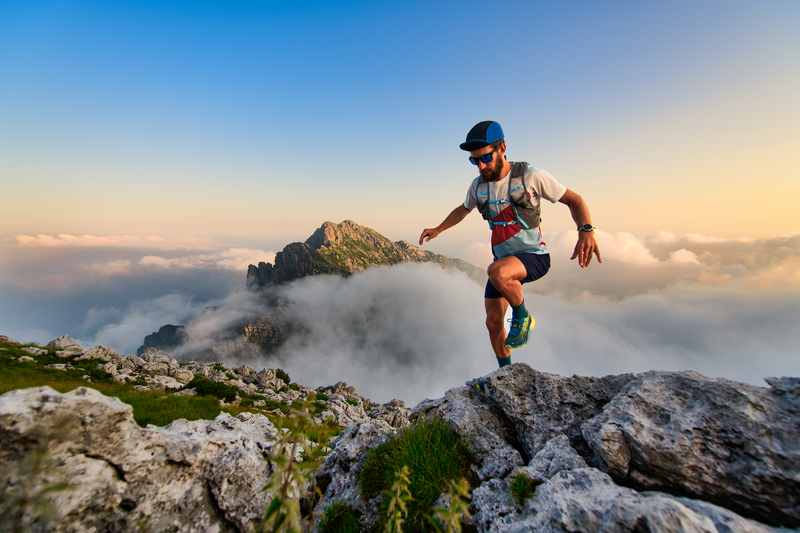
95% of researchers rate our articles as excellent or good
Learn more about the work of our research integrity team to safeguard the quality of each article we publish.
Find out more
ORIGINAL RESEARCH article
Front. Microbiol. , 21 October 2022
Sec. Microbiotechnology
Volume 13 - 2022 | https://doi.org/10.3389/fmicb.2022.965968
This article is part of the Research Topic Microbial Co-cultures: A New Era of Synthetic Biology and Metabolic Engineering View all 16 articles
Ethylene and isoprene are essential platform chemicals necessary to produce polymers and materials. However, their current production methods based on fossil fuels are not very efficient and result in significant environmental pollution. For a successful transition more sustainable economic model, producing these key polymeric building blocks from renewable and sustainable resources such as biomass or CO2 is essential. Here, inspired by the symbiotic relationship of natural microbial communities, artificial consortia composed of E. coli strains producing volatile platform chemicals: ethylene and isoprene and two strains of cyanobacteria phototrophically synthesizing and exporting sucrose to feed these heterotrophs were developed. Disaccharide produced by transgenic cyanobacteria was used as a carbon and electron shuttle between the two community components. The E. coli cscB gene responsible for sucrose transport was inserted into two cyanobacterial strains, Thermosynechococcus elongatus PKUAC-SCTE542 and Synechococcus elongatus PCC7942, resulting in a maximal sucrose yield of 0.14 and 0.07 g/L, respectively. These organisms were co-cultured with E. coli BL21 expressing ethylene-forming enzyme or isoprene synthase and successfully synthesized volatile hydrocarbons. Productivity parameters of these co-cultures were higher than respective transgenic cultures of E. coli grown individually at similar sucrose concentrations, highlighting the positive impact of the artificial consortia on the production of these platform chemicals.
Platform chemicals, defined as essential chemical building blocks serving as precursors to numerous secondary chemicals and materials are essential components of our economy. Ethylene and isoprene are platform chemicals with conjugated double bonds and the primary substrates for the production of many polymers such as synthetic rubber and resins (Easterbrook and Allen, 1987; Deepak et al., 2019). With the advance of industrialization, the demand for basic industrial materials is rising. At present, these two substances are mainly produced from petroleum raw materials, such as natural gas, naphtha, diesel, and heavy oil, which are obtained by petroleum fractionation and petroleum cracking (Valenzuela et al., 2008; Zhang et al., 2020). These methods result in significant carbon dioxide emissions and environmental polluting by-products. Therefore, it is a hot research field to seek a cleaner production method. With biosynthesis technology, the production of these by-products is avoided, and the harm to the environment is reduced. Studies have shown that the two volatile platform hydrocarbons, ethylene, and isoprene, can be produced through biological systems using their corresponding synthases and associated, native biosynthetic pathways. Isoprene in E. coli can be synthesized from dimethylallyl pyrophosphate (DMAPP) precursor generated by a native methylerythritol 4-phosphate (MEP) pathway using heterologously expressed isoprene synthase (IspS; Kuzuyama, 2002). Ethylene can, in principle, be synthesized in E. coli from methionine by NADH: Fe(II)EDTA oxidoreductase or from α-ketoglutaric acid (AKG) by the ethylene-forming enzyme (Efe; Kazuhiro et al., 1991). The first pathway requires ammonia limitation (C/N = 20) conditions, which is not suitable for the growth of E. coli or cyanobacteria; therefore, the pathway utilizing Efe is more optimal (Ishihara et al., 1995). Both precursors, DMAPP and AKG, are readily available in E. coli, making it a suitable cell factory for producing these metabolites.
Inspired by the symbiotic relationship of microbial communities in nature (Beliaev et al., 2014), researchers designed and simulated the symbiosis system with artificial substance exchange pathways (Brenner et al., 2008) and gradually developed synthetic consortia between the same microbial species (such as co-culture of multiple E. coli strains; Zhang et al., 2022), heterotrophic microbial consortia (such as co-culture of E. coli and fungi; Minty et al., 2012), or autotrophic-heterotrophic consortia (such as co-culture of cyanobacteria and heterotrophic Streptomyces or E. coli; Li et al., 2017; Weiss et al., 2017) and used them in pollution abatement and product biosynthesis (Hays et al., 2016). Studies show that this method avoids the frequent issues caused by some microbial contamination and other undesirable factors associated with cultivating a single strain in large volumes (Gavrilescu and Chisti, 2005; Venkata Mohan and Venkateswar Reddy, 2013). In this way, a variety of functional genes are expressed in different strains and work separately to avoid interaction between gene expression, which strengthens the core characteristics of each microbial strain, reducing the metabolic load of each bacterial chassis and is suitable for completing multiple complex tasks at the same time (Song et al., 2014; Ma et al., 2019).
Among the bioproduction-oriented synthetic consortia that have been designed and characterized so far (reviewed by Diender et al., 2021; Mittermeier et al., 2022), the co-cultures involving a model photosynthetic bacterium Synechococcus elongatus are primary examples of CO2-utilizing microbial platforms. Since the foundational work performed a decade ago (Ducat et al., 2012), the concept has been progressively developed. Sucrose-secreting S. elongatus was used in co-culture to support the growth of the several accompanying heterotrophic species (Bacillus subtilis, Saccharomyces cerevisiae, or Escherichia coli; Hays et al., 2016); and used for the production of an industrially relevant bioplastic polyhydroxybutyrate (PHB) in conjunction with Halomonas boliviensis (Weiss et al., 2017). Another polyhydroxyalkanoates production study explored the coupling of sucrose exporting S. elongatus with Pseudomonas putida (Löwe et al., 2017). More recently, the possibility of light-driven 3-hydroxypropionic acid production by the co-culture system composed of S. elongatus and E. coli was demonstrated (Hays et al., 2016). The same pair of organisms with different genetic modifications has been recently used to produce isoprene (Liu et al., 2021). All these studies shared the idea of designing the synthetic co-culture system capable of converting the photosynthetically produced sugar into high-value target molecules of biotechnological importance (Weiss et al., 2017). Furthermore, the stability of light-driven co-cultures can be improved by using hydrogel systems to ensure the space-efficient structure of the consortium (Gao et al., 2022). As the consortia-related methodological toolbox continues to expand (Ma et al., 2022; Singh and Ducat, 2022), the importance of the “phototroph + heterotroph” designs based on the conversion of CO2 to organic chemicals can be expected to grow.
