CFU were administered to initiate the infection (Table 3). Studies working with P. aeruginosa and K. pneumoniae used lower infectious dose than the studies working with A. baumannii (Table 3). Considering that the neutropenic model already prevails when working with this pathogen (Figure 2), these data may suggest greater difficulty in establishing a robust A. baumannii lung infection, requiring higher infectious dose.
In antibacterial research, the length of the study varies between animal species. Murine pneumonia models are mostly used for short term studies, while larger animals usually employ later endpoints (Waack et al., 2020). The vast majority of the studies reviewed here initiated antibiotic therapy at 2 h post infection (h.p.i.). However, the timing of the experimental endpoint varied according to the main study readout. Studies to determine bacterial burden were mostly terminated at 24 or 26 h.p.i., or at multiple time points between 2 and 72 h.p.i. Survival studies were most commonly terminated after 3–4 days (Table 4). A previous review of new drug application dossiers found that an endpoint of 24–29 h.p.i. was most commonly employed when antibacterial activity against P. aeruginosa, K. pneumoniae and A. baumannii was investigated. Notably, these studies followed a bimodal distribution, having 24- or 48-h endpoints (Waack et al., 2020). The endpoint used for measuring antimicrobial activity could influence the results (Andes and Craig, 2002; Cigana et al., 2020); however, the evaluation of antimicrobial treatments for chronic infection requires longer experimental time points (Cigana et al., 2020). Additionally, there might be variations in how researchers analyze animal care and humane endpoints in survival studies. Clinical disease severity scoring can be subjective, with various researchers scoring severity differently.
Unfortunately, the lung bacterial burden at the start of therapy was only reported in 28.3% of the reviewed studies. Baseline burdens fluctuated from 4.7 to 8 log10 CFU per lung, with the majority falling into a range of 6–7 log10 CFU per lung (Table 4). A lower burden at the start of therapy may reduce the PK/PD index magnitude, thus reducing the dose required for treatment effect, although the degree of influence can vary depending on the bacterial species and the drug class (Andes and Lepak, 2017). Importantly, the majority of results from PK/PD animal models that have been correlated with clinical outcomes reached 6–7 log10 CFU in the target tissue at the time therapy was initiated (Andes and Lepak, 2017). Baseline burdens further affect the bacterial growth over the study period. Bacterial growth was reported in 34 of the 53 studies reviewed, and an increase of at least 1 log10 CFU/lung over the experimental period (ranged from 2 and 72 h.p.i, median 26 h.p.i) was achieved in most of the studies. There were no marked differences among the three different bacterial species. However, bacterial growth over the study period was indeed related to immune status, with neutropenic mice showing higher bacterial growth than immunocompetent mice (Table 4). Robust infections are a prerequisite to adequately assess the antimicrobial effect of drugs. Therefore, bacterial burdens in untreated animals should not decline over the course of the study, or it becomes difficult to separate treatment effect from spontaneous resolution of infection. Depending on the selected efficacy endpoint, the effect of treatment may also be overestimated if bacterial growth is poor. The recommended specific efficacy endpoints range from 0 (stasis) to 2 log10 reductions in CFU calculated relative to the bacterial density at the start of treatment (Drusano, 2004; European Medicines Agency, 2016; Bulitta et al., 2019) and there remains some debate over which should be used. Stasis may be adequate for less severe infections or those involving concomitant non-drug treatments (e.g., surgical intervention such as debridement of infected tissue). For infections involving skin, soft tissue or the urinary tract, a 1 log10 reduction has been recommended, whereas 2 log10 reductions have been recommended for severe and/or high bacterial burden infections such as pneumonia (Drusano et al., 2018; Bulitta et al., 2019). In the control group (untreated or vehicle-treated), an increase in bacterial burden of 2–3 log10 CFU over the course of the study has been recommended (Bulitta et al., 2019). While this may be a feasible target for the standard neutropenic thigh infection model, studies reviewed suggest that it may be more difficult to achieve in the lung model especially with some pathogens (e.g., A. baumannii) or bacterial strains.
In the studies reviewed, specific information on sample processing was scarce. The method of lung homogenization was only reported in 15% of the studies. Stomacher, mini bead beater and ultra-turrax were the most common methodologies for processing samples. The media used for lung tissue culture and CFU count varied widely, with Mueller-Hinton (I and II) as the preferred media (47.6% of the studies) followed by Luria-Bertani (LB) agar (14.3%). Agar plates were usually cultured at 37°C, although several studies reported an incubation temperature of 35°C for P. aeruginosa. It is important to optimize the culture conditions for inoculation and bacterial recovery from the lung. Different sample processing techniques and media may have an impact on the study results, and it is recommended that all study samples are processed using the same methodology. Working with blinded samples is strongly encouraged, as it may reduce the potential for bias and increase the robustness of the study (Ioannidis, 2012; Bespalov et al., 2020).
Monoparametric models employing a single indicator of antibiotic efficacy represented 39.6% of the studies reviewed. Lung bacterial burden was the most common study readout (Table 4). While some studies reported the data as CFU/lung, others reported the data as CFU/ml of homogenized lung. CFU/ml requires additional data (lung weight) for normalization and comparison of results between studies, but lung weight was not generally reported. Regardless of the reporting units, the reduction of bacterial burden at the site of infection provides a relatively reproducible measure of antibiotic action that has been shown to forecast drug efficacy in patients (Zak and O’Reilly, 1991; Andes and Lepak, 2017). Bacterial burden is generally the preferred endpoint because it is a direct measurement of the drug’s ability to kill or halt growth of the infecting pathogen. However, mortality can also be a useful endpoint and, in our review, survival of the mice was the second most common readout. The relationship between bacterial burden and survival has been previously noted, and the magnitude of drug exposure required for bacteriological cure and survival has been shown in some studies to be similar (Craig and Dalhoff, 1998; Andes and Craig, 2002). However, it should be noted that survival studies which monitor the animal’s health for prolonged periods of time after the end of therapy may allow organisms that have not been eradicated to regrow and cause mortality, especially in neutropenic animals. This could substantially impact the relationship between bacterial counts and survival (Andes and Craig, 2002). In addition to survival and bacterial burden in the lungs, other outcomes in the studies reviewed included lung histopathology, bacterial load in other tissues (e.g., blood, liver or spleen), assessment of immune responses (e.g., cytokines) in BAL, serum, or lung homogenate, clinical scoring based on animal observation, body temperature, protein expression, and pulmonary endothelial permeability.
