- 1Department of Microbiology, Faculty of Biology, University of Bucharest, Bucharest, Romania
- 2Research Institute of University of Bucharest, Bucharest, Romania
- 3The Institute for Diagnostic and Animal Health (IDSA), Bucharest, Romania
- 4Faculty of Veterinary Medicine, University of Agronomic Sciences and Veterinary Medicine of Bucharest, Bucharest, Romania
- 5National Institute for Public Health and the Environment, Bilthoven, Netherlands
- 6Wageningen Livestock Research, Wageningen, Netherlands
- 7Institute for Applied Microbiology Heinrich-Buff-Ring, Justus-Liebig University, Gießen, Germany
- 8Department of Pathobiology, University of Guelph, Guelph, ON, Canada
- 9Department of Biology, Agriculture and Agri-Food Canada, University of Western Ontario, London, ON, Canada
- 10Academy of Romanian Scientists, Bucharest, Romania
- 11The Romanian Academy, Bucharest, Romania
The intensive use of antibiotics in the veterinary sector, linked to the application of manure-derived amendments in agriculture, translates into increased environmental levels of chemical residues, AR bacteria (ARB) and antibiotic resistance genes (ARG). The aim of this review was to evaluate the current evidence regarding the impact of animal farming and manure application on the antibiotic resistance pool in the environment. Several studies reported correlations between the prevalence of clinically relevant ARB and the amount and classes of antibiotics used in animal farming (high resistance rates being reported for medically important antibiotics such as penicillins, tetracyclines, sulfonamides and fluoroquinolones). However, the results are difficult to compare, due to the diversity of the used antimicrobials quantification techniques and to the different amounts and types of antibiotics, exhibiting various degradation times, given in animal feed in different countries. The soils fertilized with manure-derived products harbor a higher and chronic abundance of ARB, multiple ARG and an enriched associated mobilome, which is also sometimes seen in the crops grown on the amended soils. Different manure processing techniques have various efficiencies in the removal of antibiotic residues, ARB and ARGs, but there is only a small amount of data from commercial farms. The efficiency of sludge anaerobic digestion appears to be dependent on the microbial communities composition, the ARB/ARG and operating temperature (mesophilic vs. thermophilic conditions). Composting seems to reduce or eliminate most of antibiotics residues, enteric bacteria, ARB and different representative ARG in manure more rapidly and effectively than lagoon storage. Our review highlights that despite the body of research accumulated in the last years, there are still important knowledge gaps regarding the contribution of manure to the AMR emergence, accumulation, spread and risk of human exposure in countries with high clinical resistance rates. Land microbiome before and after manure application, efficiency of different manure treatment techniques in decreasing the AMR levels in the natural environments and along the food chain must be investigated in depth, covering different geographical regions and countries and using harmonized methodologies. The support of stakeholders is required for the development of specific best practices for prudent – cautious use of antibiotics on farm animals. The use of human reserve antibiotics in veterinary medicine and of unprescribed animal antimicrobials should be stopped and the use of antibiotics on farms must be limited. This integrated approach is needed to determine the optimal conditions for the removal of antibiotic residues, ARB and ARG, to formulate specific recommendations for livestock manure treatment, storage and handling procedures and to translate them into practical on-farm management decisions, to ultimately prevent exposure of human population.
Introduction
Antimicrobial resistance (AMR) is ranked in the top 10 public health global challenges and top 20 global causes of mortality and morbidity according to WHO projections for 2030, causing a real health care crisis, worsened by the innovation gap in the development of novel antimicrobial drugs [World Health Organization (WHO), 2015, 2021]. Even if clinical use is the main driver for AMR increase in humans, the environment represents both an important AMR reservoir (natural or intrinsic resistance being an essential component of the armamentarium used by different microbial species to maximize competition, resist predation and colonize different niches) and an important transmission route to humans (Walsh, 2013; Nguyen et al., 2020).
The intensive use of antibiotics in the clinical, industrial, veterinary and agricultural sectors led to increased environmental levels of chemical residues, AR bacteria (ARB) and antibiotic resistance genes (ARG). It was estimated that the global antimicrobials consumed in livestock production was 93,309 tonnes in 2017, and projected an increase of 11.5% by 2,030–104,079 tonnes (95% CI: [69,062, 172,711]) (Boeckel et al., 2017; Tiseo et al., 2020; Figure 1). The joint Food and Agriculture Organization of the United Nations (FAO), WHO and World Organization for Animal Health (OIE) expert meeting document on foodborne AMR shows that >80% of antimicrobials and dietary copper and zinc is excreted in active form (FAO and WHO, 2019) and accumulate in different environmental compartments.
