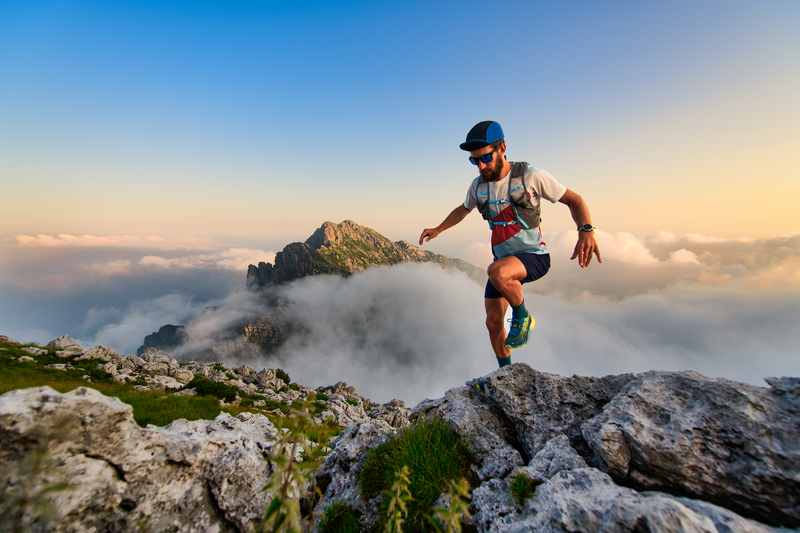
94% of researchers rate our articles as excellent or good
Learn more about the work of our research integrity team to safeguard the quality of each article we publish.
Find out more
ORIGINAL RESEARCH article
Front. Microbiol. , 08 September 2022
Sec. Aquatic Microbiology
Volume 13 - 2022 | https://doi.org/10.3389/fmicb.2022.971433
Aphanizomenon flos-aquae (A. flos-aquae) blooms are serious environmental and ecological problems. Extracellular polymeric substances (EPSs) are among the most important indicators for the growth and aggregation of A. flos-aquae. In this study, the secretion of the EPS matrix under temperature rise (7–37°C) was investigated and the role of this matrix in A. flos-aquae aggregation was quantified. First, when the temperature increased, the aggregation ratio increased from 41.85 to 91.04%. Meanwhile, we found that when soluble EPSs (S-EPSs), loosely bound EPSs (LB-EPSs), and tightly bound EPSs (TB-EPSs) were removed successively, the aggregation ratio decreased from 69.29 to 67.45%, 61.47%, and 41.14%, respectively. Second, the content of polysaccharides in the EPS matrix was higher than the content of proteins under temperature change. The polysaccharide in TB-EPSs was closely related to the aggregation ability of A. flos-aquae (P < 0.01). Third, PARAFAC analysis detected two humic-like substances and one protein-like substance in EPSs. Furthermore, Fourier transforms infrared spectroscopy (FTIR) showed that with increasing temperature, the polysaccharide-related functional groups increased, and the absolute value of the zeta potential decreased. In conclusion, these results indicated that a large number of polysaccharides in TB-EPSs were secreted under increasing temperature, and the polysaccharide-related functional groups increased correspondingly, which reduced the electrostatic repulsion between algal cells, leading to the destruction of the stability of the dispersion system, and then the occurrence of aggregation. This helps us to understand the process of filamentous cyanobacterial aggregation in lakes.
Seasonal algal blooms are increasingly threatening ecosystem functions and human health in Lake, with Microcystis predominating in summer and Aphanizomenon flos-aquae (A. flos-aquae) predominating in spring and autumn, while cyanobacteria often show seasonal replacement in Lake (Tang et al., 2019; Weber et al., 2020). Seasonal variations are reflected in temperature changes, which directly or indirectly affect the growth rate, reproduction rate, and enzyme activity of algal cells by affecting photosynthesis and respiration (Yang et al., 2020). More importantly, a large area of A. flos-aquae has appeared in some lakes during the summer in recent years. Many lakes and reservoirs have A. flos-aquae blooms, including Lake Dianchi in China (Jin et al., 2022), Lake Biwa in Japan (Yamamoto, 2009), the Baltic Sea in Europe (Laamanen et al., 2002), and Lake Ontario in Hamilton Harbour (Zastepa and Chemali, 2021), with severe eutrophic problems. A. flos-aquae is a toxigenic filamentous cyanobacterium that exhibits increased growth at high temperatures and remains active at low temperatures with high density (Yamamoto, 2009; Jin et al., 2022).
Aside from the direct effects on cyanobacterial density, higher temperatures increase nutrient diffusion to the cell surface, which may favor cyanobacterial growth (Peperzak, 2003). The external environment affects algal cells, and self-regulation changes the secretion of proteins, polysaccharides, lipids, and other extracellular polymeric substances (EPSs) to adapt to the environment (Brennan and Owende, 2010; Scott et al., 2010; Schlesinger et al., 2012). EPSs thus play an important role in relieving external stress on algal cells in the absence of additional energy input. In some algal species, EPSs are viscous polymers composed of polysaccharides, proteins, and other substances produced during cell growth that contribute to cell aggregation (Sheng et al., 2010). Because of the presence of multiple functional groups, the cyanobacterial EPS matrix can consistently bind to the surface of colloidal particles, influencing their stability/aggregation propensity (Lin et al., 2016; Si et al., 2019). Algae typically aggregate and migrate to the water surface, causing blooms (Ramesh et al., 2006), and filamentous cyanobacteria are gaining attention. This prompted us to investigate whether A. flos-aquae aggregation is related to EPSs.