Cyanobacteria are photosynthetic autotrophs with higher carbon fixation efficiency than plants (Wang et al., 2008). With a relatively simple genetic background, easy cultivation, and fast growth rates (Cheali et al., 2016), they are suitable microbial cell factories that avoid competition for land with food cultivation (Quintana et al., 2011). With the discovery of natural transformation (Kondo et al., 1994), electrotransformation (Thiel and Poo, 1989), and conjugation (Thiel and Peter Wolk, 1987) methods of plasmid uptake by the cyanobacterial cells, the existing barriers to importing target genes to cyanobacteria were eliminated, and the research on transgenic cyanobacteria is gradually increased. At present, research on cyanobacterial cell factories has covered many fields, such as the synthesis of high-value products and renewable compounds, carbon or nitrogen fixation, etc. (Choi et al., 2020; Tarawat et al., 2020; Mukherjee et al., 2021; Watanabe and Horiike, 2021). Cyanobacteria can synthesize sucrose, storage, and osmo-protective compound using triose phosphates, ATP, and electron equivalents generated during photosynthesis and native enzymes, including sucrose synthase (SPS; Huber and Huber, 1992). Although sucrose is not as common carbon source for E. coli as glucose, it has been found that about 50% of wild-type E. coli can utilize it (Holt et al., 1994). Under low carbohydrate conditions, the chromosomally coded sucrose catabolism genes—csc, namely cscA, cscB, and cscK are expressed in many E. coli strains K-12, BL21, O157:H7, and EC3132 (Hayashi et al., 2001; Jahreis et al., 2002). The imported sucrose is further broken down to glucose and fructose using CscA and metabolized as carbon and energy sources through glycolysis (Figure 1). Sucrose permease, sucrose: proton transporter encoded by the cscB gene, is a large proton symport system with 12 transmembrane helixes and three overlapping substrate specificities (Bockmann et al., 1992; Figure 1). It belongs to the oligosaccharide/H+ cotransporter subfamily in the Major Facilitator Superfamily (MFS; Marger and Saier, 1993), similar to LacY, and both can catalyze the oligosaccharide/H+ cotransport across the plasma membrane. Substrate specificity of CscB is to the fructofuranosyl ring of sucrose allows for sucrose, fructose, and lactulose, but not glucopyranosyl moiety to be metabolized (Sugihara et al., 2011). This makes sucrose a good intermediate for carbon and energy transfer between different members of an artificial community.
Figure 1. Schematic diagram of the artificial consortium system used in this study. Thermosynechococcus E542 and Synechococcus PCC7942 engineered to secrete sucrose under osmotic stress to support the growth of transgenic Escherichia coli strains capable of the synthesis of ethylene or isoprene. CO2, carbon dioxide; HCO3−, bicarbonate; G3P, glyceraldehyde 3-phosphate; GLC, glucose; FRU, fructose; PYR, pyruvate; UDP-Glc, uridine diphosphate glucose; F6P, fructose 6-phosphate; SUC, sucrose; Ac-CoA, acetyl CoA; CIT, citrate; AKG, alpha ketoglutarate; SUC, succinate; OAA, oxaloacetate; L-Glu, glutamate; L-Arg, L-Arginine; ETH, ethylene; DMAPP, dimethylallyl pyrophosphate; ISP, isoprene; ATP, adenosine triphosphate; ADP, adenosine diphosphate; NADP+, oxidized nicotinamide adenine dinucleotide phosphate; NADPH, reduced nicotinamide adenine dinucleotide phosphate; CscB, sucrose permease; CscA, sucrose hydrolase; IspS, isoprene synthase; and efe, ethylene forming enzyme.
Some microorganisms can synthesize and secrete sucrose as an osmolyte when cultivated in excessive concentrations of salts (Hagemann, 2011). Exploration of this phenomenon in cyanobacteria enables continuous production of sucrose that exceeds the productivity of sugarcane, sugar beet, and other traditional sugar crops (Han and Watson, 1992). On this basis, cyanobacteria can be engineered to express sucrose permease, CscB, to secrete the disaccharide to the growth medium, and support the growth of E. coli in the absence of other carbon sources (Hays et al., 2016). For example, the sucrose yield of Synechocystis sp. PCC 6803 (3.13 mg/L/h) under 600 mM NaCl stress can be achieved by overexpressing genes related to sucrose synthesis and downstream sucrose metabolism (sps, spp, and ugp genes; Du et al., 2013). Meanwhile, E. coli cscB gene expression in Synechococcus elongatus PCC 7942 resulted in a yield of 36.1 mg/L/h (Ducat et al., 2012). Zhang et al. (2020) pointed out that sucrose derived from cyanobacteria may be considered an attractive renewable feedstock to produce chemicals. However, its separation and purification generate considerable costs. These costs can be eliminated by employing the microbial consortium involving a cyanobacterium that provides photosynthetically produced sucrose to E. coli, which in turn serves as a producer of the target chemical.
In this study, artificial consortia consisting of cyanobacteria: Thermosynechococcus elongatus PKUAC-SCTE542 (subsequently, E542), Synechococcus elongatus PCC7942 (subsequently, PCC7942) in conjunction with E. coli BL21 expressing either IspS or Efe were constructed to produce volatile platform hydrocarbons indirectly from CO2. In this system, cyanobacteria overexpressing the E. coli cscB gene under the control of a light-driven promoter produced and exported sucrose into the medium. The second component of the consortium, E. coli engineered with ethylene-forming enzyme gene efe, or isoprene synthetase gene ispS, can use sucrose in the medium and produce volatile platform hydrocarbons.
All the strains used in this study are listed in Table 1. All plasmids (Supplementary Table 1) were created using oligonucleotides summarized in Supplementary Table 2. The polymerase chain reaction was performed using Phanta Max 2x (Vazyme, China) according to the manufacturer’s recommendations. Constructs were assembled using ligase-independent ClonExpress II One Step Cloning Kit (Vazyme, China) using the manufacturer’s guidelines, propagated in E. coli DH5α, and sequence verified by Sanger sequencing. The ethylene-forming enzyme (efe) of Pseudomonas syringae pv. Phaseolicola (AF101058.1) and isoprene synthetase (ispS) from E. globulus (BAF02831) were synthesized commercially at BGI WRITE (Beijing, China). After PCR amplification with their respective primers (Supplementary Table 2), both genes were separately cloned into the bacterial expression plasmid pBAD_LIC_cloning vector (8A) linearized with EcoRV essentially as described earlier (Cui et al., 2022; Cui and Daroch, 2022). The plasmids were propagated under 50 μg/ml ampicillin selection, and both enzymes were expressed under arabinose promoter (araBAD) with 0.2% arabinose.
The self-replicative plasmid expressing cscB gene (pLJD31) was created using a five-way assembly of the following fragments. The backbone and antibiotic resistance gene promoters were amplified from pAM5057 using MDLJCSTR224 and MDLJCSTR225, and MDLJCSTR500 and MDLJCSTR501, respectively. Plasmid pAM5057 was a gift from Susan Golden (Addgene plasmid # 120085; http://n2t.net/addgene:120085; RRID:Addgene_120,085). The kanamycin resistance gene was amplified from pETM11_Saz_CA (Hou et al., 2019) based on pETM11 backbone (EMBL Heidelberg) using MDLJCSTR120 and MDLJCSTR161. Light-driven promoter PsbA* along with the RBSV33 of Synechocystis PCC6803 (Wang et al., 2018) were custom synthesized at BGI WRITE (Beijing, China) and amplified using primer pair MDLJCSTR502 and MDLJCSTR136. The cscB gene expressing sucrose permease was amplified from E. coli DH5α using MDLJCSTR226 and MDLJCSTR227. The resultant plasmid pLJD31 was used for the transformation of E542.
The integrative plasmid expressing cscB gene was created using a three-step procedure. First, the plasmid pLJD50 was constructed based on pAM2991 backbone targeting the Neutral Site I of PCC7942 genome. The backbone was amplified with MDLJCSTR290 and MDLJCSTR291 primer pair. The plasmid pAM2991 was a gift from Susan Golden (Addgene plasmid # 40248; http://n2t.net/addgene:40248; RRID:Addgene_40,248; Ivleva et al., 2005). The antibiotic resistance promoter Pamp, spectinomycin resistance gene, the light-driven promoter of PCC7942, multiple cloning site, RBS, and terminator were amplified from pETS1 plasmid (Liang et al., 2019) using primer pair MDLJCSTR282 and MDLJCSTR228. Second, from pLJD50 vector a kanamycin-containing derivative pLJD51 was generated using a two-way assembly of PCR products amplified with primer pair MDLJCSTR207-MDLJCSTR289, and MDLJCSTR161-MDLJCSTR162. Finally, pLJD51 was linearized with MDLJCSTR319 and MDLJCSTR320 pair and assembled with cscB PCR product amplified with MDLJCSTR381 MDLJCSTR382 pair from the gDNA of E. coli DH5α. The resultant plasmid pLJD32 was used to transform PCC7942.
The modified plasmids with efe (pBAD-efe) or IspS (pBAD-IspS) were transformed to E. coli BL21 to produce ethylene or isoprene. The cscB containing plasmid (pLJD31) was transformed into E542 through the conjugation via E. coli HB101 harboring pRL443 and pRL623 (Thiel and Peter Wolk, 1987) and E. coli DH5α carrying the pLJD31 plasmids, respectively. Meanwhile, the pLJD32 was transformed into PCC7942 using the recently optimized protocol (Riaz et al., 2022).
Two co-culture media, Co-BG11 and M9-BG11, were tested to establish synthetic consortia between E. coli BL21 and E542 and PCC 7942. The Co-BG11 medium was prepared as described earlier (Zhang et al., 2020). In short, the medium was based on a conventional BG-11 medium and modified with the supplementation of 150 mM NaCl, 4 mM NH4Cl, and 3 g/L 2-[[1,3-dihydroxy-2-(hydroxymethyl) propan-2-yl] amino] ethanesulfonic acid (TES) to support the growth of E. coli. In addition, NaCl was also added to induce stress in cyanobacterial strains for sucrose production and secretion. The second co-culture medium, M9-BG11 was designed based on regular BG-11 and M9 minimal media optimal for cyanobacteria and E. coli. The original BG11 medium (Stanier et al., 1971) was supplemented with 6 g/L K2HPO4, 3 g/L KH2PO4, 150 mM NaCl, 1 g/L NH4Cl, 0.12 g/L MgSO4, 0.014 g/L CaCl2, and 0.001 g/L vitamin B1. In the consortia of ethylene, 0.2 g/l FeSO4 was supplemented.