Standardization of the murine pneumonia model would allow better translation to the clinic and reduce animal use by providing more useful and relevant data. This literature review revealed marked differences in methodology for the murine pneumonia model used to test efficacy of small molecule antibiotics. Several parameters were relatively consistent across most of the models reviewed. These included animal sex, stage of bacterial growth for the inoculum, the starting point for treatment and the primary study outcome. Other variables differed widely, such as the immune status and age of the mice, the infection route and sample processing methodologies. Some variables, such as immune status and infectious dose, are expected to impact the study outcome more than others and can affect the PK/PD magnitude measured for a given endpoint. Although there is little direct evidence to indicate what effect, if any, a particular variable has on the outcome of a study, the myriad combinations of variables that any particular investigator uses is likely to impact the observed results. This can complicate preclinical-to-clinical translation, makes it difficult to compare drugs and/or bacterial isolates tested in different laboratories, and highlights the need for development of a standardized murine pneumonia model that has been benchmarked appropriately. Overall, standardization of animal infection models is expected to strengthen the reproducibility and comparability of data generated during the evaluation of novel antibiotics. Furthermore, the combination of standardized protocols with quality controls, such as bacterial reference strains and benchmark control compounds with specified potency, should increase the robustness of preclinical data and improve our ability to translate from animals to humans.
RA: data curation, analysis, and writing—original draft. All authors: conceptualization, manuscript review and editing, and read and approved the final manuscript.
COMBINE project has received funding from the Innovative Medicines Initiative 2 Joint Undertaking under grant agreement No. 853967. This Joint Undertaking receives support from the European Union’s Horizon 2020 Research and Innovation Programme and EFPIA companies’ in-kind contribution. https://www.imi.europa.eu/.
We thank Evotec, GlaxoSmithKline, Statens Serum Institut, Erasmus University Medical Center, University of Poitiers, University of Liverpool, Monash University, Pharmacology Discovery Services, the University of North Texas, and the University of Florida for kindly sharing their established institutional protocols with COMBINE.
JLH was employed by GlaxoSmithKline Pharmaceuticals. SS and SR were employed by Evotec.
The remaining authors declare that the research was conducted in the absence of any commercial or financial relationships that could be construed as a potential conflict of interest.
All claims expressed in this article are solely those of the authors and do not necessarily represent those of their affiliated organizations, or those of the publisher, the editors and the reviewers. Any product that may be evaluated in this article, or claim that may be made by its manufacturer, is not guaranteed or endorsed by the publisher.
This communication reflects the views only of the authors from the COMBINE consortium and does not necessarily reflect the position of IMI, the European Union, EFPIA partners or regulatory agencies.
The Supplementary Material for this article can be found online at: https://www.frontiersin.org/articles/10.3389/fmicb.2022.988728/full#supplementary-material
Andes, D., and Craig, W. A. (2002). Animal model pharmacokinetics and pharmacodynamics: A critical review. Int. J. Antimicrob. Agents 19, 261–268. doi: 10.1016/S0924-8579(02)00022-5
Andes, D. R., and Lepak, A. J. (2017). In vivo infection models in the pre-clinical pharmacokinetic/pharmacodynamic evaluation of antimicrobial agents. Curr. Opin. Pharmacol. 36, 94–99. doi: 10.1016/j.coph.2017.09.004
Avery, L. M., Abdelraouf, K., and Nicolau, D. P. (2018). Assessment of the in vivo efficacy of WCK 5222 (Cefepime-Zidebactam) against carbapenem-resistant Acinetobacter baumannii in the neutropenic murine lung infection model. Antimicrob. Agents Chemother. 62:e00948-18. doi: 10.1128/AAC.00948-18
Begley, C. G., and Ioannidis, J. P. A. (2015). Reproducibility in science: Improving the standard for basic and preclinical research. Circ. Res. 116, 116–126. doi: 10.1161/CIRCRESAHA.114.303819
Bekeredjian-Ding, I. (2020). Challenges for clinical development of vaccines for prevention of hospital-acquired bacterial infections. Front. Immunol. 11:1755. doi: 10.3389/fimmu.2020.01755
Berkhout, J., Melchers, M. J., Van Mil, A. C., Seyedmousavi, S., Lagarde, C. M., Schuck, V. J., et al. (2016). Pharmacodynamics of ceftazidime and avibactam in neutropenic mice with thigh or lung infection. Antimicrob. Agents Chemother. 60, 368–375. doi: 10.1128/AAC.01269-15
Bespalov, A., Wicke, K., and Castagné, V. (2020). Blinding and randomization. Handb. Exp. Pharmacol. 257, 81–100. doi: 10.1007/164_2019_279
Bielen, K., Jongers, B., Malhotra-Kumar, S., Jorens, P. G., Goossens, H., and Kumar-Singh, S. (2017). Animal models of hospital-acquired pneumonia: Current practices and future perspectives. Ann. Transl. Med. 5:132. doi: 10.21037/atm.2017.03.72
Bowers, D. R., Cao, H., Zhou, J., Ledesma, K. R., Sun, D., Lomovskaya, O., et al. (2015). Assessment of minocycline and polymyxin B combination against Acinetobacter baumannii. Antimicrob. Agents Chemother. 59, 2720–2725. doi: 10.1128/AAC.04110-14