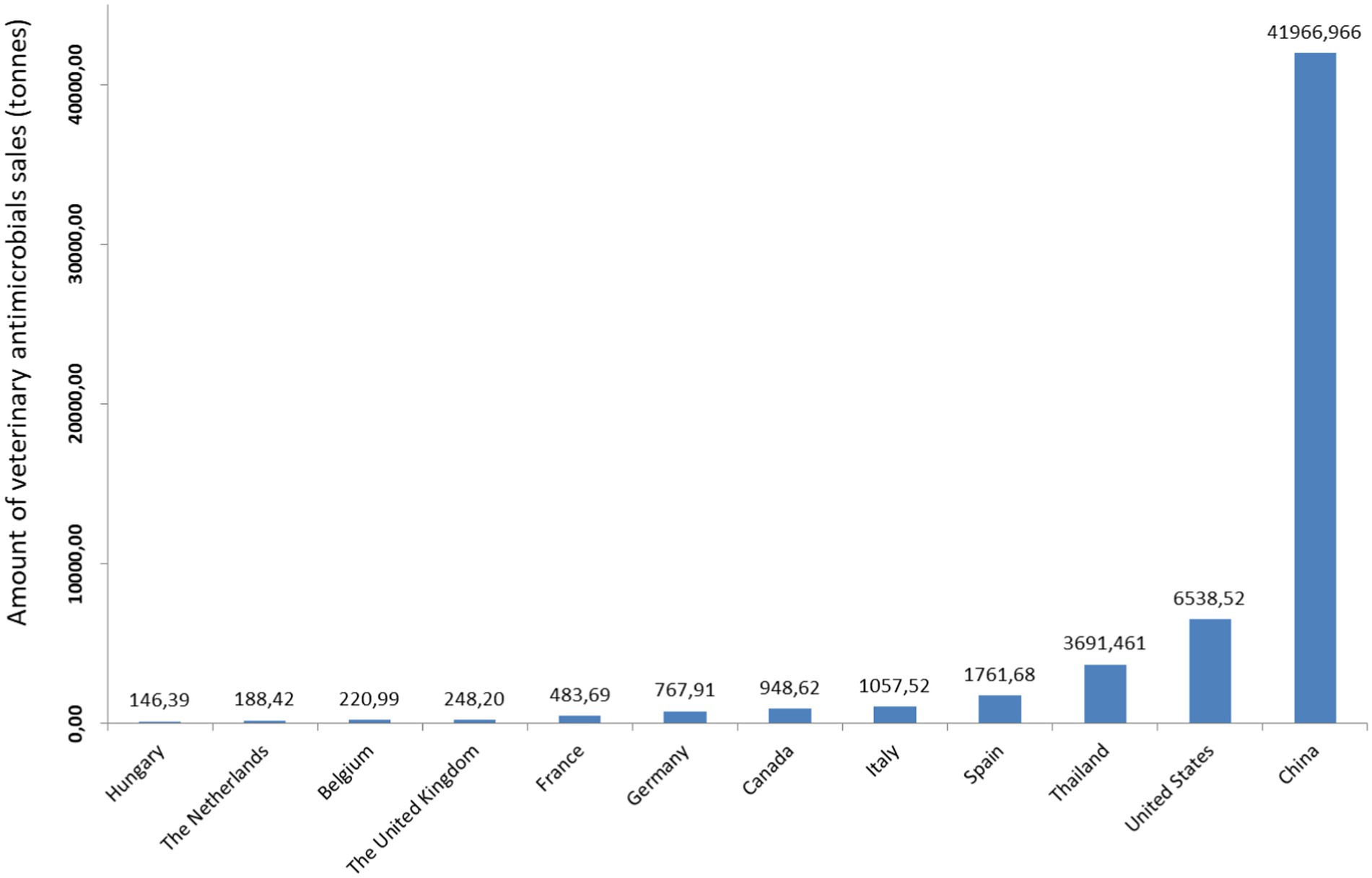
Figure 1. Reported sales of veterinary antimicrobials by country in 2017 (Tiseo et al., 2020). The figure is created using Biorender.com.
Under the selection pressure of different chemical contaminants released and accumulated in different environmental compartments, ARG can be exchanged through transduction or conjugation, between even distantly related bacteria (van Hoek et al., 2011; Vikesland et al., 2017; Popa et al., 2018) or novel resistance factors could occur through mutation (Huijbers et al., 2019). Sub-inhibitory levels of selective agents such as antibiotics or heavy metals have been shown to increase the abundance of ARG and mobile genetic elements (MGEs) through co-resistance (selectable for different ARG on the same MGE) and cross-resistance (selectable for an ARG encoding for a common mechanism of resistance to both antibiotics and biocides; Jutkina et al., 2016; Zhang et al., 2018; Cao et al., 2020). Moreover, the ARG associated with dead bacteria or free DNA could also contribute to acquired resistance through transformation (Woegerbauer et al., 2020). Also, the environmental pollution with antibiotic residues may alter the soil microbiota composition, enriching soils with bacteria able to derive nutritional benefits from these drugs, resulting in their accelerated biodegradation (Topp et al., 2013).