For cloning and precultures, E. coli cells were routinely grown in liquid LB medium in a shaking incubator (HZQ-X300C, Yiheng, Shanghai, China) at 180 rpm or LB agar (solid) plates at 37°C. Further, 50 μg/ml of ampicillin was added to maintain plasmids, and 0.2% arabinose was used to induce the expression of efe and IspS genes.
Cyanobacteria were cultivated in BG11 medium in an illuminated shaking incubator (Jintan Jingda Instruments TS-2112B) under constant illumination with white light at 2000 LUX (~36 μmol m−2 s−1) at 180 rpm at 37°C for PCC7942 and 45°C for E542. In addition, the medium was supplemented with 50 μg/ml of kanamycin to maintain the plasmids and genomic integration.
Before establishing synthetic consortia, cyanobacteria grown in the exponential phase were diluted into one of the two co-culture media (Co-BG11 and M9-BG11) at a cell density of OD750 ≈ 0.15 (corresponding to 9 × 106 cells/ml) and grown at 37°C (PCC7942) or 45°C (E542) for 48 h to reach the cell density of OD730 of 0.3. The E. coli BL21 cells were preincubated in the M9 medium supplemented with antibiotic and 2 g/L sucrose instead of glucose for 12 h. Later, the cells were collected by centrifugation, washed twice with deionized water, and inoculated into the 15 ml cyanobacterial culture described above to the final cell density of OD600 = 0.1–0.2 (corresponding to 1 × 107–2 × 107 cells/ml). After adding arabinose to the concentration of 0.2%, the co-culture was incubated at 30°C for 48 h to produce ethylene or isoprene.
For pure cultures of cyanobacteria and E. coli, cell density was measured at OD730 and OD600 with an EPOCH microplate reader (BIOTEK, United States). For the co-culture, the cell number of E. coli was calculated by counting colony-forming units (CFU) in LB plate after 14 h of incubation at 37°C, and the cell number of cyanobacteria was counted by Countstar-IC1000 Cell Analyzer (Ruiyu, China).
Approximately 1.5–2 ml E. coli culture at OD600 = 0.4–0.6 was collected by centrifugation at 4°C, 8,000 rpm for 3 min to isolate RNA with Total RNA kit I (Omega Bio-Tec, United States) to compare the mRNA levels of sucrose catabolism genes (cscB, cscA, and cscK) and efe or IspS gene in M9 media supplemented with 2 g/L sucrose or conventional LB media. The reference gene for E. coli RT-qPCR is 16S rRNA. In the case of cyanobacteria, approximately 50–100 ml cultured cells were centrifugated at 4°C 8,000 rpm for 15 min to isolate total RNA to verify the mRNA levels of cscB gene in engineered strains. The reference gene for E542 is an “analysis fragment” from the literature at positions “2,985–3,144” of CP032152, and purl was used for PCC7942 (Riaz et al., 2021). The total RNA of E. coli was isolated with Total RNA kit I (Omega Bio-Tech, United States), while the total RNA of cyanobacterial was isolated with Trizol Reagent (Invitrogen, United States; Chomczynski and Sacchi, 2006). PrimeScript™ RT reagent Kit with gDNA Eraser (Takara, Japan) was used to remove the gDNA and reverse transcribe RNA to cDNA. The RT-qPCR reaction mix was prepared with TB Green Premix Ex Taq II (TaKaRa) in a QuantStudio 5 real-time system (ABI, United States). All the primers used for RT-qPCR analysis are given in Supplementary Table 2.
The ethylene production in the gas phase was performed in a 60 ml sealed bottle headspace with 15 ml cyanobacterial-E. coli co-culture essentially as described before (Cui et al., 2022). In short, 1 ml air sample was injected into Agilent Technology 6850 GC FID with Porapak Q 3 M × 1/8 column to measure the concentration of ethylene. Three biological replicates were prepared to measure ethylene production, while one was maintained to determine the cellular density (CFU or OD). Ethylene production was defined as the amount (1 μmol) of ethylene produced by 1011 cells. The ethylene production per day was calculated as follows:
C: concentration of ethylene in the bottle, measured by GC (ppm); Va: gas volume in the bottle (mL); N: cell number; and T: reaction time (day).
Isoprene production in the gas phase was analyzed in a headspace of a 60 ml sealed bottle with 15 ml co-cultured cells, as described before (Cui and Daroch, 2022). Briefly, toluene was injected into the bottle to absorb isoprene and incubated overnight at 4°C to condense the isoprene. The Agilent Technology 6850 N-5975 GC–MS with HP-5MS 5% Phenyl Methyl Siloxa column was used to measure the isoprene concentration. Different gradient volumes of liquid isoprene were added to the bottle and detected with GC–MS to make the standard curve. Three replicas for each strain were prepared to measure isoprene, while one replica was used for the cell density measurement (CFU or OD). The efficiency of isoprene production was calculated as follows:
W: the content of isoprene in the bottle (μg); N: cell number; and T: reaction time (day).
The 20 ml cyanobacterial culture supernatants grown under NaCl stress were collected and concentrated ten times to 2 ml at 65°C. The concentrated sucrose solution was hydrolyzed with 2 ml 6 mol/l HCl for 10 min at 100°C, then pH was adjusted to 9, and ddH2O was added to the final volume of 10 ml. Subsequently, 0.8 ml of this liquid was mixed with 0.6 ml DNS detection reagent (Casmart, China) and boiled for 5 min at 100°C. The sucrose concentration was determined at OD520 against the standard curve prepared for known concentrations of sucrose treated under the same conditions (Miller, 1959).
E542 and PCC7942 were engineered to secrete sucrose by expressing the sucrose permease encoding gene cscB under the light-driven promoters (Supplementary Figure 1) and the kanamycin resistance gene. The plasmids were transferred into the cyanobacteria E542 and PCC7942 using conjugation and natural transformation, respectively. The insertion of cscB gene and sucrose secretion did not significantly affect the growth of cyanobacteria under their optimal growth conditions (Supplementary Figure 2). As is shown in RT-qPCR results (Table 2), the cscB gene was successfully transcribed in engineered strains. The mRNA expression levels of the cells grown under constant illumination were 8.01 and 7.22 fold higher than the reference for PCC7942 and E542, respectively.
The cells of E542_cscB+ and PCC7942_ cscB+ were cultured from an initial OD730 = 0.1–0.13 at 45°C (E542) or 37°C (PCC7942). Their growth and sucrose secretion was monitored for 3.5 and 7.5 days, as shown in Figure 2. Compared with the wild-type strains (WT), the sucrose yield of the engineered strains increased when cultivated under the same culture conditions. Compared with the yield in the dark environment and in the culture environment without NaCl, the sucrose yield of the engineered strains significantly increased under 150 mM NaCl with light. The sucrose yield in E542_cscB+ reached 0.073 g/L (0.234 g/L/OD730) and 0.136 g/L (0.221 g/L/OD730) after 3.5 and 7.5 days, respectively, and the secretion efficiency remained at about 0.02 g/L/day. However, sucrose yield in PCC7942_cscB+ was 0.054 g/L (0.226 g/L/OD730) and 0.075 g/L (0.166 g/L/OD730) after 3.5 and 7.5 days, respectively. The yield decreased slightly under long-term culture, and the maximum secretion efficiency was about 0.015 g/L/day. The secretion efficiency is lower than the recent study with Synechococcus elongatus UTEX 2973 cultured in BG11 + 150 mM NaCl (~0.1 g/L/day, ~0.59 g/L/OD730 for 3 days; Zhang et al., 2020) and the other studies with PCC7942 and lac promoter in other media (0.05–0.3 g/L/day for 2–4 days, total production is 0.156–0.625 g/L, 0.07–0.25 g/L/OD730; Hays et al., 2016; Li et al., 2017). The carbon allocation ratio analysis shows an interesting difference between both transgenic cyanobacteria. Examination of the PCC7942_ cscB+ indicates that sucrose secretion at dark remains relatively stable, which, combined with low biomass production, yields relatively high carbon partitioning to sucrose (Figures 2A–C). A different effect is observed in E542_cscB+ where the dry weight upon transfer to dark conditions remains stable and sucrose secretion almost halts, resulting in very low sucrose to biomass ratio. Conversely, prolonged incubation at saline illuminated conditions results in low biomass accumulation and maximal sucrose productivity and carbon partitioning to sucrose significantly exceeding 1, indicating the majority of the flux being directed toward sucrose secretion. Similar results have been obtained before (Ducat et al., 2012), indicating that a high stress level is required to maximize sucrose production.