Bravo, D., Silva, C., Carter, J. A., Hoare, A., Álvarez, S. A., Blondel, C. J., et al. (2008). Growth-phase regulation of lipopolysaccharide O-antigen chain length influences serum resistance in serovars of Salmonella. J. Med. Microbiol. 57, 938–946. doi: 10.1099/jmm.0.47848-0
Brunetti, J., Falciani, C., Roscia, G., Pollini, S., Bindi, S., Scali, S., et al. (2016). In vitro and in vivo efficacy, toxicity, bio-distribution and resistance selection of a novel antibacterial drug candidate. Sci. Rep. 6:26077. doi: 10.1038/srep26077
Bulik, C. C., Okusanya, Ó. O., Lakota, E. A., Forrest, A., Bhavnani, S. M., Hoover, J. L., et al. (2017). Pharmacokinetic-pharmacodynamic evaluation of gepotidacin against gram-positive organisms using data from murine infection models. Antimicrob. Agents Chemother. 61:e00115-16. doi: 10.1128/AAC.00115-16
Bulitta, J. B., Hope, W. W., Eakin, A. E., Guina, T., Tam, V. H., Louie, A., et al. (2019). Generating robust and informative nonclinical in vitro and in vivo bacterial infection model efficacy data to support translation to humans. Antimicrob. Agents Chemother. 63:e02307-18. doi: 10.1128/AAC.02307-18
Cai, K. C., van Mil, S., Murray, E., Mallet, J. F., Matar, C., and Ismail, N. (2016). Age and sex differences in immune response following LPS treatment in mice. Brain Behav. Immun. 58, 327–337. doi: 10.1016/j.bbi.2016.08.002
Carter, J. A., Blondel, C. J., Zaldívar, M., Álvarez, S. A., Marolda, C. L., Valvano, M. A., et al. (2007). O-antigen modal chain length in Shigella flexneri 2a is growth-regulated through RfaH-mediated transcriptional control of the wzy gene. Microbiology 153, 3499–3507. doi: 10.1099/mic.0.2007/010066-0
Cheah, S. E., Wang, J., Nguyen, V. T. H. T., Turnidge, J. D., Li, J., and Nation, R. L. (2015). New pharmacokinetic/pharmacodynamic studies of systemically administered colistin against Pseudomonas aeruginosa and Acinetobacter baumannii in mouse thigh and lung infection models: Smaller response in lung infection. J. Antimicrob. Chemother. 70, 3291–3297. doi: 10.1093/jac/dkv267
Chen, C., Deslouches, B., Montelaro, R. C., and Di, Y. P. (2018). Enhanced efficacy of the engineered antimicrobial peptide WLBU2 via direct airway delivery in a murine model of Pseudomonas aeruginosa pneumonia. Clin. Microbiol. Infect. 24, 547.e1–547.e8. doi: 10.1016/j.cmi.2017.08.029
Choi, Y., Park, H. Y., Park, S. J., Park, S. J., Kim, S. K., Ha, C., et al. (2011). Growth phase-differential quorum sensing regulation of anthranilate metabolism in Pseudomonas aeruginosa. Mol. Cells 32, 57–65. doi: 10.1007/s10059-011-2322-6
Cigana, C., Bernardini, F., Facchini, M., Alcalá-Franco, B., Riva, C., De Fino, I., et al. (2016). Efficacy of the novel antibiotic POL7001 in preclinical models of Pseudomonas aeruginosa pneumonia. Antimicrob. Agents Chemother. 60, 4991–5000. doi: 10.1128/AAC.00390-16
Cigana, C., Ranucci, S., Rossi, A., De Fino, I., Melessike, M., and Bragonzi, A. (2020). Antibiotic efficacy varies based on the infection model and treatment regimen for Pseudomonas aeruginosa. Eur. Respir. J. 55:1802456. doi: 10.1183/13993003.02456-2018
Craig, W., and Dalhoff, A. (1998). “Pharmacodynamics of fluoroquinolones in experimental animals,” in Quinolone antibacterials. handbook of experimental pharmacology, Vol. 127, eds J. Kuhlmann, A. Dalhoff, and H. J. Zeiler (Berlin: Springer), 207–232. doi: 10.1007/978-3-642-80364-2_7
de Paula, T. P., Santos, P. C., do Nascimento Arifa, R. D., Vieira, A. T., de Matos Baltazar, L., Ávila, T. V., et al. (2018). Treatment with atorvastatin provides additional benefits to imipenem in a model of gram-negative pneumonia induced by Klebsiella pneumoniae in mice. Antimicrob. Agents Chemother. 62:e00764-17. doi: 10.1128/AAC.00764-17
Demko, C. A., Byard, P. J., and Davis, P. B. (1995). Gender differences in cystic fibrosis: Pseudomonas aeruginosa infection. J. Clin. Epidemiol. 48, 1041–1049. doi: 10.1016/0895-4356(94)00230-N
Denayer, T., Stöhrn, T., and Van Roy, M. (2014). Animal models in translational medicine: Validation and prediction. New Horiz. Transl. Med. 2, 5–11. doi: 10.1016/j.nhtm.2014.08.001
Dietert, K., Gutbier, B., Wienhold, S. M., Reppe, K., Jiang, X., Yao, L., et al. (2017). Spectrum of pathogen- and model-specific histopathologies in mouse models of acute pneumonia. PLoS One 12:e0188251. doi: 10.1371/journal.pone.0188251
Docobo-Pérez, F., Nordmann, P., Domínguez-Herrera, J., López-Rojas, R., Smani, Y., Poirel, L., et al. (2012). Efficacies of colistin and tigecycline in mice with experimental pneumonia due to NDM-1-producing strains of Klebsiella pneumoniae and Escherichia coli. Int. J. Antimicrob. Agents 39, 251–254. doi: 10.1016/j.ijantimicag.2011.10.012
Drusano, G. L. (2004). Antimicrobial pharmacodynamics: Critical interactions of “bug and drug.”. Nat. Rev. Microbiol. 2, 289–300. doi: 10.1038/nrmicro862
Drusano, G. L., Corrado, M. L., Girardi, G., Ellis-Grosse, E. J., Wunderink, R. G., Donnelly, H., et al. (2018). Dilution factor of quantitative bacterial cultures obtained by bronchoalveolar lavage in patients with ventilator-associated bacterial pneumonia. Antimicrob. Agents Chemother. 62:e01323-17. doi: 10.1128/AAC.01323-17
Ericsson, A. C., and Franklin, C. L. (2021). The gut microbiome of laboratory mice: Considerations and best practices for translational research. Mamm. Genome 32, 239–250. doi: 10.1007/s00335-021-09863-7
European Medicines Agency (2016). Guideline on the use of pharmacokinetics and pharmacodynamics in the development of antimicrobial medicinal products. Doc. EMA/CHMP/594085/2015. London: European Medicines Agency.