The increase in manure inputs and/or agriculture derived antibiotics due to the intensification of livestock production raises serious concerns for both human and environmental health, following direct application of manures to farmlands (e.g., exposure of crops and consumers to ARB, emergence/accumulation/spread of resistance to antibiotics used human medicine etc.; Van Epps and Blaney, 2016; Wepking et al., 2017; Collignon and McEwen, 2019).
The aim of this review is to provide insights into the impact of animal farming and manure application on antibiotics residues and antibiotic resistance in the environment, to identify the knowledge gaps and highlight the best practices for livestock manure treatment, storage and handling procedures and for prudent-cautious use of antibiotics in livestock production.
Livestock production as a source of antibiotic residues
One of the critical points to mitigate dissemination, accumulation and transmission of AMR into the environment is to identify the reservoirs of ARB, ARG and antibiotics. Worldwide, it is estimated that 73% of all antibiotics are used in livestock farming, not in human medicine, supporting a growing awareness of the associated risks (Boeckel et al., 2017; Van et al., 2020). A large proportion of these antimicrobials are used for controlling infectious diseases that would otherwise inflict severe losses or even prevent intensive production completely (Aarestrup, 2015). WHO reports that 57% of all antimicrobials used in animal production are essential for human medicine (Figure 2). In this regard, CIA (critically important antimicrobials) lists have been created by WHO and national governments in order to prioritize and protect the most essential antibiotics [World Health Organization (WHO), 2019].
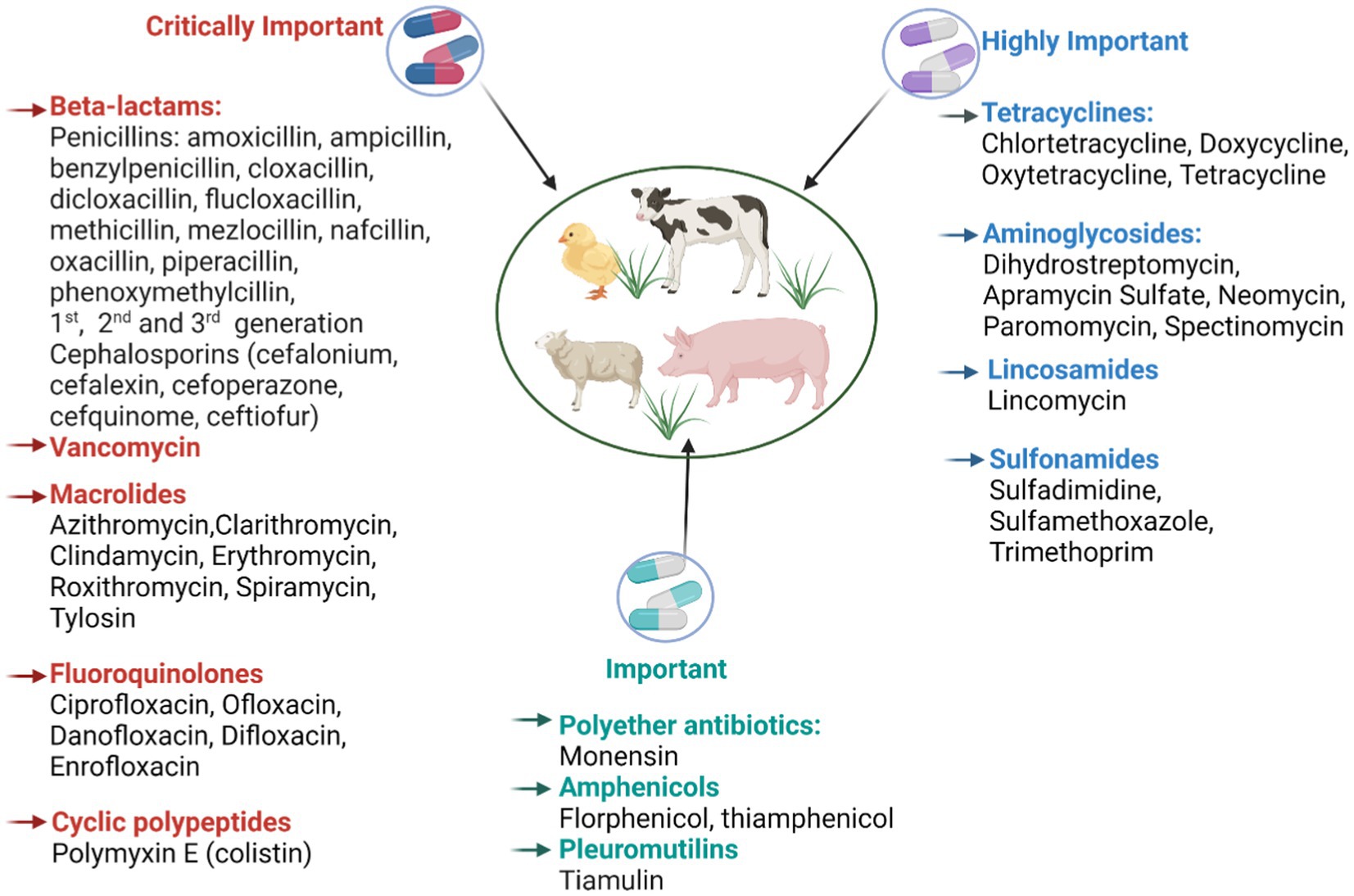
Figure 2. Major classes of antibiotics and representatives used in livestock production. The figure is created using Biorender.com.