Figure 2. The comparison of sucrose yield of the PCC7942_cscB+ (A), E542_cscB+ (D), accumulated dry weight (B,E), and sucrose to biomass ratio (C,F) grown under different light and osmotic pressure in BG11 growth medium. Height of the bars shows the mean of three independent experiments with error bars representing SD from measurements.
Both the strain and the constructs may influence the sucrose production of the transgenic cells and the sucrose secretion efficiency. Besides, cell density may be a factor affecting sucrose secretion yield. If the sucrose secretion efficiency of single cells is the same, a lower growth rate and cell density may lead to a lower sucrose yield per liter cell culture.
The two engineered strains were cultured at different temperatures (30, 37, and 45°C), and samples were taken at 3.5 and 7.5 days to measure and compare the sucrose content in the culture medium and the growth of the strains (Figure 3). At 30°C, E542_cscB+ grows slowly (Figure 3B), and the sucrose yield (0.053 g/L for 3.5 days; 0.040 g/L for 7.5 days; Figure 3A) was also lower, while the sucrose yield of PCC7942_cscB+ (0.057 g/L for 3.5 days; 0.085 g/L for 7.5 days) was better at this temperature. At 37°C, both strains could grow well, and the 0.094 g/L sucrose yield of E542_cscB+ for 7.5 days was better than 0.067 g/L of PCC7942_cscB+. At 45°C, the E542 grew well, and the sucrose yield was the highest (0.136 g/L for 7.5 days), while PCC7942 died gradually due to poor tolerance to high temperature. Consequently, no sucrose was secreted by PCC7942_cscB+ at 7.5 days. The changes in sucrose yield were consistent with the changes in cell density during this process. Sucrose yield was higher when cells grew well and lowered at lower growth rates. Therefore, the temperature significantly affected the sucrose secretion by the engineered strains by impacting their sucrose biosynthesis, growth, or both. Since sucrose biosynthesis in cyanobacteria strains was not artificially regulated in this experiment, the biosynthesis mainly came from the overflow of the photosynthate produced, and the efficiency may be biased toward strains’ respective optimal growth temperatures (Warr et al., 1985). Longer cultivation of the co-culture exceeding 10 days resulted in the cell growth approaching a plateau, probably due to decreased cell viability and saturation of sucrose secretion capacity of the community.
Figure 3. The comparison of sucrose yield (A), and growth curve (B), of engineered E542_cscB+ and 7,942_cscB+ strains at different temperatures grown in BG-11 medium under salt stress. Distribution of the data points shows the mean of three independent experiments with error bars representing SD from measurements.
To ensure the suitability of selected co-culture growth media for the cultivation of engineered E. coli strains and sucrose uptake; the two transgenic strains were cultured in derivatives of sucrose-containing media to choose the optimal composition for co-culture studies. Previous studies showed that E. coli ATCC#9637 ΔcscR strain required about 1.2 g/L sucrose to grow well (that varied depending on other elements of the growth medium; Hays et al., 2016), while the sucrose yield achieved by the transgenic cyanobacteria typically does not reach this level. Therefore, in the preliminary experiment designed to determine the initial cultivation conditions, the co-culture environment was simulated by adding sucrose to the final concentration of 0.2 and 2 g/L in both of the co-culture media, i.e., co-BG11 and M9-BG11. The experiment was performed at 30 and 37°C (Supplementary Figure 3). Results showed the good growth of engineered E. coli BL21_Efe_PS at 37°C when 2 g/L sucrose was added to each of the co-culture media, while the growth at 30°C was much poorer, with the final cell density of OD600 = 0.4, markedly lower than other conditions tested (Supplementary Figure 3). This may be due to the high energy consumption of the cells at low temperature, and sucrose being gradually consumed during 96 h of cultivation and insufficient to provide sufficient carbon and energy for the rapid proliferation of E. coli. The lower concentration (0.2 g/L) of sucrose in the medium significantly inhibited the growth of E. coli.
The expression of sucrose metabolism-related genes (cscA, cscB, and cscK) and the volatile platform hydrocarbon synthase genes (efe and IspS) in engineered E. coli strains cultured with sucrose as the sole carbon source was studied. After BL21_efe_PS+ and BL21_IspS_EG+ strains were cultured to OD600 = 0.4–0.6 in LB and M9 medium (glucose in M9 medium was replaced with 2 g/L sucrose), 0.2% arabinose was added to induce the expression. After induction at 30°C for 5 h, total RNA was extracted and detected. mRNA levels of the two strains in the M9 medium containing sucrose were compared with those in the LB medium (Figure 4). As shown in Figure 4, the RNA transcription levels of sucrose hydrolase gene cscA and fructokinase gene cscK increased significantly in the BL21_efe+ and BL21_IspS+, while the increase for sucrose permease gene cscB was not significant, indicating sufficient sucrose flux. In the two strains, the transcription of all csc genes in BL21_IspS_EG+ was significantly lower than that of BL21_efe_PS+. This may be related to the different synthesis pathways of their precursors in E. coli (Figure 1). In E. coli, the main precursors of ethylene synthesis are α-ketoglutaric acid and arginine. Arginine is an amino acid, which can be obtained directly from tryptone in LB medium, while α-ketoglutaric acid is synthesized by the TCA cycle (Li et al., 2006; Xiao et al., 2016). Acetyl-CoA, an essential node in the TCA cycle, can be derived from glycolysis and the decomposition of fatty acids and amino acids; meanwhile, it is also a building block for synthesizing these compounds (Han et al., 2001; Luo et al., 2014). In LB medium, raw materials such as amino acids are abundant, but the carbohydrate content is low, so presumably lower portion of acetyl-CoA is synthesized from glycolysis. However, in an M9 medium supplemented with sucrose, amino acids and other building blocks are likely to be limiting. Presumably, the resultant metabolism depends primarily on glycolysis to synthesize acetyl-CoA and, ultimately α-ketoglutaric acid, amino acids, and other essential substances to keep the cells alive. Therefore, the transcription and expression of related genes (csc genes) were likely to be increased under the increased metabolic requirements of glycolysis. However, since the synthesis of α -ketoglutaric acid was lower, the supply of arginine was limited; consequently, the ethylene synthesis could also be limited by the availability of precursors. On the contrary, the precursors of isoprene synthesis in this E. coli strain are pyruvate and glyceraldehyde-3-phosphate, both directly linked to glycolysis, and csc series genes may have been expressed in LB medium. Compared with the LB medium, precursors can be directly synthesized from sucrose available in the M9 medium, and the increase in precursor supply was conducive to the synthesis of isoprene and promoted the transcription of related genes.
Figure 4. Changes of gene expression of BL21_efe-PS and BL21_IspS_EG+ in the cultures with sucrose as the sole carbon source calculated using the relevant ratio of the mRNA value in the M9 medium to the mRNA value in the LB with 2−ΔΔCT method (Livak and Schmittgen, 2001).
The addition of arabinose and extra ions to the co-culture media may affect the growth of cyanobacteria. E542_cscB+ and PCC7942_cscB+ were cultured in BG11 and the two co-culture media to explore these effects. Cyanobacteria can grow normally in both co-culture media, but the growth in the co-BG11 medium was somewhat slower (Supplementary Figure 4). Meanwhile, the addition of 0.2% arabinose had no significant effect on the growth of the strains. The sucrose yield in different media was tested to explore the sucrose secretion capacity of engineered strains in the co-culture system (Figure 5). The culture was started with OD730 = 0.15 and the sucrose yield of the strains cultured at their optimal growth temperatures for 2 days was detected. As shown in Figure 5, the sucrose yield of E542_cscB+ was higher than that of PCC7942_cscB+ in all media; this was consistent with the prior results of measurement for sucrose secretion by other transgenic cyanobacteria. Both the OD730 and sucrose yield in the M9-BG11 medium was higher than that of the co-BG11 medium (Figure 5; Supplementary Figure 4), indicating its more optimal composition for this task.
Figure 5. The comparison of sucrose yield of the engineered strains of E542_cscB+ and PCC7942_cscB+ in different co-culture media during 48-h long cultivation. Height of the bars shows the mean of three independent experiments with error bars representing SD from measurements.
Based on the literature and previous studies (Cui et al., 2022; Cui and Daroch, 2022), ethylene synthase and isoprene synthase were expressed well when the induced temperature was lower than the growth temperature of E. coli (37°C), and both proteins were prone to inclusion body formation at high temperature. In analyzing sucrose secretion by cyanobacteria above, engineered PCC7942 performed better at 30 and 37°C. Meanwhile, E542 secreted little sucrose at 30°C due to the poor growth parameters of the strain at this temperature. To compensate for those drawbacks, sucrose secretion during co-culture could be enhanced by increasing initial cell density. Based on the productivity data per cell, these lower growth rates may have better channeled the available cellular resources for sucrose production. Therefore, 30°C was selected as the reaction condition for co-cultures. Before that, cells were cultured separately to maintain good initial activity and density.
