- 1Key Laboratory of (Guangxi) Agricultural Environment and Products Safety, College of Agronomy, Guangxi University, Nanning, China
- 2Chongqing Key Laboratory of Soil Multiscale Interfacial Process, Southwest University, Chongqing, China
- 3Guangxi Bossco Environmental Protection Technology Co., Ltd., Nanning, China
It is unknown whether nirBDs, which conventionally encode an NADH nitrite reductase, play other novel roles in nitrogen cycling. In this study, we explored the role of nirBDs in the nitrogen cycling of Pseudomonas putida Y-9. nirBDs had no effect on organic nitrogen transformation by strain Y-9. The △nirBD strain exhibited higher ammonium removal efficiency (90.7%) than the wild-type strain (76.1%; P < 0.05) and lower end gaseous nitrogen (N2O) production. Moreover, the expression of glnA (control of the ammonium assimilation) in the △nirBD strain was higher than that in the wild-type strain (P < 0.05) after being cultured in ammonium-containing medium. Furthermore, nitrite noticeably inhibited the ammonium elimination of the wild-type strain, with a corresponding removal rate decreasing to 44.8%. However, no similar impact on ammonium transformation was observed for the △nirBD strain, with removal efficiency reaching 97.5%. In conclusion, nirBDs in strain Y-9 decreased the ammonium assimilation and increased the ammonium oxidation to nitrous oxide.
Introduction
Microorganisms show multiple nitrogen transformation pathways, contributing to the natural nitrogen-cycling balance (Canfield et al., 2010; Kuypers et al., 2018). Ammonium (NH4+) is the preferred nitrogen source for most bacteria and archaea (Burkovski, 2003; Muro-Pastor et al., 2005). Ammonium assimilation by microorganisms leads to high ammonium removal efficiency and mitigation of harmful effects on the environment. However, the role of ammonium assimilation in NH4+ removal is often neglected.
Simultaneous nitrification and denitrification (SND) has been widely applied in wastewater treatment plants as an attractive biological approach to nitrogen removal, owing to its low investment costs and high efficiency (Jin et al., 2015; Lei et al., 2016). Numerous studies have focused on isolating, identifying, and characterizing nitrogen removal associated with SND strains (Jin et al., 2015; Chen et al., 2016, 2021; Lei et al., 2019). Usually, the SND pathway by bacteria is NH4+→NH2OH→NO2–→NO3–→NO2–→NO→N2O →N2. Ammonia monooxygenase catalyzes NH4+ oxidization to NH2OH and is encoded by amoA, amoB, or amoC (Kuypers et al., 2018). Nitrate reductase encoded by narG, narH, napA, or nasA can catalyze NO3– reduction to NO2–. Nitrite reductase catalyzing NO2– reduction to NO or NH4+ is encoded by nirK, nirS, nirB, or nirD (Kuypers et al., 2018; Yang L. et al., 2019; Xia et al., 2020; Zhang et al., 2020).
nirBDs, which conventionally encode an NADH nitrite reductase in many bacteria (Jackson et al., 1981; Lin and Stewart, 1997; Malm et al., 2009), show different characteristics under different conditions. For example, dissimilatory nitrite reductase encoded by nirBDs in Escherichia coli and other enterobacteria is only expressed under anaerobic conditions (Macdonald et al., 1985; Gennis and Stewart, 1996), while nirBDs in Streptomyces coelicolor only encode the assimilatory nitrite reductase under aerobic conditions (Tiffert et al., 2008; Fischer et al., 2012). Recent studies have shown that nirBDs in S. coelicolor also play an integral role in the nitric oxide (NO) homeostatic regulation system that eliminates nitrite (NO2–) from cultures during NO3– reduction (Yukioka et al., 2017).
We previously observed that the SND strain Pseudomonas putida Y-9 exhibited excellent removal ability for NH4+ and NO3– (Xu et al., 2017). Further studies clarified that strain Y-9 could transform NH4+ into nitrous oxide (N2O) under aerobic conditions (Huang et al., 2019) and remove NH4+ mainly through assimilation (Huang et al., 2021a). In addition, strain Y-9 can remove NO3– via simultaneous nitrate assimilation, dissimilatory nitrate reduction to ammonium (DNRA), and denitrification under aerobic conditions. Within these contexts, the enzyme encoded by nirBDs catalyzes NO2– reduction to NH4+ during assimilation and DNRA (Huang et al., 2020). We hypothesized that nirBDs in strain Y-9 might be functional in other roles than encoding the traditional nitrite reductase. In this study, the effects of nirBDs on different nitrogen transformation pathways in strain Y-9 were explored. Results revealed that knocking out nirBDs promoted ammonium assimilation and weakened the emission of nitrous oxide. These findings provide a new understanding on how to use strain Y-9 for the treatment of ammonium nitrogen polluted water.
Materials and methods
Strain and culture media
The SND bacterium P. putida Y-9 (GenBank No. KP410740) used here was obtained from our previous study (Xu et al., 2017). nirBDs were knocked out from the genome of strain Y-9 using homologous recombination technology, as previously described (Huang et al., 2020), mediated via plasmid pLP12. The primer sequences for nirBDs knockout are shown in Supplementary Table 1. Supplementary Figure 1 shows the successful construction of the nirBD deletion mutants.
Lysogeny broth (LB) liquid medium consisting of (per liter) 10.0 g Tryptone, 5.00 g Yeast extract, and 10.0 g NaCl (pH adjusted to 7.0–7.2) was used for strain enrichment.
Nitrification medium (NM) comprised (per liter) 7.00 g K2HPO4, 3.00 g KH2PO4, 0.10 g MgSO4⋅7H2O, 0.50 g (NH4)2SO4, 0.05 g FeSO4⋅7H2O, and 5.13 g CH3COONa (pH adjusted to 7.2). NM was used to determine the ammonium transformation characteristics of strain Y-9.
The composition of the denitrification medium (DM) was (per liter) 7.00 g K2HPO4, 3.00 g KH2PO4, 0.10 g MgSO4⋅7H2O, 0.72 g KNO3 (DM-1) or 0.49 g NaNO2 (DM-2), 0.05 g FeSO4⋅7H2O, and 5.13 g CH3COONa (pH adjusted to 7.2). The two DM formulae were used to evaluate the nitrate or nitrite transformation ability of strain Y-9.
The organic nitrogen medium (OM) was composed of (per liter, pH 7.2) 7.00 g K2HPO4, 3.00 g KH2PO4, 0.10 g MgSO4⋅7H2O, 0.79 g tryptone, 0.05 g FeSO4⋅7H2O, and 0.788 g peptone (pH adjusted to 7.2). OM was used to evaluate the organic nitrogen conversion ability of strain Y-9.
The SND medium contained 7.00 g K2HPO4, 3.00 g KH2PO4, 0.10 g MgSO4⋅7H2O, 0.50 g (NH4)2SO4, 0.72 g KNO3 (SND-1) or 0.49 g NaNO2 (SND-2), 0.05 g FeSO4⋅7H2O, and 10.3 g CH3COONa (pH adjusted to 7.2). Two types of SND media were used to assess the nitrogen transformation ability of strain Y-9 with ammonium and either nitrate or nitrite.