Festing, M. F. W. (2014). Evidence should trump intuition by preferring inbred strains to outbred stocks in preclinical research. ILAR J. 55, 399–404. doi: 10.1093/ilar/ilu036
Festing, M. F. W. (2018). On determining sample size in experiments involving laboratory animals. Lab. Anim. 52, 341–350. doi: 10.1177/0023677217738268
Friberg, L. E. (2021). Pivotal role of translation in anti-infective development. Clin. Pharmacol. Ther. 109, 856–866. doi: 10.1002/cpt.2182
García-Gómez, E., González-Pedrajo, B., and Camacho-Arroyo, I. (2013). Role of sex steroid hormones in bacterial-host interactions. Biomed Res. Int. 2013:928290. doi: 10.1155/2013/928290
Geller, B. L., Li, L., Martinez, F., Sully, E., Sturge, C. R., Daly, S. M., et al. (2018). Morpholino oligomers tested in vitro, in biofilm and in vivo against multidrug-resistant Klebsiella pneumoniae. J. Antimicrob. Chemother. 73, 1611–1619. doi: 10.1093/jac/dky058
Ghia, P., Ten Boekel, E., Rolink, A. G., and Melchers, F. (1998). B-cell development: A comparison between mouse and man. Immunol. Today 19, 480–485. doi: 10.1016/S0167-5699(98)01330-9
Harada, Y., Morinaga, Y., Kaku, N., Nakamura, S., Uno, N., Hasegawa, H., et al. (2014). In vitro and in vivo activities of piperacillin-tazobactam and meropenem at different inoculum sizes of ESBL-producing Klebsiella pneumoniae. Clin. Microbiol. Infect. 20, O831–O839. doi: 10.1111/1469-0691.12677
He, J., Abdelraouf, K., Ledesma, K. R., Chow, D. S. L., and Tam, V. H. (2013). Pharmacokinetics and efficacy of liposomal polymyxin B in a murine pneumonia model. Int. J. Antimicrob. Agents 42, 559–564. doi: 10.1016/j.ijantimicag.2013.07.009
Hengzhuang, W., Høiby, N., and Ciofu, O. (2014). Pharmacokinetics and pharmacodynamics of antibiotics in biofilm infections of Pseudomonas aeruginosa in vitro and in vivo. Methods Mol. Biol. 1147, 239–254. doi: 10.1007/978-1-4939-0467-9_17
Hirsch, E. B., Guo, B., Chang, K. T., Cao, H., Ledesma, K. R., Singh, M., et al. (2013). Assessment of antimicrobial combinations for Klebsiella pneumoniae carbapenemase-producing K. pneumoniae. J. Infect. Dis. 207, 786–793. doi: 10.1093/infdis/jis766
Holladay, S. D., and Smialowicz, R. J. (2000). Development of the murine and human immune system: Differential effects of immunotoxicants depend on time of exposure. Environ. Health Perspect. 108, 463–473. doi: 10.1289/ehp.00108s3463
Hooijmans, C. R., and Ritskes-Hoitinga, M. (2013). Progress in using systematic reviews of animal studies to improve translational research. PLoS Med. 10:e1001482. doi: 10.1371/journal.pmed.1001482
Hughes, D., and Karlén, A. (2014). Discovery and preclinical development of new antibiotics. Ups. J. Med. Sci. 119, 162–169. doi: 10.3109/03009734.2014.896437
Ioannidis, J. P. A. (2012). Extrapolating from animals to humans. Sci. Transl. Med. 4, s15–s151. doi: 10.1126/scitranslmed.3004631
Jackson, S. J., Andrews, N., Ball, D., Bellantuono, I., Gray, J., Hachoumi, L., et al. (2017). Does age matter? The impact of rodent age on study outcomes. Lab. Anim. 51, 160–169. doi: 10.1177/0023677216653984
Jacqueline, C., Roquilly, A., Desessard, C., Boutoille, D., Broquet, A., Le Mabecque, V., et al. (2013). Efficacy of ceftolozane in a murine model of Pseudomonas aeruginosa acute pneumonia: In vivo antimicrobial activity and impact on host inflammatory response. J. Antimicrob. Chemother. 68, 177–183. doi: 10.1093/jac/dks343
Johnson, A., McEntee, L., Farrington, N., Kolamunnage-Dona, R., Franzoni, S., Vezzelli, A., et al. (2020). Pharmacodynamics of cefepime combined with the novel extended-spectrum-lactamase (ESBL) inhibitor enmetazobactam for murine pneumonia caused by ESBLproducing Klebsiella pneumonia. Antimicrob. Agents Chemother. 64:e00180-20. doi: 10.1128/AAC.00180-20
Kaku, N., Kosai, K., Takeda, K., Uno, N., Morinaga, Y., Hasegawa, H., et al. (2017a). Efficacy and pharmacokinetics of the combination of OP0595 and cefepime in a mouse model of pneumonia caused by extended-spectrum-beta-lactamase-producing Klebsiella pneumoniae. Antimicrob. Agents Chemother. 61:e00828-17. doi: 10.1128/AAC.00828-17
Kaku, N., Morinaga, Y., Takeda, K., Kosai, K., Uno, N., Hasegawa, H., et al. (2017b). Efficacy and pharmacokinetics of ME1100, a novel optimized formulation of arbekacin for inhalation, compared with amikacin in a murine model of ventilator-associated pneumonia caused by Pseudomonas aeruginosa. J. Antimicrob. Chemother. 72, 1123–1128. doi: 10.1093/jac/dkw517
Kilkenny, C., Browne, W., Cuthill, I. C., Emerson, M., and Altman, D. G. (2010). Animal research: Reporting in vivo experiments: The ARRIVE guidelines. Br. J. Pharmacol. 160, 1577–1579. doi: 10.1111/j.1476-5381.2010.00872.x
Kirby, B. D., Al Ahmar, R., Ryan Withers, T., Valentine, M. E., Valentovic, M., Long, T. E., et al. (2019). Efficacy of aerosolized rifaximin versus tobramycin for treatment of Pseudomonas aeruginosa pneumonia in mice. Antimicrob. Agents Chemother. 63:e02341-18. doi: 10.1128/AAC.02341-18
Kraft, B. D., Piantadosi, C. A., Benjamin, A. M., Lucas, J. E., Zaas, A. K., Betancourt-Quiroz, M., et al. (2014). Development of a novel preclinical model of pneumococcal pneumonia in nonhuman primates. Am. J. Respir. Cell Mol. Biol. 50, 995–1004. doi: 10.1165/rcmb.2013-0340OC
Ku, N. S., Lee, S. H., Lim, Y. S., Choi, H., Ahn, J. Y., Jeong, S. J., et al. (2019). In vivo efficacy of combination of colistin with fosfomycin or minocycline in a mouse model of multidrug-resistant Acinetobacter baumannii pneumonia. Sci. Rep. 9:17127. doi: 10.1038/s41598-019-53714-0
Leenaars, M., Hooijmans, C. R., van Veggel, N., ter Riet, G., Leeflang, M., Hooft, L., et al. (2012). A step-by-step guide to systematically identify all relevant animal studies. Lab. Anim. 46, 24–31. doi: 10.1258/la.2011.011087
Lepak, A. J., and Andes, D. R. (2016). In vivo pharmacodynamic target assessment of delafloxacin against Staphylococcus aureus, Streptococcus pneumoniae, and Klebsiella pneumoniae in a murine lung infection model. Antimicrob. Agents Chemother. 60, 4764–4769. doi: 10.1128/AAC.00647-16
