- 1Red de Manejo Biorracional de Plagas y Vectores, Clúster Científico y Tecnológico Biomimic®, Instituto de Ecología, A.C., Xalapa, Mexico
- 2Instituto de Biología Integrativa de Sistemas (I2SysBio), Universidad de Valencia and Consejo Superior de Investigaciones Científicas (CSIC), Valencia, Spain
- 3Fundación para el Fomento de la Investigación Sanitaria y Biomédica de la Comunidad Valenciana (FISABIO), Valencia, Spain
- 4Red de Estudios Moleculares Avanzados, Clúster Científico y Tecnológico Biomimic®, Instituto de Ecología, A.C., Xalapa, Mexico
- 5Red de Biodiversidad y Sistemática, Clúster Científico y Tecnológico Biomimic®, Instituto de Ecología, A.C., Xalapa, Mexico
The gut microbiota is key for the homeostasis of many phytophagous insects, but there are few studies comparing its role on host use by stenophagous or polyphagous frugivores. Guava (Psidium guajava) is a fruit infested in nature by the tephritids Anastrepha striata and A. fraterculus. In contrast, the extremely polyphagous A. ludens infests guava only under artificial conditions, but unlike A. striata and the Mexican A. fraterculus, it infests bitter oranges (Citrus x aurantium). We used these models to analyze whether the gut microbiota could explain the differences in host use observed in these flies. We compared the gut microbiota of the larvae of the three species when they developed in guava and the microbiota of the fruit pulp larvae fed on. We also compared the gut microbiota of A. ludens developing in C. x aurantium with the pulp microbiota of this widely used host. The three flies modified the composition of the host pulp microbiota (i.e., pulp the larvae fed on). We observed a depletion of Acetic Acid Bacteria (AAB) associated with a deleterious phenotype in A. ludens when infesting P. guajava. In contrast, the ability of A. striata and A. fraterculus to infest this fruit is likely associated to a symbiotic interaction with species of the Komagataeibacter genus, which are known to degrade a wide spectrum of tannins and polyphenols. The three flies establish genera specific symbiotic associations with AABs. In the case of A. ludens, the association is with Gluconobacter and Acetobacter, but importantly, it cannot be colonized by Komagataeibacter, a factor likely inhibiting its development in guava.
Introduction
The gut of most living organisms harbors complex microbial communities, which are involved in multiple processes of the host’s biology (Wu and Wu, 2012; Cénit et al., 2014; Shin et al., 2015; Chen et al., 2016). In insects, the gut microbiota is frequently associated with essential functions for the host, as occurs in bees (Kwong and Moran, 2016), olive fly (Capuzzo et al., 2005; Ben-Yosef et al., 2010, 2015), flea beetles (Shukla and Beran, 2020) and bark beetles (Adams et al., 2013). In some phytophagous insects, certain components of the gut microbiota are essential for survival, as is the case with the olive fly, Bactrocera oleae (Gmelin) (Diptera: Tephritidae) and its obligate endosymbiont “Candidatus Erwinia dacicola”. This bacteria is essential for B. oleae larvae, playing a key role for its development in the immature olive (Capuzzo et al., 2005; Ben-Yosef et al., 2015), as unripe olives contain high concentrations of secondary metabolites that are lethal to the B. oleae larva, such as oleuropein (Gutierrez-Rosales et al., 2012). “Candidatus Erwinia dacicola” can metabolize oleuropein, allowing the fly larva to feed on unripe olives and successfully complete their development (Shukla and Beran, 2020). Recently, Aluja et al. (2021), while studying the gut microbiota of Anastrepha ludens Loew (Diptera: Tephritidae) larvae and adults stemming from six natural hosts including the native ancestral Casimiroa edulis La Llave and C. greggii (S. Watson) F. Chiang (both Rutaceae), the exotics Mangifera indica L. cv. Ataulfo (Anacardiaceae), Prunus persica (L.) Batsch (Rosaceae), and Citrus x aurantium L. (Rutaceae), and the occasional native host Capsicum pubescens Ruiz and Pav. cv. Manzano (Solanaceae), observed a decrement in the relative abundance of representatives within the Acetobacteraceae, followed by an increment of representatives within the Enterobacteriaceae in larvae developing in certain hosts. This shift in the gut microbiota, with an increment in the ratio Enterobacteriaceae/Acetobacteraceae was most apparent in the marginal host C. pubescens, most likely leading to the poor larval development and high fitness costs observed by Birke and Aluja (2018).
Tephritid flies are a highly diverse group of insects with over 5,000 species described so far (Norrbom et al., 2018). A small proportion of these species are key pests worldwide causing significant economic losses via direct damage to the fruit (larvae feeding in them render the fruit unmarketable) or indirectly via severe trade restrictions (Aluja and Mangan, 2008). A relevant genus among the Tephritidae group is the genus Anastrepha, which is endemic to the Neotropical region (Aluja, 1994), with over 300 species identified so far (Norrbom et al., 2018). In Mexico, and several countries in Central America, A. ludens is the most economically important species attacking various citrus species (e.g., Citrus x sinensis L. Osbeck, C. x aurantium L.) and mango (M. indica), as well as many other commercial fruit and vegetables (e.g., manzano pepper, C. pubescens) (Birke et al., 2015). Importantly for the purposes here, the limit to the extreme polyphagy of A. ludens is represented by guava (Psidium guajava), a chemically defended fruit widely attacked by A. striata and the Mexican form of A. fraterculus (Hernandez-Ortiz, 1993). In the case of A. ludens, only artificial infestations in fully ripe guavas under field cage or laboratory conditions have been possible, and even under such artificial conditions, the negative effect on larval and adult fitness were highly significant (Birke et al., 2015; Birke and Aluja, 2018). Under these conditions, few larvae survived, their development was slow, and their pupal weight was significantly lower when compared to pupae originating from natural hosts such as C. edulis (ancestral host), C. x aurantium or M. indica (Birke et al., 2015; Birke and Aluja, 2018). In contrast, A. striata is a stenophagous tephritid species specialized in infesting Psidium spp., while the Mexican form of A. fraterculus is unable to attack citrus (Aluja et al., 2003) [it does so in South America (Salles, 2000)], but infests various species within Psidium, Syzygium jambos and Prunus persica (Aluja et al., 2000; Sivinski et al., 2004). Although the simultaneous occurrence of A. striata and A. fraterculus in P. guajava is common (Sivinski et al., 2004), infestation occurs at different phenological stages of the fruit (Birke et al., 2015; De Oliveira et al., 2015). While A. striata infests hard, immature guavas that are chemically astringent with high levels of tannins (Lee et al., 2010), A. fraterculus prefers stages closer to maturation, with a higher sugar content and lower levels of polyphenols (De Oliveira et al., 2015).
Following the “call for further research on this underappreciated component of insect plant (and enemy) interactions” by Hammer and Bowers (2015), here we compared the gut microbiota of wild A. striata and A. fraterculus larvae developing in P. guajava, plus A. ludens larvae artificially developing in this fruit. The gut microbiota of A. ludens larvae developing in the natural host C. x aurantium was also analyzed to contrast the composition of the gut microbiota in a natural and a conditional/artificial host (sensu Aluja and Mangan, 2008). In both cases, we also analyzed the pulp surrounding the larvae. We predicted that the inability of A. ludens to infest P. guajava in nature reported by Birke (Birke et al., 2015) could be due, in part, by a dysbiotic larval gut microbiota caused by the deleterious guava pulp rife with polyphenols or the lack of adequate bacterial associations enabling the metabolization of toxic pulp components.
Materials and methods
Collection of biological material
Wild A. striata, A. fraterculus and A. ludens larvae were collected from mature fruit with signs of infestation in the orchard “Finca Costa Rica” (Tuzamapan, Veracruz 19°23′56.58″N, 96°53′11.79″W, 834 masl). Additionally, we collected A. ludens from Teocelo, Veracruz (19°24′57.26″N, 96°58′18.74 ″W, 1,193 masl). Guavas were harvested from various trees and were used to obtain A. striata and A. fraterculus. Meanwhile, bitter oranges (C. x aurantium) were harvested to obtain A. ludens. In both cases, the fruit were transported to the laboratory and immediately dissected to search for third instar larvae.
Since it is common to find A. striata and A. fraterculus simultaneously infesting the same guava fruit (Sivinski et al., 2004), all larvae were identified to species. In the third instar, A. striata larvae exhibit a clear division in the anal lobe, which is missing in the larvae of A. fraterculus, leading to a reliable differentiation of both species (Steck et al., 1990). There was no need to identify the larvae found in bitter oranges as in Mexico only A. ludens infests citrus (Aluja et al., 2000). Voucher specimens of each species were kept in INECOL’s Biorational Pest and Vector Management Network (RMBPV).
To characterize the microbiota of fruit where larvae develop (larval niche) and determine the likely microbial exchange between the insect and the fruit, we also collected fruit pulp surrounding the larvae. Both larvae and pulp samples were frozen in liquid nitrogen and stored at −80°C until processing.
Forced infestation of guavas by A. ludens
Forced infestation tests were run in an abandoned guava orchard in Piedra de Agua, Veracruz (19°33′15.54 ″ N, 96°57′46.89 ″ W, 1,531 masl), with A. ludens adults originating from infested bitter oranges (C. x aurantium) collected in neighboring localities. Procedures for fruit handling and harvesting of pupae/adults are described in detail in Aluja et al. (2000). Once the A. ludens adults emerged, they were kept in 30 × 30 × 30 cm3 plexiglass cages, covered with Teflon mosquito netting. They were supplied with water and an artificial diet ad libitum. The diet consisted of hydrolyzed protein (MP Biomedicals, Agrisent de Mexico S.A. de C.V., Mexico) and refined cane sugar in a 1:3 ratio. The flies were kept for 15 days at 27 ± 1°C, 63 ± 5% RH and 12:12 h light: dark photoperiod until they reached sexual maturity. Females were not supplied with an oviposition substrate to promote the accumulation of eggs in their ovaries and thus facilitate oviposition into the non-natural host guava (Aluja and Mangan, 2008).
Uninfested guavas were obtained in the field as follows: fruiting trees of a manageable size were identified in the above mentioned abandoned guava orchard and some fruit-bearing branches were covered with organza cloth bags several weeks prior to the bioassay (at this stage fruit were very unripe and not suitable for infestation by either A. striata or A. fraterculus). We inspected all fruit to make sure that they did not show signs of infestation by Conotrachelus (Coleoptera: Curculionidae: Molytinae), a beetle commonly infesting guavas in the study region (Palemon-Alberto et al., 2021). Two-week-old sexually mature, mated A. ludens adults, stemming from the oranges previously collected in the field, were transported to the study site in Piedra de Agua from our laboratory at INECOL’s headquarters. Following the protocols of Aluja et al. (2000) and Birke et al. (2015), six sexually mature A. ludens specimens in perfect physical condition were selected (i.e., intact wings, with active locomotor activity). These flies were released into the fruit-bearing branches we had previously covered to preclude wild flies and beetles from laying eggs into them. The sex ratio of flies in each bag was 1:1, and the amount of fruit in each bag was between two and four. At the moment of fly release, the guavas were at the ‘yellow in transition’ maturity stage (Birke et al., 2015). Females were allowed to oviposit into guavas for three days and thereafter fruit were monitored daily to detect any that had naturally detached from the branch. Once natural detachment occurred, fruit were collected and transported to the laboratory where they were kept under the conditions described above. Fruit was dissected when they began to show signs of decay. Larvae were immediately processed as described in what follows.