Solid plates were prepared using 2.0% (w/v) agar added into the above liquid media. Before use, all of the above media were autoclaved for 30 min at 0.11 MPa and 121°C.
Estimation of the role of nirBDs in nitrogen transformation by strain Y-9
Single colonies of the Y-9 and △nirBD strains were enriched for 36 h using LB liquid medium. Preculture (8 mL) was harvested and washed twice with sterile pure water by centrifugation (4,000 rpm, 8 min), inoculated into 100 mL of NM, DM-1, DM-2, OM, SND-1, or SND-2, and then cultivated at 15°C with shaking at 150 rpm. No strains were added for control treatments. Three replicates were performed for each experiment. Culture samples in different nitrogen media were taken out to measure the optical density of the strain (OD600) and different types of nitrogen using a spectrophotometer (Huang et al., 2019).
Detection of N2O and N2 after Y-9 and △nirBD strains cultured in ammonium medium
The precultures of the Y-9 and the △nirBD strains were inoculated into media containing (15NH4)2SO4 (10 atom%) in 250 mL serum bottles. Then, the serum bottles were sealed with a rubber septum, aerated with oxygen, and incubated at 15°C for 48 h with shaking at 150 rpm. Finally, the 15N2O and 15N2 present in the headspace were collected with a needle and detected using GC-MS (Agilent, USA) and GC-IRMS (Thermo Fisher Scientific, USA), respectively (Ai et al., 2011; Ye et al., 2016; Huang et al., 2019).
Monitoring the expression of glnA
DNA fragments of the Y-9 and △nirBD strains containing upstream regions of glnA were PCR-amplified and ligated into the upstream region of the promoter-less lacZ in pRG970Km (Table 1) to generate the reporter plasmids p970 Km-glnA. Then, the Y-9 and △nirBD strains containing the reporter plasmids were cultured in NM medium with shaking at 150 rpm, and aliquots were collected after 2 days of incubation. The activity of β-galactosidase was measured as described by Miller (1972). Relative expression of glutamine synthetase encoded by glnA was represented by OD420/OD600 (Xu et al., 2018). Moreover, total RNA of Y-9 and △nirBD strains incubated in NM medium for 48 h was extracted and converted to cDNA to investigate the relative expression of glnA using qPCR. The primers of glnA were GACCACGAAATCCGTACTGC and TTTCAGGGCCTGTACTTCGT. The 16S rRNA gene was used as an internal standard, and its primers were GTGCCAGCMGCCGCGG (515F) and CCGTCAATTCMTTTRAGTTT (907R). The PCR cycling conditions were as follows: initial denaturation at 95°C for 30 s; 38 cycles at 95°C for 15 s, 60°C for 30 s, and 72°C for 30 s; 1 cycle of 95°C for 15 s; and finally, stepwise temperature increases from 55 to 95°C to generate the melting curve. Standard curves were established using a dilution series of pMD19-T vectors containing the target gene (Huang et al., 2021b).
Analytical methods
Total nitrogen (TN, including cells) content in the suspension was estimated using the alkaline potassium persulfate digestion-UV spectrophotometric method. The contents of NH4+, NO2–, and NO3– in the supernatant were quantified using the indophenol blue method, the hydrochloric acid photometry method, and the N-(1-naphthalene)-diaminoethane spectrophotometry method, respectively, after samples were centrifuged at 8,000 rpm for 5 min. The above analyses were carried out according to the guidelines set by the State Environmental Protection Administration of China (2002). The decline rate of nitrogen (TN, NH4+, NO2–, and NO3–) was calculated using the equation: Rv = (T1-T2)/T1 × 100%, where Rv represents the nitrogen decrease efficiency, and T1 and T2 are the original and eventual contents of nitrogen in the system (mg L–1). Culture pH was measured by a pH meter.
The SPSS Statistics program (version 22) and Microsoft Excel 2010 were used for statistical analysis, and Origin 8.6 was used to produce the graphics.
Results and discussion
Impact of nirBDs on organic nitrogen transformation by strain Y-9
Both the wild-type and △nirBD strains grew vigorously in the OM and did not reach the stationary phase until 4 days (Figure 1), consistent with data from Fischer et al. (2012), who reported that strain S. coelicolor A3(2) (△nirBD) grew well on the plate containing casamino acids. The TN concentrations in the △nirBD and wild-type strain culture systems decreased gradually throughout the experiments and finally only dropped by 18.0 mg L–1 without NO2– accumulation. This phenomenon suggested that strain Y-9 preferred to utilize organic nitrogen for cellular growth rather than converted it into gaseous nitrogen. Besides, variations in all of the measured nitrogen species within the culture medium of the wild-type and △nirBD strains were consistent across the entire incubation period. These results indicated that knocking out nirBDs did not affect organic nitrogen transformation by strain Y-9.
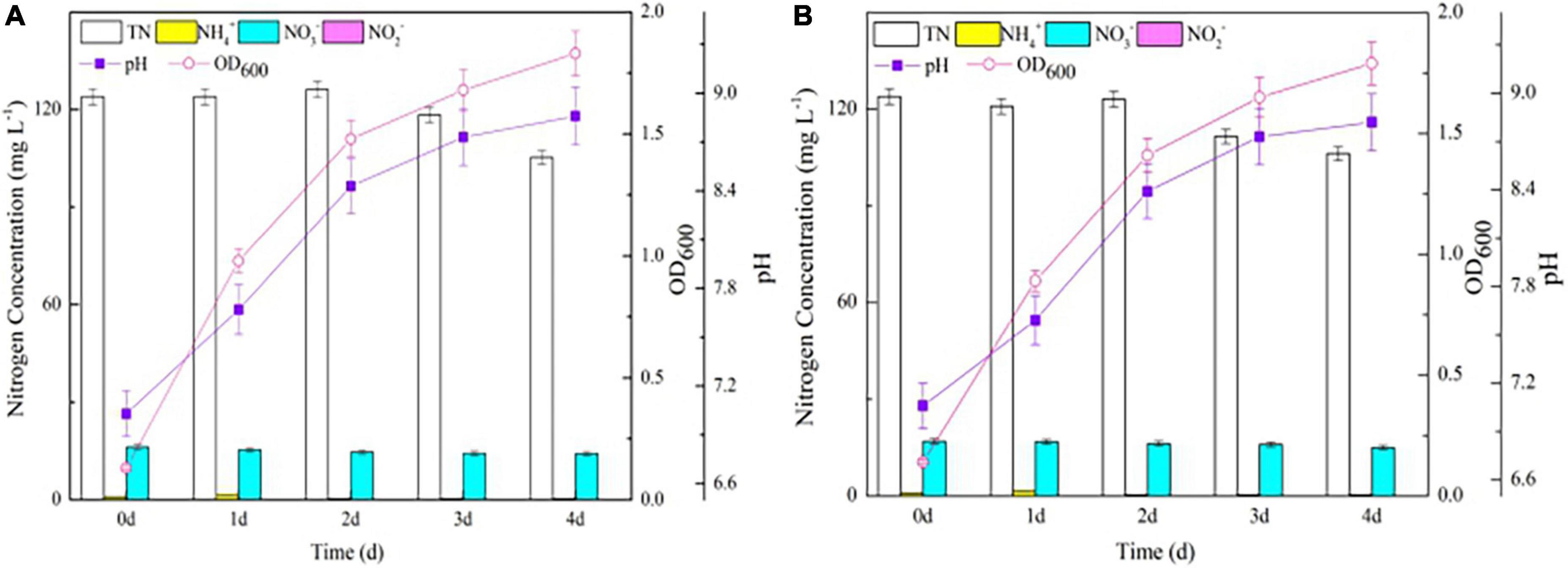
Figure 1. The growth curve and nitrogen transformation performance of strain Y-9 in organic nitrogen medium at 15°C. (A) The wild-type strain Y-9. (B) The △nirBD strain Y-9.