The artificial consortium systems of engineered strains of cyanobacteria E542_cscB+ and PCC7942_cscB+ with engineered strains of E. coli BL21_efe_PS+ were established at co-BG11 medium and M9-BG11 media. After culturing for 48 h at 30°C, ethylene was detected by the GC. At the same time, the number of E. coli cells in the co-culture system was calculated by the CFU. The results of ethylene productivity are shown in Table 3. Escherichia coli BL21_efe_PS+ was cultured in the co-culture medium supplemented with 2 g/L sucrose without cyanobacteria as the control group.
During the co-culture process, E. coli cell density was stable at the level of 1.5 × 107–2 × 107/ml and cyanobacteria grew normally in the system. The ratio of cyanobacteria and E coli cells was from 8.3 (15 × 107 cyanobacteria per milliliter/1.8 × 107 E.coli per milliliter, at the beginning) to 10.6 (20 × 107 vs. 1.89 × 107, after 1 day) then 12.5 (25 × 107 vs. 2.0 × 107, after 2 days) in consortium with M9-BG11. While the ratio was from 8.2 (15 × 107 cyanobacteria per milliliter/1.83 × 107 E.coli per milliliter, at the beginning) to 10.2 (19 × 107 vs. 1.86 × 107, after 1 day), and then 11.5 (22 × 107 vs. 1.92 × 107, after 2 days) in the consortium with Co-BG11. No other species was observed under microscopy.
The ethylene production was observed in all consortia, but the ethylene production was lower than that of the individual E. coli strain grown in LB (794.07 μmol/L for 5 h), or in the two co-culture media with 2 g/L sucrose (70.93 and 89.16 μmol/L for 48 h). In the studies that utilized E. coli as a single producer, the ethylene production level was 6–500 μmol/L/h in the strains with different vectors (Digiacomo et al., 2014; Eckert et al., 2014). In PCC7942, the productivity of ~20 μmol/L/h was recorded with psbAI promoter (Takahama et al., 2003) and ~ 6.25 μmol/L/h was recorded with trc promoter (Carbonell et al., 2019), while in the engineered strain of Synechocystis PCC 6803 the levels of 12–42 μmol/L/h were observed (Lee et al., 2015; Veetil et al., 2017; Wang et al., 2018). The comparison shows that the E542 strain and M9-BG11 medium combination had the highest ethylene yield, 52.71 μmol/L culture for 2 days, among the conditions tested. Combined with sucrose secretion and the growth of E. coli, inadequate nutrition leading to low cell density and low metabolic efficiency may be the reason for sub-par productivity. It is worth mentioning here that the approach of submerged cultivation is not the only one reported for ethylene bioproduction. A system based on cyanobacterial biofilms was designed to achieve a 2.2-fold increase in the ethylene production yield compared to the cell suspension (Vajravel et al., 2020).
The consortium systems of cyanobacteria and E. coli for isoprene production were constructed in the same media and conditions as for ethylene production. Isoprene concentration was detected by GC after culturing for 48 h. At the same time, the number of E. coli cells in the co-culture system was calculated by CFU. The results of isoprene productivity are shown in Table 3. In three instances, the isoprene productivity of the consortium was lower than the sensitivity of the assay, i.e., 0.2 μmol/L. The control group of E. coli BL21_IspS_EG+ was cultured in the co-culture medium supplemented with 2 g/L sucrose without cyanobacteria.
The change in cell ratio was similar in the ethylene co-culture system. The comparison shows that the PCC7942 strain and M9-BG11 medium combination had the highest isoprene yield, 0.75 μmol/L culture for 48 h, among the conditions tested. Compared with the production per liter of individual BL21_IspS_EG+ cell culture under carbon saturation, the isoprene production per liter of cell culture in each co-culture system also decreased, but not significantly. Especially, isoprene production per cell was especially stable, suggesting that the decrease resulted from lower sucrose availability. The isoprene production of individual strains expressing IspS gene in the other studies was 0.4–1.25 mg/L E. coli for 18 h in M9 media (Zurbriggen et al., 2012) and 3–7 mg/L S. elongatus PCC 7942 for 72 h in BG-11 containing 100 mM NaHCO3 (Gao et al., 2016). Notably, it was demonstrated that the S. elongatus + E. coli co-cultivation system could provide isoprene titers as high as 0.4 g/L (Liu et al., 2021). The promoter, source of the synthase, and host organism may be reasons for the yield difference. It is also worth optimizing the ratio of the cyanobacteria and E. coli for more stable production and operates on higher cellular densities to improve the production per liter of cell culture (Zhao et al., 2011). Designing an effective inoculation approach may lead to considerable enhancement in terms of isoprene titers; however, it also affects the time required to reach satisfactory product levels in S. elongatus + E. coli co-cultures (Liu et al., 2021).
When the individual strain BL21_efe_PS+ and BL21_IspS_EG+ was cultured in co-culture media with 0.05 g/L sucrose (the sucrose yield of cyanobacteria in 2 days), the production of volatile platform hydrocarbons was lower than that of the consortium. Therefore, there are two possible explanations for these results. First, the gradual sucrose secretion during the co-culturing improved the yield of isoprene, and the consumption of sucrose by E. coli may allow the cyanobacteria to secrete more sucrose. Alternatively, cyanobacteria natively produce and secrete other than carbohydrates metabolites that can be metabolized by E. coli. Moreover, the higher availability of oxygen thanks to the oxygen evolution reaction can also have a positive impact on the co-culture system when compared with the sucrose supplementation study.
When the individual E. coli strain was cultured in consortium media with sufficient sucrose (2 g/L), the production of individual strain BL21_efe_PS+ in co-culture media supplemented with 2 g/L sucrose was 73.93 μmol/L (Co-BG11) and 89.16 μmol/L (M9-BG11), and the production of individual strain BL21_IspS_EG+ in co-culture mediums supplemented with 2 g/L sucrose is 0.38 μmol/L (Co-BG11) and 0.67 μmol/L (M9-BG11). In other words, E. coli can produce 37–45 μmol ethylene or 0.19–0.34 μmol isoprene with 1 g of sucrose, which was also used as the energy and carbon source. Cells grew better with sufficient sucrose (the final cell density is OD600 = 0.6 with 2 g/L sucrose while cell density is OD600 = 0.2 in the consortium system), and the production of ethylene and isoprene per liter culture was higher than that of the consortium system, but the production per cell had little difference. On the one hand, sucrose secreted by cyanobacteria in the co-culture system may be mainly used to produce volatile platform hydrocarbons with a high utilization rate; on the other hand, E. coli cells hardly grew with limited sucrose resulting in lower total production per liter culture.
Sucrose yield in this study was 0.14 g/L (E542_cscB+) and 0.07 g/L (PCC7942_cscB+; Figure 2). This yield is lower than in previous studies (Hays et al., 2016; Li et al., 2017; Zhang et al., 2020), and it may be influenced by the strain, constructs, and, importantly, the lack of external inorganic carbon supply in the form of highly concentrated CO2 or bicarbonate. Consequently, the growth parameters under salt stress in this study are inferior to many of those presented earlier. For example, it took 10 more days to grow from OD730 = 0.05 to OD730 = 0.8 (Supplementary Figure 2) under a light intensity of ~36 μEm−2 s−1 in BG11 with 150 mM NaCl and no CO2 supplementation than it did in other studies. For instance, PCC7942 cells incubated at 35°C, 2% CO2, 150 rpm, ~65 μEm−2 s−1 light intensity, grew to OD750 = 1 in 7 days in BG11 containing 150 mM NaCl (Ducat et al., 2012). Elsewhere, cell density of OD750 = 3 was achieved in 3 days in Co-BG11 (106 mM NaCl and other ions), 35°C, 2% CO2, 150 rpm, and initial cell density OD750 = 0.25 (Hays et al., 2016). All of these indicate that light intensity and CO2 supplementation are crucial for faster growth and are significant factors to improve the sucrose secretion efficiency and to provide more sucrose to E. coli in the consortium system.
Two kinds of co-culture media were compared for the cell growth, gene expression, and production of volatile hydrocarbon. The results showed that when sucrose was served as the only carbon source, sucrose utilization-related genes (csc series genes) showed an obvious upward transcription to provide energy for cell growth, and the volatile platform hydrocarbons could be successfully expressed (Figure 4, Supplementary Figure 3). The transgenic cyanobacterial cells exhibited satisfactory growth and sucrose secretion in both growth media, and its performance in M9-BG11 medium was superior to that in Co-BG11 (Figure 5, Supplementary Figure 4). In the consortium system, both ethylene and isoprene were synthesized, and the maximum yields were 52.71 μmol/l (ethylene production) and 0.75 μmol/l (isoprene production), respectively (Tables 2, 3).