Sample preparation
Larvae from guavas and oranges were superficially sanitized by the following series of washes: 1-min wash in 500 μl of washing solution (SDS 1%, Tris 10 mM and NaCl 10 mM), followed by a 1-min wash in 500 μl of 1% commercial sodium hypochlorite, and a 1-min wash in 500 μl of 70% ethanol. Finally, two 1-min washes were performed with 500 μl of sterile distilled water. After sanitization, the digestive tracts were dissected from the proventriculus to the terminal region of the hindgut. The dissection of larvae was performed with sterile forceps using a stereoscope (Nikon SMZ 1500, Tokyo, Japan). The sample unit was defined as a pool of five gut tracts. In addition, five mg of the pulp surrounding each larva were collected. Then, pools of 25 mg were obtained for each pulp sample. In turn, each sample unit had five replicates. Samples of larvae guts were kept in 450 μl of RNAlater™ Stabilization Solution (Thermo Scientific©) until processing within 24 h of collection.
gDNA extraction and 16S rRNA gene sequencing
Once the RNAlater™ was removed, all samples were deep frozen in liquid nitrogen and pulverized with a sterile pistil. gDNA extraction was performed with the QIAamp DNA Mini Kit from QIAGEN© (Hilden, Germany). The gDNA obtained was used for the amplification of the 16S rRNA gene. Primers for 16S rRNA gene v3-v4 amplification were selected from Klindworth et al. (2013) and adapted according to the 16S metagenomic sequencing library preparation guide1 : 16S Amplicon PCR Forward 5′ TCGTCGGCAGCGTCAGATGTGTA TAAGAGACAGCCTA CGGGNGGCWGCAG and 16S Amplicon PCR Reverse 5′ GTCTCGTGGGCTCGGAGATGTGTATAAGAGACAGGACT ACHVGGGTATCTAATCC. PCR amplification was performed using an enrichment strategy. The enrichment consisted of an initial amplification of 15 cycles, starting from 300 ng of gDNA. Then, 2 μl of PCR1 were used as a template to carry out a PCR2 of 25 cycles. The composition of PCR1 (25 μL) and PCR2 (50 μL) consisted of Qiagen buffer 1X, dNTPs 0.2 mM, MgCl2 0.1 μM, 16s Amp F and R 0.2 μM each one, and Taq Polymerase 0.05 U, with an amplification program of 94 °C/2 min, 15 (PCR1) or 25 (PCR2) cycles of 94 °C/15 seg, 55 °C/30 seg y 72 °C/1 min, and finally 72 °C/5 min. Amplicons were purified with the Promega Wizard® SV Gel and PCR Clean-Up System kit and their concentration was determined by a Qubit 2.0 Fluorometer (Thermo®, United States). Purified PCR products were indexed with Ilumina© sequencing adapters using the Nextera XT Index Kit from Illumina© and were purified and quantified as previously described. The quality of the library was determined using the Agilent Bioanalyzer 2100® system. The indexed amplicons were sequenced in a paired-end format (2 × 300 bp) using a MiSeq Reagent Kit V3 (600 cycles), on a MiSeq platform from Illumina©. Sequencing was carried out by the Sequencing Unit of the BioMimic™ Scientific and Technological Cluster at the Instituto de Ecologia, A.C.—INECOL.
Bioinformatic and statistical analyses
The raw reads in paired-end layout (2 × 300) were processed in the QIIME2 (v. 2020.6) platform (Bolyen et al., 2019). We used the dada2 (Callahan et al., 2016) plugin to denoise and resolve the amplicon sequences variants (ASVs) with the following parameters: for forward reads, trimming in the position 20 at the 5′ end and truncating to a length of 270 pb; for reverse reads, trimming in the position 20 at the 5′ end and truncating to a length of 200 pb; removing the chimeric reads with the “consensus” method; the other parameters were used as default. The representative sequences of the resolved ASVs were classified with the classify-consensus-v-search plugin (Rognes et al., 2016), using the SILVA v.132 database as reference (Quast et al., 2013). A phylogeny of the representative sequences of ASVs was built, using the align-to-tree-mafft-fasttree plugin, which uses MAFFT (Katoh, 2002) for the alignment, and FastTree2 to build the phylogeny (Price et al., 2010). All data, the abundance table and phylogeny, were exported to the R environment.
In R (v. 4.1.2), we used the phyloseq (McMurdie and Holmes, 2013) package to perform the diversity analysis. Our sampling protocol allowed for the detection of bacteria inhabiting both, lumen and intracellular gut microbial niches, as we dissected the gut of individuals under aseptic conditions, avoiding pulling other organs/structures. We ended with a clean gut, and therefore are confident that the bacterial DNA studied stems only from gut tissue. So, we sampled the microbiota and endosymbionts. Therefore, first we filtered out the plastid and mitochondrial ASVs. Then, upon the taxonomic classification, we separated the complete data set in two analyzable subunits: gut microbiota and endosymbionts (ASVs classified as within Wolbachia) which were analyzed separately. To gain insight into specific species of ASVs classified within Wolbachia, we performed a BLASTn search against the SILVA database (v132) with a cutoff of e-value < 0.0003 and “-max_target_seqs” 3. To correct the bias related to the different size in the sample counts, both data sets were normalized with the cumulative sum scaling (CSS) method using the metagenomeSeq package (Paulson et al., 2013). The composition at class and genus level was scaled to relative abundance and visualized with bar plots using the ggplot2 package (Wilkinson, 2011). The alpha diversity indexes, observed species, and Shannon were then calculated. To assess the significant differences in beta diversity between gut microbiota in flies, and between pulp and gut microbiota, the weighted and unweighted UniFrac distance matrixes were calculated, and a Permutational Multivariate Analysis of Variance (PERMANOVA) was applied with the vegan package (Oksanen, 2015) in one way and pairwise mode; p-values in pairwise mode were adjusted with the Benjamini–Hochberg (BH) method. A Principal Coordinate Analysis was plotted to visualize the ordination of samples based on UniFrac distance metrics (beta diversity). With the aim of detecting differentially abundant genera in the gut microbiota between species, we also performed a linear discriminant analysis Effect Size (LEfSe) (Segata et al., 2011). Statistical significance was set to a p-value < 0.05.
Results
General characteristics of the gut microbiota of A. striata, A. fraterculus and A. ludens
The entire data set comprised 4,091,529 high quality reads, distributed in 40 samples with a mean of 102,288 reads. The data set with only the gut and pulp microbiota (excluding the endosymbiont Wolbachia) was composed of 3,398,023 reads, with a sample mean of 84,951 reads. Considering all samples, we detected a total of 1,884 ASVs, distributed among 19 phyla, and 29 classes. Within the wide taxonomic spectrum, 11 classes exhibited more than 1% of relative abundance in at least one sample (Figure 1A). Of the latter, three classes were dominant (i.e., with mean abundances over 1%): Alphaproteobacteria (49.8%), Gammaproteobacteria (34.1%), and Bacilli (5.1%).
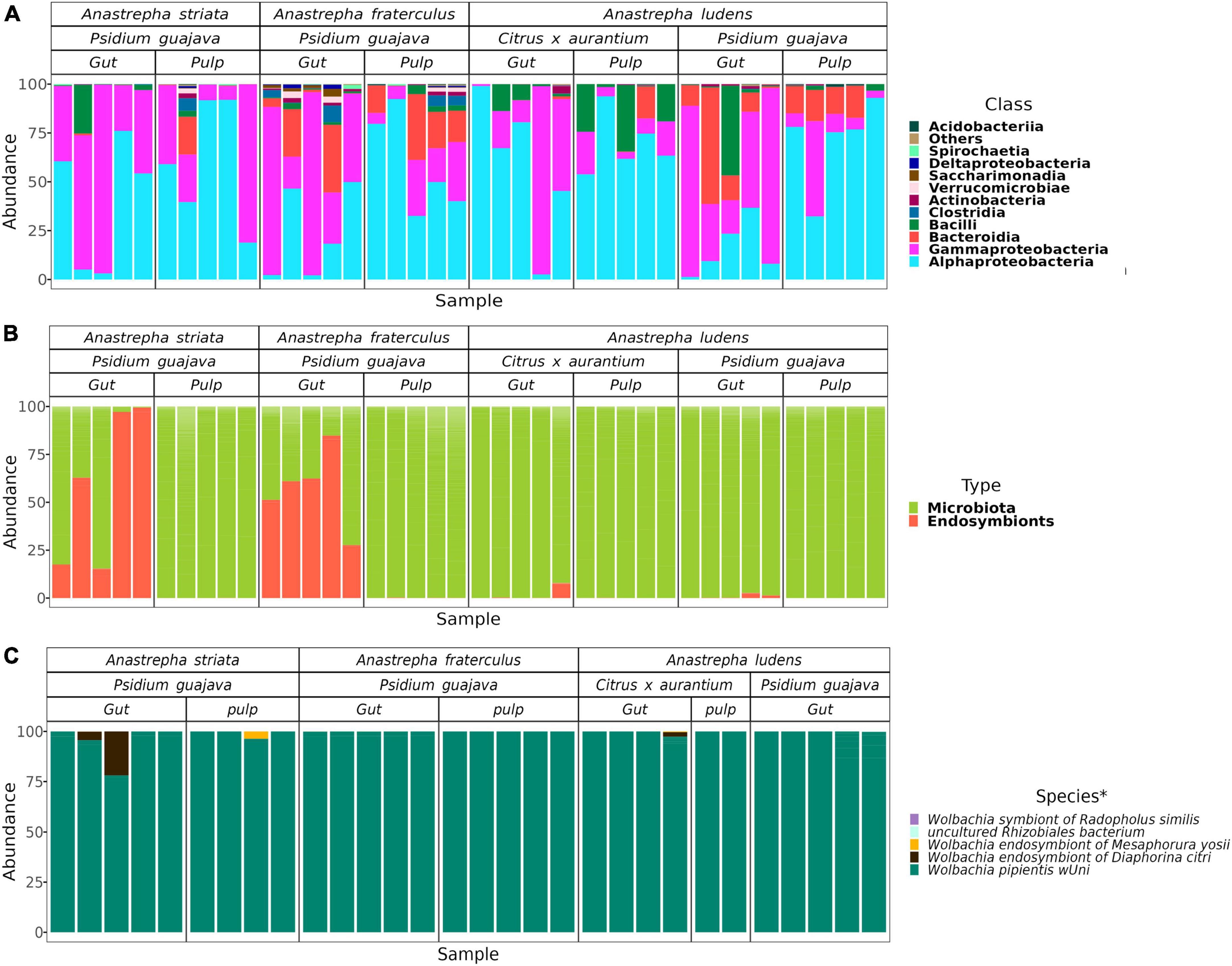
Figure 1. Relative abundance of the microbial components in the gut of Anastrepha striata, A. fraterculus and A. ludens and the microbiota of pulp where they feed on. (A) Relative abundance at the class level of the gut and pulp microbiota; classes with relative abundance lower than 1% were placed in the “Others” category; (B) Composition of both types of microbial components in the gut in all samples; (C) Relative abundance of the endosymbionts in the samples where they were present. In the pulp of C. aurantium there were only a mean of 9 Wolbachia reads (0.0156% of the complete data set) and therefore these extremely low numbers do not appear in (B). *The species related to the ASVs of endosymbionts were identified by sequence similarity via a BLAST search.