Impact of nirBDs on ammonium transformation by strain Y-9
To explore the effects of nirBD on the ammonium transformation process, the Y-9 and △nirBD strains were cultured in NM. Both strains grew vigorously (Figure 2), consistent with a previous study (Fischer et al., 2012), with the △nirBD strain of S. coelicolor growing similar to the wild-type strain on glucose minimal medium agar plates supplemented with NH4+ as the nitrogen source. These results illustrated that nirBD was not essential for the utilization of ammonium by strain Y-9. Intriguingly, the NH4+ removal efficiency by △nirBD strain (90.7%) was higher than that by the wild-type strain (76.1%) after 2 days of incubation (P < 0.05), which might be attributed to the stronger assimilation or ammonium oxidation ability of the △nirBD strain compared to the wild-type strain (Li et al., 2017; Jin et al., 2019).
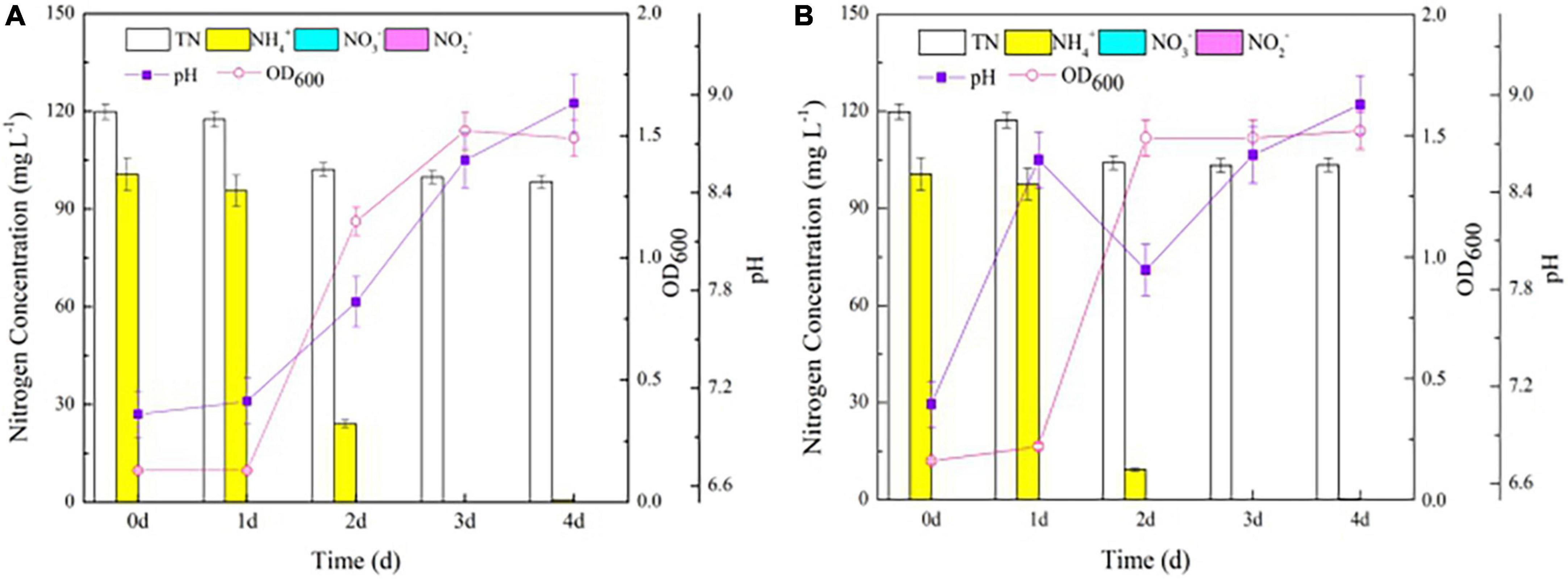
Figure 2. The growth curve and nitrogen transformation performance of strain Y-9 in nitrification medium at 15°C. (A) The wild-type strain Y-9. (B) The △nirBD strain Y-9.
The gas produced during the NH4+ removal process was N2O and not N2, according to the results of the GC test, consistent with our previous studies (Huang et al., 2019). Moreover, the δ15N/14N ratio of N2O in the △nirBD strain culture system (2.46) was lower than that in the wild-type strain culture system (3.63). Similarly, the decrease in TN in the △nirBD strain culture system (15.7 mg L–1) was lower than that in the wild-type strain culture system (21.5 mg L–1; P < 0.05; Figure 2). These results illustrated that knocking out nirBDs reduced the production of N2O, suggesting that the knockout accelerated ammonium assimilation instead of the ammonium oxidation by strain Y-9.
Previous studies have proven that the glutamine synthetase encoded by glnA gene plays an important role in the ammonium assimilation process (Gupta et al., 2012; Van Heeswijk et al., 2013). In this study, glnA was found in strain Y-9 according to the results of the genome-wide scan. Considering that knocking out the nirBDs accelerated ammonium assimilation by strain Y-9, we speculated that the expression of nirBDs might influence the expression of glnA. Thus, the expression of glnA in Y-9 and △nirBD strains was further detected. β-Galactosidase was utilized as a reporter to examine glnA promoter activity. The results showed that the β-galactosidase activity in the △nirBD strain was obviously higher than that in the wild-type strain (P < 0.05; Figure 3A). Moreover, qPCR results showed that the expression of glnA in the △nirBD strain was higher than that in wild-type strain Y-9 (Figure 3B). These findings suggested that knocking out nirBDs would promote the expression of glnA, accelerating ammonium assimilation.
