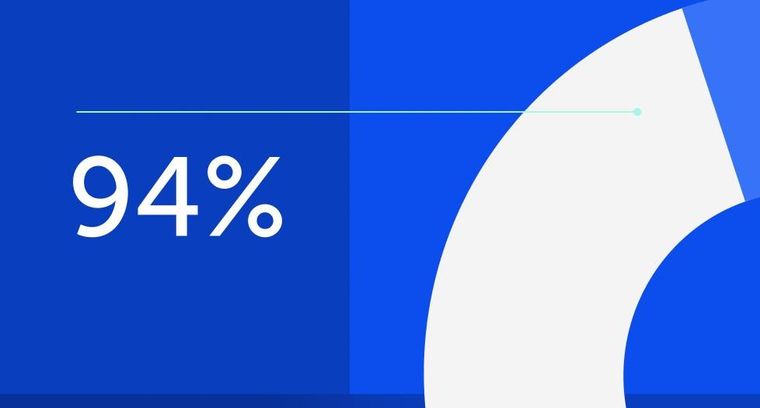
94% of researchers rate our articles as excellent or good
Learn more about the work of our research integrity team to safeguard the quality of each article we publish.
Find out more
ORIGINAL RESEARCH article
Front. Microbiol., 05 August 2022
Sec. Terrestrial Microbiology
Volume 13 - 2022 | https://doi.org/10.3389/fmicb.2022.978296
This article is part of the Research TopicRising Stars in Terrestrial Microbiology: 2022View all 9 articles
The importance of acetogens for H2 turnover and overall anaerobic degradation in peatlands remains elusive. In the well-studied minerotrophic peatland fen Schlöppnerbrunnen, H2-consuming acetogens are conceptualized to be largely outcompeted by iron reducers, sulfate reducers, and hydrogenotrophic methanogens in bulk peat soil. However, in root zones of graminoids, fermenters thriving on rhizodeposits and root litter might temporarily provide sufficient H2 for acetogens. In the present study, root-free peat soils from around the roots of Molinia caerulea and Carex rostrata (i.e., two graminoids common in fen Schlöpnnerbrunnen) were anoxically incubated with or without supplemental H2 to simulate conditions of high and low H2 availability in the fen. In unsupplemented soil treatments, H2 concentrations were largely below the detection limit (∼10 ppmV) and possibly too low for acetogens and methanogens, an assumption supported by the finding that neither acetate nor methane substantially accumulated. In the presence of supplemental H2, acetate accumulation exceeded CH4 accumulation in Molinia soil whereas acetate and methane accumulated equally in Carex soil. However, reductant recoveries indicated that initially, additional unknown processes were involved either in H2 consumption or the consumption of acetate produced by H2-consuming acetogens. 16S rRNA and 16S rRNA gene analyses revealed that potential acetogens (Clostridium, Holophagaceae), methanogens (Methanocellales, Methanobacterium), iron reducers (Geobacter), and physiologically uncharacterized phylotypes (Acidobacteria, Actinobacteria, Bacteroidetes) were stimulated by supplemental H2 in soil treatments. Phylotypes closely related to clostridial acetogens were also active in soil-free Molinia and Carex root treatments with or without supplemental H2. Due to pronounced fermentation activities, H2 consumption was less obvious in root treatments, and acetogens likely thrived on root organic carbon and fermentation products (e.g., ethanol) in addition to H2. Collectively, the data highlighted that in fen Schlöppnerbrunnen, acetogens are associated to graminoid roots and inhabit the peat soil around the roots, where they have to compete for H2 with methanogens and iron reducers. Furthermore, the study underscored that the metabolically flexible acetogens do not rely on H2, potentially a key advantage over other H2 consumers under the highly dynamic conditions characteristic for the root-zones of graminoids in peatlands.
Acetogens are a polyphyletic group of anaerobes that can use the reductive acetyl-CoA pathway for dissimilation (Drake et al., 2008; Ragsdale, 2008). During hydrogenotrophic acetogenesis H2 is used as electron donor (4H2 + CO2 → CH3COO– + H+) whereas organic electron donors (e.g., glucose [C6H12O6 → 3CH3COO– + 3H+] or ethanol [2CH3CH2OH + 2CO2 → 3CH3COO– + 3H+]) are used during organotrophic acetogenesis (Lever, 2012; Schuchmann and Müller, 2014, 2016). Considering their metabolic versatility, it is not surprising that acetogens were isolated from various ecosystems including peatlands (Kotsyurbenko et al., 1995; Simankova et al., 2000; Drake et al., 2006; Gößner et al., 2008). Peatlands are waterlogged soil ecosystems that are of global relevance, especially because of their function as sinks for CO2 and sources for CH4 (Aselmann and Crutzen, 1989; Yu et al., 2010; Abdalla et al., 2016; Harenda et al., 2018). Despite recent findings showing that acetogens contribute to the production of acetate in some peatlands, their ecological significance in these ecosystems is still conceptualized rather than resolved (Drake et al., 2009; Hunger et al., 2011; Hädrich et al., 2012; Ye et al., 2014; Kotsyurbenko et al., 2019).
The marked accumulation of acetate and increasing relative abundances of acetogenic taxa in peat soil incubations with supplemental H2 suggested that acetogens can successfully compete for H2 when it is available at sufficiently high concentrations in peatlands (Kotsyurbenko et al., 1996; Bräuer et al., 2004; Wüst et al., 2009; Hädrich et al., 2012; Hunger et al., 2015). However, at the low H2 concentrations that are characteristic for bulk peat soil, acetogens are conceptualized to be outcompeted by methanogens and other H2 consumers with lower H2 thresholds (Drake et al., 2009; Estop-Aragonés et al., 2013; Kotsyurbenko et al., 2019). The competitiveness of acetogens increases at low temperatures predominating in northern peatlands (Conrad and Wetter, 1990; Nozhevnikova et al., 1994; Kotsyurbenko et al., 2001; Metje and Frenzel, 2005, 2007; Lever, 2012). Furthermore, it was suggested that acetogens may thrive in microenvironments within the peat soil in which H2 concentrations might be higher than in the surrounding bulk peat (Hädrich et al., 2012; Ye et al., 2014; Schmidt et al., 2016).
The rootzones of graminoids (i.e., grass-like wetland plants) may represent such microenvironments suited for peat acetogens. Graminoids like Carex rostrata (bottle sedge; hereafter Carex) and Molinia caerulea (purple moor grass; hereafter Molinia) are common especially in minerotrophic peatlands (i.e., fens; Eurola et al., 1984; Končalová, 1990). Recently, product profiles in Carex and Molinia root treatments with or without fen soil showed that H2, formed during the fermentation of root-derived organic carbon, accumulated to 0.7–4.5 mM (0.8–5.1 kPa); thus, sufficiently high to support hydrogenotrophic acetogenesis (Meier et al., 2021). In another study, formate [or formate-derived H2 (i.e., H2 released during the oxidation of formate by formate hydrogenlyase-containing taxa)], which is likely released during the fermentative degradation of root exudates (Koelbener et al., 2010), stimulated acetate production in soil-free Carex root treatments as well as root-free fen soil treatments, suggesting that acetogens are associated to graminoid roots and inhabit the soil surrounding the roots (Hunger et al., 2016).
Based on the findings of the two earlier studies (Hunger et al., 2016; Meier et al., 2021), roots and soil from the root zones of Carex and Molinia were incubated separately, and the effects of supplemental H2 on the product profiles and prokaryotic communities were evaluated in order to address the following hypotheses: (1) acetogens are associated to the roots of fen graminoids and inhabit the peat soil surrounding these roots; (2) acetogens can thrive on H2 derived from the fermentation of root organic carbon; (3) in the absence of root-derived organic carbon, acetogens are outcompeted for endogenous H2 by H2 consumers with lower thresholds.
Fen Schlöppnerbrunnen is a moderately acidic (pH 4.3–5.6), minerotrophic, CH4-emitting fen that is completely overgrown with M. caerulea, intermingled with patches of sedges (e.g., C. rostrata), rushes, and mosses; the fen is located in the Lestenbach catchment in the Fichtelgebirge (50°07′53″N and 11°52′51″E), Germany (Hamberger et al., 2008; Reiche et al., 2009; Hädrich et al., 2012).
The sampling of roots and soil as well as the setup of anoxic incubations largely resembled that of previous studies (Hunger et al., 2016; Meier et al., 2021) and is summarized in Supplementary Figure 1. Carex roots and Carex soil (i.e., peat soil from around the Carex roots) were sampled in July 2016; Molinia roots and Molinia soil (i.e., peat soil from around the Molinia roots) were sampled in July 2018. Samples were transported to the lab in airtight sterile plastic bags on ice and transferred in an anoxic chamber (100% N2 atmosphere, Mecaplex, Grenchen, Switzerland). Roots were separated from the soil and washed gently with sterile anoxic water to remove residual soil particles; soil was sieved to obtain soil largely devoid of roots, termed root-free soil. One gram fresh weight of roots or soil were transferred in 27 ml glass tubes and 9 ml of anoxic mineral solution (Hunger et al., 2015) were added to make up a total volume of 10 ml. Tubes were sealed with butyl-rubber stoppers and flushed with 100% N2. Approximately 10 μmol H2 per ml liquid volume was added to H2 treatments, whereas no H2 was added to unsupplemented treatments. The following abbreviations are used for H2 treatments and unsupplemented treatments: SUC, unsupplemented Carex soil; SHC, H2 supplemented Carex soil; SUM, unsupplemented Molinia soil; SHM, H2 supplemented Molinia soil; RUC, unsupplemented Carex roots; RHC, H2 supplemented Carex roots; RUM, unsupplemented Molinia roots; and RHM, H2 supplemented Molinia roots. All treatments were setup in triplicates and incubated in the dark at 15°C for 17 days (without a pre-incubation).