The data set with only Wolbachia amplicons was composed of 693,473 reads, having very different coverage between the three flies (Figure 1B). In the gut of A. ludens larvae that fed on guava pulp, the mean number of reads was 1,181, while in the guava pulp Wolbachia was not detected. The gut of A. ludens larvae that fed on C. x aurantium pulp, had a sample mean of 1,378, while in the pulp Wolbachia was barely detected in very low counts, with a sample mean of 9 reads. Conversely, the guts of A. striata and A. fraterculus, both feeding on guava pulp, had similar compositions with high Wolbachia counts (sample mean of 68,036), but in the pulp, counts were very low, with a sample mean of 58. That is, the few Wolbachia’s detected in the pulp of guava most likely stemmed from the feces of the larvae, as opposed to be naturally living there. In this data set, we detected 40 ASVs. The most abundant ASVs where closely related or belonging to Wolbachia pipientis wUni (having a 96–99% of sequence similarity, Supplementary Table 1), with a mean relative abundance of 98.9%. The other three species of Wolbachia, classified in the SILVA database were: Wolbachia endosymbiont of Diaphorina citri, Wolbachia endosymbiont of Mesaphorura yosii, and Wolbachia ensymbiont of Radopholus similis, all present in low abundances, with a mean of less than 1% (Figure 1C).
The microbiota of A. striata, A. fraterculus and A. ludens developing in P. guajava
The microbiota composition of the three fly species was significantly different when they fed on P. guajava. The PERMANOVA test based on weighted and unweighted UniFrac distances detected 27 and 31% of variance, respectively, explained between species (Supplementary Table 1). However, when the PERMANOVA was run in a pairwise mode (one to one), only the unweighted metric detects significant differences among the three species, explaining a range of variance from 23 to 28% (Table 1). Likewise, the PCoA analysis showed a clear separation in three clusters (Figure 2). By contrast, the pulp microbiota was not significantly different with respect to the gut microbiota in the alpha (Supplementary Figure 1 and Supplementary Table 2) and beta diversity (Figure 2 and Supplementary Table 2).
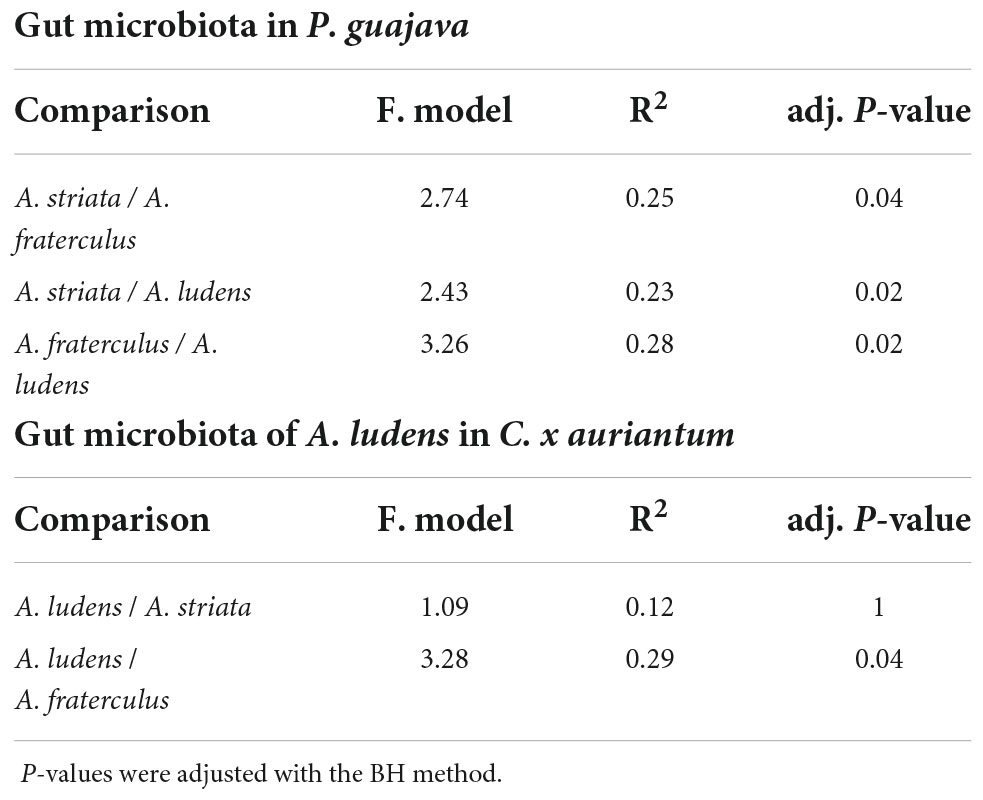
Table 1. Pairwise PERMANOVA comparisons of the gut microbiota of Anastrepha ludens, A. striata, and A. fraterculus larvae stemming from P. guajava or C. x aurantium.
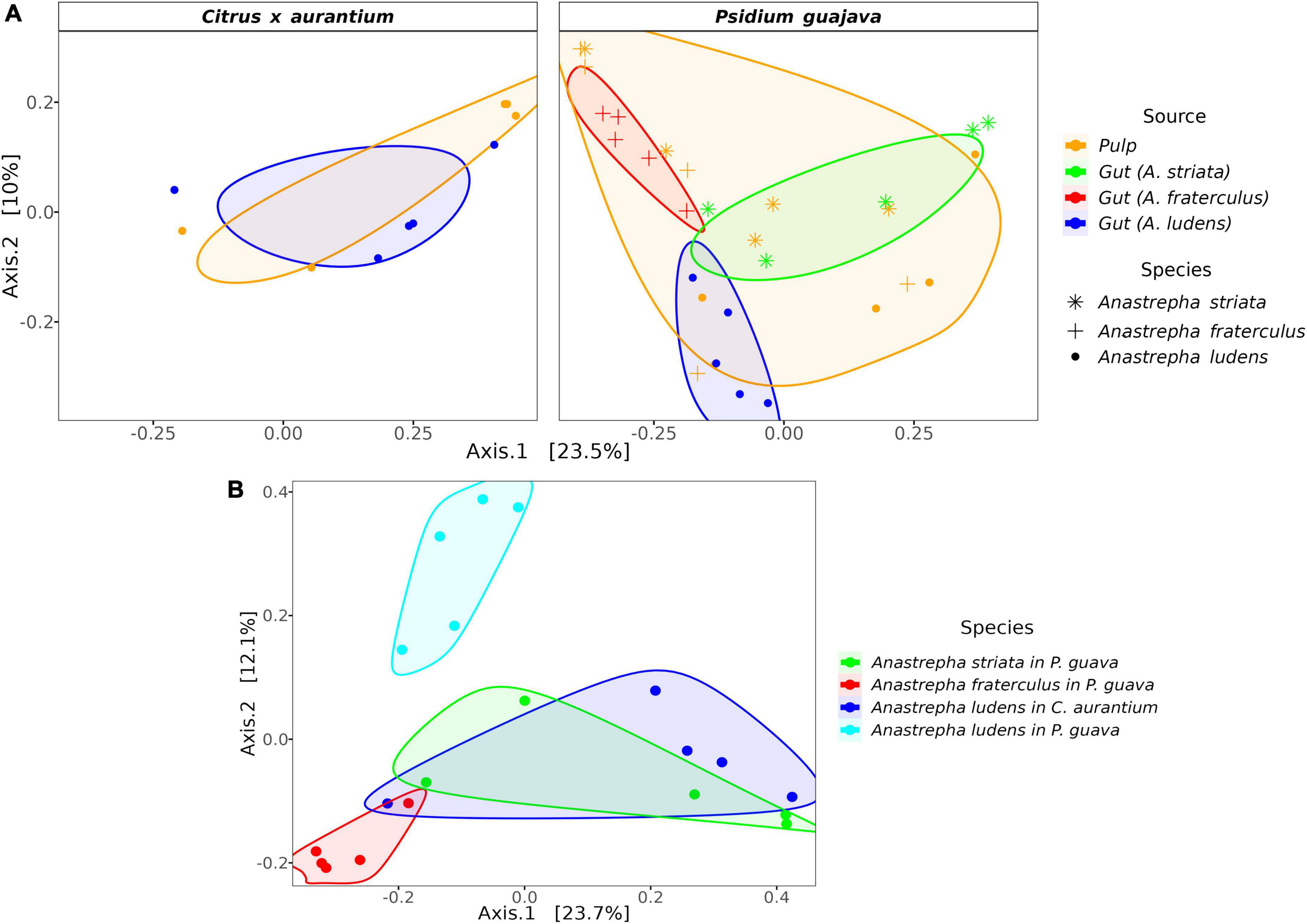
Figure 2. PCoA based on the Unweighted UniFrac distance metric. (A) PCoA showing the ordination of the gut and pulp microbiota but separated by host plant; samples of pulp where each species feed on are more like their gut microbiota; (B) PCoA only showing the ordination of the gut microbiota and illustrating how A. ludens separated from the rest when reared on P. guajava. Note that the microbiota of larvae stemming from C. x aurantium is more like A. striata.
We detected 26 genera with differential abundance between A. ludens and A. fraterculus, and 19 differential genera between A. ludens and A. striata (Figures 3, 4A). The enriched genera in A. ludens were: Pantoea, Gluconacetobacter, Ochrobactrum, Asaia, Curtobacterium, and Leucobacter. Interestingly, the differential genus Komagataeibacter was shared between A. striata and A. fraterculus, with a higher LDA score and relative abundance in A. striata (Figures 3A,C, 4C,D).