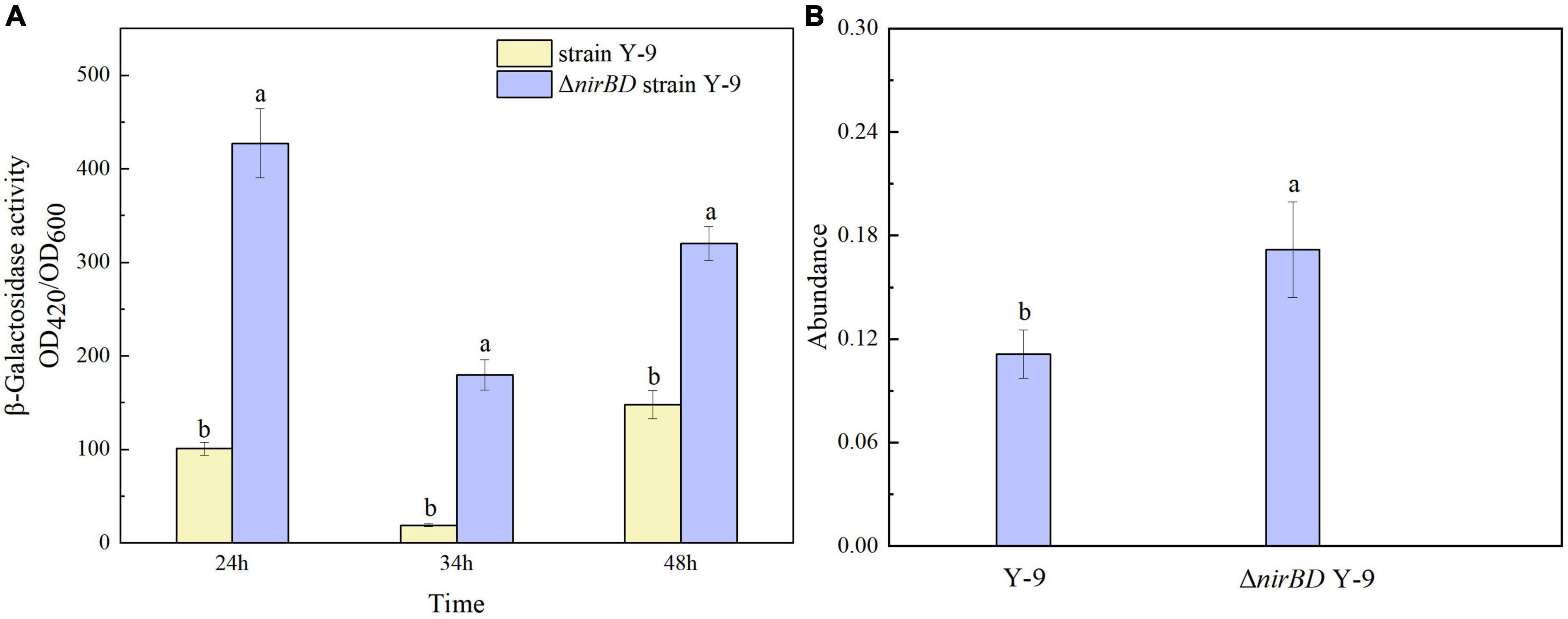
Figure 3. GlnA expression in strain Y-9 and △nirBD strain Y-9. (A) β-Galactosidase activity. (B) Relative expression abundance of glnA. The different lowercase letters above the bars indicate significant differences among treatments (P < 0.05).
Impact of nirBDs on nitrate transformation by strain Y-9
The OD600 of the wild-type strain increased significantly from 0.17 to 1.23, while the △nirBD strain exhibited a slower growth trend when culturing in SND-1 medium (P < 0.05; Figures 4A,B). These results are consistent with those observed when the two strains grew on agar plates supplemented with NO3– as the sole nitrogen source but differed from those when strain NM7 (△nirBD) failed to grow under similar conditions (Fischer et al., 2012). The NirBD protein in strain Y-9 was previously shown to catalyze NO2– reduction to NH4+ (Huang et al., 2020). Accordingly, a little amount of NO2– was detected in our experiments, while NH4+ gradually increased during the cultivation of the wild type strain Y-9 (Figure 4A). In contrast, the accumulation of NO2– was nearly equivalent to the decrease in NO3–, but NH4+ was undetectable during the entire NO3– transformation process of the △nirBD strain (Figure 4B). These findings suggest that NO2– converted from NO3– could not be further reduced to NH4+ by strain Y-9 when nirBDs was knocked out, resulting in NO2– accumulation in the cultures.
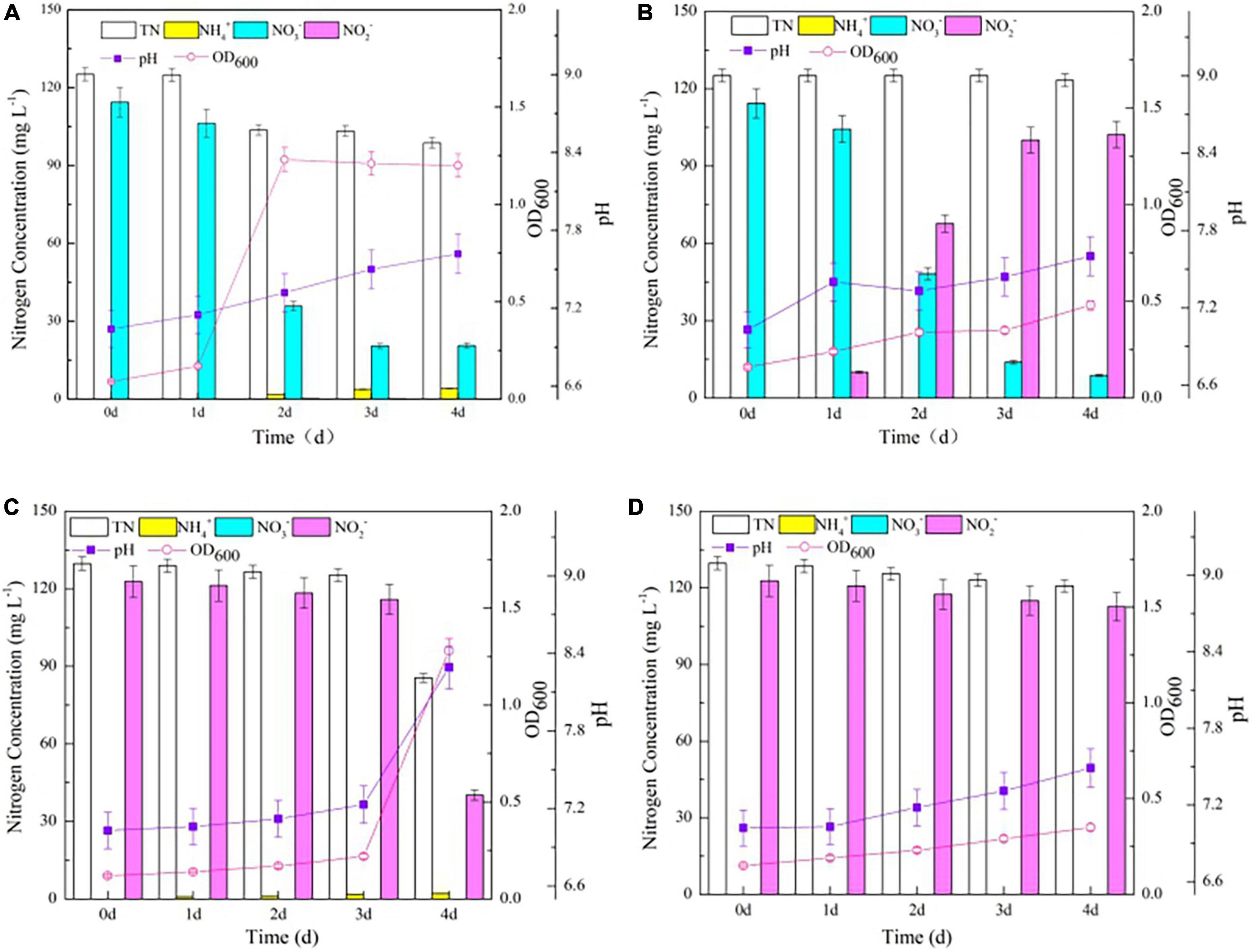
Figure 4. The growth curve and nitrogen transformation performance of strain Y-9 in denitrification medium at 15°C. (A) The wild-type strain Y-9 in DM-1. (B) The △nirBD strain Y-9 in DM-1. (C) The wild-type strain Y-9 in DM-2. (D) The △nirBD strain Y-9 in DM-2.