Extracellular polymeric substances were divided into three fractions soluble EPS (SL-EPS), loosely bound EPS (LB-EPS), and tightly bound EPS (TB-EPS) (Xu et al., 2013a). The majority of organic matter is distributed in TB-EPS, with a minor portion distributed in S-EPS and LB-EPS (Xu et al., 2013b). Polysaccharides are mostly found in TB-EPS, while proteins are mostly found in S-EPS and LB-EPS. Takeda et al. (1992) investigated the EPS aggregation mechanism of Nocardia amarae and discovered that proteins were highly active. Allen et al. (2004) discovered that Thauera sp. aggregation in a sewage system was closely related to polysaccharides in EPSs. These disparate results prompted us to consider which specific components of the EPS matrix had the greatest influence on the aggregation of A. flos-aquae. To the best of our knowledge, however, this information has not been elucidated.
Therefore, to reveal the bloom formation mechanism of A. flos-aquae, it is necessary to study its aggregation characteristics. In this study, by combining physical and chemical analyses, the fluorescence excitation-emission matrix (EEM), Fourier transform infrared spectroscopy (FTIR), and the zeta potential by simulating seasonal changes under increasing temperatures, the effects of the release of EPSs on the aggregation ability of A. flos-aquae under temperature changes were investigated. The aggregation rate and the content of the components of EPSs were examined for correlations. The findings contribute to a better understanding of filamentous cyanobacterial aggregation behavior and provide theoretical support for cyanobacteria control in lakes.
The cyanobacterial strain Aphanizomenon flos-aquae FACHB-1039, which was isolated from cyanobacterial blooms, was provided by the Institute of Hydrobiology, Chinese Academy of Sciences, and it was cultured in batch mode in conical flasks. Exponentially growing A. flos-aquae was inoculated into the BG-11 medium an initial absorbance of 0.1 and cultured under a light: dark cycle of 12:12 at a light intensity of 30 μmol photons m–2 s–1. Growth was monitored by measuring the absorbance of A. flos-aquae cultures at 680 nm using a spectrophotometer (Tecan SPARK, Austria). To simulate a temperature change, the culture temperature was increased by 2°C every 3 days from 7 to 37°C before being cultured at 7°C for a week. Algal samples were collected on the last day of every temperature setting for aggregation potential measurement and EPS extraction. All flasks were shaken three times per day at regular intervals during the experimental period. Each treatment was conducted in triplicate.
The aggregation ability of the algal cells is usually evaluated by the aggregation rate (Tan et al., 2018). The aggregation rate was determined according to Xu et al. (2014). At 680 nm, the optical density of the A. flos-aquae sample was determined (A0). After 6 h of standing in a clean test tube, the algal liquid from 2 cm of the upper layer was carefully removed and the optical density was measured again at 680 nm (At). The aggregation ratio of the A. flos-aquae samples was calculated by using the following equation:
Extracellular polymeric substances consist of S-EPS, LB-EPS, and TB-EPS (Li and Yang, 2007; Sheng et al., 2010; Xu et al., 2010). In this study, EPS extraction was performed according to Xu et al. (2013a).
The three-dimensional excitation-emission matrix fluorescence spectrum (3D-EEM) was measured using a fluorescence spectrometer (LF-1301009, Thermo Fisher, United States) and the measurement was carried out in accordance with Xu et al. (2013b). The difference is that the emission (Em) and excitation (Ex) wavelengths range from 250 to 450 nm at 5 nm increments and from 200 to 550 nm at 10 nm increments, respectively. Other Rayleigh and Raman scattering data were filtered using interpolation adopted from Bahram et al. (2006). PARAFAC modeling was carried out for all EEM spectra of the EPS samples collected over the whole growth period (Yamashita and Jaffé, 2008). PARAFAC analysis was conducted in Matlab 2016b (Mathworks, Natick, MA, United States) using the DOMFluor toolbox.1 No outliers were found by leverage comparison, and a total of 48 fluorescence EEM data arrays (48 samples × 21 Ex × 21 Em) were obtained for the PARAFAC models, and 2–7 components were computed. The residual analysis was conducted according to Stedmon and Bro (2008).
Extracellular polymeric substance extractions were completely dried to powder in a freeze dryer. After freeze-drying, the EPS samples and dried KBr were mixed at a ratio of 1:100 and homogenized in an agate grinder. In total, 150 mg of the mixture was compressed and analyzed using a spectrum FTIR spectrometer (Nexus 670, Nicolet). The scanning conditions were as follows: a spectral range of 4000–400 cm–1, 32 scans and a resolution of 4 cm–1.