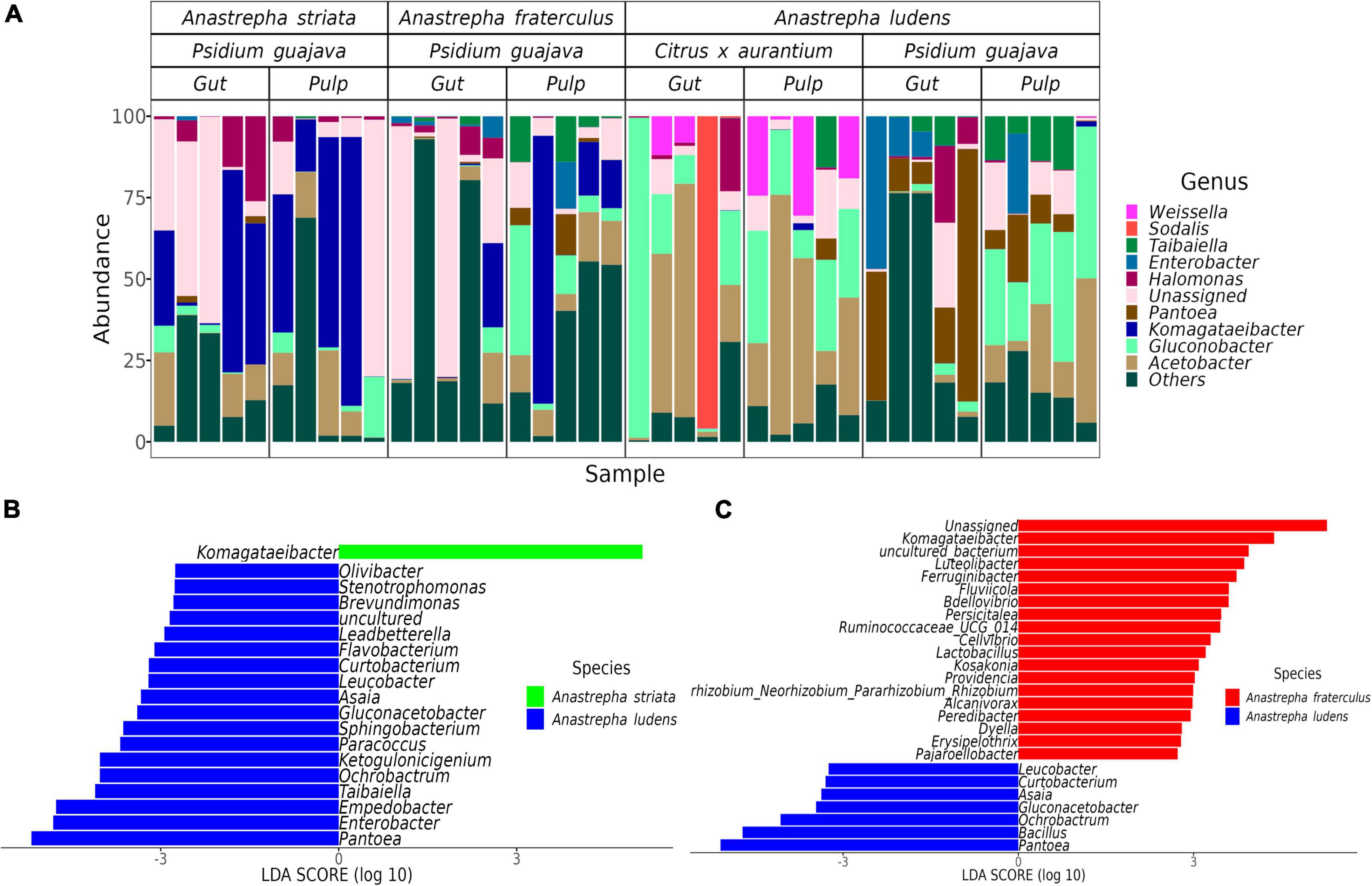
Figure 3. Microbiota composition at genus level and the differences when the flies feed on P. guajava. (A) Relative abundance of the top 10 genera present in all samples; remaining genera where agglomerated in the “Others” category. Differential genera detected by the LEfSe analysis in paired comparisons of A. ludens with A. fraterculus (B) and A. striata (C).
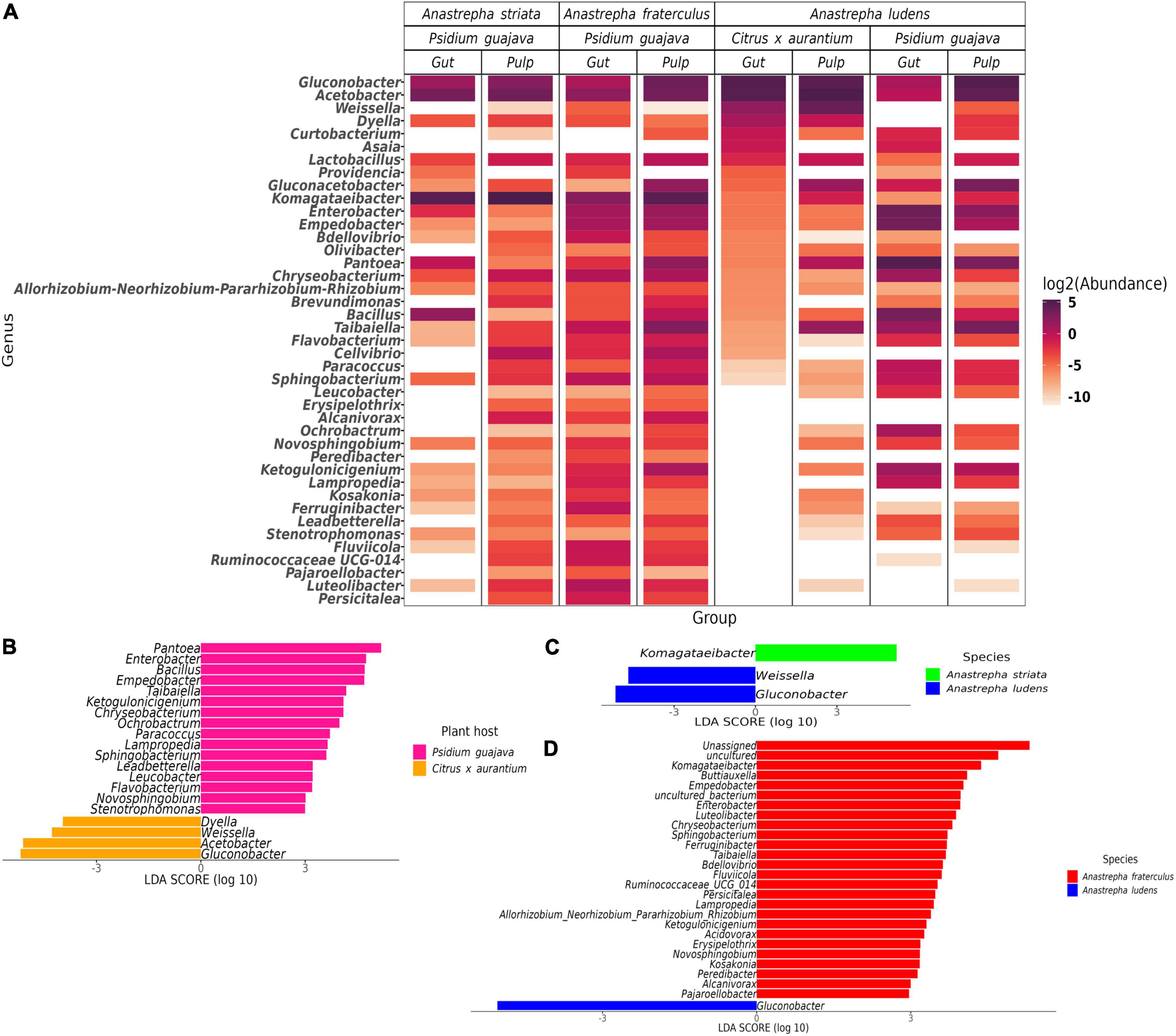
Figure 4. Differences in the normal gut microbiota of A. ludens in Citrus x aurantium compared with A. striata, A. fraterculus and its dysbiotic state in P. guajava. (A) Heatmap of all differential genera identified among the three fly species in all samples; (B) Differentially abundant genera in A. ludens when developing in C. x aurantium (normal) or P. guajava (dysbiotic); Differentially abundant genera of the normal gut microbiota of A. ludens compared with A. striata (C) and A. fraterculus (D).
Composition of the gut microbiota of larvae of A. ludens developing in bitter oranges and guavas
The gut microbiota ofA. ludens larvae exhibited a clear dysbiotic state when they fed on P. guajava pulp compared with C. x aurantium, a natural host. The PCoA showed two clusters clearly separated between both types of fruit (Figure 2B), and the PERMANOVA test detected 26% of variance explained by host type (Table 1). The LEfSe analysis detected 20 differentially abundant genera (Figure 4B). When A. ludens fed on guava, we observed a depletion of Gluconobacter, Acetobacter, Weissella, and Dyella. Conversely, the most incremented genera were Pantoea and Enterobacter.
Surprisingly, the gut microbiota of A. ludens larvae reared in C. x aurantium was not significantly different from the A. striata one, and both microbiotas clustered together (Figure 2B and Table 1). LEfSe analysis detected only three differential genera between both fly species, with Weissella and Gluconobacter being overrepresented in A. ludens, and Komagataeibacter overrepresented in A. striata (Figure 4C). From a general taxonomic perspective, we detected a shift in the abundance ratio of Enterobacteriaceae/Acetobacteraceae families in A. ludens (Figure 5A), and the total absence of the genus Komagataeibacter, which belongs to the Acetobacteraceae family (Figure 5B).
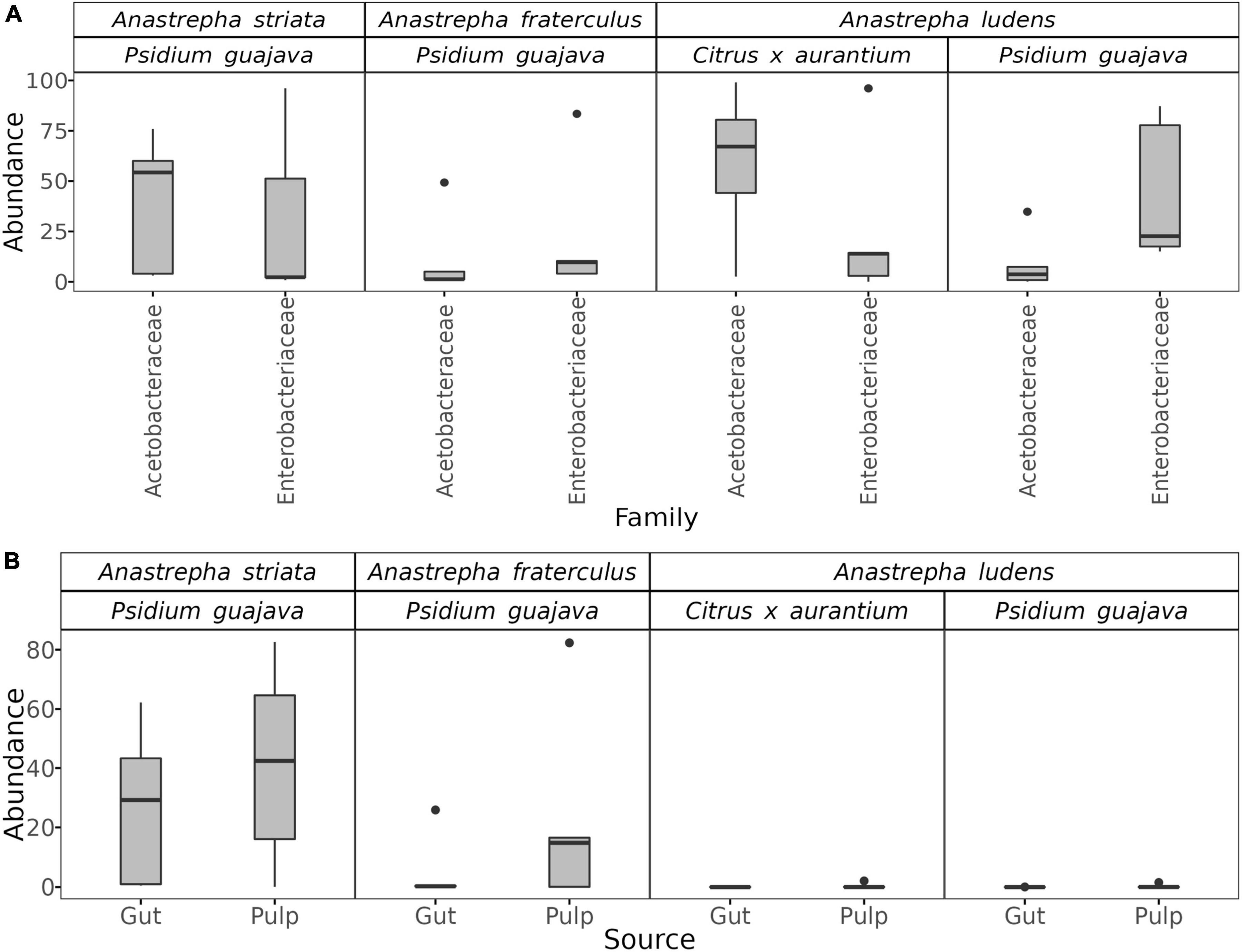
Figure 5. Boxplots showing the abundance distributions of the Acetobacteraceae and Enterobacteriaceae families in the gut microbiota of Anastrepha striata, A. fraterculus and A. ludens (A), and the distribution of the genus Komagataeibacter in the gut and pulp microbiota according to fly species and host type (B).