The FDA has highlighted three CIA classes, i.e., third generation cephalosporins, macrolides and fluoroquinolones as the critically important classes. According to the most recent FDA (2020) report on antibiotic sales, these three CIA classes accounted for around 5% of total antibiotics sales for use in farm animals (FDA, 2020). The same three, along with fourth generation cephalosporins, were tagged by WHO as priorities for attention and funding in the battle against AMR.
In the US, 70%–80% of the total antibiotics sold per year are used in animals (Elliott et al., 2017) and according to the FDA, 57% of medically important ones belong to the same classes as drugs used in human health (FDA, 2020). In the US, 82% of the medically important antibiotics were estimated to be used in cattle (41%) and swine (941%; FDA, 2020). Tetracyclines are among the most widely used in the US, accounting for 66%–67% of the medically important antimicrobials, being primarily sold for use in cattle and swine (FDA, 2019, 2020; Scott et al., 2019).
According to the fourth edition of OIE annual report on antimicrobial agents intended for use in animals, in Europe, bovines account for the highest percentage of antibiotic consumption (38.3%) followed by swine (27.1%; OIE, 2018). The total use of antibiotics in the EU has been estimated in different studies to range from 20 to 188 mg kg−1 of animal (slaughtered pigs, poultry and cattle plus estimated biomass of live dairy cattle; Grave et al., 2010; Pikkemaat et al., 2016). In the EU, tetracyclines accounted for <40% (35.3%) in 2016 (OIE, 2018).
Antibiotics administered to animals modify the gut microbiota signatures, as demonstrated for chlortetracycline, sulfamethazine, and penicillin which induced, after 14 days of administration in swine, an increase in Escherichia coli populations, known for its pathogenic potential, but also in the abundance and diversity of ARGs, some of them, such as aminoglycoside O-phosphotransferases, conferring resistance to antibiotics that were not administered, demonstrating the potential for indirect selection of resistance to classes of antibiotics not fed (Looft et al., 2011). Moreover, the decrease of gut diversity by antibiotics might facilitate the overgrowth of already resistant microbes and the HGT of ARGs. It has been demonstrated that antibiotics could promote the HGT by: (i) activation of the SOS response, which increases the transfer of diverse mobile genetic elements (pathogenicity islands, integrating conjugative element, conjugative transposons, plasmids etc.; Couce and Blázquez, 2009); (ii) promoting transduction, by stimulating prophage excision and host cell lysis and also the receptor strain (Hastings et al., 2004; Lopatkin et al., 2016); (iii) increasing PT, as demonstrated, e.g., for ESBL carrying plasmids in E. coli (Liu et al., 2019). Moreover, the mutations and recombinations are also increased in the presence of antibiotic stress (Couce and Blázquez, 2009).
Antibiotics released in the natural environment could affect microbial diversity with important ecological consequences (Duygan et al., 2021).
The use of antibiotics in livestock production is mirrored by the presence of these agents in animal manures. Animal manures are mixtures of animal feces, urine, bedding materials and other materials associated with animal production (Shober and Maguire, 2018). Due to their high organic nutrient content of animal manures, they are widely used as natural fertilizers to increase food production yields. The antibiotic residue concentrations found in manures vary from study to study (Table 1). There are at least three reasons for this occurrence: (i) the diversity of the quantification techniques, recovery efficiencies, sensitivity and reliability measures; (ii) having different quantities and types of antibiotics, with different degradation times (DT) given in animal feed which differ from country to country; (iii) different fate of antibiotics in the animal gut (catabolization pathway, the resulting residues) and soil (biodegradation depends on the structure of soil microbiota etc.). In relation to the first reason, suitable analytical methods for the quantification of antibiotics in the complex sample matrix represented by animal manure and treatment products are needed. A single liquid–liquid extraction step and analysis via liquid chromatography (LC) and triple quadrupole mass spectrometry, with a detection limit ranging from 0.01 to 0.08 mg/kg has been developed for the analysis of different sulfonamides and tetracyclines in biogas plant input and output samples (Spielmeyer et al., 2014). Antibiotics can be removed by physical adsorption, chemical oxidation, photodegradation, and biodegradation (Liu et al., 2021). Although biodegradation is receiving increasing attention due to its multiple advantages (green, low cost and easy to perform operation), it must be taken into account that many of the clinically important antibiotics are not easily biodegradable (Alexy et al., 2004).