The zeta potential represents the electrostatic force on the cell surface, and it can be used to evaluate the stability of dispersions (Tan et al., 2020). The zeta potential was measured at 25°C using a Zeta sizer (Nano ZS90, Malvern Instruments Ltd., United Kingdom), according to Hadjoudja et al. (2010). This method was carried out using Doppler electrophoresis, which is based on the relative displacement of charged particles in an applied electric field. A 50 μL sample was taken and diluted 100 times with PBS solution before the test. Three copies of each measurement were taken.
Contact angle measurement (θ) was performed by using a contact angle analyzer (JC2000D, Powereach, Shanghai, China), according to the standard sessile drop method (Van Oss, 1995). Pure water was dropped onto the membrane containing A. flos-aquae. The arithmetic means of at least seven independent measurements were used to calculate all contact angle values.
All chemicals used in this work were of analytical grade. Data are presented as the means ± standard deviations. Significant differences were analyzed by one-way analysis of variance (ANOVA). SPSS 22.0 was used for all statistical analyses. Pearson correlation was performed to describe the relationships among the EPS content, aggregation ability and algal growth.
Using glucose as a standard, the polysaccharide content was determined using the phenol-sulfuric acid method (Dubois et al., 1956). Protein contents were determined according to Bradford (1976) with bovine serum albumin as a standard. Cell density was measured at an absorbance of 680 nm. Furthermore, maximum photoelectron production (Fv/Fm) and actual photoelectron production (Fv′/Fm′) were measured using a pulse amplitude-modulated fluorometer (AquaPen, PSI, Czechia).
The density of A. flos-aquae generally showed an increasing trend with increasing temperature (Figure 1A). At low temperature, the algal density was low, and it recovered at about 15°C, changed significantly at 20°C, and reached a stable growth state at 35°C.
Figure 1. Effects of rising temperature on the growth of A. flos-aquae and aggregation with temperature. (A) Variation of algal density with temperature; (B) Variation of photosynthetic activity with temperature; (C) Variation of aggregation ratio with temperature.
The photosynthetic activity first increased and then decreased with increasing temperature (Figure 1B). Fv/Fm increased gradually from 7 to 21°C, and reached a maximum value of 0.45 at 21°C. At this temperature, the specific growth rate also reached a maximum value, indicating that the optimal growth temperature was 21°C.
The aggregation ratio of A. flos-aquae gradually increased from the initial 41.85–91.04% with increasing temperature (Figure 1C). At 7–11°C, the aggregation rate gradually decreased. From 11 to 27°C, aggregation increased with increasing temperature. It reached the maximum value at 31°C (91.04%) and then tended to a stable state, indicating that increasing temperature promoted aggregation of A. flos-aquae. Many factors influence the aggregation, including the surface properties of algal cells, which have a direct impact on the aggregation of the cells (Xu et al., 2016).
Under increasing temperature, the polysaccharide content continued to increase and reached a stable state at 33°C. Among EPSs, the polysaccharide TB-EPS fraction exhibited a significant increase during the whole process, showing a significant correlation with the growth and aggregation of A. flos-aquae (Table 1). Additionally, the ratio of polysaccharides in EPSs changed. For example, the proportion of polysaccharides in the TB-EPS component increased from an initial 8% (3.05 mg/L out of 37.04 mg/L) to 77% (21.72 mg/L out of 28.19 mg/L) at 31°C. Similarly, the ratio of proteins in the EPSs also changed. As shown, the content of polysaccharides in the EPS matrix was higher than that of proteins, regardless of the temperature differences. Furthermore, it was clear that high temperature (29–37°C) induced a greater release of EPSs compared with low temperature (7–15°C).
Table 1. Pearson correlation analysis of the aggregation, algal density, zeta potential, and protein and polysaccharide in EPS components.
The changes in the aggregation ratio during the extraction of EPSs at the optimum growth temperature of A. flos-aquae showed that when the EPS matrix was extracted, the aggregation ratio of A. flos-aquae decreased (Figure 2C). This finding indicated that the EPS matrix played a more important role in A. flos-aquae aggregates. Among EPSs, the aggregation ratio after extraction of TB-EPS decreased significantly, which may be related to the substance composition of TB-EPS.
Figure 2. Variations of composition in EPS matrix with temperature and the relationship between aggregation and EPS fractions for A. flos-aquae. (A) Variation of polysaccharides with temperature; (B) Variation of protein with temperature; (C) Variation of aggregation with removed EPS fraction in turn.
The EEM spectra of EPSs at different temperatures showed that the fluorescence intensity changed significantly with the increase in temperature, but the organic composition in the EPS matrix did not change. Specifically, S-EPS exhibited two humic-like substances (peak A and peak B) and one protein-like substances (peak C), while both LB-EPS (peak C) and TB-EPS (peak D) exhibited a protein-like substances (Figure 3). The results of the EEM contours demonstrated that protein-like substances were detected in all fractions of EPSs, whereas humic-like substances were mainly distributed in the S-EPS fraction.
Figure 3. EEM spectra of EPS fractions of A. flos-aquae at different temperatures (peak A and peak B standed for humic-like, peak C and peak D standed protein). (A–C) Represented the EEM diagram of S-EPS at 11, 21, and 31°C; (D–F) represented the EEM diagram of LB-EPS at 11, 21, and 31°C; (G–I) represented the EEM diagram of TB-EPS at 11, 21, and 31°C.