Discussion
Several findings stand out that we believe merit discussion: (1) Overall (considering the three fly species and two fruits studied), Alphaproteobacteria (49.8%), Gammaproteobacteria (34.1%), and Bacilli (5.1%) where the dominant groups of bacteria identified in guts of larva and fruit pulp; (2) the ubiquitous presence of Komagataeibacter (Acetobacteraceae), likely playing a key role in metabolizing deleterious secondary metabolites in A. striata, a tephritid fly that attacks guava when still very unripe and rife with tannins; (3) the less abundant presence of the same bacteria in A. fraterculus, another tephritid species naturally developing in guava, albeit in a much more developed ripening stage when compared to A. striata; (4) the lack of Komagataeibacter in the guts of A. ludens forcibly infesting ripe P. guajava fruit and the dysbiotic state of the larval microbiota of this species when developing in guavas; (5) the apparent critical role of the Enterobacteriaceae/Acetobacteraceae ratio in the fitness of the three tephritid flies studied; 6) The endosymbiont W. pipientis wUni was present in large numbers in A. striata and A. fraterculus, but almost inexistent in A. ludens and the pulp of guava and C. x aurantium.
At a gross taxonomic level, the gut microbiota composition was similar among the three fly species studied. Dominance of these classes, belonging to Proteobacteria and Firmicutes phyla, has also been reported in the few studies performed so far on the gut microbiota of Anastrepha species (Ventura et al., 2018; Gallo-Franco and Toro-Perea, 2020; Salgueiro et al., 2020; Aluja et al., 2021), and is a common pattern in the gut of phytophagous insects (Yun et al., 2014; Gales et al., 2018). The same pattern was observed in the surrounding pulp microbiota where the flies fed on. The cause of the latter could be that after egg hatch, larvae begin to move and feed in pulp, but with a limited digestive system, especially in the first instar (Carroll and Wharton, 1989). Most fruit fly larvae can only absorb liquid substrates, and thus regurgitate saliva with digestive enzymes such as proteases to dissolve solid food (Singh et al., 1988). The latter, added to the larval feces, could modify the conditions of the environment larvae face early in their development, hydrolyzing sugars, proteins, and other nutrients generating a “house foundation.” This seems to be the behavior of the three species of larvae in this study since the surrounding pulp microbiota where the larvae feed was most like the gut microbiota of each species.
We note that in this study we aimed at determining the potential role that gut microbiota plays in the survival of three species of fruit fly larvae in an environment rife with deleterious chemicals to the insects (e.g., tannins). We were interested in learning how larvae construct their niche in this “toxic” environment and transfer their gut microbiota (mostly acquired from the mother via vertical transmission) to their surroundings by defecating, regurgitating, and sucking. This process could ameliorate the tannin-rich pulp condition via the microbiota metabolism, enriching its surroundings with beneficial taxa that potentially break down toxic compounds, as we infer from our results. We fully recognize, that ideally, we should have compared the two types of pulp, totally clean, uninfested pulp, and pulp in the vicinity of the feeding larvae. However, based on literature reports (Drobya and Wisniewskib, 2018; Huang et al., 2020; Zhang et al., 2021; Malacrinò et al., 2022; Wassermann et al., 2022), we knew that pulp from an undamaged/intact (i.e., insect, pathogen, bird damage) fruit, contain a very different microbial profile (endophytic bacteria and fungi), and once a wound occurs, the microbiota profile changes rapidly. In our study we found components of the gut microbiotas of some of the fruit fly species studied in the pulp, such as the endosymbiont Wolbachia, which suggests that the larvae modify the pulp microbiota as Wolbachia is not a fruit endophyte. We nevertheless recognize that the lack of a bacterial profile of the clean guava pulp in our study could be a caveat that we will need to remediate in future studies. But importantly, the missing absolute control did not distort our results here. Furthermore, as we knew that females are very selective when choosing a particular oviposition site (i.e., fruit; Birke and Aluja, 2018), we avoided biasing our sampling approach by only working with infested fruit (i.e., circumventing the risk of sampling fruit that the females had rejected).
We were surprised/intrigued at the similarity of the gut microbiota of A. striata reared in guava and A. ludens reared in bitter orange, when this similarity would have been expected in the cases of A. striata and A. fraterculus, both attacking guava in nature (A. ludens and A. striata do not share a single host in nature). It is known that when a fruit fly larva develops in guavas, particularly in unripe fruit, it is exposed to high concentrations of secondary metabolites (Gull et al., 2012; Dos Santos et al., 2017; Monribot-Villanueva et al., 2022), especially tannins and other polyphenols, many of them toxic to the larvae (Lee et al., 2010; Birke et al., 2015; Birke and Aluja, 2018). In this sense, while A. striata prefers to oviposit into totally unripe fruit (Birke et al., 2015) that has still not reached full development (stage four or “player marble size”), A. fraterculus prefers stages five or six, green but fully developed and ripe “turning yellow” stages, respectively (Birke et al., 2015; De Oliveira et al., 2015). Thus, in fruit simultaneously infested by both species, the larvae of A. fraterculus likely ingested pulp that had been “contaminated” by the feces and saliva of larvae of A. striata as the latter started to feed much earlier in the same rearing medium (pulp).
Importantly, only three genera, Komagataeibacter, Weissellla, and Gluconobacter, were differential between A. ludens and A. striata. Of them, Komagataeibacter, an Acetic Acid Bacteria (AAB) was represented in large numbers in A. striata, in lower ones in A. fraterculus and absent in A. ludens when forcibly infesting guava and naturally infesting bitter oranges. This observation suggests a trend from up to low abundance of this bacterial genus related with the maturation stage. The ASVs of Komagataeibacter that we found have high sequence similarity with Komagataeibacter intermedius and K. hansenii. K. intermedius is used for industrial production of bacterial cellulose (Lin et al., 2016; Fernández et al., 2019), and rotten guava can be used for its enrichment and isolation (Lotfy et al., 2021). Besides, many Komagataeibacter species can synthesize enzymes as tannase, polyphenol oxidase, and pectinase, which are used in incomplete oxidation to metabolize a wide range of tannins and polyphenols, as gallic, tannic, gentisic, vanillic, caffeic, syringic, coumaric and ferulic acids (Usha-Rani and Anu-Appaiah, 2012; Chakravorty et al., 2016; Emiljanowicz and Malinowska-Pańczyk, 2019; Vasconcellos et al., 2019; Netzel et al., 2020). Thus, considering the robust tannin-degrading metabolic machinery among Komagataeibacter species (Cannazza et al., 2021), we suggest that this genus could perform important metabolic activities in A. striata and A. fraterculus that allow these flies to successfully develop in guava. We surmise that Komagataeibacter could degrade toxic guava compounds, allowing the development of A. striata and A. fraterculus larvae in this fruit, producing a symbiotic relationship with the benefit of adding a detoxification capacity. In addition to this presumable detoxification role, it is also possible that Komagataeibacter species contribute by favoring the colonization/infestation of A. striata and A. fraterculus of guavas. We hypothesize that this symbiotic relationship helps their host specificity (Myrtaceae), as guava is only infested by relatively few fruit fly species worldwide (White and Elson-Harris, 1992). In contrast, A. ludens establishes symbiotic relationships with a different group of AABs, and cannot acquire or coexist with Komagataeibacter, a fact that we now infer likely impedes its development in the tannin rich guavas.
Related to the above, we found that when A. ludens is forced to attack guavas, even fully ripe ones, the gut microbiota exhibited a clear dysbiotic state. Birke et al. (2015) and Birke and Aluja (2018) had already reported that guavas represented a strict ecological limit to the extreme polyphagy of A. ludens and that when females were forced to lay eggs into guavas, the progeny suffered severe fitness costs, such as very small pupae/adults and delayed ontogeny when compared to optimal hosts such as ones within the Rutaceae. When comparing the gut microbiota of larvae developing in guava versus ones developing in the natural host C. x aurantium, we observed a depletion of members within the Acetobacteraceae, specifically the genera Gluconobacter and Acetobacter, with an enrichment of representatives within the Enterobacteriaceae family, specifically the genera Pantoea and Enterobacter, resulting in a higher Enterobacteriaceae/Acetobacteraceae ratio between the dysbiotic/normal microbiota. A similar dysbiotic pattern was recently reported by Aluja et al. (2021) in the case of A. ludens attacking the marginal host C. pubescens cv. Manzano. We observed a shift in the gut microbiota, with the same increment in the Enterobacteriaceae/Acetobacteraceae ratio.
This shift could be related to the differential pupal weight of larvae developing in C. x aurantium, an optimal host, and C. pubescens, a marginal host, observed by Birke and Aluja (2018). These authors reported a very low pupal weight when A. ludens is forced to develop in P. guajava (9.5 ± 0.2 mg) when compared to the ancestral host, C. edulis (23.02 ± 0.2) and grapefruit (18.2 ± 0.2) (both Rutaceae). Based on the results obtained here on the microbiota of A. ludens larvae developing in P. guajava, as well, as those obtained by Aluja et al. (2021) in the case of A. ludens developing on the poor host C. pubescens, a plausible explanation is the depletion of AABs symbionts in these two hosts.
We note further that C. edulis, one of the purported ancestral hosts of A. ludens, can be considered a “sugar bomb” with high carbohydrate content and low protein levels [carbohydrate/protein ratio of 18.4 (16.6/0.9%)], contrasting to what happens in P. guajava [8.7 (15.8/1.8%)] and C. pubescens [6.3 (6.32/1%) (Morton and Dowling, 1987; Rivera et al., 2010; Birke and Aluja, 2018)]. That is, the latter fruit species have more than two times the amount of protein content than C. edulis, which is a common nutrient trend in native/domesticated fruit (Aluja and Mangan, 2008). Phytophagous specialists mostly prefer hosts that maximize offspring fitness; specificity also guarantees the same nutritional availability generation after generation, producing a “phylogenetic-specific” phytophagia, since phylogenetically related plant species have similar nutritional compounds and similar types of secondary metabolites and volatiles (Birke and Aluja, 2018). On the other hand, the ability to grow adequately in nitrogen poor sources (high C:N ratio) could be key for the polyphagia exhibited by A. ludens, reducing the need for specificity as any host with high carbohydrate content will be adequate, granted there are no toxic secondary metabolites. Thus, we hypothesize that A. ludens lacks a phylogenetic-specific host-fly relationship but could rather exhibit a nutritional-specific host-fly relationship, capable of infesting any host with low toxicity and a high C:N ratio in nutrient composition. The type of bacterial relationships found in the case of A. ludens and C. x aurantium here, plus the ones recently reported by Aluja et al. (2021) in the case of other hosts, lends support to our hypothesis.