The headspaces and liquid phases of H2 treatments and unsupplemented treatments were sampled regularly during the incubation using sterile syringes. The devices and instrumental setup used for (a) gas chromatographic analysis of headspace gasses, (b) high performance liquid chromatography analysis of organic acids and ethanol, and (c) pH measurements were those recently described in detail (Meier et al., 2021). Amounts of CO2 (including pH-dependent amounts of bicarbonate), H2, and CH4 in the headspaces and liquid phases were calculated as described before (Meier et al., 2021), and molar concentrations of gasses were calculated by dividing total amounts of a gas (in μmol) by 9.5 ml (the volume of the liquid phase after initial sampling). Dry weight contents (determined by weighing before and after drying at 60°C for 72 h) of the roots and soil in the three experiments were as follows: Carex soil/roots, 11%/13%; Molinia soil/roots, 16%/36%. Millimolar concentrations of gasses, organic acids, and ethanol can be converted to μmol per g dry weight by multiplying with 86/73 for Carex soil/roots and 59/26 for Molinia soil/roots.
Reductant recoveries were calculated to determine whether the enhanced accumulation of acetate and methane in H2 supplemented soil treatments compared to unsupplemented soil treatments could be explained by the consumption of exogenous H2. Reductant recoveries for acetate (RA) and CH4 (RM) were calculated according to the following Equations.
In the equations above, eight refers to the number of reducing equivalents per molecule acetate and methane; two refers to the reducing equivalents per molecule H2. [Ah], [Au], [Mh], [Mu], [Hh], and [Hu] are the concentrations of acetate in H2 supplemented soil treatments, acetate in unsupplemented soil treatments, CH4 in H2 supplemented soil treatments, CH4 in unsupplemented soil treatments, H2 in H2 supplemented soil treatments, and H2 in unsupplemented soil treatments, respectively, at the start (t1) and the end (t2) of the respective time frame.
Gibb’s free energies (ΔG) were calculated using the Nernst and Van’t Hoff equations (Conrad and Wetter, 1990).
Three replicate nucleic acid extractions were performed with fresh washed roots and sieved soil to analyze the in situ microbial community at the time of sampling. At the end of the 17-day incubation, nucleic acids were extracted from all replicates of root and soil treatments separately. Samples of soil treatments were centrifuged at 16,000 × g for 15 min at 4°C (1-15-K Sartorius, Göttingen, Germany) to retrieve pelleted soil suitable for nucleic acid extraction. Nucleic acid extraction, digestion of DNA (to retrieve pure RNA) or RNA (to retrieve pure DNA), and cDNA synthesis were performed as described before (Meier et al., 2021).
PCR amplification, Illumina MiSeq amplicon sequencing, and data processing were performed as stated elsewhere (Zeibich et al., 2019; Meier et al., 2021). In short, primers Pro341f (5′-CCT ACG GGN BGC ASC AG-3′) and Pro805r (5′-GAC TAC NVG GGT ATC TAA TCC-3′; Takahashi et al., 2014) were used for 16S rRNA amplicon generation, quality filtered sequences were clustered using a 97% similarity cut-off, and chloroplast- and mitochondria-related sequences were excluded from further analyses.
Linear discriminant analysis effect size (LEfSe; Segata et al., 2011) was conducted with 16S rRNA and 16S rRNA gene sequence data separately for Carex and Molinia soil treatments. Phylotypes that fulfilled the following three criteria based on 16S rRNA or 16S rRNA gene sequence data were designated as “stimulated” by supplemental H2: Phylotypes (a) were significantly (P ≤ 0.05; Kruskal–Wallis test) more abundant in H2 supplemented soil treatments compared to unsupplemented soil treatments, (b) had an effect size (LDA score) of ≥3, and (c) were on average at least twice as abundant in H2 supplemented soil treatments as in unsupplemented soil treatments (the proof of criteria c is not implemented in LEfSe and was performed manually in order to eliminate phylotypes that were only slightly more abundant in H2 supplemented soil treatments compared to unsupplemented soil treatments).
One-sided Wilcoxon rank sum test implemented in R1 was used to identify statistically significant differences (P value of ≤ 0.05) between the amounts of CH4 and acetate formed in H2 treatments and unsupplemented treatments during the 17-day incubation. Differences in the overall composition of prokaryotic communities before incubation and after incubation in H2 treatments and unsupplemented treatments were visualized by non-metric multidimensional scaling (NMDS) based on the Bray-Curtis distance matrix calculated using the software Past32 (Hammer et al., 2001).
Sequences were deposited at the European Nucleotide Archive under study numbers PRJEB37304 and PRJEB37863 for Carex and Molinia experiments, respectively. Representative sequences of phylotypes stimulated by supplemental H2 in soil treatments were deposited under the accession numbers LR792771-LR792783 and LR792811-LR792818.
In the fen Schlöppnerbrunnen, excess H2 formed by root associated fermenters might occasionally diffuse into the peat soil surrounding graminoid roots where it might stimulate H2 consumers that relay on H2 concentrations higher than the 0.2–28 nmol l–1 dissolved H2 (corresponds to a H2 partial pressure of approximately 0.03–4 Pa) observed in bulk peat soil in situ (Knorr et al., 2009; Estop-Aragonés et al., 2013; Hunger et al., 2016; Meier et al., 2021). To simulate contrasting H2 availabilities in the peat soil, soil treatments with supplemental H2 und unsupplemented soil treatments were conducted.
Supplemental H2 was consumed linearly in H2 supplemented soil treatments at rates of 0.45 mM H2 d–1 (R2 = 0.98) for Carex soil (Treatment SHC) and 0.48 mM H2 d–1 (R2 = 0.97) for Molinia soil (Treatment SHM; Figure 1A). That H2 was consumed without delay suggested that H2-consuming microbes in the root zones of both plants were poised to respond quickly to the sudden availability of H2 at high concentrations. Initially, the consumption of H2 was in stark contrast to the accumulation of little acetate and CH4 in treatments SHC and SHM (Figure 1A), and reductant recoveries confirmed that both products accounted for only a small fraction of the H2 that was consumed within the first 6 to 7 days (Table 1). Thus, neither hydrogenotrophic acetogenesis nor hydrogenotrophic methanogenesis seemed to be main H2-consuming processes during the first stage of incubation. With time acetate and CH4 accumulation accelerated (Figure 1A), and during the second stage of incubation, both products collectively accounted for 87.4 and 89.6% of consumed exogenous H2 in Treatments SHC and SHM, respectively, (Table 1), pointing toward hydrogenotrophic acetogenesis and hydrogenotrophic methanogenesis as the main H2-consuming processes between day 6 or 7 and day 17. CO2 is the electron acceptor of both processes (Thauer et al., 2008; Schuchmann and Müller, 2014), and its subsequent consumption toward the end of the incubation is in line with ongoing hydrogenotrophic acetogenesis and hydrogenotrophic methanogenesis in Treatments SHC and SHM (Figure 1A). Similar amounts of CH4 and acetate were formed in Treatment SHC, whereas at least three times more acetate than CH4 accumulated in Treatment SHM (Figure 1A). Small amounts of propionate accumulated toward the end of the incubation in H2 supplemented soil treatments (Supplementary Figure 2), a finding in line with the formation of propionate in formate treatments of fen Schlöppnerbrunnen soil (Hunger et al., 2011). In unsupplemented Molinia soil (Treatment SUM) low amounts of acetate and CH4 accumulated toward the end of the incubation, and in unsupplemented Carex soil (Treatment SUC) neither acetate nor CH4 accumulated (Figure 1A).
Figure 1. Concentrations of H2, CO2, CH4, and acetate in soil treatments (A) and root treatments (B). Treatment identifiers: SUC, unsupplemented Carex soil; SHC, H2 supplemented Carex soil; SUM, unsupplemented Molinia soil; SHM, H2 supplemented Molinia soil; RUC, unsupplemented Carex roots; RHC, H2 supplemented Carex roots; RUM, unsupplemented Molinia roots; RHM, H2 supplemented Molinia roots. Symbols: circles, replicate 1; triangles, replicate 2; squares, replicate 3. The asterisks indicate significant differences (one-sided Wilcoxon rank sum test; P ≤ 0.05) between the amounts of CH4 and acetate formed in H2 treatments and unsupplemented treatments during incubation. See Supplementary Figure 2 for ethanol, butyrate, propionate, and pH.