After cultivating the wild-type strain for 4 days, the decrease in NO3– and TN reached 93.7 mg L–1 and 26.4 mg L–1, respectively. Moreover, culture pH increased over the whole cultivation period for the wild-type strain (Figure 4A). These dynamics were due to a small amount of NO3– being removed by strain Y-9 via weak denitrification (Huang et al., 2020). The △nirBD strain achieved a total NO3– reduction of 105.6 mg L–1, while TN was barely diminished after 4 d of incubation (Figure 4B). This finding could be because the knocking out of nirBDs resulted in NO2–1 accumulation in the medium, finally inhibiting the growth of strain Y-9 and its TN degrading ability.
Impact of nirBDs on nitrite transformation by strain Y-9
Growth of the wild-type and △nirBD strains increased slowly during the initial 3 days of cultivation in the NO2– -containing medium (Figures 4C,D). The probable reason for the slow growth was the high content of free nitrous acid (FNA, > 0.021 mg HNO2-N L–1 at 3 days) released due to the NO2– inhibition in strain metabolism (Vadivelu et al., 2006). The wild-type strain grew quickly, with a concomitant considerable reduction of NO2– and TN between days 3 and 4. After cultivation, the decrease in TN in suspension (44.2 mg L–1) was lower than the reduced amount of NO2– in the supernatant (82.6 mg L–1; P < 0.05). Thus, some amount of NO2– (44.2 mg L–1) might have been lost from the system through denitrification, while the remainder (38.4 mg L–1) could have been assimilated by the wild-type strain Y-9. NO2– has well-documented toxicity to bacterial cells (Zemke et al., 2017), and strain Y-9 cannot directly absorb NO2–. Moreover, NH4+ accumulation was tracked throughout the NO2– transformation process (Figure 4C). Therefore, it is possible that most of the NO2– that had not been removed through denitrification could have been reduced to NH4+ through assimilation and DNRA by the wild-type strain Y-9, in accordance with our previous results (Huang et al., 2020). The △nirBD strain still grew at a slow rate after 3 days and could seldom remove NO2–. After 4 days of cultivation, NO2– in the supernatant only decreased by 10.0 mg L–1, which was nearly equal to the decreased TN levels in the culture suspension (8.8 mg L–1), indicating that the △nirBD strain also conducted weak denitrification. Additionally, NH4+ was undetectable throughout the cultivation of the △nirBD strain (Figure 4D). Taken together, these results show that knocking out nirBDs does not allow noxious NO2– to be reduced to NH4+, thereby inhibiting cellular growth and denitrification ability.
Influence of nirBDs on nitrogen transformation of strain Y-9 in SND-1 medium
Knocking out nirBDs accelerated the assimilation of NH4+ by strain Y-9 (Figure 2) and led to the near complete conversion of NO3– into NO2– while inhibiting the transformation of NO2– (Figures 4B,D). We further evaluated the impact of nirBDs on nitrogen transformation when NH4+ and NO3– coexisted in the medium. The wild-type and △nirBD strains grew vigorously after a 1-day lag phase and reached the stationary phase on days 3 and 2, respectively (Figures 5A,B). Concomitantly, the transformation of NH4+ by the △nirBD strain was faster than that of the wild-type strain (P < 0.05), consistent with the results when using NH4+ as the sole nitrogen source (Figure 2). These results were attributed to nirBD knockout that accelerated the assimilation of NH4+. The final NH4+ removal efficiency by the wild-type and △nirBD strains was 92.13 and 95.87%, respectively, which were both slightly lower than the NH4+ removal efficiency when incubating the two strains in NM (both approximately 100%) (Figure 2). Consequently, the existence of NO3– led to little inhibition of NH4+ transformation. Moreover, the contents of TN in suspension both dropped down by approximately 20 mg L–1 in the two systems containing the wild type or △nirBD strain, consistent with the decrease in TN in suspension when NH4+ was used as the sole nitrogen source (Figure 2). Thus, no denitrification occurred in strain Y-9 when NH4+ and NO3– coexisted in the system.