Li, C., Wang, J., Wang, Y., Gao, H., Wei, G., Huang, Y., et al. (2019). Recent progress in drug delivery. Acta Pharm. Sin. B 9, 1145–1162. doi: 10.1016/j.apsb.2019.08.003
Li, Y., Tang, C., Zhang, E., and Yang, L. (2017). Electrostatically entrapped colistin liposomes for the treatment of Pseudomonas aeruginosa infection. Pharm. Dev. Technol. 22, 436–444. doi: 10.1080/10837450.2016.1228666
Li, Y. T., Huang, J. R., Li, L. J., and Liu, L. S. (2017). Synergistic activity of berberine with azithromycin against Pseudomonas aeruginosa isolated from patients with cystic fibrosis of lung in vitro and vn vivo. Cell. Physiol. Biochem. 42, 1657–1669. doi: 10.1159/000479411
Li Bassi, G., Rigol, M., Marti, J. D., Saucedo, L., Ranzani, O. T., Roca, I., et al. (2014). A novel porcine model of ventilator-associated pneumonia caused by oropharyngeal challenge with Pseudomonas aeruginosa. Anesthesiology 120, 1205–1215. doi: 10.1097/ALN.0000000000000222
Lin, Y. W., Zhou, Q., Onufrak, N. J., Wirth, V., Chen, K., Wang, J., et al. (2017a). Aerosolized polymyxin B for treatment of respiratory tract infections: Determination of pharmacokinetic-pharmacodynamic indices for aerosolized polymyxin B against Pseudomonas aeruginosa in a mouse lung infection model. Antimicrob. Agents Chemother. 61:e00211-17. doi: 10.1128/AAC.00211-17
Lin, Y. W., Zhou, Q. T., Cheah, S. E., Zhao, J., Chen, K., Wang, J., et al. (2017b). Pharmacokinetics/pharmacodynamics of pulmonary delivery of colistin against Pseudomonas aeruginosa in a mouse lung infection model. Antimicrob. Agents Chemother. 61:e02025-16. doi: 10.1128/AAC.02025-16
Lin, Y. W., Zhou, Q. T., Han, M. L., Chen, K., Onufrak, N. J., Wang, J., et al. (2018). Elucidating the pharmacokinetics/pharmacodynamics of aerosolized colistin against multidrug-resistant Acinetobacter baumannii and Klebsiella pneumoniae in a mouse lung infection model. Antimicrob. Agents Chemother. 62:e01790-17. doi: 10.1128/AAC.01790-17
López-Rojas, R., Sánchez-Céspedes, J., Docobo-Pérez, F., Domínguez-Herrera, J., Vila, J., and Pachón, J. (2011). Pre-clinical studies of a new quinolone (UB-8902) against Acinetobacter baumannii resistant to ciprofloxacin. Int. J. Antimicrob. Agents 38, 355–359. doi: 10.1016/j.ijantimicag.2011.06.006
Lou, W., Venkataraman, S., Zhong, G., Ding, B., Tan, J. P. K., Xu, L., et al. (2018). Antimicrobial polymers as therapeutics for treatment of multidrug-resistant Klebsiella pneumoniae lung infection. Acta Biomater. 78, 78–88. doi: 10.1016/j.actbio.2018.07.038
Louie, A., Liu, W., Fikes, S., Brown, D., and Drusano, G. L. (2013). Impact of meropenem in combination with tobramycin in a murine model of Pseudomonas aeruginosa pneumonia. Antimicrob. Agents Chemother. 57, 2788–2792. doi: 10.1128/AAC.02624-12
Louie, A., Liu, W., Vanguilder, M., Neely, M. N., Schumitzky, A., Jelliffe, R., et al. (2015). Combination treatment with meropenem plus levofloxacin is synergistic against Pseudomonas aeruginosa infection in a murine model of pneumonia. J. Infect. Dis. 211, 1326–1333. doi: 10.1093/infdis/jiu603
Luna, B. M., Yan, J., Reyna, Z., Moon, E., Nielsen, T. B., Reza, H., et al. (2019). Natural history of Acinetobacter baumannii infection in mice. PLoS One 14:e0219824. doi: 10.1371/journal.pone.0219824
Ma, X. L., Guo, Y. Z., Wu, Y. M., Gong, W. T., Sun, J., and Huang, Z. (2020). In vivo bactericidal effect of colistin–linezolid combination in a murine model of MDR and XDR Acinetobacter baumannii pneumonia. Sci. Rep. 10:17518. doi: 10.1038/s41598-020-74503-0
Ma, Y., Wang, C., Li, Y., Li, J., Wan, Q., Chen, J., et al. (2020). Considerations and caveats in combating ESKAPE pathogens against nosocomial infections. Adv. Sci. 7:1901872. doi: 10.1002/advs.201901872
Madla, C. M., Gavins, F. K. H., Merchant, H. A., Orlu, M., Murdan, S., and Basit, A. W. (2021). Let’s talk about sex: Differences in drug therapy in males and females. Adv. Drug Deliv. Rev. 175:113804. doi: 10.1016/j.addr.2021.05.014
Malachowa, N., Kobayashi, S. D., Porter, A. R., Freedman, B., Hanley, P. W., Lovaglio, J., et al. (2019). Vaccine protection against multidrug-resistant Klebsiella pneumoniae in a nonhuman primate model of severe lower respiratory tract infection. mBio 10:e2994-19. doi: 10.1128/mBio.02994-19
Mardirossian, M., Pompilio, A., Crocetta, V., De Nicola, S., Guida, F., Degasperi, M., et al. (2016). In vitro and in vivo evaluation of BMAP-derived peptides for the treatment of cystic fibrosis-related pulmonary infections. Amino Acids 48, 2253–2260. doi: 10.1007/s00726-016-2266-4
Martins, N. E., Faria, V. G., Teixeira, L., Magalhães, S., and Sucena, É. (2013). Host adaptation is contingent upon the infection route taken by pathogens. PLoS Pathog. 9:e1003601. doi: 10.1371/journal.ppat.1003601
Matute-Bello, G., Downey, G., Moore, B. B., Groshong, S. D., Matthay, M. A., Slutsky, A. S., et al. (2011). An official american thoracic society workshop report: Features and measurements of experimental acute lung injury in animals. Am. J. Respir. Cell Mol. Biol. 44, 725–738. doi: 10.1165/rcmb.2009-0210ST
McCaughey, L. C., Ritchie, N. D., Douce, G. R., Evans, T. J., and Walker, D. (2016). Efficacy of species-specific protein antibiotics in a murine model of acute Pseudomonas aeruginosa lung infection. Sci. Rep. 6:30201. doi: 10.1038/srep30201
McMahan, R. H., Hulsebus, H. J., Najarro, K. M., Giesy, L. E., Frank, D. N., Orlicky, D. J., et al. (2022). Age-related intestinal dysbiosis and enrichment of gut-specific bacteria in the lung are associated with increased susceptibility to Streptococcus pneumoniae infection in mice. Front. Aging 3:859991. doi: 10.3389/fragi.2022.859991
Metersky, M., and Waterer, G. (2020). Can animal models really teach us anything about pneumonia? Con. Eur. Respir. J. 55:1901525. doi: 10.1183/13993003.01525-2019
Mikerov, A. N., Cooper, T. K., Wang, G., Hu, S., Umstead, T. M., Phelps, D. S., et al. (2011). Histopathologic evaluation of lung and extrapulmonary tissues show sex differences in Klebsiella pneumoniae - infected mice under different exposure conditions. Int. J. Physiol. Pathophysiol. Pharmacol. 3, 176–190.