The collective data suggested that H2 consuming acetogens and methanogens inhabiting the peat soil surrounding Carex and Molinia roots were substrate limited in unsupplemented soil treatments and became increasingly important in H2 supplemented soil treatments. While acetogenic and methanogenic potentials were similar in H2 treatments with Carex soil, acetogenesis was found to exceed methanogenesis in H2 treatments with Molinia soil. In any way, anaerobic respiratory microbes that use electron acceptors others than CO2 were presumably involved in the mineralization of peat organic carbon and in the consumption of supplemental H2. It cannot be excluded that such respiratory processes also consumed acetate produced by acetogens, and aceticlastic methanogenesis is another possible sink for acetate.
Non-metric multidimensional scaling analyses of 16S rRNA and 16S rRNA gene phylotypes (≥97% sequence similarity), alpha diversity parameters, and phylum/family level-based community profiling collectively suggested that supplemental H2 had a minor effect on the overall microbial community composition in soil treatments and root treatments of both plants (Supplemental Text 1, Supplementary Figures 3, 4, and Supplementary Tables 1, 2). Thus, a more detailed analysis was necessary to identify potential soil-born or root-associated H2 consumers.
In order to identify the most important 16S rRNA phylotypes that responded to supplemental H2 in soil treatments a two-step approach was conducted (see section “Material and methods” for details). LEfSe analyses (Segata et al., 2011) identified 17 Carex phylotypes and 10 Molinia phylotypes that (1) were significantly more abundant in H2 supplemented soil treatments than in unsupplemented soil treatments and (2) had LDA-scores of three or higher (Table 2). 13 of the 17 Carex phylotypes and 6 of the 10 Molinia phylotypes were at least twice as abundant in H2 supplemented soil treatments than in unsupplemented soil treatments and only these phylotypes were considered as “stimulated by H2” (Table 2). Phylogenetic analysis revealed that 6 of the 13 Carex phylotypes shared 100% 16S rRNA gene sequence similarity with Molinia phylotypes that fulfilled the LEfSe criteria; hereafter, these phylotypes were designated as “shared phylotypes” (S, e.g., phylotype S1 comprises Carex phylotype C50 and Molinia phylotype M7; Figure 2).
Figure 2. 16S rRNA gene-based phylogenetic trees of bacterial (A) or archaeal (B) phylotypes stimulated by supplemental H2 in root-free soil treatments (bold; see Table 2) and related prokaryotes. S, shared phylotypes (i.e., Carex phylotypes that shared 100% 16S rRNA gene sequence similarity with Molinia phylotypes). The phylogenetic trees were calculated using the neighbor joining function (correction model: Jukes-Cantor) implemented in the ARB software (Ludwig et al., 2004). Bootstrap values (1,000 resamplings) higher than 70% are shown. Methanosarcina acetivorans C2A (AE010299) and Telmatobacter bradus TPB6017 (AM887760) were used as outgroup in (A,B), respectively.
The phylotypes stimulated by H2 collectively accounted for 7.9–13.4% of the 16S rRNA sequences and 3.8–5.7% of the 16S rRNA gene sequences in Treatment SHC or SHM, which was higher than in unsupplemented soil (Treatments SUC and SUM) and fresh soil (SFC and SFM; Figure 3). Some of these phylotypes were phylogenetically affiliated with acetogenic Clostridium species, methanogenic Euryarchaeota, and iron reducers of the genus Geobacter. Other phylotypes fell within the physiologically diverse phylum Acidobacteria or were only distantly related to any cultured organism (Figure 2). In Table 3, potential ecological functions of the phylotypes stimulated by H2 were discussed based on physiological traits and genomic potentials of cultured relatives.
Figure 3. Relative abundance of phylotypes stimulated by supplemental H2 in Carex (A) and Molinia (B) soil treatments. Displayed phylotypes were considered as “stimulated by H2” based on a manually refined LEfSe approach (Table 2). Samples/Treatments: SFC, fresh Carex soil; SUC, unsupplemented Carex soil; SHC, H2 supplemented Carex soil; SFM, fresh Molinia soil; SUM, unsupplemented Molinia soil; SHM, H2 supplemented Molinia soil; numbers specify replicates. Phylotype identifiers: C/M, phylotypes from experiments with Carex/Molinia soil; S, shared phylotypes (i.e., Carex phylotypes that shared 100% 16S rRNA gene sequence similarity with Molinia phylotypes; Figure 2); closest cultured relatives and BLASTn identities are given in parentheses.
In summary, the manually refined LEfSe approach conducted in this study was appropriate to reveal phylotypes stimulated by H2 in soil treatments. Some of these phylotypes were related either to hydrogenotrophic acetogens or hydrogenotrophic methanogens and these phylotypes might have contributed to the observed accumulation of acetate and CH4, respectively, (Figure 1A). Other phylotypes presumably represent iron reducers and might have been involved in early consumption of exogenous H2 (Table 1); alternatively, they were stimulated by acetate derived from hydrogenotrophic acetogenesis.
H2 partial pressures in unsupplemented soil treatments were mostly below the detection limit of ∼10 ppmV. Hence, the calculation of Gibbs free energies (ΔGs) for hydrogenotrophic acetogenesis and hydrogenotrophic methanogenesis was not possible. However, solely CO2 but neither acetate nor CH4 accumulated in treatments SUC and SUM (Figure 1A), suggesting that, in the absence of root organic carbon, respiratory anaerobes thriving on alternative electron acceptors like ferric iron or sulfate were involved in the mineralization of organic carbon and outcompeted acetogens and methanogens for endogenous H2. This assumption is supported by long lag phases for methane accumulation and immediate iron reduction in unsupplemented bulk peat soil incubations from the study site (Reiche et al., 2008). Furthermore, thermodynamic calculations indicated that in situ iron and sulfate reducers can outcompete acetogens and methanogens for dissolved H2 in bulk peat soil of fen Schlöppnerbrunnen (Knorr et al., 2009; Estop-Aragonés et al., 2013). Notably, 6–12 Pa H2 were detected at day 17 in Treatment SUM and at this time hydrogenotrophic methanogenesis was sufficiently exergonic (Figure 4A) and CH4 concentrations finally increased (Figure 1A). At the same time the mean ΔG for hydrogenotrophic acetogenesis was −5 kJ mol–1, a value that has been shown to be the thermodynamic limit of the acetogen Acetobacterium carbinolicum (Conrad and Wetter, 1990). In the aforementioned study A. carbinolicum had H2 thresholds of 10 Pa at 15°C (the incubation temperature in the present study). Thus, it cannot be excluded that H2-consuming acetogens contributed to the slight acetate accumulation observed in Treatment SUM at day 17 (Figure 1A).
Figure 4. Gibbs free energies (ΔG) of anaerobic processes in soil treatments (A) and root treatments (B). ΔGs were calculated based on the following reactions: hydrogenotrophic acetogenesis, 4H2 + 2CO2 → CH3COO– + H+ + 2H2O; hydrogenotrophic methanogenesis, 4H2 + CO2 → CH4 + 2H2O; aceticlastic methanogenesis, CH3COO– + H+ → CH4 + CO2. •, H2 treatments; °, unsupplemented treatments; when H2 was below the detection limit (∼10 ppmV) no ΔGs could be calculated for hydrogenotrophic acetogenesis and hydrogenotrophic methanogenesis. Values represent means of triplicate analysis and error bars indicate standard deviations. Treatments: SHC, H2 supplemented Carex soil; SUC, unsupplemented Carex soil; SHM, H2 supplemented Molinia soil; SUM, unsupplemented Molinia soil; RHC, H2 supplemented Carex roots; RUC, unsupplemented Carex roots; RHM, H2 supplemented Molinia roots; and RUM, unsupplemented Molinia roots.
In the initial incubation phase in H2 supplemented soil treatments, consumption of exogenous H2 was not coupled to the accumulation of acetate and CH4 (Figure 1A and Table 1), pointing toward the activity of anaerobic H2 consumers using electron acceptors others than CO2. In this regard, consumption of exogenous H2 (or formate) decoupled from methanogenesis and acetogenesis has been reported for soil incubations of fen Schlöppnerbrunnen before (Reiche et al., 2008; Hädrich et al., 2012; Hausmann et al., 2016). Nitrate, ferric iron, sulfate, and humic substances are alternative electron acceptors available in this fen (Estop-Aragonés et al., 2013). Since reported nitrate concentrations are low (0–150 μM) in the fen Schlöppnerbrunnen, it is unlikely that denitrification, a process that is proposed to be important in situ (Palmer et al., 2010, 2016), accounted for much of the consumed H2 in soil treatments. In contrast, the cumulative electron acceptor capacity of ferric iron, sulfate, and, potentially, humic substances might suffice to account for the unresolved sink of H2 in the incubated fen soil (Reiche et al., 2008; Knorr and Blodau, 2009; Knorr et al., 2009; Pester et al., 2012; Estop-Aragonés et al., 2013). In this regard, H2 consumption coupled to the reduction of ferric iron is supported by the finding that four phylotypes (S5, C980, C198, and M106) that were stimulated by supplemental H2 were related (97.7–99.5% identity) to H2-consuming iron reducers of the genus Geobacter (Figure 3 and Table 3). In addition, two phylotypes (S6 and C21) were related to Acidobacteria that might play a role in H2 consumption coupled to the reduction of ferric iron or sulfate (Figure 3 and Table 3). However, only few isolates of the Acidobacteria have been physiologically characterized thus far, and potential capabilities to reduce ferric iron or sulfate among the Acidobacteria have been largely inferred from genomic data and await validation (Ward et al., 2009; Hausmann et al., 2018).