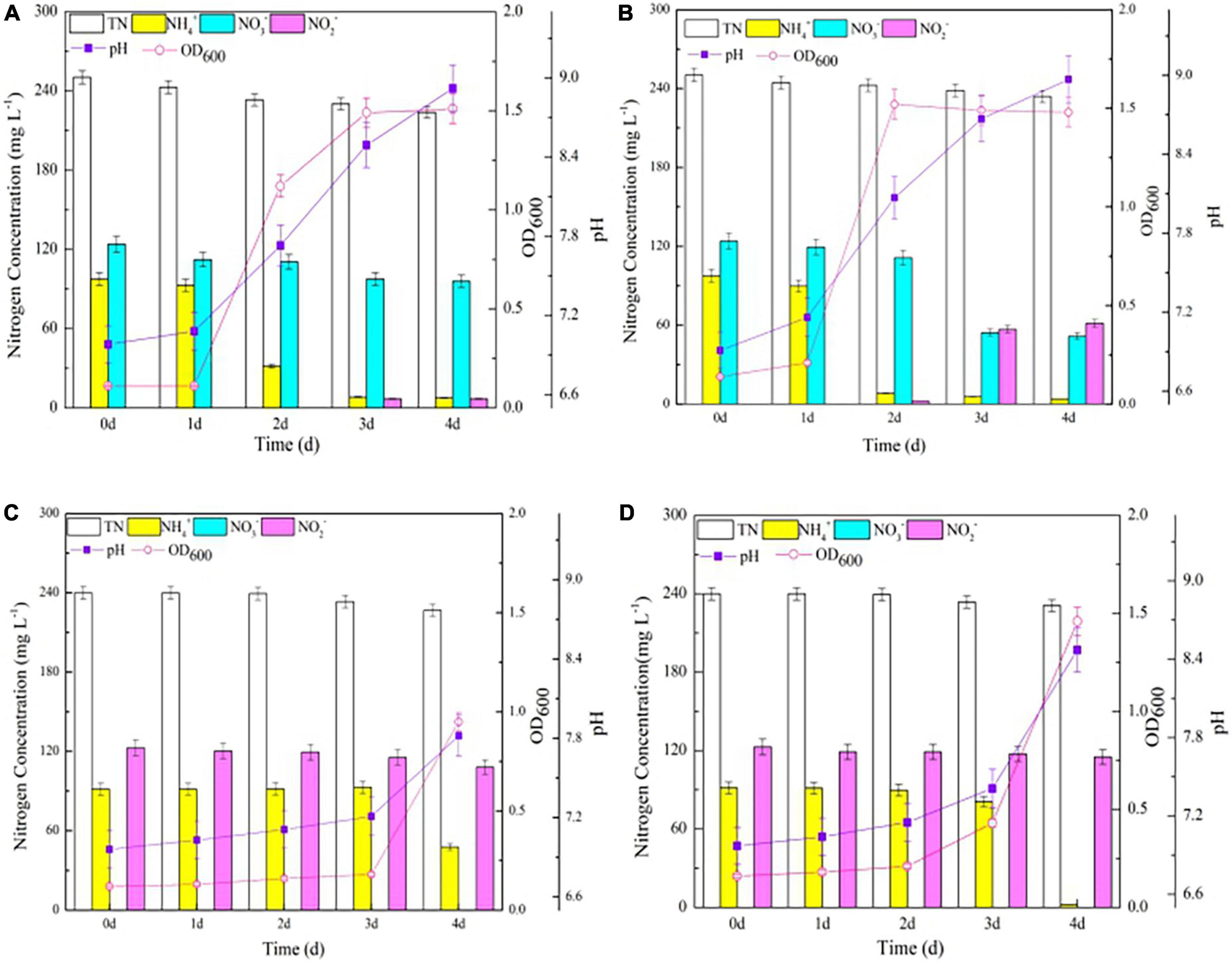
Figure 5. The growth curve and nitrogen transformation performance of strain Y-9 in simultaneous nitrification and denitrification medium. (A) The wild-type strain Y-9 in SND-1. (B) The △nirBD strain Y-9 in SND-1. (C) The wild-type strain Y-9 in SND-2. (D) The △nirBD strain Y-9 in SND-2).
The decrease in NO3– content in suspension reached only 26.4 mg L–1, and the NO2– was undetected after 4 days of wild type strain cultivation, indicating that strain Y-9 utilized NH4+ preferentially when NH4+ and NO3– coexisted in the medium. Similar results were observed by Xu et al. (2017), who used 200 mg L–1 of NH4+ and NO3– to cultivate strain Y-9. Yang J. R. et al. (2019) and Zhang et al. (2022) also reported that Acinetobacter sp. JR1 and P. taiwanensis EN-F2 preferred to remove NH4+ from a medium containing both NH4+ and NO3–. Nevertheless, the contents of NO3– in the △nirBD strain cultures dropped by 72.3 mg L–1 and the accumulation of NO2– reached 61.5 mg L–1 at the end of the experiment (Figure 5B). The above results combined with variation in NO3– and NO2– concentrations when cultivating the △nirBD strain in DM-1 medium (Figure 4B) suggested that nirBDs reduced the NO2– resulting from NO3– respiration to NH4+, and the denitrification ability of the △nirBD strain was weak. In addition, when using NH4+ or NO3– as the sole nitrogen source, the pH increased over the entire incubation process of the wild-type strain Y-9 but fluctuated during △nirBD strain growth (Figures 2, 4A,B). Intriguingly, the pH increased during the entire cultivation period of the wild-type and △nirBD strains when NH4+ and NO3– were both available (Figures 5A,B). Thus, the coexistence of NH4+ and NO3– might counteract the effects of nirBDs knockout with regard to culture pH. Nevertheless, these dynamics require further investigation.
Influence of nirBDs on the nitrogen transformation of strain Y-9 in the SND-2 medium
When NH4+ and NO2– coexisted in the medium, the wild-type and △nirBD strains both barely grew within the first 2 days. Nevertheless, the △nirBD strain exhibited higher growth than the wild type strain over the entire cultivation period (P < 0.05; Figures 5C,D), owing to the acceleration of NH4+ assimilation by strain Y-9, due to nirBDs knockout (Figure 2). The NH4+ removal rate by the △nirBD strain (97.5%) was considerably higher than that of the wild-type strain (47.7%), but the decrease in TN in the △nirBD strain culture system (9.1 mg L–1) was lower than that in the wild-type strain culture system (13.2 mg L–1; P < 0.05). This finding could be attributed to the denitrification that was inhibited when nirBDs were knocked out (Figures 4A,B). The decrease in NO2– in the wild-type strain culture system was consistently greater than that of the △nirBD strain culture system over the entire incubation period (P < 0.05), which might have been due to the stronger denitrification rate of the wild-type strain compared to the △nirBD strain. However, the high concentration of NO2– in the system could still inhibit the utilization of NH4+ by the wild-type strain, with residual NH4+ levels reaching 47.8 mg L–1 at the end of the experiment (Figure 5C), consistent with the reports that the addition of NO2– had a negative impact on ammonium removal of bacterium (Yang et al., 2012; Zhang et al., 2015, 2022). A noteworthy observation is that NO2– had no impact on the ammonium efficiency of the △nirBD strain, which reached 97.5% (Figure 5D), possibly due to nirBDs knockout leading to increased NH4+ assimilation and strain growth, thereby enhancing the tolerance of the strain to the toxic NO2–.
Conclusion
nirBDs, which conventionally encode an NADH nitrite reductase, also influence the ammonium transformation of P. putida Y-9. Knocking out nirBDs accelerated the ammonium assimilation and inhibited the emission of the greenhouse gas N2O, thus alleviating the toxicity of nitrite in an ammonium and nitrite system.
Data availability statement
The datasets presented in this study can be found in online repositories. The names of the repository/repositories and accession number(s) can be found below: GenBank, KP410740.
Author contributions
XH: visualization, investigation, data curation, writing—original draft preparation, reviewing, and funding acquisition. YL: investigation, data curation, and formal analysis. LL: investigation and data curation. DX: project administration and supervision. ZL: conceptualization, methodology, and writing—reviewing and editing. All authors contributed to the article and approved the submitted version.
Funding
This work was financially supported by the National Natural Science Fund of China (42107333 and 42077217) and the Natural Science Foundation of Guangxi (2022GXNSFBA035606).
Acknowledgments
We would like to thank Editage (www.editage.cn) for English language editing.
Conflict of interest
XH was employed by Guangxi Bossco Environmental Protection Technology Co., Ltd.
The remaining authors declare that the research was conducted in the absence of any commercial or financial relationships that could be construed as a potential conflict of interest.
Publisher’s note
All claims expressed in this article are solely those of the authors and do not necessarily represent those of their affiliated organizations, or those of the publisher, the editors and the reviewers. Any product that may be evaluated in this article, or claim that may be made by its manufacturer, is not guaranteed or endorsed by the publisher.