Compared to the productivity in the LB medium, ethylene and isoprene yield per liter of cell culture for individual E. coli strain, decreased in the co-culture medium supplemented with 2 g/L sucrose. It may be caused by the inhibition of cell growth and metabolism due to incomplete sucrose utilization. In many studies utilizing sucrose as a shuttle molecule in the consortium, csc series genes were artificially regulated (overexpressed cscA, cscB, and cscK, deleted gene for sucrose catabolism operon repressor cscR etc.) to improve sucrose utilization in E. coli (Weiss et al., 2017; Zhang et al., 2020). It may be worth exploring similar strategies in the future to improve the performance of consortia in the production of volatile hydrocarbons. Under the conditions tested in this study, the consortium producing isoprene showed better yields than that for ethylene production. The result was consistent with the results of RT-qPCR describing gene transcription under sucrose utilization (IspS gene mRNA level increased, while efe gene mRNA level decreased) caused by different synthesis pathways for the two platform hydrocarbons in E. coli (Figure 1). Arginine and α-ketoglutaric acid are the precursors of ethylene synthesis. In LB medium, arginine can be obtained directly from tryptone present in the medium. Meanwhile, sufficient protein and other energy sources can produce enough acetyl-CoA to drive the synthesis of α-ketoglutaric acid. However, in the co-culture medium, sucrose was used as the sole carbon source, which made acetyl-CoA synthesis dependent on glycolysis and simultaneously exploited for amino acid synthesis and other important metabolic pathways, resulting in a decrease of flux to α-ketoglutaric acid. Lower precursor availability resulted in a significant decrease in ethylene synthesis. In contrast, isoprene precursors in E. coli BL21 are synthesized via the MEP pathway from pyruvate and glyceraldehyde-3-phosphate, mainly derived from glycolysis. Compared with the LB medium, the co-culture system directly provided sucrose to E. coli., which was beneficial to the synthesis of isoprene, but not ethylene.
In this study, artificial consortia comprising E. coli and cyanobacteria, using sucrose as carbon and electron shuttle, for the production of volatile hydrocarbons were successfully constructed. The cscB gene was successfully expressed in both cyanobacterial strains. The results showed that the sucrose yield of the strains was 0.14 g/L (E542_cscB+) and 0.07 g/L (PCC7942_cscB+) under their respective growth temperatures, similar to other studies that did not utilize carbon dioxide supplementation but lower compared to the studies that applied high light and external CO2 supplementation. This suggests that sucrose production by cyanobacteria expressing the sucrose transporter is typically carbon limited and an increased flux toward the secreted carbon can be generated using higher inorganic carbon loads and fine-tuning electron supply from the photosystem for carbon fixation and biosynthesis of sucrose. The growth parameters and gene expression of both engineered members of the artificial community were compared and analyzed, suggesting different metabolic responses of the community members depending on the type of product being synthesized. Various combinations of the consortia cultivation parameters were tested and the maximal yields of hydrocarbon production were achieved using 52.71 μmol/L for ethylene in M9-BG11 with E542 and 0.75 μmol/L for isoprene in M9-BG11 with PCC7942. The productivity of co-culture systems was similar to or superior to pure cultures containing an equivalent carbon source, showing the consortium partners’ positive effect on producing these platform chemicals. However, from the applicative point of view, there is still a significant productivity gap that will allow these systems to replace fossil-derived volatile platform chemicals. The areas that could further improve the productivity of such a system include optimization of carbon and light supply, enhanced cellular concentrations, and in the longer scheme formation of hierarchical structures in which different community members could be arranged to facilitate the transfer of carbon between community members.
The original contributions presented in the study are included in the article/Supplementary material, further inquiries can be directed to the corresponding author.
YC: conceptualization, methodology, validation, formal analysis, investigation, data curation, writing—original draft, writing—review and editing, and visualization. FR: methodology, validation, investigation, data curation, writing—review and editing, and supervision. YJ and YZ: investigation and data curation. SZ: supervision and project administration. TB: resources, validation, formal analysis, writing—review and editing, and visualization. SR: methodology and supervision. MD: conceptualization, methodology, resources, validation, formal analysis, investigation, data curation, writing—original draft, writing—review and editing, visualization, supervision, project administration, and funding acquisition. All authors contributed to the article and approved the submitted version.
This research was funded by Shenzhen Fundamental Research Program (GXWD20201231165807007-20200806170221001).
The authors declare that the research was conducted in the absence of any commercial or financial relationships that could be construed as a potential conflict of interest.
All claims expressed in this article are solely those of the authors and do not necessarily represent those of their affiliated organizations, or those of the publisher, the editors and the reviewers. Any product that may be evaluated in this article, or claim that may be made by its manufacturer, is not guaranteed or endorsed by the publisher.
The Supplementary material for this article can be found online at: https://www.frontiersin.org/articles/10.3389/fmicb.2022.965968/full#supplementary-material
Beliaev, A. S., Romine, M. F., Serres, M., Bernstein, H. C., Linggi, B. E., Markillie, L. M., et al. (2014). Inference of interactions in cyanobacterial-heterotrophic co-cultures via transcriptome sequencing. ISME J. 8, 2243–2255. doi: 10.1038/ismej.2014.69
Bockmann, J., Heuel, H., and Lengeler, J. W. (1992). Characterization of a chromosomally encoded, non-PTS metabolic pathway for sucrose utilization in Escherichia coli EC3132. Mol. Gen. Genet. MGG 235, 22–32. doi: 10.1007/BF00286177
Brenner, K., You, L., and Arnold, F. H. (2008). Engineering microbial consortia: a new frontier in synthetic biology. Trends Biotechnol. 26, 483–489. doi: 10.1016/j.tibtech.2008.05.004
Carbonell, V., Vuorio, E., Aro, E.-M., and Kallio, P. (2019). Enhanced stable production of ethylene in photosynthetic cyanobacterium Synechococcus elongatus PCC 7942. World J. Microbiol. Biotechnol. 35:77. doi: 10.1007/s11274-019-2652-7
Cheali, P., Posada, J. A., Gernaey, K. V., and Sin, G. (2016). Economic risk analysis and critical comparison of optimal biorefinery concepts. Biofuels Bioprod. Biorefin. 10, 435–445. doi: 10.1002/bbb.1654
Choi, Y.-N., Lee, J., Kim, J., and Park, J. (2020). Acetyl-CoA-derived biofuel and biochemical production in cyanobacteria: a mini review. J. Appl. Phycol. 32, 1643–1653. doi: 10.1007/s10811-020-02128-x
Chomczynski, P., and Sacchi, N. (2006). The single-step method of RNA isolation by acid guanidinium thiocyanate–phenol–chloroform extraction: twenty-something years on. Nat. Protoc. 1, 581–585. doi: 10.1038/nprot.2006.83
Cui, Y., and Daroch, M. (2022). “Clean synthesis of isoprene with six E. coli engineering strains” in The 2022 International Conference on Energy, Resources, Environment and Chemical Engineering. (China), 2, 322, 329.
Cui, Y., Jiang, Y., Xiao, M., Munir, M. Z., Riaz, S., Rasul, F., et al. (2022). Discovery of five new ethylene-forming enzymes for clean production of ethylene in E. coli. Int. J. Mol. Sci. 23:4500. doi: 10.3390/ijms23094500
Deepak, V. D., Mahmud, I., and Gauthier, M. (2019). Synthesis of carboxylated derivatives of poly(isobutylene-co-isoprene) by azide–alkyne “click” chemistry. Polym. J. 51, 327–335. doi: 10.1038/s41428-018-0130-y
Diender, M., Parera Olm, I., and Sousa, D. Z. (2021). Synthetic co-cultures: novel avenues for bio-based processes. Curr. Opin. Biotechnol. 67, 72–79. doi: 10.1016/j.copbio.2021.01.006
Digiacomo, F., Girelli, G., Aor, B., Marchioretti, C., Pedrotti, M., Perli, T., et al. (2014). Ethylene-producing bacteria that ripen fruit. ACS Synth. Biol. 3, 935–938. doi: 10.1021/sb5000077
Du, W., Liang, F., Duan, Y., Tan, X., and Lu, X. (2013). Exploring the photosynthetic production capacity of sucrose by cyanobacteria. Metab. Eng. 19, 17–25. doi: 10.1016/j.ymben.2013.05.001
Ducat, D. C., Avelar-Rivas, J. A., Way, J. C., and Silver, P. A. (2012). Rerouting carbon flux to enhance photosynthetic productivity. Appl. Environ. Microbiol. 78, 2660–2668. doi: 10.1128/aem.07901-11
Easterbrook, E. K., and Allen, R. D. (1987). “Ethylene-Propylene Rubber,” in Rubber Technology. ed. M. Morton (Dordrecht: Springer), 260–283.