Subsequently, acetate and CH4 accumulated in H2 supplemented soil treatments, and reductant recoveries indicated that alternative electron acceptors might have been largely depleted and acetogens as well as methanogens were finally main H2 consumers during this second incubation phase (Figure 1A and Table 1). That supplemental H2 stimulated acetogenesis and methanogenesis in peat soil treatments corroborated previous studies suggesting that, in fen Schlöppnerbrunnen and other peatlands, acetogens and methanogens are limited by H2 availability and both are poised to respond to temporarily higher H2 concentrations (Kotsyurbenko et al., 1996; Bräuer et al., 2004; Wüst et al., 2009; Hunger et al., 2011, 2015, 2016; Hädrich et al., 2012). Four phylotypes that were stimulated by H2 (Figure 3) were related to cultured acetogens (S1 to Clostridium drakei, C2605 to C. magnum, S2 and C200 to Holophaga foetida) and three to hydrogenotrophic methanogens (S3 and M78 to Methanolcella species and S4 to Methanobacterium lacus; Table 3). However, BLAST identities of Holophaga- and Methanocella-related phylotypes to cultured relatives were “only” 95.1–96.6%; thus, isolation and physiological characterization are necessary to validate that these fen phylotypes indeed represent acetogens and methanogens.
Recently, high relative 16S rRNA abundances of Methanosaeta and Methanosarcina in fresh peat soil from patches covered with Carex indicated that, locally, aceticlastic methanogens can make up a considerable fraction of the active prokaryotes in fen Schlöppnerbrunnen (Meier et al., 2021). In this regard, one phylotype (C44) was related to the aceticlastic methanogen Methanosarcina acetivorans (98.7% identity) and it accounted for 1.8–2.5% of all 16S rRNA sequences in the H2 supplemented Carex soil (SHC, Supplementary Figure 4). Thus, it is possible that some of the acetate produced by acetogens thriving on supplemental H2 was subsequently converted into CH4 in Treatment SHC (Figure 1A). Likewise, acetate is a growth substrate of the iron reducing Geobacter species related to phylotypes S5, C980, C198, and M106 (Table 3), and one may speculate that these phylotypes were not exclusively thriving on supplemental H2 but consumed acetate as well. This could explain why acetate accumulation was slow or absent at the beginning of incubation in treatments SHC and SHM, although hydrogenotrophic acetogenesis was sufficiently exergonic right from the start (Figure 4A). Consequently, the calculated reductant recoveries for H2 supplemented soil treatments likely underestimated the contribution of acetogens to the consumption of supplemental H2 due to presumed acetate consumption by iron reducers and aceticlastic methanogens (Table 1). In situ, effective removal of acetate would be advantageous for acetogens since their capability to utilize low H2 concentrations depends on low acetate concentrations (Schmidt et al., 2016).
Favorable thermodynamics for aceticlastic methanogenesis (<−60 kJ mol–1; Figure 4A) and the finding that the Methanosarcina-related phylotype C44 accounted for 1.3–5.9% of the 16S rRNA sequences in unsupplemented Carex soil (Treatment SUC, Supplementary Figure 4) raised the question why methane accumulation in this treatment was not observed. Efficient CH4 removal via anaerobic CH4 oxidation potentially coupled to the reduction of ferric iron is one possible explanation and was suggested to occur in peatlands before (Smemo and Yavitt, 2007, 2011; Yan et al., 2018).
To evaluate H2 consumption at contrasting H2 availabilities by root-associated acetogens and methanogens, Carex roots and Molinia roots were anoxically incubated with and without supplemental H2. Product profiles of unsupplemented roots (Treatments RUC and RUM; Figure 1B and Supplementary Figure 2) corroborated recent findings that (1) H2 is produced along with ethanol, CO2, acetate, butyrate, and propionate during the fermentative degradation of root-derived organic carbon, and (2) the extent of H2 accumulation varies between replicates (Meier et al., 2021). Toward the end of the 17-day incubation, H2 consumption exceeded H2 production in replicates RUC2 and RUM3, whereas H2 production and consumption were balanced in RUC1 and RUC3, and H2 production exceeded its consumption in RUM1 and RUM2 (Figure 1B). Independent of overall increasing or decreasing H2 concentrations, it is likely that acetogens and fermenters were active at the same time. However, the experimental design did not allow to distinguish between acetate production by hydrogenotrophic acetogenesis, organotrophic acetogenesis, and fermentation.
Previously, formate-derived H2, which was subsequently consumed, stimulated the production of acetate and methane in Carex root treatments (Hunger et al., 2016), and a similar stimulatory effect was anticipated for supplemental H2 in the present study. However, H2 concentrations were slowly decreasing or stayed constant in most replicates of root treatments with supplemental H2, and neither the accumulation of acetate nor that of CH4 (note that CH4 was marginal in all root treatments) were significantly higher (P ≤ 0.05; Wilcoxon) in the H2 supplemented roots (Treatments RHC and RHM) compared to unsupplemented roots (Treatments RUC and RUM; Figure 1B). Nevertheless, the strong accumulation of acetate paralleled by a decrease in H2, as observed in RHC1 between day 10 and day 15 and in RHM3 between day 7 and day 11 (Figure 1B), were a strong indicator of hydrogenotrophic acetogenesis in these two replicates.
The manually refined LEfSe approach conducted to reveal phylotypes that were stimulated by supplemental H2 in soil treatments was not appropriate for the identification of such phylotypes in root treatments (data not shown). In this regard, fermentation of root-derived organic carbon yielded presumably sufficient H2 for root-associated H2 consumers, largely obliterating the expected stimulative effect of supplemental H2 in root treatments (Figure 1B). In an alternative approach, phylotypes with either ≥1% 16S rRNA or ≥0.5% 16S rRNA gene relative abundance in at least one replicate of any of the root treatments (RUC, RUM, RHC, and RHM) were subjected to a BLASTn analysis and the closest cultured relatives were manually screened for potential H2 consumers (e.g., acetogens, methanogens, and iron reducers).
This analysis revealed one phylotype [S1; this phylotype was also active in soil treatments (Figure 3)] with 97.8% identity to the peat bog acetogen Clostridium drakei FP (Gößner et al., 2008). In RHM3 and RHC1, i.e., the two replicates of root H2 treatments in which H2 consumption was most obvious (Figure 1B), phylotype S1 accounted for 9.4% and 3.8% of all 16S rRNA sequences, respectively, (Table 4). However, 16S rRNA and 16S rRNA gene relative abundances of S1 were high in Treatment RUM (Table 4), and in replicates of this treatment H2 consumption was less obvious (Figure 1B). Thus, the relative abundance of S1 did not in all cases correlate with observable H2 consumption.
Table 4. Relative abundances of potentially acetogenic phylotypes in fresh and anoxically incubated roots a.
A second potentially acetogenic phylotype [M227; 98.3% identity to the hydrogenotrophic acetogen Clostridium magnum (Bomar et al., 1991)] was observed in Molinia root treatments (Table 4). Collectively, S1 and M227, which were marginal in fresh Molinia root samples, accounted for up to 10.6% of the 16S rRNA sequences (in RHM3) and 8.8% of the 16S rRNA gene sequences (in RUM3), indicating that root-associated acetogens thrived in Molinia root treatments with and without supplemental H2 (Table 4).
Phylotypes S1 and M227 were 100% similar to G2 (acc. no. LR702023) and G1 (LR702022), respectively, and the latter two phylotypes were detected in unsupplemented Carex and Molinia root treatments before (Meier et al., 2021). Furthermore, S1 was 100% similar to an acetogen (LT009683; Figure 2) present in an acetogenic enrichment (FH) that was derived from a mixture of Carex and Molinia roots collected at fen Schlöppnerbrunnen; enrichment FH converted formate and H2 to acetate (Hunger et al., 2016). That phylotypes related to C. drakei (S1) and C. magnum (M227) were repeatedly observed in treatments with Carex and Molinia roots suggests that clostridial acetogens colonize the roots of these graminoids in fen Schlöppnerbrunnen.