Supplementary material
The Supplementary Material for this article can be found online at: https://www.frontiersin.org/articles/10.3389/fmicb.2022.982674/full#supplementary-material
Supplementary Figure 1 | nirBD deletion mutations detection (Lanes 1–6: deletion mutant; Lane 8: wild-type strain Y-9; Lane 9: DL5000 DNA Marker).
References
Ai, G. M., Zheng, H. Y., Zhang, M., and Liu, Z. P. (2011). Isotopic confirmation of occurrence of microbial denitrification based on N2 and N2O production monitored by gas chromatography/isotope ratio mass spectrometry and gas chromatography/mass spectrometry. Chin. J. Anal. Chem. 39, 1141–1146. doi: 10.1016/S1872-2040(10)60460-4
Burkovski, A. (2003). Ammonium assimilation and nitrogen control in Corynebacterium glutamicum and its relatives: An example for new regulatory mechanisms in actinomycetes. FEMS Microbiol. Rev. 27, 617–628.
Canfield, D. E., Glazer, A. N., and Falkowski, P. G. (2010). The evolution and future of Earth’s nitrogen cycle. Science 330, 192–196. doi: 10.1126/science.1186120
Chen, J. L., Xu, J., Zhang, S. N., Liu, F., Peng, J. W., Peng, Y. X., et al. (2021). Nitrogen removal characteristics of a novel heterotrophic nitrification and aerobic denitrification bacteria, Alcaligenes faecalis strain WT14. J. Environ. Manage. 282, 111961. doi: 10.1016/j.jenvman.2021.111961
Chen, J., Gu, S. Y., Hao, H. H., and Chen, J. M. (2016). Characteristics and metabolic pathway of Alcaligenes sp. TB for simultaneous heterotrophic nitrification-aerobic denitrification. Appl. Microbiol. Biot. 100, 9787–9794.
Fischer, M., Schmidt, C., Falke, D., and Sawers, R. G. (2012). Terminal reduction reactions of nitrate and sulfate assimilation in Streptomyces coelicolor A3 (2): Identification of genes encoding nitrite and sulfite reductases. Res. Microbiol. 163, 340–348. doi: 10.1016/j.resmic.2012.05.004
Gennis, R. B., and Stewart, V. (1996). “Respiration,” in Escherichia coli and Salmonella-Cellular and Molecular Biology, eds F. C. Neidhardt, R. Curtiss, III J. L. Ingraham, E. C. C. Lin, K. B. Low, B. Magasanik, et al. (Washington, DC: ASM Press).
Gupta, N., Gupta, A. K., Gaur, V. S., and Kumar, A. (2012). Relationship of nitrogen use efficiency with the activities of enzymes involved in nitrogen uptake and assimilation of finger millet genotypes grown under different nitrogen inputs. Sci. World J. 2012:625731. doi: 10.1100/2012/625731
Huang, X. J., Jiang, D. H., Ni, J. P., Xie, D. T., and Li, Z. L. (2021a). Removal of ammonium and nitrate by the hypothermia bacterium Pseudomonas putida Y-9 mainly through assimilation. Environ. Technol. Innov. 22:101458.
Huang, X. J., Tie, W. Z., Xie, D. T., Jiang, D. H., and Li, Z. L. (2021b). Certain environmental conditions maximize ammonium accumulation and minimize nitrogen loss during nitrate reduction process by Pseudomonas putida Y-9. Front. Microbiol. 12:764241. doi: 10.3389/fmicb.2021.764241
Huang, X. J., Weisene, C. G., Ni, J. P., He, B. H., Xie, D. T., and Li, Z. L. (2020). Nitrate assimilation, dissimilatory nitrate reduction to ammonium, and denitrification coexist in Pseudomonas putida Y-9 under aerobic conditions. Bioresour. Technol. 312:123597. doi: 10.1016/j.biortech.2020.123597
Huang, X. J., Xu, Y., He, T. X., Jia, H. J., Feng, M., Xiang, S. D., et al. (2019). Ammonium transformed into nitrous oxide via nitric oxide by Pseudomonas putida Y-9 under aerobic conditions without hydroxylamine as intermediate. Bioresour. Technol. 277, 87–93. doi: 10.1016/j.biortech.2019.01.040
Jackson, R. H., Cornish-Bowden, A., and Cole, J. A. (1981). Prosthetic groups of the NADH-dependent nitrite reductase from Escherichia coli K12. Biochem J. 193, 861–867. doi: 10.1042/bj1930861
Jin, P., Chen, Y. Y., Yao, R., Zheng, Z. W., and Du, Q. Z. (2019). New insight into the nitrogen metabolism of simultaneous heterotrophic nitrification-aerobic denitrification bacterium in mRNA expression. J. Hazard Mater. 371, 295–303. doi: 10.1016/j.jhazmat.2019.03.023
Jin, R. F., Liu, T. Q., Liu, G. F., Zhou, J. T., Huang, J. Y., and Wang, A. J. (2015). Simultaneous heterotrophic nitrification and aerobic denitrification by the marine origin bacterium Pseudomonas sp. ADN-42. Appl. Biochem. Biotech. 175, 2000–2011. doi: 10.1007/s12010-014-1406-0
Kuypers, M. M. M., Marchant, H. K., and Kartal, B. (2018). The microbial nitrogen-cycling network. Nat. Rev. Microbiol. 16, 263–276. 2018.10.060
Lei, X., Jia, Y. T., Chen, Y. C., and Hu, Y. Y. (2019). Simultaneous nitrification and denitrification without nitrite accumulation by a novel isolated Ochrobactrum anthropic LJ81. Bioresour. Technol. 272, 442–450. doi: 10.1016/j.biortech
Lei, Y., Wang, Y. Q., Liu, H. J., Xi, C. W., and Song, L. Y. (2016). A novel heterotrophic nitrifying and aerobic denitrifying bacterium, Zobellella taiwanensis DN-7, can remove high-strength ammonium. Appl. Microbiol. Biot. 100, 4219–4229. doi: 10.1007/s00253-016-7290-5
Li, Y. T., Wang, Y. R., Fu, L., Gao, Y. Z., Zhao, H. X., and Zhou, W. Z. (2017). Aerobic-heterotrophic nitrogen removal through nitrate reduction and ammonium assimilation by marine bacterium Vibrio sp. Y1-5. Bioresour. Technol. 230, 103–111. doi: 10.1016/j.biortech.2017.01.049
Lin, J. T., and Stewart, V. (1997). Nitrate assimilation by bacteria. Adv. Microb. Physiol. 39, 1–30. doi: 10.1016/s0065-2911(08)60014-4
Macdonald, H., Pope, N. R., and Cole, J. A. (1985). Isolation, characterization and complementation analysis of nirB mutants of Escherichia coli deficient only in NADH-dependent nitrite reductase activity. Microbiology 131, 771–2782.
Malm, S., Tiffert, Y., Micklinghoff, J., Schultze, S., Joost, I., Weber, I., et al. (2009). The roles of the nitrate reductase NarGHJI, the nitrite reductase NirBD and the response regulator GlnR in nitrate assimilation of Mycobacterium tuberculosis. Microbiology 155, 1332–1339. doi: 10.1099/mic.0.023275-0
Miller, J. H. (1972). Experiments in Molecular Genetics. Cold Spring Harbor, NY: Cold spring harbor laboratory.