The DOMFluor-PARAFAC results indicated the Ex/Em loading of the three components (Figure 4). The fluorescence spectra revealed three components: a protein-like component and two humic-like components. Component 1 (C1) and Component 2 (C2) were defined as humic-like substances, with C1 having a much wider Ex spectrum than C2. Component 3 (C3), which exhibited a single Ex/Em peak of 280/350 nm, was identified as a tryptophan-like substance (Xu et al., 2013a). The proportion of the fluorescence intensity of the three components (C1–C3) in the three fractions of EPSs differed under temperature changes (Figure 4). Although EEM-PRAFAC analysis has been used for the characterization of EPSs, it has not been applied to investigate A. flos-aquae growth to date. The scatter plots of the PARAFAC derived component scores are shown in Figure 5. C3 had the highest fluorescence intensity scores in the S-EPS fraction, followed by C1 and C2. In the TB-EPS fraction, however, C2 had the highest fluorescence intensity scores, followed by C3 and C1. C3 had the highest fluorescence intensity scores in the LB-EPS fraction, at low temperatures, C2 > C1, and with increasing temperatures, C1 > C2. The fluorescence intensity scores of C1 and C2 in all fractions of EPSs, as well as of C3 in the S-EPS and TB-EPS fractions, increased as the temperature increased. Humic-like substances in the S-EPS fraction were positively correlated with A. flos-aquae growth, whereas tryptophan-like and humic-like substances were associated with A. flos-aquae growth in the TB-EPS fraction.
Figure 4. EEM contours, emission (solid lines) and excitation (dotted lines) loading of the three components identified by the DOMFluor-PARAFAC analysis for EPS produced by A. flos-aquae (Component 1, Component 2, and Component 3 were obtained by PARAFAC of 48 fluorescence EEM data arrays).
Figure 5. The scatter plots of fluorescence intensity scores in different EPS fractions with increasing temperature of A. flos-aquae (C1 and C2 defined as humic-like substances, C3 defined as tryptophan-like substances; R stands for Pearson correlation coefficient; p represents the significance level).
The characteristic peaks of the FTIR spectra of EPSs varied significantly at different temperatures, mainly in the part of the functional groups related to polysaccharides (Figure 6). Specifically, the broad band at 3450 cm–1 was attributed to the stretching vibrations of both O-H (of polysaccharides) and N-H (of proteins), the band at 2430 cm–1 was attributed to -COO- stretching vibrations (Ueshima et al., 2008), and the band at 1790 cm–1 was attributed to C = O stretching vibrations (Song et al., 2014). Proteins are associated with the amide I (1700–1600 cm–1), amide II (1600–1500 cm–1), and amide III (1300–1200 cm–1) ranges (Ueshima et al., 2008; Wang et al., 2012; Fang et al., 2019), while polysaccharides and nucleic acids are associated with the 1200–900 cm–1 range (Yuan et al., 2011). Thus, bands attributed to the C-O-C ring and C-O stretching vibrations at 1150–1140 cm–1 were derived from polysaccharides (Yuan et al., 2011; Fang et al., 2019). These functional groups of EPS are closely related to the chemical properties (Yu et al., 2012; Zhu et al., 2012). In this study, the comparison of the functional groups changes at different temperatures showed that EPSs had obvious spectral changes in the band range of 3450 cm–1 and 1200–900 cm–1 at different temperatures. Polysaccharide synthesis was inhibited at low temperature, resulting in fewer polysaccharide-related functional groups. As the temperature increased, the algal cells secreted more polysaccharides, and more functional groups were discovered.
The surface properties of algal cells, which are primarily determined by surface charge and hydrophilicity, influence cell aggregation. The measured contact angle and zeta potential data are shown in Table 2. The higher the absolute value of the zeta potential, the stronger the electrostatic repulsion on the surface of the algae and the less likely they are to aggregate. As shown, from 7 to 35°C, the zeta potential of the algal surface decreased from –15.29 ± 1.297 mV to –17.43 ± 1.19 mV and then increased to –13.77 ± 1.60 mV. The absolute value of the zeta potential increased first and then decreased with the increase in temperature. This indicated that the higher the temperature, the less negative the charge on the surface, the lower the repulsion between the cells, and the greater the aggregation ability. The lower surface electronegativity of A. flos-aquae made it more conducive to aggregation. This trend was consistent with the change in the aggregation rate. In addition, the change of the zeta potential was related to polysaccharides in LB-EPS and TB-EPS (Table 1).
The contact angle between samples and water can characterize hydrophobicity. The results showed that A. flos-aquae at all experimental temperatures was characterized as being hydrophilic (θH2 OθH2O ≤ 90°), and the algae were the least hydrophobic at 15°C, and the hydrophilicity gradually increased with the increase in temperature.