The apparent critical role of the Enterobacteriaceae/Acetobacteraceae ratio in the fitness in the of the three tephritid flies studied represents an interesting phenomenon. Many insects that develop in hosts with high C:N ratios overcome the nitrogen deficiency via their gut microbiota (Bar-Shmuel et al., 2020; Ren et al., 2022). The gut microbiota can make the nitrogen available to the insect host via biological nitrogen fixation (BNF), where nitrogen-fixing bacteria, using the nitrogenase complex can transform the atmospheric dinitrogen into ammonia, which can be later assimilated as the non-essential amino acids glutamine and glutamate (Kneip et al., 2007; Ren et al., 2022). This type of symbiosis has been reported in the tephritid flies Ceratitis capitata and Bactrocera tryoni, involving bacteria of the genera Enterobacter and Klebsiella (Murphy et al., 1994; Behar et al., 2005). Here, as noted before, we found high abundance of Acetic Acid Bacteria (AAB) in the three Anastrepha species. AAB’s integrate the Acetobacteraceae family, and the genera Acetobacter, Gluconacetobacter, Gluconobacter, Asaia, and Saccharibacter are known to establish symbiotic interactions with insects within Diptera, Hemiptera, and Hymenoptera developing in sugar rich diets (Crotti et al., 2009, 2010; Bar-Shmuel et al., 2020). To date, among all Acetobacteraceae several representatives of the genera Gluconacetobacter, Acetobacter, Komagataeibacter, Swaminathania, Asaia, and Acetobacter have been reported as nitrogen fixing bacteria (Cavalcante and Dobereiner, 1988; Pedraza, 2008; Reis and dos Teixeira, 2015; Dwivedi, 2020).
We also observed an interesting shift in the gut microbiota of A. ludens, with the genera Gluconobacter and Acetobacter being enriched when larvae developed in C. x aurantium but notably depleted when forcibly infesting P. guajava. This observation is congruent with our recently published work (Aluja et al., 2021), where we show that the infestation of C. pubescens, a marginal/poor host only used in extreme drought conditions, produces a high fitness cost resulting in a depletion of Acetobacteraceae and a concomitant enrichment of Enterobacteriaceae (Aluja et al., 2021). A similar observation has been reported in the mosquitoes Anopheles stephensi and A. gambiae, where the loss of Asaia spp. (an AAB) delays the development of its larvae (Chouaia et al., 2012; Mitraka et al., 2013). The most enriched Enterobacteriaceae when A. ludens forcibly infested P. guajava was Pantoea. This genus comprises a versatile group of species that are common commensals or symbionts of plants and insects but that can also become parasitic or play key functions in habitat restoration and pesticide degradation (Walterson and Stavrinides, 2015; Dutkiewicz et al., 2016a,b). Given the versatile ecology of Pantoea, we could envisage it as a “Jekyll and Hyde” ectosymbiont, that under an A. ludens physiological imbalance condition, starts to proliferate and ultimately develops the dysbiotic condition we detected. In contrast to AAB, Pantoea comprise anaerobic or facultative anaerobic bacteria, thus, a shift between AAB/Pantoea could indicate a shift in the redox condition or oxygen availability for A. ludens, but its mechanism of action did not become obvious or could not be formally disentangled in this study.
Finally, we found a high coverage of ASVs belonging to Wolbachia pipientis in the guts of A. striata and A. fraterculus, but notably the presence of this endosymbiont was almost inexistent in the pulp in which both flies feed on. Wolbachia is a common endosymbiont of insects and its vertical transmission in both fly species has been documented (Martínez et al., 2012; Conte et al., 2019; Mateos et al., 2020); thus, the presence of Wolbachia in pulp likely originated from the guts of the larvae when defecating into the pulp. We also detected Wolbachia with very low coverage in the guts of A. ludens and in the pulp of C. x aurantium, but importantly, this endosymbiont was not found in the pulp of P. guajava when A. ludens forcibly infested this fruit. The origin of Wolbachia in A. ludens is not clear, but according to a previous report (Martínez et al., 2012), this endosymbiont seems unable to infect or prevail in this fly, but additional studies are necessary in this respect.
In conclusion, changes in microbiota composition of the gut of A. striata, A. fraterculus and A. ludens are related to its phytophagous habits and host use capabilities. Beneficial traits from the microbiota are different in A. striata and A. fraterculus compared with A. ludens. In the first two species, the specificity and capability to use a host rich in tannins is likely supported by AABs within Komagateibacter. We thus surmise that this relationship promotes the host specificity observed in nature (Birke et al., 2015), which secures the same nutritional availability in each generation, and excludes competitors that cannot infest P. guajava rife with tannins and other deleterious polyphenols. On the other hand, we now know that the fact that A. ludens cannot effectively develop in guava could be likely related to the adverse effects of toxic metabolites in this fruit that this polyphagous insect cannot metabolize, either because the metabolic machinery is missing, or the appropriate gut microbiota is not present. In this sense, our prediction that the deleterious guava pulp would generate a dysbiotic gut microbiota condition, was confirmed. We suggest that A. ludens harbors a microbiota which mainly ameliorates a poor nitrogen content in its hosts. Such microbiota allows this species to infest many hosts with a deficient nutritional content, particularly low nitrogen content, thus promoting its polyphagia. Further functional studies for the detection of nitrogenase activity are still needed, as well as tests with gnotobiotic flies to determine the specific functions provided by the gut microbiota of each fly species. These, as well as many other outstanding questions related with the role microorganisms possibly play in metabolizing toxic chemicals in plants, offer fertile ground for future research (Hansen and Moran, 2014).
Data availability statement
The raw data presented in this study are available in the NCBI repository (BioProject PRJNA684483). Processed data to replicate the analysis are in the Supplementary material.
Author contributions
MA, AM, and MO-S: conceptualization. MO-S and DC-G: data curation and formal analysis. MA: funding acquisition. MO-S, AA-M, DC-G, and MA: investigation and methodology. MA and AA-M: project administration and resources. DC-G: software and visualization. MA, AA-M, EI-L, DD, and AM: supervision. MO-S, MA, and DC-G: writing—original draft. MA, DC-G, MO-S, AM, AA-M, DD, and EI-L: writing—review and editing. All authors contributed to the article and approved the submitted version.
Funding
MO-S would like to thank CONACyT for the graduate-school scholarship he received throughout his MSc studies (No. 1800821). This study was principally funded with resources from the Mexican Campaña Nacional Contra Moscas de la Fruta [DGSV-SENASICA-SAGARPA (currently SADER)] via the Consejo Nacional Consultivo Fitosanitario (CONACOFI) through projects 41011–2017, 41012-2018, 41013-2019, 80124-2020, and 80147-2021 awarded to MA. Additional funds were provided by the Universidad de Valencia, Valencia, Spain via a Distinguished Professor Fellowship to MA (UV-INV-EPC17-548793) and the Consejo Nacional de Ciencia y Tecnología (CONACyT)—Gobierno del Estado de Veracruz FOMIX grant (Project VER-2017-01-292397) to MA. The project was also supported by the Generalitat Valenciana, Valencia, Spain (Project Prometeo/2018/A/133 to AM). Finally, the logistical and financial support of the Instituto de Ecología, A.C. (INECOL) is also acknowledged.
Acknowledgments
We fully recognize the extraordinary support of Emilio Acosta during field work, including the forced infestation experiments in guava with A. ludens. The technical assistance of Jazmín Ibarra and Alexandro Alonso is also gratefully acknowledged. The support of Enrique Ibarra Laclette’s team for processing the samples in the sequencing laboratory was critical and is also gratefully acknowledged. We also appreciate the support of Gabriel Hernández, Juan Carlos Conde, Violeta Navarro, Andrea Birke, Larissa Guillén, and Rafael Ortega. Finally, we acknowledge the contribution of Jesús Alejandro Zamora Briseño to a few words/lines in the “Abstract,” “Introduction,” and “Materials and methods” sections.
Conflict of interest
The authors declare that the research was conducted in the absence of any commercial or financial relationships that could be construed as a potential conflict of interest.
The reviewer BS-M declared a shared parent affiliation with the author AM to the handling editor at the time of review.
Publisher’s note
All claims expressed in this article are solely those of the authors and do not necessarily represent those of their affiliated organizations, or those of the publisher, the editors and the reviewers. Any product that may be evaluated in this article, or claim that may be made by its manufacturer, is not guaranteed or endorsed by the publisher.
Supplementary material
The Supplementary Material for this article can be found online at: https://www.frontiersin.org/articles/10.3389/fmicb.2022.979817/full#supplementary-material
Footnotes
- ^ https://emea.illumina.com/content/dam/illumina-support/documents/documentation/chemistry_documentation/16s/16s-metagenomic-library-prep-guide-15044223-b.pdf
References
Adams, A. S., Aylward, F. O., Adams, S. M., Erbilgin, N., Aukema, B. H., Currie, C. R., et al. (2013). Mountain pine beetles colonizing historical and naïve host trees are associated with a bacterial community highly enriched in genes contributing to terpene metabolism. Appl. Environ. Microbiol. 79, 3468–3475. doi: 10.1128/AEM.00068-13
Aluja, M. (1994). Bionomics and management of Anastrepha. Annu. Rev. Entomol. 39, 155–178. doi: 10.1146/annurev.en.39.010194.001103
Aluja, M., and Mangan, R. L. (2008). Fruit fly (Diptera: Tephritidae) host status determination: critical conceptual, methodological, and regulatory considerations. Annu. Rev. Entomol. 53, 473–502. doi: 10.1146/annurev.ento.53.103106.093350
Aluja, M., Pérez-Staples, D., Macías-Ordóñez, R., Piñero, J., McPheron, B., and Hernández-Ortiz, V. (2003). Nonhost status of Citrus sinensis cultivar Valencia and C. paradisi cultivar Ruby Red to Mexican Anastrepha fraterculus (Diptera: Tephritidae). J. Econ. Entomol. 96, 1693–1703. doi: 10.1093/jee/96.6.1693
Aluja, M., Pinero, J., Lopez, M., Ruiz, C., Zuñiga, A., Piedra, E., et al. (2000). New host plant and distribution records in Mexico for Anastrepha spp., Toxotrypana curvicauda Gerstacker, Rhagoletis zoqui Bush, Rhagoletis sp., and Hexachaeta sp. (Diptera: Tephritidae). Proc. Entomol. Soc. Wash. 102, 802–815.