Fluoroquinolone, sulfonamide, and tetracycline classes are the most widely detected antibiotics in different types of manure (Table 1). One study has shown that the consumption of tetracyclines, as the most frequently detected antibiotics in agricultural soils fertilized with animal manure, was directly correlated with the size of size of the farm and the amounts of tetracyclines found in the fertilized soils (Carballo et al., 2016).
As shown in Table 1, the amount of antibiotics in swine, poultry and cattle manure ranged between 0.01 and 100 mg/kg, the concentration can be higher, of >1,000 mg/kg, however (Youngquist et al., 2016).
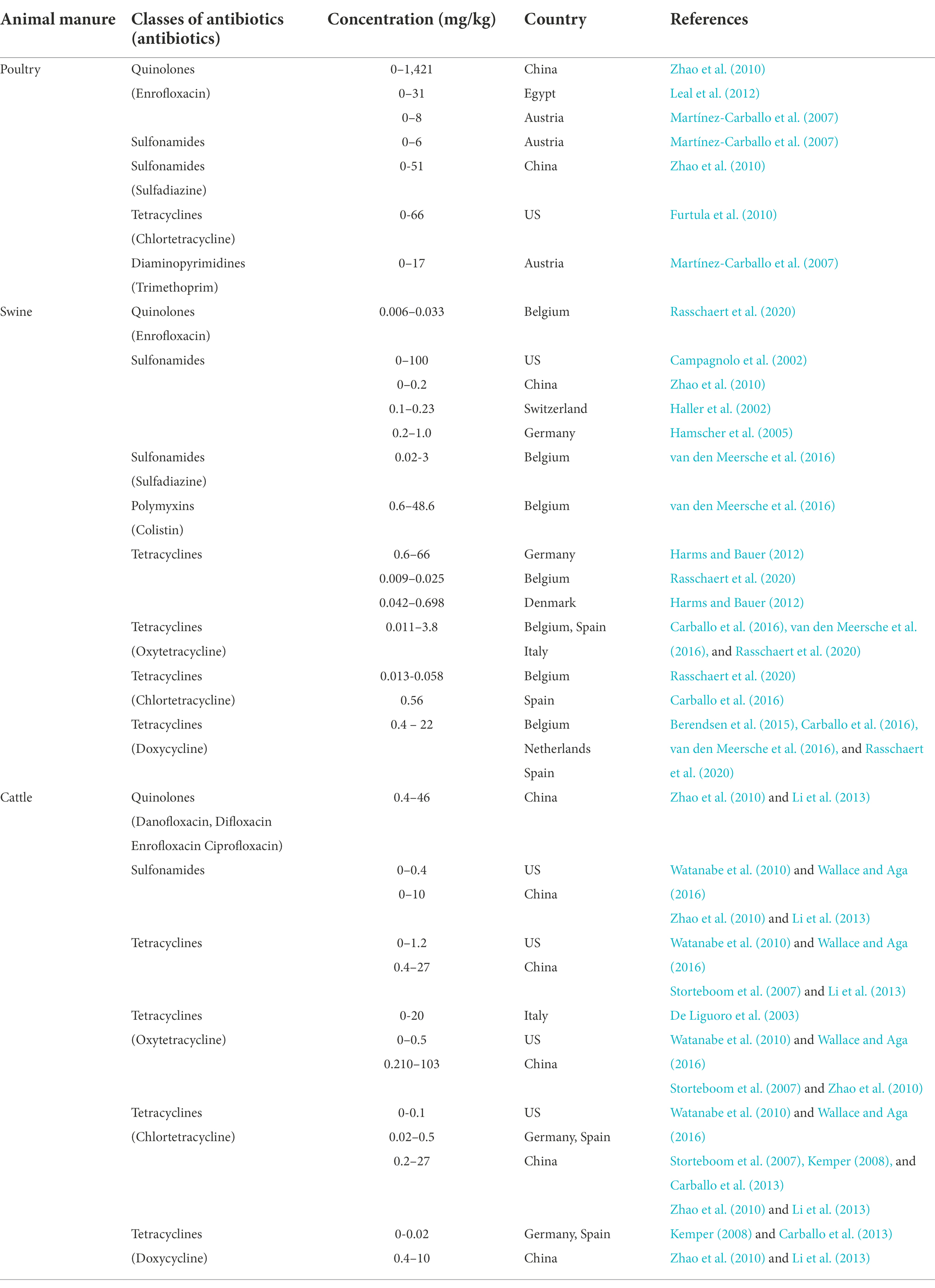
Table 1. The amount of antibiotics detected in poultry, swine and cattle manure in different countries.
The highest concentrations are reported in poultry, for fluoroquinolones, in cattle for oxytetracycline and for sulfonamides in swine manure (Figure 3).