Thermodynamic calculations revealed that hydrogenotrophic acetogenesis was feasible in unsupplemented roots (Treatments RUC and RUM; Figure 4B) as soon as sufficient fermentation-derived H2 accumulated (after 3 to 6 days; Figure 1B). Furthermore, supplemental H2 did neither significantly stimulate acetate production (Figure 1B) nor were 16S rRNA and 16S rRNA gene relative abundances of potentially acetogenic phylotypes significantly higher in root treatments with supplemental H2 than in those without (Table 4). These findings indicated that acetogens in root treatments did not relay on supplemental H2. Nevertheless, H2 consumption in root treatments was most obvious in two replicates that received supplemental H2 (RHC1 and RHM3; Figure 1B). In RHM3 between day 7 and 11, concentrations of H2 and CO2 decreased by 6 mM and 2.8 mM, respectively, and acetate increased by 12.8 mM. However, only 1.5 mM acetate can be formed from 6 mM H2 via hydrogenotrophic acetogenesis. This discrepancy can be explained as follows: (1) A part of the accumulated acetate was presumably produced by fermenters thriving on root-derived organic carbon (Meier et al., 2021). (2) Only net consumption/production of H2, CO2, and acetate could be determined, but fermentative production of H2 and CO2 were most likely ongoing concomitantly to their consumption by acetogens in all root treatments. In this regard, radiotracer experiments indicated that 30–40% of acetate in rice root treatments originated from H2-CO2, although net consumption of H2 could only account for 4% of accumulated acetate, pointing toward a fast turnover of H2 in these rice root treatments (Conrad and Klose, 1999). (3) The metabolic versatility of acetogens is widely recognized (Drake et al., 2008), and, in the root treatments, acetogens might have utilized root-derived organic carbon (e.g., sugars) and fermentation products [e.g., formate, lactate, and ethanol (Weghoff et al., 2015; Bertsch et al., 2016; Hunger et al., 2016)] in addition to H2-CO2. In this respect, ethanol concentrations decreased by 4.8 mM in RHM3 between day 7 and 11 (Supplementary Figure 2), and this amount of ethanol can theoretically yield 7.2 mM acetate.
Methanogens can be associated with roots of wetland plants (Kimura et al., 1991; King, 1994; Conrad and Klose, 1999), and, previously, supplemental formate or formate-derived H2 stimulated CH4 production in treatments with washed Carex roots (Hunger et al., 2016). In the present study, CH4 accumulation was negligible in root treatments with and without supplemental H2 (Figure 1B), although hydrogenotrophic and aceticlastic methanogenesis were highly exergonic (Figure 4B). Since the 16S rRNA and 16S rRNA gene relative abundances of methanogens (and archaea in general) were low (Supplementary Figure 4), it is possible that methanogens were loosely attached to the root surface and were largely removed by the gentle washing procedure. In addition, methanogens may have been inhibited by the high concentrations of organic acids (Figure 1B and Supplementary Figure 2) that at the moderately acidic pH persist largely in their undissociated form and can cause a decoupling of the proton motive force (Luli and Strohl, 1990; Horn et al., 2003).
H2 was an important product of fermenters thriving on decaying roots and root litter in Carex and Molinia root treatments conducted in this and previous studies (Hunger et al., 2016; Meier et al., 2021). in situ, exudates, constantly released by roots of photosynthetically active plants, are another important source of root-derived organic carbon (Jones et al., 2009), and they might be partially converted to H2 as well. H2 formed by root-associated fermenters can theoretically (1) be transported through the plants via the aerenchyma and emitted to the atmosphere, (2) be consumed by aerobic or anerobic microbial H2 consumers colonizing the roots, or (3) radially diffuse into the soil surrounding the roots where it is eventually consumed by soil microbes (Conrad, 1996). Acetogens inhabiting the root zone of graminoids could profit from locally and temporarily higher H2 concentrations than the 0.2–28 nmol l–1 dissolved H2 (corresponds to a H2 partial pressure of approximately 0.03–4 Pa) observed in bulk peat soil of the fen Schlöppnerbrunnen (Knorr et al., 2009; Estop-Aragonés et al., 2013). In fact, acetogens have been shown to be associated to the roots of several aquatic plants, and their ability to cope with oxic stress makes them less vulnerable to O2 released from the roots than methanogens (Conrad and Klose, 1999; Küsel et al., 1999, 2001; Leaphart et al., 2003; Gößner et al., 2006). As discussed earlier (Conrad and Klose, 1999; Hunger et al., 2016; Meier et al., 2021), the conducted incubations cannot simulate the complex processes ongoing in the root zone of a living plant, but show the potential of its anaerobic microbial community to thrive on supplemental H2, fermentation-derived H2, and root or peat organic carbon.
In Figure 5 the interwoven trophic links in root and soil treatments were graphically summarized to help addressing the initial hypotheses: (1) Phylotypes related to cultured acetogens (Clostridium and Holophaga) were identified in root treatments and soil treatments, underscoring that acetogens are associated to graminoid roots and inhabit the surrounding peat soil. However, these potential acetogens have yet to be cultured to validate their assumed physiology. (2) Acetogens most likely consumed fermentation derived H2 in root treatments and supplemental H2 in soil treatments, suggesting that H2, temporarily formed in excess at the immediate vicinity of graminoid roots, can be utilized by acetogens, if it accumulates to sufficiently high concentrations. In situ, H2 will likely diffuse away (Conrad, 1996) and not accumulate to concentrations as high as in root treatments or H2 supplemented soil treatments. Therefore, radial H2 profiles at high resolution around roots of living graminoids are required to prove that their root zones are indeed microenvironments with higher H2 availabilities. Thus far, in situ H2 concentrations could only be resolved at larger scales at fen Schlöppnerbrunnen (Knorr et al., 2009; Estop-Aragonés et al., 2013). (3) Product profiles in unsupplemented soil treatments corroborated the assumption that in the absence of root-derived organic carbon, acetogens are outcompeted for endogenous H2 by H2 consumers with lower thresholds. In the iron rich fen Schlöppnerbrunnen, not only hydrogenotrophic methanogens (e.g., Methanocellales and Methanobacterium) but also iron reducers (e.g., Geobacter) and, presumably, sulfate reducers compete with acetogens for available H2 (Reiche et al., 2008; Hausmann et al., 2016). However, acetogens are metabolically flexible and do not rely on H2 (Drake et al., 2008; Schuchmann and Müller, 2016). In situ, acetogens could thrive on root-derived organic carbon, organic fermentation products, and CO in addition to H2. In this respect, acetogens can co-metabolize several energy sources (e.g., H2 and formate) at the same time or grow mixotrophically (i.e., H2 is used as a lithotrophic energy source and organic compounds are used as heterotrophic carbon source). Furthermore, acetogens are well adapted to changing redox conditions that are characteristic for the root-zone of wetland plants (Conrad, 1996; Brune et al., 2000). Thus, when O2 and alternative electron acceptors are temporarily and locally depleted, acetogens might dominate H2 oxidation until hydrogenotrophic methanogens reestablish H2 concentrations below the threshold of acetogens.
Figure 5. Hypothetical model summarizing the main processes and taxa observed in root treatments (left) and soil treatments (right). TEAox, unknown terminal electron acceptors such as ferric iron or sulfate; TEAred, unknown reduced products (e.g., ferrous iron or sulfide) of anaerobic respiratory prokaryotes. Dotted lines indicate that some of the acetate produced by acetogens may have been consumed by aceticlastic methanogenesis or other acetate-consuming processes.
The root-zones of fen graminoids are hotspots for H2-producing fermenters in the fen Schlöppnerbrunnen, and it was hypothesized that acetogens may thrive on H2 diffusing into the soil around graminoid roots (Hunger et al., 2016; Meier et al., 2021). In the present study, potential acetogenic phylotypes successfully competed with methanogens in soil and root treatments when H2 was available in sufficiently high concentrations. However, acetogens and methanogens were outcompeted, possibly by iron reducers, when H2 concentrations were low (unsupplemented root-free soil treatments). To prove that acetogens can indeed thrive on H2 in the root-zones of graminoids in the fen Schlöppnerbrunnen and other peatlands, radial H2 profiles at high resolution around roots of living plants would be required. Nevertheless, especially those acetogens that are more tightly associated to the roots (e.g., acetogenic phylotypes that were detected in root-treatments; Table 4) could thrive directly on root-derived organic carbon or organic fermentation products (e.g., ethanol) in addition to H2.
By shifting the flow of carbon and reductant toward acetate during the anaerobic degradation of root organic carbon, hydrogenotrophic and organotrophic acetogens collectively can limit the H2 availability for hydrogenotrophic methanogens (Conrad, 1999; Schuchmann and Müller, 2016). When acetate is subsequently not consumed by aceticlastic methanogens, as observed in a peatland in Alaska (Duddleston et al., 2002), the overall production of CH4 is low. Thus, acetogens in the root zone of graminoids could be involved in controlling CH4 production in some peatlands, and further studies are needed to better resolve trophic links between acetogens and acetate consuming prokaryotes in these globally relevant ecosystems.
The data presented in the study are deposited in the European Nucleotide Archive (ENA) repository, accession numbers: PRJEB37304 and PRJEB37863; the data have been released.
AM, SO, HD, and OS conceived to the study. AM performed the experiments. AM and OS analyzed the data. OS wrote the manuscript with input from all authors. All authors have read and agreed to the final version of the manuscript.
Support for this study was provided by the University of Bayreuth. The APC for this article has been covered by UiT The Arctic University of Norway.
We thank Maraike Staege for technical assistance.
The authors declare that the research was conducted in the absence of any commercial or financial relationships that could be construed as a potential conflict of interest.
All claims expressed in this article are solely those of the authors and do not necessarily represent those of their affiliated organizations, or those of the publisher, the editors and the reviewers. Any product that may be evaluated in this article, or claim that may be made by its manufacturer, is not guaranteed or endorsed by the publisher.