Cyanobacteria have a competitive advantage due to their higher growth rate at higher temperatures (Paerl and Huisman, 2008). Due to the increase in algal cell accumulation and growth rate, the biomass of A. flos-aquae in our increasing temperature experiment showed an increasing tendency as the temperature increased from 11 to 35°C (Figure 1A). This is consistent with the research findings under the influence of temperature on cyanobacterial biomass in Lake Taihu (Yang et al., 2020). Although rising temperatures promote cyanobacterial growth, high temperatures do not promote the formation of large colonies (Zhu et al., 2016), and temperatures that are too high can result in damage to proteins, DNA, and lipids and modulation of membrane stability in algal cells (Babele et al., 2017). Our simulation experiments also revealed a decreasing trend in photosynthetic activity and growth rate as the temperature gradient increased to 21 and 31°C, respectively (Figure 1B). Thus, 20–30°C may be the optimum growth temperature for A. flos-aquae.
Beyond the direct effects on cyanobacterial growth rates, higher temperatures increase nutrient diffusion toward the cell surface which may be beneficial to cyanobacteria (Peperzak, 2003). EPSs are distributed on the surface of algal cells and are mainly composed of proteins and polysaccharides (Richert et al., 2005). Therefore, the quantitative study of extracellular polysaccharides and proteins during the rising temperature process revealed the same results as Vogel (1995). The concentration of extracellular protein and polysaccharide increased gradually with increasing temperature (Figure 2), indicating that the higher temperature promoted the release of organic matter by algae cells to the external environment.
Extracellular polymeric substances have been reported to increase the aggregation ability of algal cells to adapt to the external environment (Su and Yu, 2005; Yang et al., 2012). Therefore, this study explored the relationship between the EPS matrix and A. flos-aquae aggregation (Figure 2C) and discovered that the aggregation ratio gradually decreased after the removal of S-EPS, LB-EPS, and TB-EPS, indicating that EPSs play a significant role in A. flos-aquae aggregation, which is consistent with the results reported by Xu et al. (2014). A variety of biomacromolecules in EPSs has been reported to have high activity, such as proteins produced by Nocardia amarae (Takeda et al., 1992), polysaccharides from Thauera sp. (Allen et al., 2004), and glycoprotein from Arcuadendron sp. (Lee et al., 1995). In this study, it was found that the polysaccharide activity in TB-EPS was higher, which was closely related to the aggregation of A. flos-aquae (P < 0.01). The composition and principal functional groups of the EPS matrix were further studied by 3D-EEM and FTIR, and it was found that organic matter in TB-EPS was related to the growth of A. flos-aquae and the polysaccharide functional groups dominated (Figure 6). This is in agreement with the results obtained by Xu et al. (2014). However, Xu et al. (2014) found that each fraction of EPS exhibited two fluorescence peaks, which is different from the results of this study. In addition, compared with the peak locations reported previously (Sheng and Yu, 2006; Ji et al., 2021), the location peaks in this study showed shifts. On the one hand, this may be due to the specificity of A. flos-aquae. On the other hand, it may be caused by differences in the extraction methods used for EPSs. This study also measured the zeta potential of A. flos-aquae, and it was discovered that the surface charge of algal cells changed with temperature, which may be related to the composition of EPSs. As a result of these changes, the aggregation of A. flos-aquae changed with temperature.
In this study, the EPSs of A. flos-aquae were divided into three fractions, S-EPS, LB-EPS, and TB-EPS, to investigate the effect of EPSs on the aggregation process of A. flos-aquae as the temperature increased. The results showed that TB-EPS might play an important role in the formation of aggregates of A. flos-aquae. Polysaccharides in TB-EPS, in particular, contributed significantly to algal cell aggregation. With multiple methods, we concluded that as the temperature increased, many polysaccharides were synthesized and released into the extracellular environment, reducing the electrostatic repulsion between cells and promoting cell aggregation. This work provides basic information on the aggregation of A. flos-aquae, which helps us to understand the process of filamentous cyanobacterial aggregation in lakes.
The original contributions presented in this study are included in the article/supplementary material, further inquiries can be directed to the corresponding authors.
DD: investigation, methodology, data analysis, and writing – original draft. HM: investigation, methodology, conceptualization, data analysis, and writing – review and editing. YM: investigation, methodology, and data analysis. YG and ZW: methodology and investigation. HH: supervision and writing – review and editing. J-eL: methodology, investigation, conceptualization, supervision, and writing – review and editing. LZ: methodology, investigation, conceptualization, supervision, and writing – review and editing. All authors contributed to the article and approved the submitted version.
This work was supported by the National Science Foundation of China (grant number: 41877336, 41907202, 91951112, and 41773077), Project funded by China Postdoctoral Science Foundation (2019M651877), Natural Science Found of Jiangsu Province (SBK2019043965), and Yancheng fishery high quality development project (YCSCYJ2021030).
The authors declare that the research was conducted in the absence of any commercial or financial relationships that could be construed as a potential conflict of interest.
All claims expressed in this article are solely those of the authors and do not necessarily represent those of their affiliated organizations, or those of the publisher, the editors and the reviewers. Any product that may be evaluated in this article, or claim that may be made by its manufacturer, is not guaranteed or endorsed by the publisher.