Eckert, C., Xu, W., Xiong, W., Lynch, S., Ungerer, J., Tao, L., et al. (2014). Ethylene-forming enzyme and bioethylene production. Biotechnol. Biofuels 7:33. doi: 10.1186/1754-6834-7-33
Gao, X., Gao, F., Liu, D., Hao, Z., Nie, X., and Yang, C. (2016). Engineering the methylerythritol phosphate pathway in cyanobacteria for photosynthetic isoprene production from CO2. Energy Environ. Sci. 9, 1400–1411. doi: 10.1039/C5EE03102H
Gao, H., Manishimwe, C., Yang, L., Wang, H., Jiang, Y., Jiang, W., et al. (2022). Applications of synthetic light-driven microbial consortia for biochemicals production. Bioresour. Technol. 351:126954. doi: 10.1016/j.biortech.2022.126954
Gavrilescu, M., and Chisti, Y. (2005). Biotechnology—a sustainable alternative for chemical industry. Biotechnol. Adv. 23, 471–499. doi: 10.1016/j.biotechadv.2005.03.004
Hagemann, M. (2011). Molecular biology of cyanobacterial salt acclimation. FEMS Microbiol. Rev. 35, 87–123. doi: 10.1111/j.1574-6976.2010.00234.x
Han, Y. W., and Watson, M. A. (1992). Production of microbial Levan from sucrose, sugarcane juice and beet molasses. J. Ind. Microbiol. 9, 257–260. doi: 10.1007/BF01569633
Han, M. J., Yoon, S. S., and Lee, S. Y. (2001). Proteome analysis of metabolically engineered Escherichia coli producing poly(3-hydroxybutyrate). J. Bacteriol. 183, 301–308. doi: 10.1128/jb.183.1.301-308.2001
Hayashi, T., Makino, K., Ohnishi, M., Kurokawa, K., Ishii, K., Yokoyama, K., et al. (2001). Complete genome sequence of enterohemorrhagic Escherichia coli O157:H7 and genomic comparison with a laboratory strain K-12. DNA Res. 8, 11–22. doi: 10.1093/dnares/8.1.11
Hays, S., Yan, L., Silver, P., and Ducat, D. (2016). Synthetic photosynthetic consortia define interactions leading to robustness and photoproduction. J. Biol. Eng. 11:4. doi: 10.1186/s13036-017-0048-5
Holt, J. H., Krieg, N. R., Sneath, P. H. A., Staley, J. T., and Williams, S. T. (1994). Bergey’s Manual of Determinative Bacteriology. 9th Edn. Baltimore: Williams and Wilikins, 786–788.
Hou, J., Xingkang, L., Kaczmarek, M., Chen, P., Li, K., Jin, P., et al. (2019). Accelerated CO2 hydration with thermostable Sulfurihydrogenibium azorense carbonic anhydrase-chitin binding domain fusion protein immobilised on chitin support. Int. J. Mol. Sci. 20:1494. doi: 10.3390/ijms20061494
Huber, S. C., and Huber, J. L. (1992). Role of sucrose-phosphate synthase in sucrose metabolism in leaves. Plant Physiol. 99, 1275–1278. doi: 10.1104/pp.99.4.1275
Ishihara, K., Matsuoka, M., Inoue, Y., Tanase, S., Ogawa, T., and Fukuda, H. (1995). Overexpression and in vitro reconstitution of the ethylene-forming enzyme from pseudomonas syringae. J. Ferment. Bioeng. 79, 205–211. doi: 10.1016/0922-338X(95)90604-X
Ivleva, N. B., Bramlett, M. R., Lindahl, P. A., and Golden, S. S. (2005). LdpA: a component of the circadian clock senses redox state of the cell. EMBO J. 24, 1202–1210. doi: 10.1038/sj.emboj.7600606
Jahreis, K., Bentler, L., Bockmann, J., Hans, S., Meyer, A., Siepelmeyer, J., et al. (2002). Adaptation of sucrose metabolism in the Escherichia coli wild-type strain EC3132. J. Bacteriol. 184, 5307–5316. doi: 10.1128/JB.184.19.5307-5316.2002
Kazuhiro, N., Ogawa, T., Fujii, T., Tazaki, M., Tanase, S., Morino, Y., et al. (1991). Purification and properties of an ethylene-forming enzyme from pseudomonas syringae pv. Phaseolicola PK2. J. Gen. Microbiol. 137, 2281–2286. doi: 10.1099/00221287-137-10-2281
Kondo, T., Tsinoremas Nicholas, F., Golden Susan, S., Johnson Carl, H., Kutsuna, S., and Ishiura, M. (1994). Circadian clock mutants of cyanobacteria. Science 266, 1233–1236. doi: 10.1126/science.7973706
Kuzuyama, T. (2002). Mevalonate and nonmevalonate pathways for the biosynthesis of isoprene units. Biosci. Biotechnol. Biochem. 66, 1619–1627. doi: 10.1271/bbb.66.1619
Lee, T.-C., Xiong, W., Paddock, T., Carrieri, D., Chang, I.-F., Chiu, H.-F., et al. (2015). Engineered xylose utilization enhances bio-products productivity in the cyanobacterium Synechocystis sp. PCC 6803. Metab. Eng. 30, 179–189. doi: 10.1016/j.ymben.2015.06.002
Li, M., Ho, P. Y., Yao, S., and Shimizu, K. (2006). Effect of sucA or sucC gene knockout on the metabolism in Escherichia coli based on gene expressions, enzyme activities, intracellular metabolite concentrations and metabolic fluxes by 13C-labeling experiments. Biochem. Eng. J. 30, 286–296. doi: 10.1016/j.bej.2006.05.011
Li, T., Li, C.-T., Butler, K., Hays, S. G., Guarnieri, M. T., Oyler, G. A., et al. (2017). Mimicking lichens: incorporation of yeast strains together with sucrose-secreting cyanobacteria improves survival, growth, ROS removal, and lipid production in a stable mutualistic co-culture production platform. Biotechnol. Biofuels 10:55. doi: 10.1186/s13068-017-0736-x
Liang, Y., Tang, J., Luo, Y., Kaczmarek, M., Xingkang, L., and Daroch, M. (2019). Thermosynechococcus as a thermophilic photosynthetic microbial cell factory for CO2 utilisation. Bioresour. Technol. 278, 255–265. doi: 10.1016/j.biortech.2019.01.089
Liu, H., Cao, Y., Guo, J., Xu, X., Long, Q., Song, L., et al. (2021). Study on the isoprene-producing co-culture system of Synechococcus elongates–Escherichia coli through omics analysis. Microb. Cell Factories 20:6. doi: 10.1186/s12934-020-01498-8
Livak, K. J., and Schmittgen, T. (2001). Analysis of relative gene expression data using real-time quantitative PCR and the 2-DDCt method. Methods 25, 402–408. doi: 10.1006/meth.2001.1262
Löwe, H., Hobmeier, K., Moos, M., Kremling, A., and Pflüger-Grau, K. (2017). Photoautotrophic production of polyhydroxyalkanoates in a synthetic mixed culture of Synechococcus elongatus cscB and Pseudomonas putida cscAB. Biotechnol. Biofuels 10:190. doi: 10.1186/s13068-017-0875-0
Luo, Y., Zhang, T., and Wu, H. (2014). The transport and mediation mechanisms of the common sugars in Escherichia coli. Biotechnol. Adv. 32, 905–919. doi: 10.1016/j.biotechadv.2014.04.009
Ma, J., Guo, T., Ren, M., Chen, L., Song, X., and Zhang, W. (2022). Cross-feeding between cyanobacterium Synechococcus and Escherichia coli in an artificial autotrophic–heterotrophic co-culture system revealed by integrated omics analysis. Biotechnol. Biofuels Bioproducts 15:69. doi: 10.1186/s13068-022-02163-5
Ma, W., Liu, Y., Lv, X., Li, J., Du, G., and Liu, L. (2019). Combinatorial pathway enzyme engineering and host engineering overcomes pyruvate overflow and enhances overproduction of N-acetylglucosamine in Bacillus subtilis. Microb. Cell Factories 18:1. doi: 10.1186/s12934-018-1049-x
Marger, M. D., and Saier, M. H. (1993). A major superfamily of transmembrane facilitators that catalyse uniport, symport and antiport. Trends Biochem. Sci. 18, 13–20. doi: 10.1016/0968-0004(93)90081-W
Miller, G. L. (1959). Use of Dinitrosalicylic acid reagent for determination of reducing sugar. Anal. Chem. 31, 426–428. doi: 10.1021/ac60147a030
Minty, J., Singer, M., Bae, C. H., Ahn, J. H., Foster, C., Liao, J., et al. (2012). “Design and construction of synthetic fungi-bacteria consortia for direct production of isobutanol from cellulosic feedstocks.”