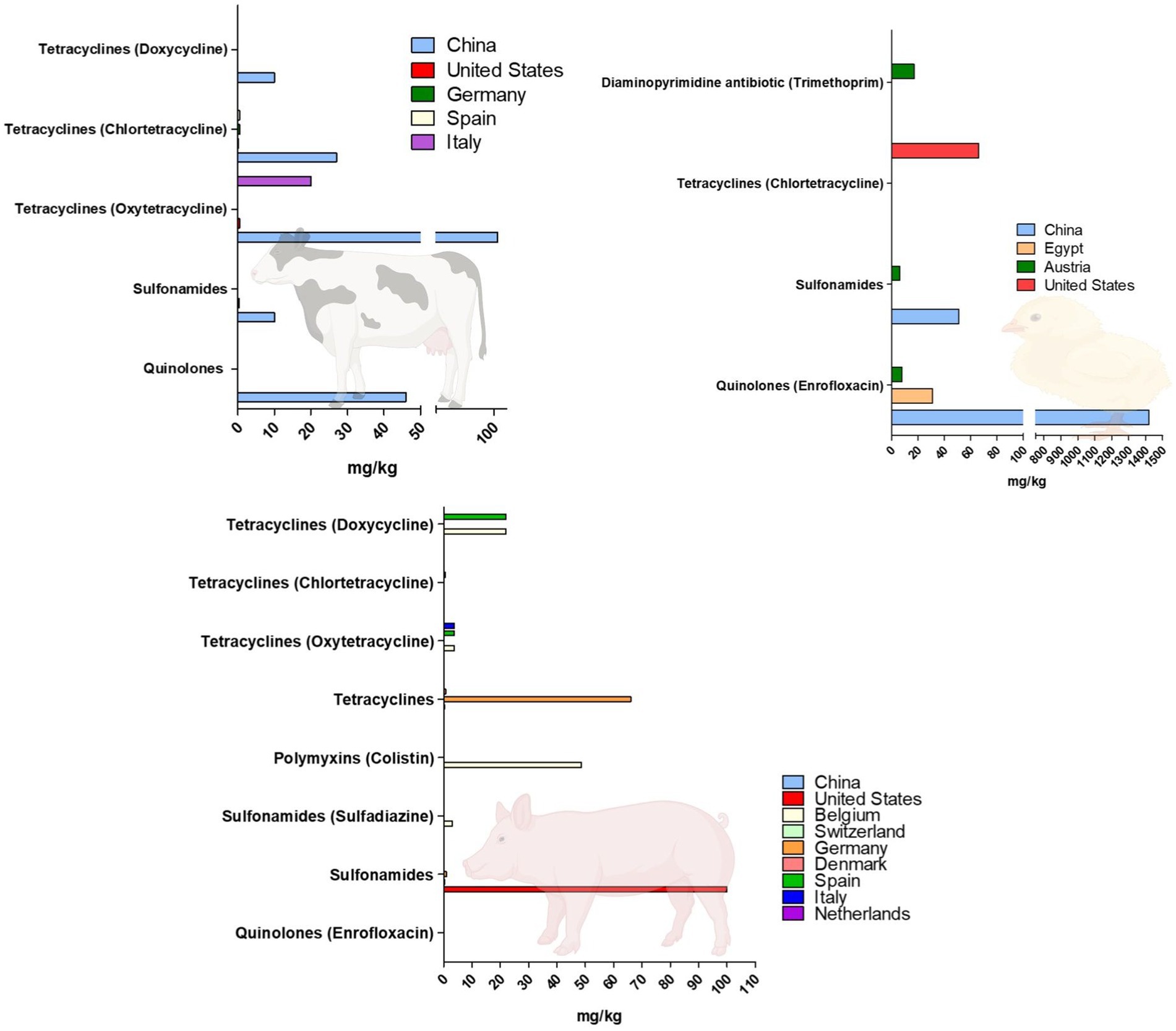
Figure 3. Graphic representation of the highest concentrations of antibiotics detected in animal manure (cattle, poultry, swine), reported in different studies. The figure is created using Biorender.com.
Therefore, antibiotic residues enter the environment through the use of animal wastes, raising increasing concerns about their contribution to the AMR reservoir (Amador et al., 2019; Tyrrell et al., 2019).
Some of the antibiotic residues are excreted intact in manure, where they form complexes with soluble organics, preventing their degradation and removal during manure storage (Massé et al., 2014). Other antibiotics such as sulfamethazine, tylosin and chlortetracycline are rapidly degraded in manure amended soils, the half-live or DT50 ranging from 2 to 42 days (Halling-Sørensen et al., 2005; Carlson and Mabury, 2006; Accinelli et al., 2007; Topp et al., 2013). The half-life of oxytetracycline in manure from calves was 30 days but the compound was still detectable in this matrix (820 μg/kg) after 5 months maturation (De Liguoro et al., 2003).