Aluja, M., Zamora-Briseño, J. A., Pérez-Brocal, V., Altúzar-Molina, A., Guillén, L., Desgarennes, D., et al. (2021). Metagenomic survey of the highly polyphagous Anastrepha ludens developing in ancestral and exotic hosts reveals the lack of a stable microbiota in larvae and the strong influence of metamorphosis on adult gut microbiota. Front. Microbiol. 12:685937. doi: 10.3389/fmicb.2021.685937
Bar-Shmuel, N., Behar, A., and Segoli, M. (2020). What do we know about biological nitrogen fixation in insects? evidence and implications for the insect and the ecosystem. Insect Sci. 27, 392–403. doi: 10.1111/1744-7917.12697
Behar, A., Yuval, B., and Jurkevitch, E. (2005). Enterobacteria-mediated nitrogen fixation in natural populations of the fruit fly Ceratitis capitata. Mol. Ecol. 14, 2637–2643. doi: 10.1111/J.1365-294X.2005.02615.X
Ben-Yosef, M., Aharon, Y., Jurkevitch, E., and Yuval, B. (2010). Give us the tools and we will do the job: symbiotic bacteria affect olive fly fitness in a diet-dependent fashion. Proc. Royal Soc. B: Biol. Sci. 277, 1545–1552. doi: 10.1098/rspb.2009.2102
Ben-Yosef, M., Pasternak, Z., Jurkevitch, E., and Yuval, B. (2015). Symbiotic bacteria enable olive fly larvae to overcome host defenses. R. Soc. Open Sci. 2:150170. doi: 10.1098/rsos.150170
Birke, A., Acosta, E., and Aluja, M. (2015). Limits to the host range of the highly polyphagous tephritid fruit fly Anastrepha ludens in its natural habitat. Bull. Entomol. Res. 105, 743–753. doi: 10.1017/S0007485315000711
Birke, A., and Aluja, M. (2018). Do mothers really know best? complexities in testing the preference-performance hypothesis in polyphagous frugivorous fruit flies. Bull. Entomol. Res. 108, 674–684. doi: 10.1017/S0007485317001213
Bolyen, E., Rideout, J. R., Dillon, M. R., Bokulich, N. A., Abnet, C. C., Al-Ghalith, G. A., et al. (2019). Reproducible, interactive, scalable and extensible microbiome data science using QIIME 2. Nat. Biotechnol. 37, 852–857. doi: 10.1038/s41587-019-0209-209
Callahan, B. J., McMurdie, P. J., Rosen, M. J., Han, A. W., Johnson, A. J. A., and Holmes, S. P. (2016). DADA2: high-resolution sample inference from Illumina amplicon data. Nat. Methods 13, 581–583. doi: 10.1038/nmeth.3869
Cannazza, P., Rissanen, A. J., Guizelini, D., Losoi, P., Sarlin, E., Romano, D., et al. (2021). Characterization of komagataeibacter isolate reveals new prospects in waste stream valorization for bacterial cellulose production. Microorganisms 9:2230. doi: 10.3390/microorganisms9112230
Capuzzo, C., Firrao, G., Mazzon, L., Squartini, A., and Girolami, V. (2005). “Candidatus Erwinia dacicola”, a coevolved symbiotic bacterium of the olive fly Bactrocera oleae (Gmelin). Int. J. Syst. Evol. Microbiol. 55, 1641–1647. doi: 10.1099/ijs.0.63653-63650
Carroll, L. E., and Wharton, R. A. (1989). Morphology of the immature stages of Anastrepha ludens (Diptera: Tephritidae). Ann. Entomol. Soc. Am. 82, 201–214. doi: 10.1093/AESA/82.2.201
Cavalcante, V. A., and Dobereiner, J. (1988). A new acid-tolerant nitrogen-fixing bacterium associated with sugarcane. Plant Soil 108, 23–31. doi: 10.1007/BF02370096
Cénit, M. C., Matzaraki, V., Tigchelaar, E. F., and Zhernakova, A. (2014). Rapidly expanding knowledge on the role of the gut microbiome in health and disease. Biochim. Biophys. Acta 1842, 1981–1992. doi: 10.1016/j.bbadis.2014.05.023
Chakravorty, S., Bhattacharya, S., Chatzinotas, A., Chakraborty, W., Bhattacharya, D., and Gachhui, R. (2016). Kombucha tea fermentation: microbial and biochemical dynamics. Int. J. Food Microbiol. 220, 63–72.
Chen, B., The, B. S., Sun, C., Hu, S., Lu, X., Boland, W., et al. (2016). Biodiversity and activity of the gut microbiota across the life history of the insect herbivore Spodoptera littoralis. Sci. Rep. 6:29505. doi: 10.1038/srep29505
Chouaia, B., Rossi, P., Epis, S., Mosca, M., Ricci, I., Damiani, C., et al. (2012). Delayed larval development in Anopheles mosquitoes deprived of Asaia bacterial symbionts. BMC Microbiol. 12:S2. doi: 10.1186/1471-2180-12-S1-S2
Conte, C. A., Segura, D. F., Milla, F. H., Augustinos, A., Cladera, J. L., Bourtzis, K., et al. (2019). Wolbachia infection in Argentinean populations of Anastrepha fraterculus sp1: preliminary evidence of sex ratio distortion by one of two strains. BMC Microbiol. 19:289. doi: 10.1186/s12866-019-1652-y
Crotti, E., Damiani, C., Pajoro, M., Gonella, E., Rizzi, A., Ricci, I., et al. (2009). Asaia, a versatile acetic acid bacterial symbiont, capable of cross-colonizing insects of phylogenetically distant genera and orders. Environ. Microbiol. 11, 3252–3264. doi: 10.1111/J.1462-2920.2009.02048.X
Crotti, E., Rizzi, A., Chouaia, B., Ricci, I., Favia, G., Alma, A., et al. (2010). Acetic acid bacteria, newly emerging symbionts of insects. Appl. Environ. Microbiol. 76, 6963–6970. doi: 10.1128/AEM.01336-1310
De Oliveira, F. Q., Boiça Júnior, A. L., Zamboni Costa, M. L., Batista, J. L., Costa, K. Z., and Melges Walder, J. M. (2015). Infestation of guava by Anastrepha fraterculus (Diptera: Tephritidae): preferred ripening stages and influence of fruit peel coloration. Turk. J. Agric. For. 39, 1–9. doi: 10.3906/tar-1312-1320
Dos Santos, W. N. L., da Silva, Sauthier, M. C., dos Santos, A. M. P., de Andrade Santana, D., Almeida Azevedo, R. S., et al. (2017). Simultaneous determination of 13 phenolic bioactive compounds in guava (Psidium guajava L.) by HPLC-PAD with evaluation using PCA and Neural Network Analysis (NNA). Microchem. J. 133, 583–592. doi: 10.1016/j.microc.2017.04.029
Drobya, S., and Wisniewskib, M. (2018). The fruit microbiome: a new frontier for postharvest biocontrol and postharvest biology. Postharvest Biol. Technol. 140, 107–112. doi: 10.1016/j.postharvbio.2018.03.004
Dutkiewicz, J., Mackiewicz, B., Lemieszek, M. K., Golec, M., and Milanowski, J. (2016a). Pantoea agglomerans: a mysterious bacterium of evil and good. Part III. deleterious effects: infections of humans, animals and plants. Agric. Environ. Med. 23, 197–205. doi: 10.5604/12321966.1203878
Dutkiewicz, J., Mackiewicz, B., Lemieszek, M. K., Golec, M., and Milanowski, J. (2016b). Pantoea agglomerans: a mysterious bacterium of evil and good. Part IV. beneficial effects. Ann. Agric. Environ. Med. 23, 206–222. doi: 10.5604/12321966.1203879
Dwivedi, M. (2020). “Gluconobacter,” in Beneficial Microbes in Agro-Ecology, eds N. Amaresan, M. Senthil-Kumar, K. Annpurna, K. Kumar, and A. Sankaranaranyan (Amsterdam: Elsevier).
Emiljanowicz, K. E., and Malinowska-Pańczyk, E. (2019). Kombucha from alternative raw materials - the review. Crit. Rev. Food Sci. Nutr. 60, 3185–3194. doi: 10.1080/10408398.2019.1679714
Fernández, J., Morena, A. G., Valenzuela, S. V., Pastor, F. I. J., Díaz, P., and Martínez, J. (2019). Microbial cellulose from a Komagataeibacter intermedius strain isolated from commercial wine vinegar. J. Polym. Environ. 27, 956–967. doi: 10.1007/S10924-019-01403-4/TABLES/4
Gales, A., Chatellard, L., Abadie, M., Bonnafous, A., Auer, L., Carrère, H., et al. (2018). Screening of phytophagous and xylophagous insect gut microbiota abilities to degrade lignocellulose in bioreactor. Front. Microbiol. 9:2222. doi: 10.3389/FMICB.2018.02222
Gallo-Franco, J. J., and Toro-Perea, N. (2020). Variations in the bacterial communities in Anastrepha obliqua (Diptera: Tephritidae) according to the insect life stage and host plant. Curr. Microbiol. 77, 1283–1291. doi: 10.1007/s00284-020-01939-y
Gull, J., Sultana, B., Anwar, F., Naseer, R., Ashraf, M., and Ashrafuzzaman, M. (2012). Variation in antioxidant attributes at three ripening stages of guava (Psidium guajava L.) fruit from different geographical regions of Pakistan. Molecules 17, 3165–3180. doi: 10.3390/molecules17033165
Gutierrez-Rosales, F., Romero, M. P., Casanovas, M., Motilva, M. J., and Mínguez-Mosquera, M. I. (2012). β-Glucosidase involvement in the formation and transformation of oleuropein during the growth and development of olive fruits (Olea europaea L. cv. Arbequina) grown under different farming practices. J. Agric. Food Chem. 60, 4348–4358. doi: 10.1021/jf205209y
Hammer, T. J., and Bowers, M. D. (2015). Gut microbes may facilitate insect herbivory of chemically defended plants. Oecologia 179, 1–14. doi: 10.1007/s00442-015-3327-3321
Hansen, A. K., and Moran, N. A. (2014). The impact of microbial symbionts on host plant utilization by herbivorous insects. Mol. Ecol. 23, 1473–1496. doi: 10.1111/mec.12421
Hernandez-Ortiz, A. M. (1993). Listado de especies del género Neotropical Anastrepha (Diptera: Tephritidae) con notas. Folia Entomol. Mex. 88, 89–101.