The Supplementary Material for this article can be found online at: https://www.frontiersin.org/articles/10.3389/fmicb.2022.978296/full#supplementary-material
Abdalla, M., Hastings, A., Truu, J., Espenberg, M., Mander, Ü, and Smith, P. (2016). Emissions of methane from northern peatlands: A review of management impacts and implications for future management options. Ecol. Evol. 6, 7080–7102. doi: 10.1002/ece3.2469
Aklujkar, M., Young, N. D., Holmes, D., Chavan, M., Risso, C., Kiss, H. E., et al. (2010). The genome of Geobacter bemidjiensis, exemplar for the subsurface clade of Geobacter species that predominate in Fe(III)-reducing subsurface environments. BMC Genomics 11:490. doi: 10.1186/1471-2164-11-490
Aselmann, I., and Crutzen, P. J. (1989). Global distribution of natural freshwater wetlands and rice paddies, their net primary productivity, seasonality and possible methane emissions. J. Atmospheric Chem. 8, 307–358. doi: 10.1007/BF00052709
Bak, F., Finster, K., and Rothfuß, F. (1992). Formation of dimethylsulfide and methanethiol from methoxylated aromatic compounds and inorganic sulfide by newly isolated anaerobic bacteria. Arch. Microbiol. 157, 529–534. doi: 10.1007/BF00276773
Bertsch, J., Siemund, A. L., Kremp, F., and Müller, V. (2016). A novel route for ethanol oxidation in the acetogenic bacterium Acetobacterium woodii: The acetaldehyde/ethanol dehydrogenase pathway. Environ. Microbiol. 18, 2913–2922. doi: 10.1111/1462-2920.13082
Blöthe, M., Akob, D. M., Kostka, J. E., Göschel, K., Drake, H. L., and Küsel, K. (2008). pH gradient-induced heterogeneity of Fe(III)-reducing microorganisms in coal mining-associated lake sediments. Appl. Environ. Microbiol. 74, 1019–1029. doi: 10.1128/AEM.01194-07
Bomar, M., Hippe, H., and Schink, B. (1991). Lithotrophic growth and hydrogen metabolism by Clostridium magnum. FEMS Microbiol. Lett. 83, 347–349. doi: 10.1111/j.1574-6968.1991.tb04488.x
Bräuer, S. L., Yavitt, J. B., and Zinder, S. H. (2004). Methanogenesis in McLean Bog, an acidic peat bog in Upstate New York: Stimulation by H2/CO2 in the presence of rifampicin, or by low concentrations of acetate. Geomicrobiol. J. 21, 433–443. doi: 10.1080/01490450490505400
Brune, A., Frenzel, P., and Cypionka, H. (2000). Life at the oxic–anoxic interface: Microbial activities and adaptations. FEMS Microbiol. Rev. 24, 691–710. doi: 10.1111/j.1574-6976.2000.tb00567.x
Cadillo-Quiroz, H., Bräuer, S. L., Goodson, N., Yavitt, J. B., and Zinder, S. H. (2014). Methanobacterium paludis sp. nov. and a novel strain of Methanobacterium lacus isolated from northern peatlands. Int. J. Syst. Evol. Microbiol. 64, 1473–1480. doi: 10.1099/ijs.0.059964-0
Conrad, R. (1996). Soil microorganisms as controllers of atmospheric trace gases (H2, CO, CH4, OCS, N2O, and NO). Microbiol. Rev. 60, 609–640. doi: 10.1128/mr.60.4.609-640.1996
Conrad, R. (1999). Contribution of hydrogen to methane production and control of hydrogen concentrations in methanogenic soils and sediments. FEMS Microbiol. Ecol. 28, 193–202. doi: 10.1111/j.1574-6941.1999.tb00575.x
Conrad, R., and Klose, M. (1999). Anaerobic conversion of carbon dioxide to methane, acetate and propionate on washed rice roots. FEMS Microbiol. Ecol. 30, 147–155. doi: 10.1111/j.1574-6941.1999.tb00643.x
Conrad, R., and Wetter, B. (1990). Influence of temperature on energetics of hydrogen metabolism in homoacetogenic, methanogenic, and other anaerobic bacteria. Arch. Microbiol. 155, 94–98. doi: 10.1007/BF00291281
Drake, H. L., Gößner, A. S., and Daniel, S. L. (2008). Old acetogens, new light. Ann. N. Y. Acad. Sci. 1125, 100–128. doi: 10.1196/annals.1419.016
Drake, H. L., Horn, M. A., and Wüst, P. K. (2009). Intermediary ecosystem metabolism as a main driver of methanogenesis in acidic wetland soil. Environ. Microbiol. Rep. 1, 307–318. doi: 10.1111/j.1758-2229.2009.00050.x
Drake, H. L., Küsel, K., and Matthies, C. (2006). “Acetogenic prokaryotes,” in The Prokaryotes, eds M. Dworkin, S. Falkow, E. Rosenberg, K.-H. Schleifer, and E. Stackebrandt (New York, NY: Springer), 354–420. doi: 10.1007/978-3-642-30141-4_61
Duddleston, K. N., Kinney, M. A., Kiene, R. P., and Hines, M. E. (2002). Anaerobic microbial biogeochemistry in a northern bog: Acetate as a dominant metabolic end product. Glob. Biogeochem. Cycles 16:1063. doi: 10.1029/2001GB001402
Estop-Aragonés, C., Knorr, K.-H., and Blodau, C. (2013). Belowground in situ redox dynamics and methanogenesis recovery in a degraded fen during dry-wet cycles and flooding. Biogeosciences 10, 421–436. doi: 10.5194/bg-10-421-2013
Eurola, S., Hicks, S., and Kaakinen, E. (1984). “Key to finnish mire types,” in European Mires, ed. P. D. Moore (London: Academic Press), 11–117. doi: 10.1016/B978-0-12-505580-2.50006-4
Gößner, A. S., Küsel, K., Schulz, D., Trenz, S., Acker, G., Lovell, C. R., et al. (2006). Trophic interaction of the aerotolerant anaerobe Clostridium intestinale and the acetogen Sporomusa rhizae sp. nov. isolated from roots of the black needlerush Juncus roemerianus. Microbiology 152, 1209–1219. doi: 10.1099/mic.0.28725-0
Gößner, A. S., Picardal, F., Tanner, R. S., and Drake, H. L. (2008). Carbon metabolism of the moderately acid-tolerant acetogen Clostridium drakei isolated from peat. FEMS Microbiol. Lett. 287, 236–242. doi: 10.1111/j.1574-6968.2008.01313.x
Hädrich, A., Heuer, V. B., Herrmann, M., Hinrichs, K.-U., and Küsel, K. (2012). Origin and fate of acetate in an acidic fen. FEMS Microbiol. Ecol. 81, 339–354. doi: 10.1111/j.1574-6941.2012.01352.x
Hamberger, A., Horn, M. A., Dumont, M. G., Murrell, J. C., and Drake, H. L. (2008). Anaerobic consumers of monosaccharides in a moderately acidic fen. Appl. Environ. Microbiol. 74, 3112–3120. doi: 10.1128/AEM.00193-08
Hammer, O., Harper, D. A. T., and Ryan, P. D. (2001). PAST: Paleontological statistics software package for education and data analysis. Palaeontol. Electron. 4, 1–9.