Miller, M. A., Stabenow, J. M., Parvathareddy, J., Wodowski, A. J., Fabrizio, T. P., Bina, X. R., et al. (2012). Visualization of murine intranasal dosing efficiency using luminescent Francisella tularensis: Effect of instillation volume and form of anesthesia. PLoS One 7:e31359. doi: 10.1371/journal.pone.0031359
Mizgerd, J. P., and Skerrett, S. J. (2008). Animal models of human pneumonia. Am. J. Physiol. Lung Cell. Mol. Physiol. 294, L387–L398. doi: 10.1152/ajplung.00330.2007
Monogue, M. L., Sakoulas, G., Nizet, V., and Nicolau, D. P. (2018). Humanized exposures of a β-Lactam-β-lactamase inhibitor, tazobactam, versus non-β-lactam-β-lactamase inhibitor, avibactam, with or without colistin, against Acinetobacter baumannii in murine thigh and lung infection models. Pharmacology 101, 255–261. doi: 10.1159/000486445
Morehead, M. S., and Scarbrough, C. (2018). Emergence of global antibiotic resistance. Prim. Care 45, 467–484. doi: 10.1016/j.pop.2018.05.006
Moser, C., Johansen, H. K., Song, Z., Hougen, H. P., Rygaard, J., and Høiby, N. (1997). Chronic Pseudomonas aeruginosa lung infection is more severe in Th2 responding BALB/c mice compared to Th1 responding C3H/HeN mice. Apmis 105, 838–842. doi: 10.1111/j.1699-0463.1997.tb05092.x
Murray, C. J., Ikuta, K. S., Sharara, F., Swetschinski, L., Robles Aguilar, G., Gray, A., et al. (2022). Global burden of bacterial antimicrobial resistance in 2019: A systematic analysis. Lancet 399, 629–655. doi: 10.1016/s0140-6736(21)02724-0
Nakamura, R., Ito-Horiyama, T., Takemura, M., Toba, S., Matsumoto, S., Ikehara, T., et al. (2019). In vivo pharmacodynamic study of cefiderocol, a novel parenteral siderophore cephalosporin, in murine thigh and lung infection models. Antimicrob. Agents Chemother. 63:e02031-18. doi: 10.1128/AAC.02031-18
Nguyen, N. T. Q., Gras, E., Tran, N. D., Nguyen, N. N. Y., Lam, H. T. H., Weiss, W. J., et al. (2021). Pseudomonas aeruginosa ventilator-associated pneumonia rabbit model for preclinical drug development. Antimicrob. Agents Chemother. 65:e0272420. doi: 10.1128/AAC.02724-20
Nosek, B. A., Alter, G., Banks, G. C., Borsboom, D., Bowman, S. D., Breckler, S. J., et al. (2015). Promoting an open research culture. Science 348, 1422–1425. doi: 10.1126/science.aab2374
Oliver, A., Cantón, R., Campo, P., Baquero, F., and Blázquez, J. (2000). High frequency of hypermutable Pseudomonas aeruginosa in cystic fibrosis lung infection. Science 288, 1251–1253. doi: 10.1126/science.288.5469.1251
Oshima, K., Nakamura, S., Iwanaga, N., Takemoto, K., Miyazaki, T., Yanagihara, K., et al. (2017). Efficacy of high-dose meropenem (six grams per day) in treatment of experimental murine pneumonia induced by meropenem-resistant Pseudomonas aeruginosa. Antimicrob. Agents Chemother. 61:e02056-16. doi: 10.1128/AAC.02056-16
Pachón-Ibáñez, M. E., Docobo-Pérez, F., Jiménez-Mejias, M. E., Ibáñez-Martínez, J., García-Curiel, A., Pichardo, C., et al. (2011). Efficacy of rifampin, in monotherapy and in combinations, in an experimental murine pneumonia model caused by panresistant Acinetobacter baumannii strains. Eur. J. Clin. Microbiol. Infect. Dis. 30, 895–901. doi: 10.1007/s10096-011-1173-6
Parra Millán, R., Jiménez Mejías, M. E., Sánchez Encinales, V., Ayerbe Algaba, R., Gutiérrez Valencia, A., Pachón Ibáñez, M. E., et al. (2016). Efficacy of lysophosphatidylcholine in combination with antimicrobial agents against Acinetobacter baumannii in experimental murine peritoneal sepsis and pneumonia models. Antimicrob. Agents Chemother. 60, 4464–4470. doi: 10.1128/AAC.02708-15
Paterson, D. L., Isler, B., and Stewart, A. (2020). New treatment options for multiresistant gram negatives. Curr. Opin. Infect. Dis. 33, 214–223. doi: 10.1097/QCO.0000000000000627
Peers, I. S., Ceuppens, P. R., and Harbron, C. (2012). In search of preclinical robustness. Nat. Rev. Drug Discov. 11, 733–734. doi: 10.1038/nrd3849
Percie du Sert, N., Hurst, V., Ahluwalia, A., Alam, S., Avey, M. T., Baker, M., et al. (2020). The ARRIVE guidelines 2.0: Updated guidelines for reporting animal research. PLoS Biol. 18:e3000410. doi: 10.1371/journal.pbio.3000410
Pibiri, M., Sulas, P., Leoni, V. P., Perra, A., Kowalik, M. A., Cordella, A., et al. (2015). Global gene expression profile of normal and regenerating liver in young and old mice. Age 37:9796. doi: 10.1007/s11357-015-9796-7
Pires, S., Peignier, A., Seto, J., Smyth, D. S., and Parker, D. (2020). Biological sex influences susceptibility to Acinetobacter baumannii pneumonia in mice. JCI Insight 5:e132223. doi: 10.1172/JCI.INSIGHT.132223
Ren, H., Liu, Y., Zhou, J., Long, Y., Liu, C., Xia, B., et al. (2019). Combination of azithromycin and gentamicin for efficient ereatment of Pseudomonas aeruginosa infections. J. Infect. Dis. 220, 1667–1678. doi: 10.1093/infdis/jiz341