Allen, M. S., Welch, K. T., Prebyl, B. S., Baker, D. C., Meyers, A. J., and Sayler, G. S. (2004). Analysis and glycosyl composition of the exopolysaccharide isolated from the floc-forming wastewater bacterium Thauera sp. MZ1T. Environ. Microbiol. 6, 780–790. doi: 10.1111/j.1462-2920.2004.00615.x
Babele, P. K., Singh, G., Singh, A., Kumar, A., Tyagi, M. B., and Sinha, R. P. (2017). UV-B radiation and temperature stress-induced alterations in metabolic events and defense mechanisms in a bloom-forming cyanobacterium Microcystis aeruginosa. Acta Physiol. Plant. 39:248. doi: 10.1007/s11738-017-2540-4
Bahram, M., Bro, R., Stedmon, C., and Afkhami, A. (2006). Handling of Rayleigh and Raman scatter for PARAFAC modeling of fluorescence data using interpolation. J. Chemom. 20, 99–105. doi: 10.1002/cem.978
Bradford, M. M. (1976). Interactive effects of drought stress and chitosan application on physiological characteristics and essential oil yield of Thymus daenensis Celak. Crop J. 5, 407–415. doi: 10.1016/j.cj.2017.04.003
Brennan, L., and Owende, P. (2010). Biofuels from microalgae-A review of technologies for production, processing, and extractions of biofuels and co-products. Renew. Sustain. Energy Rev. 14, 557–577. doi: 10.1016/j.rser.2009.10.009
Dubois, M., Gilles, K. A., Hamilton, J. K., Rebers, P. A., and Smith, F. (1956). Colorimetric Method for Determination of Sugars and Related Substances. Anal. Chem. 28, 350–356. doi: 10.1021/ac60111a017
Fang, F., Xu, R. Z., Wang, S. N., Zhang, L. L., Huang, Y. Q., Luo, J. Y., et al. (2019). Characterization of interactions between a metabolic uncoupler O-chlorophenol and extracellular polymeric substances of activated sludge. Environ. Pollut. 247, 1020–1027. doi: 10.1016/j.envpol.2019.02.005
Hadjoudja, S., Deluchat, V., and Baudu, M. (2010). Cell surface characterisation of Microcystis aeruginosa and Chlorella vulgaris. J. Colloid Interface Sci. 342, 293–299. doi: 10.1016/j.jcis.2009.10.078
Ji, C. C., Zhou, H., Deng, S. K., Chen, K. Y., Dong, X. Y., Xu, X. H., et al. (2021). Insight into the adhesion propensities of extracellular polymeric substances (EPS) on the abiotic surface using XDLVO theory. J. Environ. Chem. Eng. 9:106563. doi: 10.1016/j.jece.2021.106563
Jin, H., Ma, H., Gan, N., Wang, H., Li, Y., Wang, L., et al. (2022). Non-targeted metabolomic profiling of filamentous cyanobacteria Aphanizomenon flos-aquae exposed to a concentrated culture filtrate of Microcystis aeruginosa. Harmful Algae 111:102170. doi: 10.1016/j.hal.2021.102170
Laamanen, M. J., Forsström, L., and Sivonen, K. (2002). Diversity of Aphanizomenon flos-aquae (cyanobacterium) populations along a Baltic Sea salinity gradient. Appl. Environ. Microbiol. 68, 5296–5303. doi: 10.1128/AEM.68.11.5296-5303.2002
Lee, S. H., Lee, S. O., Jang, K. L., and Lee, T. H. (1995). Microbial flocculant from Arcuadendron sp. TS-49. Biotechnol. Lett. 17, 95–100. doi: 10.1007/BF00134203
Li, X. Y., and Yang, S. F. (2007). Influence of loosely bound extracellular polymeric substances (EPS) on the flocculation, sedimentation and dewaterability of activated sludge. Water Res. 41, 1022–1030. doi: 10.1016/j.watres.2006.06.037
Lin, D., Drew Story, S., Walker, S. L., Huang, Q. Y., and Cai, P. (2016). Influence of extracellular polymeric substances on the aggregation kinetics of TiO 2 nanoparticles. Water Res. 104, 381–388. doi: 10.1016/j.watres.2016.08.044
Paerl, H. W., and Huisman, J. (2008). Climate: Blooms like it hot. Science 320, 57–58. doi: 10.1126/science.1155398
Peperzak, L. (2003). Climate change and harmful algal blooms in the North Sea. Acta Oecol. 24:S139–S144. doi: 10.1016/S1146-609X(03)00009-2
Ramesh, A., Lee, D. J., and Hong, S. G. (2006). Soluble microbial products (SMP) and soluble extracellular polymeric substances (EPS) from wastewater sludge. Appl. Microbiol. Biotechnol. 73, 219–225. doi: 10.1007/s00253-006-0446-y
Richert, L., Golubic, S., Le Guédès, R., Ratiskol, J., Payri, C., and Guezennec, J. (2005). Characterization of exopolysaccharides produced by cyanobacteria isolated from Polynesian microbial mats. Curr. Microbiol. 51, 379–384. doi: 10.1007/s00284-005-0069-z
Schlesinger, A., Eisenstadt, D., Bar-Gil, A., Carmely, H., Einbinder, S., and Gressel, J. (2012). Inexpensive non-toxic flocculation of microalgae contradicts theories; overcoming a major hurdle to bulk algal production. Biotechnol. Adv. 30, 1023–1030. doi: 10.1016/j.biotechadv.2012.01.011