Harenda, K. M., Lamentowicz, M., Samson, M., and Chojnicki, B. H. (2018). “The role of peatlands and their carbon storage function in the context of climate change,” in Interdisciplinary Approaches for Sustainable Development Goals, eds I. Sagan and W. Surosz (Cham: Springer International Publishing), 169–187. doi: 10.1007/978-3-319-71788-3_12
Hausmann, B., Knorr, K.-H., Schreck, K., Tringe, S. G., Glavina, del Rio, T., et al. (2016). Consortia of low-abundance bacteria drive sulfate reduction-dependent degradation of fermentation products in peat soil microcosms. ISME J. 10, 2365–2375. doi: 10.1038/ismej.2016.42
Hausmann, B., Pelikan, C., Herbold, C. W., Köstlbacher, S., Albertsen, M., Eichorst, S. A., et al. (2018). Peatland Acidobacteria with a dissimilatory sulfur metabolism. ISME J. 12, 1729–1742. doi: 10.1038/s41396-018-0077-1
Horn, M. A., Matthies, C., Küsel, K., Schramm, A., and Drake, H. L. (2003). Hydrogenotrophic methanogenesis by moderately acid-tolerant methanogens of a methane-emitting acidic peat. Appl. Environ. Microbiol. 69, 74–83. doi: 10.1128/AEM.69.1.74-83.2003
Hunger, S., Gößner, A. S., and Drake, H. L. (2015). Anaerobic trophic interactions of contrasting methane-emitting mire soils: Processes versus taxa. FEMS Microbiol. Ecol. 91:fiv045. doi: 10.1093/femsec/fiv045
Hunger, S., Schmidt, O., Gößner, A. S., and Drake, H. L. (2016). Formate-derived H2, a driver of hydrogenotrophic processes in the root-zone of a methane-emitting fen. Environ. Microbiol. 18, 3106–3119. doi: 10.1111/1462-2920.13301
Hunger, S., Schmidt, O., Hilgarth, M., Horn, M. A., Kolb, S., Conrad, R., et al. (2011). Competing formate- and carbon dioxide-utilizing prokaryotes in an anoxic methane-emitting fen soil. Appl Env. Microbiol 77, 3773–3785. doi: 10.1128/AEM.00282-11
Jones, D. L., Nguyen, C., and Finlay, R. D. (2009). Carbon flow in the rhizosphere: Carbon trading at the soil–root interface. Plant Soil 321, 5–33. doi: 10.1007/s11104-009-9925-0
Kimura, M., Murakami, H., and Wada, H. (1991). CO2, H2, and CH4 production in rice rhizosphere. Soil Sci. Plant Nutr. 37, 55–60. doi: 10.1080/00380768.1991.10415010
King, G. M. (1994). Associations of methanotrophs with the roots and rhizomes of aquatic vegetation. Appl. Environ. Microbiol. 60, 3220–3227. doi: 10.1128/aem.60.9.3220-3227.1994
Knorr, K.-H., and Blodau, C. (2009). Impact of experimental drought and rewetting on redox transformations and methanogenesis in mesocosms of a northern fen soil. Soil Biol. Biochem. 41, 1187–1198. doi: 10.1016/j.soilbio.2009.02.030
Knorr, K.-H., Lischeid, G., and Blodau, C. (2009). Dynamics of redox processes in a minerotrophic fen exposed to a water table manipulation. Geoderma 153, 379–392. doi: 10.1016/j.geoderma.2009.08.023
Koelbener, A., Ström, L., Edwards, P. J., and Olde Venterink, H. (2010). Plant species from mesotrophic wetlands cause relatively high methane emissions from peat soil. Plant Soil 326, 147–158. doi: 10.1007/s11104-009-9989-x
Končalová, H. (1990). Anatomical adaptations to waterlogging in roots of wetland graminoids: Limitations and drawbacks. Aquat. Bot. 38, 127–134. doi: 10.1016/0304-3770(90)90102-Q
Kotsyurbenko, O. R., Friedrich, M. W., Simankova, M. V., Nozhevnikova, A. N., Golyshin, P. N., Timmis, K. N., et al. (2007). Shift from acetoclastic to H2-dependent methanogenesis in a West Siberian peat bog at low pH values and isolation of an acidophilic Methanobacterium strain. Appl. Environ. Microbiol. 73, 2344–2348. doi: 10.1128/AEM.02413-06
Kotsyurbenko, O. R., Glagolev, M. V., Merkel, A. Y., Sabrekov, A. F., and Terentieva, I. E. (2019). “Methanogenesis in soils, wetlands, and peat,” in Biogenesis of Hydrocarbons, eds A. J. M. Stams and D. Z. Sousa (Cham: Springer), 211–228. doi: 10.1007/978-3-319-78108-2_9
Kotsyurbenko, O. R., Glagolev, M. V., Nozhevnikova, A. N., and Conrad, R. (2001). Competition between homoacetogenic bacteria and methanogenic archaea for hydrogen at low temperature. FEMS Microbiol. Ecol. 38, 153–159. doi: 10.1111/j.1574-6941.2001.tb00893.x
Kotsyurbenko, O. R., Nozhevnikova, A. N., Soloviova, T. I., and Zavarzin, G. A. (1996). Methanogenesis at low temperatures by microflora of tundra wetland soil. Antonie Van Leeuwenhoek J. Microbiol. 69, 75–86. doi: 10.1007/BF00641614
Kotsyurbenko, O. R., Simankova, M. V., Nozhevnikova, A. N., Zhilina, T. N., Bolotina, N. P., Lysenko, A. M., et al. (1995). New species of psychrophilic acetogens: Acetobacterium bakii sp. nov., A. paludosum sp. nov., A. fimetarium sp. nov. Arch. Microbiol. 163, 29–34. doi: 10.1007/BF00262200
Kulichevskaya, I. S., Suzina, N. E., Rijpstra, W. I. C., Damste, J. S. S., and Dedysh, S. N. (2014). Paludibaculum fermentans gen. nov., sp. nov., a facultative anaerobe capable of dissimilatory iron reduction from subdivision 3 of the Acidobacteria. Int. J. Syst. Evol. Microbiol. 64, 2857–2864. doi: 10.1099/ijs.0.066175-0
Küsel, K., Blöthe, M., Schulz, D., Reiche, M., and Drake, H. L. (2008). Microbial reduction of iron and porewater biogeochemistry in acidic peatlands. Biogeosciences 5, 1537–1549. doi: 10.5194/bg-5-1537-2008
Küsel, K., Karnholz, A., Trinkwalter, T., Devereux, R., Acker, G., and Drake, H. L. (2001). Physiological ecology of Clostridium glycolicum RD-1, an aerotolerant acetogen isolated from sea grass roots. Appl. Environ. Microbiol. 67, 4734–4741. doi: 10.1128/AEM.67.10.4734-4741.2001
Küsel, K., Pinkart, H. C., Drake, H. L., and Devereux, R. (1999). Acetogenic and sulfate-reducing bacteria inhabiting the rhizoplane and deep cortex cells of the sea grass Halodule wrightii. Appl. Environ. Microbiol. 65, 5117–5123. doi: 10.1128/AEM.65.11.5117-5123.1999
Leaphart, A. B., Friez, M. J., and Lovell, C. R. (2003). Formyltetrahydrofolate synthetase sequences from salt marsh plant roots reveal a diversity of acetogenic bacteria and other bacterial functional groups. Appl. Environ. Microbiol. 69, 693–696. doi: 10.1128/aem.69.1.693-696.2003
Lever, M. A. (2012). Acetogenesis in the energy-starved deep biosphere – a paradox? Front. Microbiol. 2:284. doi: 10.3389/fmicb.2011.00284
Liesack, W., Bak, F., Kreft, J.-U., and Stackebrandt, E. (1994). Holophaga foetida gen. nov., sp. nov., a new, homoacetogenic bacterium degrading methoxylated aromatic compounds. Arch. Microbiol. 162, 85–90. doi: 10.1007/BF00264378
Liou, J. S.-C., Balkwill, D. L., Drake, G. R., and Tanner, R. S. (2005). Clostridium carboxidivorans sp. nov., a solvent-producing clostridium isolated from an agricultural settling lagoon, and reclassification of the acetogen Clostridium scatologenes strain SL1 as Clostridium drakei sp. nov. Int. J. Syst. Evol. Microbiol. 55, 2085–2091. doi: 10.1099/ijs.0.63482-0
Lü, Z., and Lu, Y. (2012). Methanocella conradii sp. nov., a thermophilic, obligate hydrogenotrophic methanogen, isolated from Chinese rice field soil. PLoS One 7:e35279. doi: 10.1371/journal.pone.0035279
Ludwig, W., Strunk, O., Westram, R., Richter, L., Meier, H., and Yadhukumar, et al. (2004). ARB: A software environment for sequence data. Nucleic Acids Res. 32, 1363–1371. doi: 10.1093/nar/gkh293
Luli, G. W., and Strohl, W. R. (1990). Comparison of growth, acetate production, and acetate inhibition of Escherichia coli strains in batch and fed-batch fermentations. Appl. Environ. Microbiol. 56, 1004–1011. doi: 10.1128/aem.56.4.1004-1011.1990
Meier, A. B., Oppermann, S., Drake, H. L., and Schmidt, O. (2021). Organic carbon from graminoid roots as a driver of fermentation in a fen. FEMS Microbiol. Ecol. 97:fiab143. doi: 10.1093/femsec/fiab143
Metje, M., and Frenzel, P. (2005). Effect of temperature on anaerobic ethanol oxidation and methanogenesis in acidic peat from a northern wetland. Appl. Environ. Microbiol. 71, 8191–8200. doi: 10.1128/AEM.71.12.8191-8200.2005
Metje, M., and Frenzel, P. (2007). Methanogenesis and methanogenic pathways in a peat from subarctic permafrost. Environ. Microbiol. 9, 954–964. doi: 10.1111/j.1462-2920.2006.01217.x
Nevin, K. P., Holmes, D. E., Woodard, T. L., Hinlein, E. S., Ostendorf, D. W., and Lovley, D. R. (2005). Geobacter bemidjiensis sp. nov. and Geobacter psychrophilus sp. nov., two novel Fe(III)-reducing subsurface isolates. Int. J. Syst. Evol. Microbiol. 55, 1667–1674. doi: 10.1099/ijs.0.63417-0