Sakoulas, G., Rose, W., Berti, A., Olson, J., Munguia, J., Nonejuie, P., et al. (2017). Classical β-lactamase inhibitors potentiate the activity of daptomycin against methicillin-resistant Staphylococcus aureus and colistin against Acinetobacter baumannii. Antimicrob. Agents Chemother. 61:e01745-16. doi: 10.1128/AAC.01745-16
Sanderink, D., Cassisa, V., Chenouard, R., Mahieu, R., Kempf, M., Dubée, V., et al. (2019). Colistin-glycopeptide combinations against multidrug-resistant Acinetobacter baumannii in a mouse model of pneumonia. Future Microbiol. 14, 581–586. doi: 10.2217/fmb-2019-0022
Schurr, J. R., Young, E., Byrne, P., Steele, C., Shellito, J. E., and Kolls, J. K. (2005). Central role of toll-like receptor 4 signaling and host defense in experimental pneumonia caused by gram-negative bacteria. Infect. Immun. 73, 532–545. doi: 10.1128/IAI.73.1.532-545.2005
Singh, N., Vats, A., Sharma, A., Arora, A., and Kumar, A. (2017). The development of lower respiratory tract microbiome in mice. Microbiome 5:61. doi: 10.1186/s40168-017-0277-3
Smith, A. J., Clutton, R. E., Lilley, E., Hansen, K. E. A., and Brattelid, T. (2018). PREPARE: Guidelines for planning animal research and testing. Lab. Anim. 52, 135–141. doi: 10.1177/0023677217724823
Smith, C. A., Kulkarni, U., Chen, J., and Goldstein, D. R. (2019). Influenza virus inoculum volume is critical to elucidate age-dependent mortality in mice. Aging Cell 18:e12893. doi: 10.1111/acel.12893
Soldin, O. P., and Mattison, D. R. (2009). Sex differences in pharmacokinetics and pharmacodynamics. Clin. Pharmacokinet. 48, 143–157.
Stackowicz, J., Jönsson, F., and Reber, L. L. (2020). Mouse models and tools for the in vivo study of neutrophils. Front. Immunol. 10:3130. doi: 10.3389/fimmu.2019.03130
Tan, S., Gao, J., Li, Q., Guo, T., Dong, X., Bai, X., et al. (2020). Synergistic effect of chlorogenic acid and levofloxacin against Klebsiella pneumoniae infection in vitro and in vivo. Sci. Rep. 10:20013. doi: 10.1038/s41598-020-76895-5
Tang, H. J., Chuang, Y. C., Ko, W. C., Chen, C. C., Shieh, J. M., Chen, C. H., et al. (2012). Comparative evaluation of intratracheal colistimethate sodium, imipenem, and meropenem in BALB/c mice with carbapenem-resistant Acinetobacter baumannii pneumonia. Int. J. Infect. Dis. 16, e34–e40. doi: 10.1016/j.ijid.2011.09.015
Tängdén, T., Lundberg, C. V., Friberg, L. E., and Huttner, A. (2020). How preclinical infection models help define antibiotic doses in the clinic. Int. J. Antimicrob. Agents 56:106008. doi: 10.1016/j.ijantimicag.2020.106008
Thabit, A. K., Crandon, J. L., and Nicolau, D. P. (2016). Pharmacodynamic and pharmacokinetic profiling of delafloxacin in a murine lung model against community-acquired respiratory tract pathogens. Int. J. Antimicrob. Agents 48, 535–541. doi: 10.1016/j.ijantimicag.2016.08.012
Theuretzbacher, U., Outterson, K., Engel, A., and Karlén, A. (2020). The global preclinical antibacterial pipeline. Nat. Rev. Microbiol. 18, 275–285. doi: 10.1038/s41579-019-0288-0
Tuttle, A. H., Philip, V. M., Chesler, E. J., and Mogil, J. S. (2018). Comparing phenotypic variation between inbred and outbred mice. Nat. Methods 15, 994–996. doi: 10.1038/s41592-018-0224-7
Van’t Wout, J. W., Linde, I., Leijh, P. C. J., and Van Furth, R. (1989). Effect of irradiation, cyclophospahmide, and etoposide (VP-16) on number of peripheral blood and peritoneal leukocytes in mice under normal conditions and during acute inflammatory reaction. Inflammation 13, 1–14. doi: 10.1007/BF00918959
Vidaillac, C., Yong, V. F. L., Aschtgen, M.-S., Qu, J., Yang, S., Xu, G., et al. (2020). Sex steroids induce membrane stress responses and virulence properties in Pseudomonas aeruginosa. mBio 11:e01774-20. doi: 10.1128/mbio.01774-20
Visweswaraiah, A., Novotny, L. A., Hjemdahl-Monsen, E. J., Bakaletz, L. O., and Thanavala, Y. (2002). Tracking the tissue distribution of marker dye following intranasal delivery in mice and chinchillas: A multifactorial analysis of parameters affecting nasal retention. Vaccine 20, 3209–3220. doi: 10.1016/S0264-410X(02)00247-5
Waack, U., Weinstein, E. A., and Farley, J. J. (2020). Assessing animal models of bacterial pneumonia used in investigational new drug applications for the treatment of bacterial pneumonia. Antimicrob. Agents Chemother. 64:e02242-19. doi: 10.1128/AAC.02242-19
Wang, H., Wu, H., Ciofu, O., Song, Z., and Høibya, N. (2012). In vivo pharmacokinetics/pharmacodynamics of colistin and imipenem in Pseudomonas aeruginosa biofilm infection. Antimicrob. Agents Chemother. 56, 2683–2690. doi: 10.1128/AAC.06486-11
World Health Organization [WHO] (2017). Global Priority List of Antibiotic-Resistant Bacteria to Guide Research, Discovery, and Development of New Antibiotics. Geneva: WHO, 1–7.