Muro-Pastor, M. I., Reyes, J. C., and Florencio, F. J. (2005). Ammonium assimilation in cyanobacteria. Photosynth. Res. 83, 135–150. doi: 10.1007/s11120-004-2082-7
State Environmental Protection Administration of China (2002). Water and Wastewater Analysis Methods. Beijing: China Environmental Science Press, 132–286.
Tiffert, Y., Supra, P., Wurm, R., Wohlleben, W., Wagner, R., and Reuther, J. (2008). The Streptomyces coelicolor GlnR regulon: Identification of new GlnR targets and evidence for a central role of GlnR in nitrogen metabolism in actinomycetes. Mol. Microbiol. 67, 861–880. doi: 10.1016/j.biortech.2014.08.058
Vadivelu, V. M., Keller, J., and Yuan, Z. (2006). Effect of free ammonia and free nitrous acid concentration on the anabolic and catabolic processes of an enriched Nitrosomonas culture. Biotechnol. Bioeng. 95, 830–839. doi: 10.1002/bit.21018
Van Heeswijk, W. C., Westerhoff, H. V., and Boogerd, F. C. (2013). Nitrogen assimilation in Escherichia coli: Putting molecular data into a systems perspective. Microbiol. Mol. Biol. R. 77, 628–695. doi: 10.1128/MMBR.00025-13
Xia, L., Li, X. M., Fan, W. H., and Wang, J. L. (2020). Heterotrophic nitrification and aerobic denitrification by a novel Acinetobacter sp. ND7 isolated from municipal activated sludge. Bioresour. Technol. 301:122749. doi: 10.1016/j.biortech.2020.122749
Xu, L., Gu, G. Q., Wei, C., Gao, L. J., Wu, X. H., and Zhang, L. Q. (2018). The outer membrane protein OprF and the sigma factor SigX regulate antibiotic production in Pseudomonas fluorescens 2P24. Microbiol. Res. 206, 159–167. doi: 10.1016/j.micres.2017.10.006
Xu, Y., He, T. X., Li, Z. L., Ye, Q., Chen, Y. L., Xie, E. Y., et al. (2017). Nitrogen removal characteristics of Pseudomonas putida Y-9 capable of heterotrophic nitrification and aerobic denitrification at low temperature. Biomed. Res. Int. 2017:1429018. doi: 10.1155/2017/1429018
Yan, Q., Wu, X. G., Wei, H. L., Wang, H. M., and Zhang, L. Q. (2009). Differential control of the PcoI/PcoR quorum-sensing system in Pseudomonas fluorescens 2P24 by sigma factor RpoS and the GacS/GacA two-component regulatory system. Microbiol. Res. 164, 18–26. doi: 10.1016/j.micres.2008.02.001
Yang, J. R., Wang, Y., Chen, H., and Lyu, Y. K. (2019). Ammonium removal characteristics of an acid-resistant bacterium Acinetobacter sp. JR1 from pharmaceutical wastewater capable of heterotrophic nitrification-aerobic denitrification. Bioresour. Technol. 274, 56–64. doi: 10.1016/j.biortech.2018.10.052
Yang, L., Wang, X. H., Cui, S., Ren, Y. X., Yu, J., Chen, N., et al. (2019). Simultaneous removal of nitrogen and phosphorous by heterotrophic nitrification-aerobic denitrification of a metal resistant bacterium Pseudomonas putida strain NP. Bioresour. Technol. 285, 121360. doi: 10.1016/j.biortech.2019.121360
Yang, X. P., Wang, S. M., and Zhou, L. X. (2012). Effect of carbon source, C/N ratio, nitrate and dissolved oxygen concentration on nitrite and ammonium production from denitrification process by Pseudomonas stutzeri D6. Bioresour. Technol. 104, 65–72. doi: 10.1016/j.biortech.2011.10.026
Ye, J., Zhao, B., An, Q., and Huang, Y. S. (2016). Nitrogen removal by Providencia rettgeri strain YL with heterotrophic nitrification and aerobic denitrification. Environ. Technol. 37, 2206–2213. doi: 10.1080/09593330.2016.1146338
Yukioka, Y., Tanahashi, T., Shida, K., Oguchi, H., Ogawa, S., Saito, C., et al. (2017). A role of nitrite reductase (NirBD) for NO homeostatic regulation in Streptomyces coelicolorA3(2). FEMS Microbiol. Lett. 364:fnw241. doi: 10.1093/femsle/fnw241
Zemke, A. C., Kocak, B. R., and Bomberger, J. M. (2017). Sodium nitrite inhibits killing of Pseudomonas aeruginosa biofilms by ciprofloxacin. Antimicrob. Agents Chemother. 61:e00448-16. doi: 10.1128/aac.00448-16
Zhang, M. M., He, T. X., Chen, M. P., and Wu, Q. F. (2022). Ammonium and hydroxylamine can be preferentially removed during simultaneous nitrification and denitrification by Pseudomonas taiwanensis EN-F2. Bioresour. Technol. 350:1269126. doi: 10.1016/j.biortech.2022.126912
Zhang, M. X., Li, A. Z., Yao, Q., Wu, Q. P., and Zhu, H. H. (2020). Nitrogen removal characteristics of a versatile heterotrophic nitrifying-aerobic denitrifying bacterium, Pseudomonas bauzanensis DN13-1, isolated from deep-sea sediment. Bioresour. Technol. 305:122626. doi: 10.1016/j.biortech.2019.122626
Keywords: Pseudomonas putida Y-9, nirBDs, glnA, ammonium assimilation, ammonium oxidation
Citation: Huang XJ, Luo YW, Luo L, Xie DT and Li ZL (2022) The nitrite reductase encoded by nirBDs in Pseudomonas putida Y-9 influences ammonium transformation. Front. Microbiol. 13:982674. doi: 10.3389/fmicb.2022.982674
Received: 30 June 2022; Accepted: 21 September 2022;
Published: 12 October 2022.
Edited by:
Ang Li, Harbin Institute of Technology, ChinaReviewed by:
Hadiastri Kusumawardhani, Université de Lausanne, SwitzerlandShiyang Zhang, Wuhan University of Technology, China
Shanshan Pi, Harbin Institute of Technology, China
Jin Li, Qingdao University, China
Copyright © 2022 Huang, Luo, Luo, Xie and Li. This is an open-access article distributed under the terms of the Creative Commons Attribution License (CC BY). The use, distribution or reproduction in other forums is permitted, provided the original author(s) and the copyright owner(s) are credited and that the original publication in this journal is cited, in accordance with accepted academic practice. No use, distribution or reproduction is permitted which does not comply with these terms.
*Correspondence: Xuejiao Huang, aHh1ZWppYW8wNDEyQHNpbmEuY29t; Zhenlun Li, bGl6aGx1bjQ3NDBAc2luYS5jb20=