Mittermeier, F., Bäumler, M., Arulrajah, P., García Lima, J. de. J., Hauke, S., Stock, A., et al. (2022). Artificial microbial consortia for bioproduction processes. Eng. Life Sci. doi: 10.1002/elsc.202100152
Mukherjee, A., Sarkar, D., and Sasmal, S. (2021). A review of green synthesis of metal nanoparticles using algae. Front. Microbiol. 12:693899. doi: 10.3389/fmicb.2021.693899
Quintana, N., Van der Kooy, F., Van de Rhee, M. D., Voshol, G. P., and Verpoorte, R. (2011). Renewable energy from cyanobacteria: energy production optimization by metabolic pathway engineering. Appl. Microbiol. Biotechnol. 91, 471–490. doi: 10.1007/s00253-011-3394-0
Riaz, S., Jiang, Y., Xiao, M., You, D., Klepacz-Smolka, A., Rasul, F., et al. (2022). Generation of miniploid cells and improved natural transformation procedure for a model cyanobacterium Synechococcus elongatus PCC 7942. Front. Microbiol. 13:959043. doi: 10.3389/fmicb.2022.959043
Riaz, S., Xiao, M., Chen, P., Li, M., Cui, Y., and Daroch, M. (2021). Genome copy number of a thermophilic cyanobacterium Thermosynechococcus elongatus E542 is controlled by growth phase and nutrient availability. Appl. Environ. Microbiol. 87:e02993-20. doi: 10.1128/AEM.02993-20
Singh, A. K., and Ducat, D. C. (2022). Generation of stable, light-driven co-cultures of cyanobacteria with heterotrophic microbes. Methods Mol. Biol. 2379, 277–291. doi: 10.1007/978-1-0716-1791-5_16
Song, H., Ding, M. Z., Jia, X. Q., Ma, Q., and Yuan, Y. J. (2014). Synthetic microbial consortia: from systematic analysis to construction and applications. Chem. Soc. Rev. 43, 6954–6981. doi: 10.1039/c4cs00114a
Stanier, R., Kunisawa, R., Mandel, M., and Cohen-Bazire, G. (1971). Purification and properties of unicellular blue-green algae (order Chroococcales). Bacteriol. Rev. 35, 171–205. doi: 10.1128/BR.35.2.171-205.1971
Sugihara, J., Smirnova, I., Kasho, V., and Kaback, H. R. (2011). Sugar recognition by CscB and LacY. Biochemistry 50, 11009–11014. doi: 10.1021/bi201592y
Takahama, K., Matsuoka, M., Kazuhiro, N., and Ogawa, T. (2003). Construction and analysis of a recombinant cyanobacterium expressing a chromosomally inserted gene for an ethylene-forming enzyme at the psbAI locus. J. Biosci. Bioeng. 95, 302–305. doi: 10.1016/S1389-1723(03)80034-8
Tang, J., Jiang, D., Luo, Y., Liang, Y., Li, L., Shah, M. M. R., et al. (2018). Potential new genera of cyanobacterial strains isolated from thermal springs of western Sichuan, China. Algal Res. 31, 14–20. doi: 10.1016/j.algal.2018.01.008
Tarawat, S., Incharoensakdi, A., and Monshupanee, T. (2020). Cyanobacterial production of poly(3-hydroxybutyrate-co-3-hydroxyvalerate) from carbon dioxide or a single organic substrate: improved polymer elongation with an extremely high 3-hydroxyvalerate mole proportion. J. Appl. Phycol. 32, 1095–1102. doi: 10.1007/s10811-020-02040-4
Thiel, T., and Peter Wolk, C. (1987). “Conjugal transfer of plasmids to cyanobacteria,” in Methods in Enzymology. eds. R. Wu and L. Grossman (Waltham, MA: Academic Press), 232–243.
Thiel, T., and Poo, H. (1989). Transformation of a filamentous cyanobacterium by electroporation. J. Bacteriol. 171, 5743–5746. doi: 10.1128/jb.171.10.5743-5746.1989
Vajravel, S., Sirin, S., Kosourov, S., and Allahverdiyeva, Y. (2020). Towards sustainable ethylene production with cyanobacterial artificial biofilms. RSC Green Chem. 22, 6404–6414. doi: 10.1039/d0gc01830a
Valenzuela, R., Asperilla, J., and Cortés Corberán, V. (2008). Isoprene and C5 olefins production by oxidative dehydrogenation of Isopentane†. Ind. Eng. Chem. Res. 47, 8037–8042. doi: 10.1021/ie800756p
Veetil, V. P., Angermayr, S. A., and Hellingwerf, K. J. (2017). Ethylene production with engineered Synechocystis sp. PCC 6803 strains. Microb. Cell Factories 16:34. doi: 10.1186/s12934-017-0645-5
Venkata Mohan, S., and Venkateswar Reddy, M. (2013). Optimization of critical factors to enhance polyhydroxyalkanoates (PHA) synthesis by mixed culture using Taguchi design of experimental methodology. Bioresour. Technol. 128, 409–416. doi: 10.1016/j.biortech.2012.10.037
Wang, B., Eckert, C., Maness, P.-C., and Yu, J. (2018). A genetic toolbox for modulating the expression of heterologous genes in the cyanobacterium Synechocystis sp. PCC 6803. ACS Synth. Biol. 7, 276–286. doi: 10.1021/acssynbio.7b00297
Wang, B., Li, Y., Wu, N., and Lan, C. Q. (2008). CO2 bio-mitigation using microalgae. Appl. Microbiol. Biotechnol. 79, 707–718. doi: 10.1007/s00253-008-1518-y
Warr, S., Reed, R., and Stewart, W. (1985). Carbohydrate accumulation in osmotically stressed cyanobacteria (blue-green algae): interactions of temperature and salinity. New Phytol. 100, 285–292. doi: 10.1111/j.1469-8137.1985.tb02779.x
Watanabe, T., and Horiike, T. (2021). The evolution of molybdenum dependent Nitrogenase in cyanobacteria. Biology 10:329. doi: 10.3390/biology10040329
Weiss, T. L., Young, E. J., and Ducat, D. C. (2017). A synthetic, light-driven consortium of cyanobacteria and heterotrophic bacteria enables stable polyhydroxybutyrate production. Metab. Eng. 44, 236–245. doi: 10.1016/j.ymben.2017.10.009
Xiao, D., Zeng, L., Yao, K., Kong, X., Wu, G., and Yin, Y. (2016). The glutamine-alpha-ketoglutarate (AKG) metabolism and its nutritional implications. Amino Acids 48, 2067–2080. doi: 10.1007/s00726-016-2254-8
Zhang, L., Chen, L., Diao, J., Song, X., Shi, M., and Zhang, W. (2020). Construction and analysis of an artificial consortium based on the fast-growing cyanobacterium Synechococcus elongatus UTEX 2973 to produce the platform chemical 3-hydroxypropionic acid from CO2. Biotechnol. Biofuels 13:82. doi: 10.1186/s13068-020-01720-0
Zhang, G., Yang, X., Zhao, Z., Xu, T., and Jia, X. (2022). Artificial consortium of three E. coli BL21 strains with synergistic functional modules for complete phenanthrene degradation. ACS Synth. Biol. 11, 162–175. doi: 10.1021/acssynbio.1c00349
Zhao, Y., Yang, J., Qin, B., Li, Y., Sun, Y., Su, S., et al. (2011). Biosynthesis of isoprene in Escherichia coli via methylerythritol phosphate (MEP) pathway. Appl. Microbiol. Biotechnol. 90, 1915–1922. doi: 10.1007/s00253-011-3199-1
Keywords: ethylene, isoprene, cyanobacteria, co-culture, sucrose, microbial community
Citation: Cui Y, Rasul F, Jiang Y, Zhong Y, Zhang S, Boruta T, Riaz S and Daroch M (2022) Construction of an artificial consortium of Escherichia coli and cyanobacteria for clean indirect production of volatile platform hydrocarbons from CO2. Front. Microbiol. 13:965968. doi: 10.3389/fmicb.2022.965968
Received: 10 June 2022; Accepted: 23 September 2022;
Published: 21 October 2022.
Edited by:
Tarun Belwal, Zhejiang University, ChinaReviewed by:
Weiwen Zhang, Tianjin University, ChinaCopyright © 2022 Cui, Rasul, Jiang, Zhong, Zhang, Boruta, Riaz and Daroch. This is an open-access article distributed under the terms of the Creative Commons Attribution License (CC BY). The use, distribution or reproduction in other forums is permitted, provided the original author(s) and the copyright owner(s) are credited and that the original publication in this journal is cited, in accordance with accepted academic practice. No use, distribution or reproduction is permitted which does not comply with these terms.
*Correspondence: Maurycy Daroch, bS5kYXJvY2hAcGt1c3ouZWR1LmNu
†Present address: Sadaf Riaz, Department of Neuroscience, University of Connecticut School of Medicine, Farmington, CT, United States
Disclaimer: All claims expressed in this article are solely those of the authors and do not necessarily represent those of their affiliated organizations, or those of the publisher, the editors and the reviewers. Any product that may be evaluated in this article or claim that may be made by its manufacturer is not guaranteed or endorsed by the publisher.
Research integrity at Frontiers
Learn more about the work of our research integrity team to safeguard the quality of each article we publish.