Yamada, K., Yamamoto, Y., Yanagihara, K., Araki, N., Harada, Y., Morinaga, Y., et al. (2012). In vivo efficacy and pharmacokinetics of biapenem in a murine model of ventilator-associated pneumonia with Pseudomonas aeruginosa. J. Infect. Chemother. 18, 472–478. doi: 10.1007/s10156-011-0359-2
Yamada, K., Yanagihara, K., Kaku, N., Harada, Y., Migiyama, Y., Nagaoka, K., et al. (2013a). Azithromycin attenuates lung inflammation in a mouse model of ventilator-associated pneumonia by multidrug-resistant Acinetobacter baumannii. Antimicrob. Agents Chemother. 57, 3883–3888. doi: 10.1128/AAC.00457-13
Yamada, K., Yanagihara, K., Kaku, N., Harada, Y., Migiyama, Y., Nagaoka, K., et al. (2013b). In vivo efficacy of biapenem with ME1071, a novel metallo-β-lactamase (MBL) inhibitor, in a murine model mimicking ventilator-associated pneumonia caused by MBL-producing Pseudomonas aeruginosa. Int. J. Antimicrob. Agents 42, 238–243. doi: 10.1016/j.ijantimicag.2013.05.016
Yang, Y. S., Lee, Y., Tseng, K. C., Huang, W. C., Chuang, M. F., Kuo, S. C., et al. (2016). In vivo and in vitro efficacy of minocycline-based combination therapy for minocycline-resistant Acinetobacter baumannii. Antimicrob. Agents Chemother. 60, 4047–4054. doi: 10.1128/AAC.02994-15
Yokoyama, Y., Matsumoto, K., Ikawa, K., Watanabe, E., Shigemi, A., Umezaki, Y., et al. (2014). Pharmacokinetic/pharmacodynamic evaluation of sulbactam against Acinetobacter baumannii in in vitro and murine thigh and lung infection models. Int. J. Antimicrob. Agents 43, 547–552. doi: 10.1016/j.ijantimicag.2014.02.012
Zak, O., and O’Reilly, T. (1991). Animal models in the evaluation of antimicrobial agents. Antimicrob. Agents Chemother. 35, 1527–1531. doi: 10.1128/AAC.35.8.1527
Zhao, M., Lepak, A. J., and Andes, D. R. (2016). Animal models in the pharmacokinetic/pharmacodynamic evaluation of antimicrobial agents. Bioorg. Med. Chem. 24, 6390–6400. doi: 10.1016/j.bmc.2016.11.008
Zhao, M., Lepak, A. J., Marchillo, K., and Andesa, D. R. (2019). In vivo pharmacodynamic target determination for delafloxacin against Klebsiella pneumoniae and Pseudomonas aeruginosa in the neutropenic murine pneumonia model. Antimicrob. Agents Chemother. 63:e01131-19. doi: 10.1128/AAC.01131-19
Zhou, J., Ledesma, K. R., Chang, K. T., Abodakpi, H., Gao, S., and Tama, V. H. (2017). Pharmacokinetics and pharmacodynamics of minocycline against Acinetobacter baumannii in a neutropenic murine pneumonia model. Antimicrob. Agents Chemother. 61:e02371-16. doi: 10.1128/AAC.02371-16
Zhou, Y. F., Tao, M. T., Huo, W., Liao, X. P., Sun, J., and Liu, Y. H. (2017). In vivo pharmacokinetic and pharmacodynamic profiles of antofloxacin against Klebsiella pneumoniae in a neutropenic murine lung infection model. Antimicrob. Agents Chemother. 61:e02691-16. doi: 10.1128/AAC.02691-16
Zuluaga, A. F., Salazar, B. E., Rodriguez, C. A., Zapata, A. X., Agudelo, M., and Vesga, O. (2006). Neutropenia induced in outbred mice by a simplified low-dose cyclophosphamide regimen: Characterization and applicability to diverse experimental models of infectious diseases. BMC Infect. Dis. 6:55. doi: 10.1186/1471-2334-6-55
Keywords: murine pneumonia model, antimicrobial, lung infection, Gram-negative, PK/PD, antimicrobial efficacy studies
Citation: Arrazuria R, Kerscher B, Huber KE, Hoover JL, Lundberg CV, Hansen JU, Sordello S, Renard S, Aranzana-Climent V, Hughes D, Gribbon P, Friberg LE and Bekeredjian-Ding I (2022) Variability of murine bacterial pneumonia models used to evaluate antimicrobial agents. Front. Microbiol. 13:988728. doi: 10.3389/fmicb.2022.988728
Received: 07 July 2022; Accepted: 15 August 2022;
Published: 08 September 2022.
Edited by:
Rustam Aminov, University of Aberdeen, United KingdomReviewed by:
Juan Carlos Vázquez-Ucha, Institute of Biomedical Research of A Coruña (INIBIC), SpainCopyright © 2022 Arrazuria, Kerscher, Huber, Hoover, Lundberg, Hansen, Sordello, Renard, Aranzana-Climent, Hughes, Gribbon, Friberg and Bekeredjian-Ding. This is an open-access article distributed under the terms of the Creative Commons Attribution License (CC BY). The use, distribution or reproduction in other forums is permitted, provided the original author(s) and the copyright owner(s) are credited and that the original publication in this journal is cited, in accordance with accepted academic practice. No use, distribution or reproduction is permitted which does not comply with these terms.
*Correspondence: Isabelle Bekeredjian-Ding, aXNhYmVsbGUuYmVrZXJlZGppYW4tZGluZ0BwZWkuZGU=
Disclaimer: All claims expressed in this article are solely those of the authors and do not necessarily represent those of their affiliated organizations, or those of the publisher, the editors and the reviewers. Any product that may be evaluated in this article or claim that may be made by its manufacturer is not guaranteed or endorsed by the publisher.
Research integrity at Frontiers
Learn more about the work of our research integrity team to safeguard the quality of each article we publish.