Nozhevnikova, A. N., Kotsyurbenko, O. R., and Simankova, M. V. (1994). “Acetogenesis at low temperature,” in Acetogenesis Chapman & Hall Microbiology Series, ed. H. L. Drake (Boston, MA: Springer), 416–431. doi: 10.1007/978-1-4615-1777-1_15
Palmer, K., Drake, H. L., and Horn, M. A. (2010). Association of novel and highly diverse acid-tolerant denitrifiers with N2O fluxes of an acidic fen. Appl. Environ. Microbiol. 76, 1125–1134. doi: 10.1128/AEM.02256-09
Palmer, K., Köpp, J., Gebauer, G., and Horn, M. A. (2016). Drying-rewetting and flooding impact denitrifier activity rather than community structure in a moderately acidic fen. Front. Microbiol. 7:727. doi: 10.3389/fmicb.2016.00727
Pankratov, T. A., Kirsanova, L. A., Kaparullina, E. N., Kevbrin, V. V., and Dedysh, S. N. (2012). Telmatobacter bradus gen. nov., sp. nov., a cellulolytic facultative anaerobe from subdivision 1 of the Acidobacteria, and emended description of Acidobacterium capsulatum Kishimoto, et al. 1991. Int. J. Syst. Evol. Microbiol. 62, 430–437. doi: 10.1099/ijs.0.029629-0
Pester, M., Knorr, K.-H., Friedrich, M. W., Wagner, M., and Loy, A. (2012). Sulfate-reducing microorganisms in wetlands – fameless actors in carbon cycling and climate change. Front. Microbiol. 3:72. doi: 10.3389/fmicb.2012.00072
Ragsdale, S. W. (2008). Enzymology of the Wood–Ljungdahl Pathway of Acetogenesis. Ann. N. Y. Acad. Sci. 1125, 129–136. doi: 10.1196/annals.1419.015
Reiche, M., Hädrich, A., Lischeid, G., and Küsel, K. (2009). Impact of manipulated drought and heavy rainfall events on peat mineralization processes and source-sink functions of an acidic fen. J. Geophys. Res. Biogeosciences 114:G02021. doi: 10.1029/2008JG000853
Reiche, M., Torburg, G., and Küsel, K. (2008). Competition of Fe(III) reduction and methanogenesis in an acidic fen. FEMS Microbiol. Ecol. 65, 88–101. doi: 10.1111/j.1574-6941.2008.00523.x
Sakai, S., Conrad, R., Liesack, W., and Imachi, H. (2010). Methanocella arvoryzae sp. nov., a hydrogenotrophic methanogen isolated from rice field soil. Int. J. Syst. Evol. Microbiol. 60, 2918–2923. doi: 10.1099/ijs.0.020883-0
Sakai, S., Imachi, H., Hanada, S., Ohashi, A., Harada, H., and Kamagata, Y. (2008). Methanocella paludicola gen. nov., sp. nov., a methane-producing archaeon, the first isolate of the lineage ‘Rice Cluster I’, and proposal of the new archaeal order Methanocellales ord. nov. Int. J. Syst. Evol. Microbiol. 58, 929–936. doi: 10.1099/ijs.0.65571-0
Schmidt, O., Hink, L., Horn, M. A., and Drake, H. L. (2016). Peat: Home to novel syntrophic species that feed acetate- and hydrogen-scavenging methanogens. ISME J. 10, 1954–1966. doi: 10.1038/ismej.2015.256
Schmidt, O., Horn, M. A., Kolb, S., and Drake, H. L. (2015). Temperature impacts differentially on the methanogenic food web of cellulose-supplemented peatland soil. Environ. Microbiol. 17, 720–734. doi: 10.1111/1462-2920.12507
Schuchmann, K., and Müller, V. (2014). Autotrophy at the thermodynamic limit of life: A model for energy conservation in acetogenic bacteria. Nat. Rev. Microbiol. 12, 809–821. doi: 10.1038/nrmicro3365
Schuchmann, K., and Müller, V. (2016). Energetics and application of heterotrophy in acetogenic bacteria. Appl. Environ. Microbiol. 82, 4056–4069. doi: 10.1128/AEM.00882-16
Segata, N., Izard, J., Waldron, L., Gevers, D., Miropolsky, L., Garrett, W. S., et al. (2011). Metagenomic biomarker discovery and explanation. Genome Biol. 12:R60. doi: 10.1186/gb-2011-12-6-r60
Simankova, M. V., Kotsyurbenko, O. R., Stackebrandt, E., Kostrikina, N. A., Lysenko, A. M., Osipov, G. A., et al. (2000). Acetobacterium tundrae sp. nov., a new psychrophilic acetogenic bacterium from tundra soil. Arch. Microbiol. 174, 440–447. doi: 10.1007/s002030000229
Sizova, M. V., Panikov, N. S., Tourova, T. P., and Flanagan, P. W. (2003). Isolation and characterization of oligotrophic acido-tolerant methanogenic consortia from a Sphagnum peat bog. FEMS Microbiol. Ecol. 45, 301–315. doi: 10.1016/S0168-6496(03)00165-X
Smemo, K. A., and Yavitt, J. B. (2007). Evidence for anaerobic CH4 oxidation in freshwater peatlands. Geomicrobiol. J. 24, 583–597. doi: 10.1080/01490450701672083
Smemo, K. A., and Yavitt, J. B. (2011). Anaerobic oxidation of methane: An underappreciated aspect of methane cycling in peatland ecosystems? Biogeosciences 8, 779–793. doi: 10.5194/bg-8-779-2011
Straub, K. L., and Buchholz-Cleven, B. (2001). Geobacter bremensis sp. nov. and Geobacter pelophilus sp. nov., two dissimilatory ferric-iron-reducing bacteria. Int. J. Syst. Evol. Microbiol. 51, 1805–1808. doi: 10.1099/00207713-51-5-1805
Takahashi, S., Tomita, J., Nishioka, K., Hisada, T., and Nishijima, M. (2014). Development of a prokaryotic universal primer for simultaneous analysis of Bacteria and Archaea using next-generation sequencing. PLoS One 9:e105592. doi: 10.1371/journal.pone.0105592
Thauer, R. K., Kaster, A.-K., Seedorf, H., Buckel, W., and Hedderich, R. (2008). Methanogenic archaea: Ecologically relevant differences in energy conservation. Nat. Rev. Microbiol. 6, 579–591. doi: 10.1038/nrmicro1931
Ward, N. L., Challacombe, J. F., Janssen, P. H., Henrissat, B., Coutinho, P. M., Wu, M., et al. (2009). Three genomes from the phylum Acidobacteria provide insight into the lifestyles of these microorganisms in soils. Appl. Environ. Microbiol. 75, 2046–2056. doi: 10.1128/AEM.02294-08
Weghoff, M. C., Bertsch, J., and Müller, V. (2015). A novel mode of lactate metabolism in strictly anaerobic bacteria. Environ. Microbiol. 17, 670–677. doi: 10.1111/1462-2920.12493
Wüst, P. K., Horn, M. A., and Drake, H. L. (2009). Trophic links between fermenters and methanogens in a moderately acidic fen soil. Environ. Microbiol. 11, 1395–1409. doi: 10.1111/j.1462-2920.2009.01867.x
Yadav, P., Antony-Babu, S., Hayes, E., Healy, O. M., Pan, D., Yang, W. H., et al. (2021). Complete genome sequence of Geobacter sp. strain FeAm09, a moderately acidophilic soil bacterium. Microbiol. Resour. Announc. 10, e979–e920. doi: 10.1128/MRA.00979-20
Yan, Z., Joshi, P., Gorski, C. A., and Ferry, J. G. (2018). A biochemical framework for anaerobic oxidation of methane driven by Fe(III)-dependent respiration. Nat. Commun. 9:1642. doi: 10.1038/s41467-018-04097-9
Ye, R., Jin, Q., Bohannan, B., Keller, J. K., and Bridgham, S. D. (2014). Homoacetogenesis: A potentially underappreciated carbon pathway in peatlands. Soil Biol. Biochem. 68, 385–391. doi: 10.1016/j.soilbio.2013.10.020
Yu, Z., Loisel, J., Brosseau, D. P., Beilman, D. W., and Hunt, S. J. (2010). Global peatland dynamics since the last glacial maximum. Geophys. Res. Lett. 37:L13402. doi: 10.1029/2010GL043584
Zeibich, L., Schmidt, O., and Drake, H. L. (2019). Fermenters in the earthworm gut: Do transients matter? FEMS Microbiol. Ecol. 95:fiy221. doi: 10.1093/femsec/fiy221
Keywords: anaerobes, acetogenesis, methanogensis, peatlands, microbiome, wetland plant roots, clostridiaceae, holophagaceae
Citation: Meier AB, Oppermann S, Drake HL and Schmidt O (2022) The root zone of graminoids: A niche for H2-consuming acetogens in a minerotrophic peatland. Front. Microbiol. 13:978296. doi: 10.3389/fmicb.2022.978296
Received: 25 June 2022; Accepted: 19 July 2022;
Published: 05 August 2022.
Edited by:
Marc Gregory Dumont, University of Southampton, United KingdomReviewed by:
Chris Greening, Monash University, AustraliaCopyright © 2022 Meier, Oppermann, Drake and Schmidt. This is an open-access article distributed under the terms of the Creative Commons Attribution License (CC BY). The use, distribution or reproduction in other forums is permitted, provided the original author(s) and the copyright owner(s) are credited and that the original publication in this journal is cited, in accordance with accepted academic practice. No use, distribution or reproduction is permitted which does not comply with these terms.
*Correspondence: Oliver Schmidt, b2xpdmVyLnNjaG1pZHRAdWl0Lm5v
Disclaimer: All claims expressed in this article are solely those of the authors and do not necessarily represent those of their affiliated organizations, or those of the publisher, the editors and the reviewers. Any product that may be evaluated in this article or claim that may be made by its manufacturer is not guaranteed or endorsed by the publisher.
Research integrity at Frontiers
Learn more about the work of our research integrity team to safeguard the quality of each article we publish.