Animal manure as a reservoir of ARB and ARG
Currently, the application of manure and slurry in agriculture is considered as a key contributor in the flow of ARG among humans, animals and terrestrial and aquatic environments (Figure 4; Topp et al., 2018; Durso and Cook, 2019). Many studies have shown that the application of manure from antibiotic-treated animals to soil was found to enlarge the reservoir of clinically relevant ARB and ARG (Table 2) when compared to soils that received inorganic fertilizers or no fertilizers (Storteboom et al., 2010; Heuer et al., 2011; Cao et al., 2020).
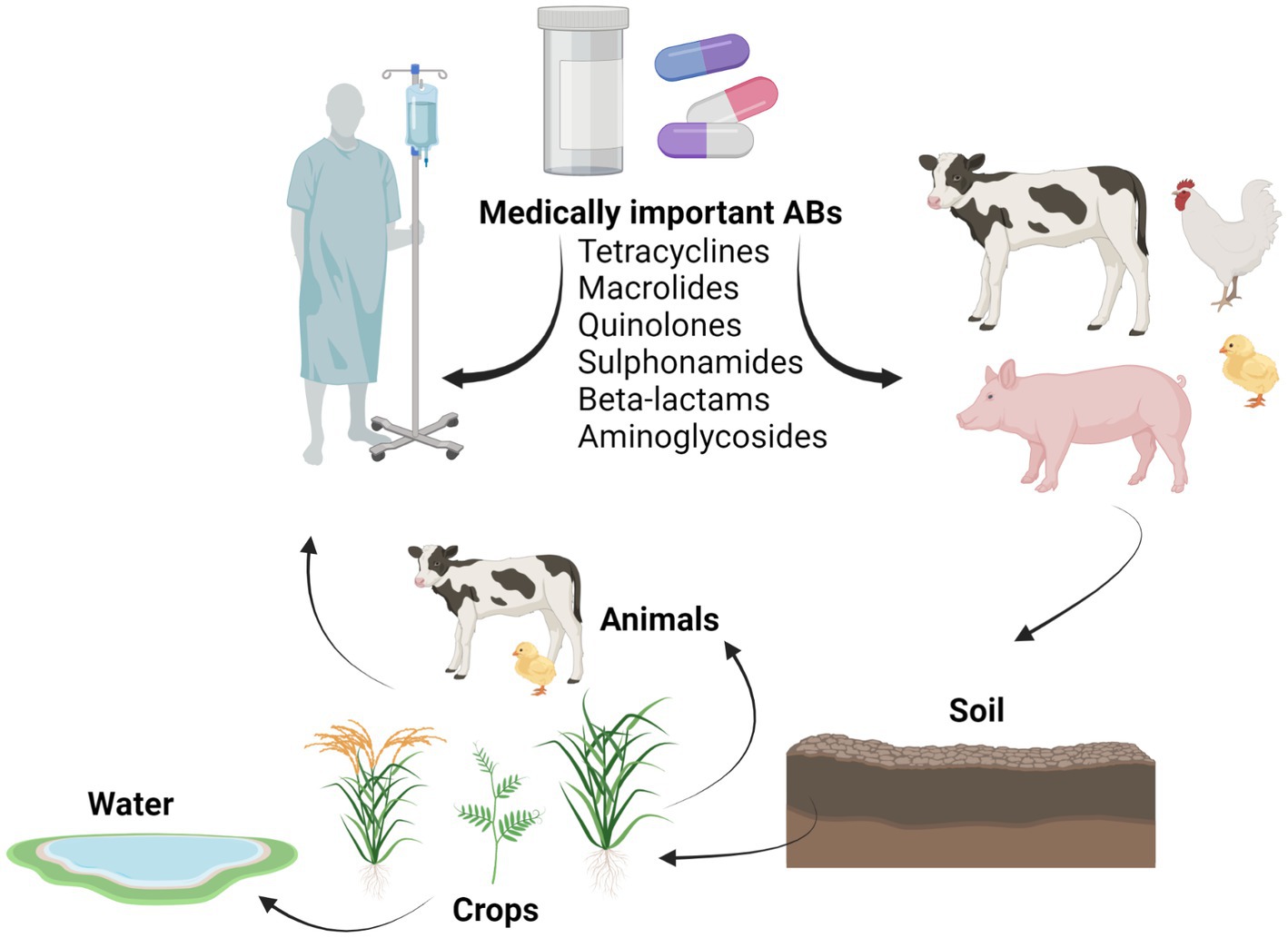
Figure 4. Contribution of manure to the flux of antibiotics from livestock to the natural environment. The figure is created using Biorender.com.
Several studies reported correlations between the prevalence of clinically relevant ARB and the amount and classes of antibiotics used in livestock production (Landers et al., 2012; Chantziaras et al., 2013; EMA, 2019). In the Netherlands, levels ranging from 10*2 to 10*4 CFU/g of E. coli, spores of sulfite reducing Clostridia and intestinal enterococci have been found in the input stream and were correlated with the presence of these microorganisms in the RO-concentrate of nine installations producing mineral concentrate from pig slurry (Hoeksma et al., 2015, 2020, 2021).