Huang, X., Ren, J., Li, P., Feng, S., Dong, P., and Ren, M. (2020). The potential of microbial endophytes to enhance resistance to postharvest diseases of fruit and vegetables. J. Sci. Food Agric. 101, 1744–1757. doi: 10.1002/jsfa.10829
Katoh, K. (2002). MAFFT: a novel method for rapid multiple sequence alignment based on fast Fourier transform. Nucleic Acids Res. 30, 3059–3066. doi: 10.1093/nar/gkf436
Klindworth, A., Pruesse, E., Schweer, T., Peplies, J., Quast, C., Horn, M., et al. (2013). Evaluation of general 16S ribosomal RNA gene PCR primers for classical and next-generation sequencing-based diversity studies. Nucleic Acids Res. 41:e1. doi: 10.1093/nar/gks808
Kneip, C., Lockhart, P., Voß, C., and Maier, U. G. (2007). Nitrogen fixation in eukaryotes - new models for symbiosis. BMC Evol. Biol. 7:55. doi: 10.1186/1471-2148-7-55
Kwong, W. K., and Moran, N. A. (2016). Gut microbial communities of social bees. Nat. Rev. Microbiol. 14, 374–384. doi: 10.1038/nrmicro.2016.43
Lee, S., Choi, H. K., Cho, S. K., and Kim, Y. S. (2010). Metabolic analysis of guava (Psidium guajava L.) fruits at different ripening stages using different data-processing approaches. J. Chromatogr. B. 878, 2983–2988. doi: 10.1016/j.jchromb.2010.09.003
Lin, S. P., Huang, Y. H., Hsu, K., Di Lai, Y. J., Chen, Y. K., and Cheng, K. C. (2016). Isolation and identification of cellulose-producing strain Komagataeibacter intermedius from fermented fruit juice. Carbohydr. Polym. 151, 827–833. doi: 10.1016/j.carbpol.2016.06.032
Lotfy, V. F., Basta, A. H., Abdel-Monem, M. O., and Abdel-Hamed, G. Z. (2021). Utilization of bacteria in rotten Guava for production of bacterial cellulose from isolated and protein waste. Carbohydr. Polym. Technol. Appl. 2:100076. doi: 10.1016/J.CARPTA.2021.100076
Malacrinò, A., Mosca, S., Li Destri, Nicosia, M. G., Agosteo, G. E., and Schena, L. (2022). Plant genotype shapes the bacterial microbiome of fruits, leaves, and soil in olive plants. Plants 11:613. doi: 10.3390/plants11050613
Martínez, H., Toledo, J., Liedo, P., and Mateos, M. (2012). Survey of heritable endosymbionts in Southern Mexico populations of the fruit fly species Anastrepha striata and A. ludens. Curr. Microbiol. 65, 711–718. doi: 10.1007/s00284-012-0223-223
Mateos, M., Martinez Montoya, H., Lanzavecchia, S. B., Conte, C., Guillén, K., Morán-Aceves, B. M., et al. (2020). Wolbachia pipientis associated with tephritid fruit fly pests: from basic research to applications. Front. Microbiol. 11:1080. doi: 10.3389/fmicb.2020.01080
McMurdie, P. J., and Holmes, S. (2013). Phyloseq: an R package for reproducible interactive analysis and graphics of microbiome census data. PLoS One 8:e61217. doi: 10.1371/journal.pone.0061217
Mitraka, E., Stathopoulos, S., Siden-Kiamos, I., Christophides, G. K., and Louis, C. (2013). Asaia accelerates larval development of Anopheles gambiae. Pathog. Glob. Health 107, 305–311. doi: 10.1179/2047773213Y.0000000106
Monribot-Villanueva, J. L., Altúzar-Molina, A., Aluja, M., Zamora-Briseño, J. A., Elizalde-Contreras, J. M., Bautista-Valle, M. V., et al. (2022). Integrating proteomics and metabolomics approaches to elucidate the ripening process in white Psidium guajava. Food Chem. 367:130656. doi: 10.1016/j.foodchem.2021.130656
Murphy, K. M., Teakle, D. S., and MacRae, I. C. (1994). Kinetics of colonization of adult Queensland Fruit Flies (Bactrocera tryoni) by dinitrogen-fixing alimentary tract bacteria. Appl. Environ. Microbiol. 60, 2508–2517. doi: 10.1128/AEM.60.7.2508-2517.1994
Netzel, G., Mikkelsen, D., Flanagan, B. M., Netzel, M. E., Gidley, M. J., and Williams, B. A. (2020). Metabolism of black carrot polyphenols during in vitro fermentation is not affected by cellulose or cell wall association. Foods 9, 1911–1911. doi: 10.3390/FOODS9121911
Norrbom, A. L., Barr, N. B., Kerr, P., and Mengual, X. (2018). Case 3772 - Anastrepha Schiner, 1868 (Insecta, Diptera, Tephritidae): proposed precedence over Toxotrypana Gerstaecker, 1860. Bull. Zool. Nomencl. 75, 165–169. doi: 10.21805/bzn.v75.a033
Oksanen, J. (2015). Multivariate analysis of ecological communities in R: vegan tutorial. R. Doc. 43, 1–43. doi: 10.1016/0169-5347(88)90124-90123
Palemon-Alberto, F., Palacios-Torres, R. E., Ruiz-Montiel, C., Birke-Biewendt, A. B., Flores-Maldonado, K. Y., and Gasca-Corona, L., (2021). New records and biological notes of the guava weevil in Mexico. Southwest Entomol. 46, 515–520.
Paulson, J. N., Stine, O. C., Bravo, H. C., and Pop, M. (2013). Differential abundance analysis for microbial marker-gene surveys. Nat. Methods 10, 1200–1202. doi: 10.1038/nmeth.2658
Pedraza, R. O. (2008). Recent advances in nitrogen-fixing acetic acid bacteria. Int. J. Food Microbiol. 125, 25–35. doi: 10.1016/J.IJFOODMICRO.2007.11.079
Price, M. N., Dehal, P. S., and Arkin, A. P. (2010). FastTree 2–approximately maximum-likelihood trees for large alignments. PLoS One 5:e9490. doi: 10.1371/journal.pone.0009490
Quast, C., Pruesse, E., Yilmaz, P., Gerken, J., Schweer, T., Yarza, P., et al. (2013). The SILVA ribosomal RNA gene database project: improved data processing and web-based tools. Nucleic Acids Res. 41, D590–D596. doi: 10.1093/nar/gks1219
Reis, V. M., and dos Teixeira, K. R. S. (2015). Nitrogen fixing bacteria in the family Acetobacteraceae and their role in agriculture. J. Basic Microbiol. 55:931. doi: 10.1002/JOBM.201400898
Ren, X., Guo, R., Akami, M., and Niu, C. (2022). Nitrogen acquisition strategies mediated by insect symbionts: a review of their mechanisms, methodologies, and Ccase studies. Insects 13:84. doi: 10.3390/INSECTS13010084
Rivera, G., Bocanegra-García, V., and Monge, A. (2010). Traditional plants as source of functional foods: a review. CyTA-J. Food 8, 159–167. doi: 10.1080/19476330903322978
Rognes, T., Flouri, T., Nichols, B., Quince, C., and Mahé, F. (2016). VSEARCH: a versatile open source tool for metagenomics. PeerJ 4:e2584. doi: 10.7717/peerj.2584
Salgueiro, J., Pimper, L. E., Segura, D. F., Milla, F. H., Russo, R. M., Asimakis, E., et al. (2020). Gut bacteriome analysis of Anastrepha fraterculus sp. 1 during the early steps of laboratory colonization. Front. Microbiol. 11:570960. doi: 10.3389/FMICB.2020.570960
Salles, L. A. (2000). Biologia e Ciclo de Vida de Anastrepha fraterculus Moscas-das-frutas de Importância Econômica no Brasil: Conhecimento Básico e Aplicado. Holos: Ribeirão Preto.
Segata, N., Izard, J., Waldron, L., Gevers, D., Miropolsky, L., Garrett, W. S., et al. (2011). Metagenomic biomarker discovery and explanation. Genome Biol. 12:R60. doi: 10.1186/gb-2011-12-6-r60
Shin, N. R., Whon, T. W., and Bae, J. W. (2015). Proteobacteria: microbial signature of dysbiosis in gut microbiota. Trends Biotechnol. 33, 496–503. doi: 10.1016/j.tibtech.2015.06.011
Shukla, S. P., and Beran, F. (2020). Gut microbiota degrades toxic isothiocyanates in a flea beetle pest. Mol. Ecol. 29, 4692–4705. doi: 10.1111/mec.15657
Singh, P., Leppla, N. C., and Adams, F. (1988). Feeding behavior and dietary substrates for rearing larvae of the Caribbean Fruit Fly, Anastrepha suspensa. Florida Entomol. 71:380. doi: 10.2307/3495449
Sivinski, J., Aluja, M., Piñero, J., and Ojeda, M. (2004). Novel analysis of spatial and temporal patterns of resource use in a group of tephritid flies of the genus Anastrepha. Ann. Entomol. Soc. Am. 97, 504–512.
Steck, G. J., Carroll, L. E., Celedonio-Hurtado, H., and Guillen-Aguilar, J. (1990). Methods for identification of Anastrepha larvae (Diptera: Tephritidae), and key to 13 species. Proc. Entomol. Soc. Wash. 92, 333–346.
Usha-Rani, M., and Anu-Appaiah, K. A. (2012). Gluconacetobacter hansenii UAC09-mediated transformation of polyphenols and pectin of coffee cherry husk extract. Food Chem. 130, 243–247. doi: 10.1016/j.foodchem.2011.07.021
Vasconcellos, V. M., Farinas, C. S., Ximenes, E., Slininger, P., and Ladisch, M. (2019). Adaptive laboratory evolution of nanocellulose-producing bacterium. Biotechnol. Bioeng. 116, 1923–1933. doi: 10.1002/BIT.26997
Ventura, C., Briones-Roblero, C. I., Hernández, E., Rivera-Orduña, F. N., and Zúñiga, G. (2018). Comparative analysis of the gut bacterial community of four Anastrepha fruit flies (Diptera: Tephritidae) based on pyrosequencing. Curr. Microbiol. 75, 966–976. doi: 10.1007/s00284-018-1473-1475
Walterson, A. M., and Stavrinides, J. (2015). Pantoea: insights into a highly versatile and diverse genus within the Enterobacteriaceae. FEMS Microbiol. Rev. 39, 968–984. doi: 10.1093/femsre/fuv027
Wassermann, B., Ahmed Abdelfattah, A., Müller, H., Korsten, L., and Berg, G. (2022). The microbiome and resistome of apple fruits alter in the post-harvest period. Environ. Microbiome 17:10. doi: 10.1186/s40793-022-00402-408
White, I. M., and Elson-Harris, M. M. (1992). Fruit flies of Economic Significance: Their Identification and Bionomics. London: CAB International.
Wilkinson, L. (2011). ggplot2: elegant graphics for data analysis by WICKHAM, H. Biometrics 67, 678–679. doi: 10.1111/j.1541-0420.2011.01616.x
Wu, H. J., and Wu, E. (2012). The role of gut microbiota in immune homeostasis and autoimmunity. Gut Microbes 3, 4–14. doi: 10.4161/gmic.19320
Yun, J. H., Roh, S. W., Whon, T. W., Jung, M. J., Kim, M. S., Park, D. S., et al. (2014). Insect gut bacterial diversity determined by environmental habitat, diet, developmental stage, and phylogeny of host. Appl. Environ. Microbiol. 80, 5254–5264. doi: 10.1128/AEM.01226-14
Zhang, H., Adwoa-Serwah-Boateng, N., Ngolong-Ngea, G. L., Hetong-Lin, Y. S., Yang, Q., Wang, K., et al. (2021). Unravelling the fruit microbiome: the key for developing effective biological control strategies for postharvest diseases. Compr. Rev. Food Sci. Food Saf. 20, 4906–4930. doi: 10.1111/1541-4337.12783
Keywords: Anastrepha, Psidium guajava, Acetobacteraceae, 16S rRNA, gut microbiota, gut dysbiosis, microbe-tephritid interactions
Citation: Ochoa-Sánchez M, Cerqueda-García D, Moya A, Ibarra-Laclette E, Altúzar-Molina A, Desgarennes D and Aluja M (2022) Bitter friends are not always toxic: The loss of acetic acid bacteria and the absence of Komagataeibacter in the gut microbiota of the polyphagous fly Anastrepha ludens could inhibit its development in Psidium guajava in contrast to A. striata and A. fraterculus that flourish in this host. Front. Microbiol. 13:979817. doi: 10.3389/fmicb.2022.979817
Received: 28 June 2022; Accepted: 29 August 2022;
Published: 28 September 2022.
Edited by:
George Tsiamis, University of Patras, GreeceReviewed by:
Arturo Vera-Ponce de León, Norwegian University of Life Sciences, NorwayBeatriz Sabater-Munoz, Polytechnic University of Valencia, Spain
Sherif Elnagdy, Cairo University, Egypt
Copyright © 2022 Ochoa-Sánchez, Cerqueda-García, Moya, Ibarra-Laclette, Altúzar-Molina, Desgarennes and Aluja. This is an open-access article distributed under the terms of the Creative Commons Attribution License (CC BY). The use, distribution or reproduction in other forums is permitted, provided the original author(s) and the copyright owner(s) are credited and that the original publication in this journal is cited, in accordance with accepted academic practice. No use, distribution or reproduction is permitted which does not comply with these terms.
*Correspondence: Daniel Cerqueda-García, ZGFuaWVsLmNlcnF1ZWRhQGluZWNvbC5teA==; Martín Aluja, bWFydGluLmFsdWphQGluZWNvbC5teA==