Scott, S. A., Davey, M. P., Dennis, J. S., Horst, I., Howe, C. J., Lea-Smith, D. J., et al. (2010). Biodiesel from algae: Challenges and prospects. Curr. Opin. Biotechnol. 21, 277–286. doi: 10.1016/j.copbio.2010.03.005
Sheng, G. P., and Yu, H. Q. (2006). Characterization of extracellular polymeric substances of aerobic and anaerobic sludge using three-dimensional excitation and emission matrix fluorescence spectroscopy. Water Res. 40, 1233–1239. doi: 10.1016/j.watres.2006.01.023
Sheng, G. P., Yu, H. Q., and Li, X. Y. (2010). Extracellular polymeric substances (EPS) of microbial aggregates in biological wastewater treatment systems: A review. Biotechnol. Adv. 28, 882–894. doi: 10.1016/j.biotechadv.2010.08.001
Si, W., Xu, H. C., Kong, M., Liu, J., Xu, M. W., and Liu, X. (2019). Effects of molecular weight fractions and chemical properties of time-series cyanobacterial extracellular polymeric substances on the aggregation of lake colloidal particles. Sci. Total Environ. 692, 1201–1208. doi: 10.1016/j.scitotenv.2019.07.360
Song, C., Sun, X. F., Xing, S. F., Xia, P. F., Shi, Y. J., and Wang, S. G. (2014). Characterization of the interactions between tetracycline antibiotics and microbial extracellular polymeric substances with spectroscopic approaches. Environ. Sci. Pollut. Res. 21, 1786–1795. doi: 10.1007/s11356-013-2070-6
Stedmon, C. A., and Bro, R. (2008). Characterizing dissolved organic matter fluorescence with parallel factor analysis: A tutorial. Limnol. Oceanogr. 6, 572–579. doi: 10.4319/lom.2008.6.572
Su, K. Z., and Yu, H. Q. (2005). Formation and characterization of aerobic granules in a sequencing batch reactor treating soybean-processing wastewater. Environ. Sci. Technol. 39, 2818–2827. doi: 10.1021/es048950y
Takeda, M., Koizumi, J. I., Matsuoka, H., and Hikuma, M. (1992). Factors affecting the activity of a protein bioflocculant produced by Nocardia amarae. J. Ferment. Bioeng. 74, 408–409. doi: 10.1016/0922-338X(92)90043-T
Tan, L. R., Xia, P. F., Zeng, R. J., Li, Q., Sun, X. F., and Wang, S. G. (2018). Low-level concentrations of aminoglycoside antibiotics induce the aggregation of cyanobacteria. Environ. Sci. Pollut. Res. 25, 17128–17136. doi: 10.1007/s11356-018-1894-5
Tan, X., Shu, X., Duan, Z., and Parajuli, K. (2020). Two types of bound extracellular polysaccharides and their roles in shaping the size and tightness of Microcystis colonies. J. Appl. Phycol. 32, 255–262. doi: 10.1007/s10811-019-01937-z
Tang, Y., Zhang, M. Y., Sun, G. X., and Pan, G. (2019). Impact of eutrophication on arsenic cycling in freshwaters. Water Res. 150, 191–199. doi: 10.1016/j.watres.2018.11.046
Ueshima, M., Ginn, B. R., Haack, E. A., Szymanowski, J. E. S., and Fein, J. B. (2008). Cd adsorption onto Pseudomonas putida in the presence and absence of extracellular polymeric substances. Geochim. Cosmochim. Acta 72, 5885–5895. doi: 10.1016/j.gca.2008.09.014
Vogel, S. (1995). Life in moving fluids: The physical biology of flow. Bull. Math. Biol. 57, 949–951. doi: 10.2307/1352661
Wang, L. L., Wang, L. F., Ren, X. M., Ye, X. D., Li, W. W., Yuan, S. J., et al. (2012). PH dependence of structure and surface properties of microbial EPS. Environ. Sci. Technol. 46, 737–744. doi: 10.1021/es203540w
Weber, S. J., Mishra, D. R., Wilde, S. B., and Kramer, E. (2020). Risks for cyanobacterial harmful algal blooms due to land management and climate interactions. Sci. Total Environ. 703:134608. doi: 10.1016/j.scitotenv.2019.134608
Xu, H. C., Cai, H. Y., Yu, G. H., and Jiang, H. L. (2013a). Insights into extracellular polymeric substances of cyanobacterium Microcystis aeruginosa using fractionation procedure and parallel factor analysis. Water Res. 47, 2005–2014. doi: 10.1016/j.watres.2013.01.019
Xu, H. C., Yu, G. H., and Jiang, H. L. (2013b). Investigation on extracellular polymeric substances from mucilaginous cyanobacterial blooms in eutrophic freshwater lakes. Chemosphere 93, 75–81. doi: 10.1016/j.chemosphere.2013.04.077
Xu, H. C., He, P. J., Wang, G. Z., and Shao, L. M. (2010). Three-dimensional excitation emission matrix fluorescence spectroscopy and gel-permeating chromatography to characterize extracellular polymeric substances in aerobic granulation. Water Sci. Technol. 61, 2931–2942. doi: 10.2166/wst.2010.197
Xu, H. C., Jiang, H. L., Yu, G. H., and Yang, L. Y. (2014). Towards understanding the role of extracellular polymeric substances in cyanobacterial Microcystis aggregation and mucilaginous bloom formation. Chemosphere 117, 815–822. doi: 10.1016/j.chemosphere.2014.10.061
Xu, J., Yu, H. Q., and Sheng, G. P. (2016). Kinetics and thermodynamics of interaction between sulfonamide antibiotics and humic acids: Surface plasmon resonance and isothermal titration microcalorimetry analysis. J. Hazard. Mater. 302, 262–266. doi: 10.1016/j.jhazmat.2015.09.058
Yamamoto, Y. (2009). Environmental factors that determine the occurrence and seasonal dynamics of Aphanizomenon flos-aquae. J. Limnol. 68, 122–132. doi: 10.4081/jlimnol.2009.122
Yamashita, Y., and Jaffé, R. (2008). Characterizing the interactions between trace metals and dissolved organic matter using excitation-emission matrix and parallel factor analysis. Environ. Sci. Technol. 42, 7374–7379. doi: 10.1021/es801357h
Yang, S. F., Lin, C. F., Wu, C. J., Ng, K. K., Yu-Chen Lin, A., and Andy Hong, P. K. (2012). Fate of sulfonamide antibiotics in contact with activated sludge - Sorption and biodegradation. Water Res. 46, 1301–1308. doi: 10.1016/j.watres.2011.12.035
Yang, Z., Zhang, M., Yu, Y., and Shi, X. (2020). Temperature triggers the annual cycle of Microcystis, comparable results from the laboratory and a large shallow lake. Chemosphere 260:127543. doi: 10.1016/j.chemosphere.2020.127543
Yu, G. H., Wu, M. J., Wei, G. R., Luo, Y. H., Ran, W., Wang, B. R., et al. (2012). Binding of organic ligands with Al(III) in dissolved organic matter from soil: Implications for soil organic carbon storage. Environ. Sci. Technol. 46, 6102–6109. doi: 10.1021/es3002212
Yuan, S. J., Sun, M., Sheng, G. P., Li, Y., Li, W. W., Yao, R. S., et al. (2011). Identification of key constituents and structure of the extracellular polymeric substances excreted by bacillus megaterium TF10 for their flocculation capacity. Environ. Sci. Technol. 45, 1152–1157. doi: 10.1021/es1030905
Zastepa, A., and Chemali, C. (2021). Bloom announcement: Late season cyanobacterial blooms co-dominated by Microcystis flos-aquae, Lyngbya birgei, and Aphanizomenon flos-aquae complex in Hamilton Harbour (Lake Ontario), an area of concern impacted by industrial effluent and residential waste. Data Brief 35:106800. doi: 10.1016/j.dib.2021.106800
Zhu, L., Qi, H. Y., Lv, M. L., Kong, Y., Yu, Y. W., et al. (2012). Component analysis of extracellular polymeric substances (EPS) during aerobic sludge granulation using FTIR and 3D-EEM technologies. Bioresour. Technol. 124, 455–459. doi: 10.1016/j.biortech.2012.08.059
Keywords: cyanobacterial bloom, temperature, Aphanizomenon flos-aquae, aggregation, extracellular polymeric substances
Citation: Deng D, Meng H, Ma Y, Guo Y, Wang Z, He H, Liu J-e and Zhang L (2022) Effects of extracellular polymeric substances on the aggregation of Aphanizomenon flos-aquae under increasing temperature. Front. Microbiol. 13:971433. doi: 10.3389/fmicb.2022.971433
Received: 28 June 2022; Accepted: 25 August 2022;
Published: 08 September 2022.
Edited by:
Ramesh K. Goel, The University of Utah, United StatesCopyright © 2022 Deng, Meng, Ma, Guo, Wang, He, Liu and Zhang. This is an open-access article distributed under the terms of the Creative Commons Attribution License (CC BY). The use, distribution or reproduction in other forums is permitted, provided the original author(s) and the copyright owner(s) are credited and that the original publication in this journal is cited, in accordance with accepted academic practice. No use, distribution or reproduction is permitted which does not comply with these terms.
*Correspondence: Jin-e Liu, bGl1amluZUBuam51LmVkdS5jbg==; Limin Zhang, bG16aGFuZ0Buam51LmVkdS5jbg==
†These authors have contributed equally to this work and share first authorship
Disclaimer: All claims expressed in this article are solely those of the authors and do not necessarily represent those of their affiliated organizations, or those of the publisher, the editors and the reviewers. Any product that may be evaluated in this article or claim that may be made by its manufacturer is not guaranteed or endorsed by the publisher.
Research integrity at Frontiers
Learn more about the work of our research integrity team to safeguard the quality of each article we publish.