However, most studies investigating the presence of ARB in livestock have focused on Enterobacteriaceae including Escherichia coli and zoonotic Salmonella spp. (Feßler and Schwarz, 2017) and fewer have focused on other genera, such as Enterococcus, Staphylococcus etc. (Oliver et al., 2020). The available reports indicated high resistance rates for the most frequently used antibiotic classes: beta-lactams (Dandachi et al., 2018), tetracyclines and sulfonamides (Amador et al., 2019). For some of the nine European countries included in a cross-sectional study, an association was observed between the use of penicillins and resistance to ampicillin in isolates from healthy broilers (Hou et al., 2017; Ceccarelli et al., 2020). The E. coli isolates from broilers exhibited the highest resistance to (fluoro) quinolones, and multidrug resistance (MDR) was also detected in broilers and fattening turkeys (Ceccarelli et al., 2020). The decrease of colistin usage in the veterinary medicine world widely led to the decrease of colistin resistance to very low levels (Doi, 2019; Miguela-Villoldo et al., 2022). In the Netherlands, a considerably reduced antimicrobial consumption in food-producing animals was correlated with a low prevalence of ARB from broilers (MARAN, 2018). Similarly, in Germany, the level of AMR in commensal E. coli from livestock has been significantly decreased (EFSA, 2018).
Many studies have indicated that farmlands could host multiple ARG (Table 3; Baquero et al., 2008; Cheng et al., 2013; Udikovic-Kolic et al., 2014; Wichmann et al., 2014; Rahman et al., 2018). Moreover, Graham et al. (2016) suggested a historical association between ARG in animal manure and humans, demonstrating that higher β-lactam ARG levels were detected in soils fertilized with manure versus those amended with inorganic fertilizers, after the introduction of penicillin in 1940 (Graham et al., 2016). A cross sectional study regarding possible correlations between antimicrobial usage and the pig faecal mobile resistome conducted in nine European countries provided robust evidence of direct ARG selection by the two widely used antimicrobial classes, i.e., macrolides and tetracyclines (Van Gompel et al., 2019). Additionally, cross-resistance to macrolide and lincosamide usage and co-selection of ARG was also observed (Van Gompel et al., 2019). In a very recent study conducted in a high-density farming area of Northern Italy, it has been found that flumequine concentrations increased after manure application, positively correlated with the oqxA and qnrS genes abundance (Laconi et al., 2021). A recent metagenomic study evaluated the impact of bovine and poultry manure on the diversity and abundance of ARGs and the associated mobilome in soil and crops (Buta-Hubeny et al., 2022). The poultry manure contributed four times more than bovine manure to the total number of ARGs for different classes of antibiotics (tetracyclines, aminoglycosides, sulfonamides, bacitracin, chloramphenicol, and macrolide-lincosamide-streptogramin) found in manure, the fertilized soil and bacteria isolated from crops (Buta-Hubeny et al., 2022).
Antibiotic resistance genes can persist in soil for >120 days (Gou et al., 2018; Han et al., 2018) and can take from three to 6 months to attenuate to levels less than or equal to background (Chen et al., 2019; Lopatto et al., 2019). This implies that soils which are in constant agricultural use might continuously harbor a relatively stable ARB population and ARG pool (Schmitt et al., 2006; Cheng et al., 2013; Ruuskanen et al., 2016). However, more evidence, including quantitative data from exposure-relevant sites and environmental compartments is needed to evaluate the contribution of antibiotics containing manures to the environmental selection for AMR and its dispersal routes (Huijbers et al., 2015; Hassell et al., 2019). This evidence will allow the implementation of targeted monitoring programs and interventions to prevent resistant pathogens, as well as novel ARG, from reaching humans (Bengtsson-Palme et al., 2018; MARAN, 2018).
Studies are also reporting evidence for the potential of ARG transfer between animal-related and human-associated bacteria (Hu H. et al., 2016a, Hu Y. et al., 2016b; Pal et al., 2016; Pérez-Valera et al., 2019; Yuan et al., 2020). Manure offers some particular features, such as nutrients richness, high abundance and diversity of bacterial populations and antibiotic residues, that could favor the ARGs dissemination by horizontal gene transfer (Heuer et al., 2011; Lima et al., 2020). The insertion sequences (IS), including those associated with the mobility of ARGs in the population of ESKAPE pathogens, are introduced to soil with manure and remain stable for up to several months, indicating an increased risk of rapid ARG transfer, particularly when associated with bacteria from phylum Proteobacteria (Buta-Hubeny et al., 2022).
The abundance of 95 ARGs and MGEs from soils fertilized with dairy cow manure-derived amendments (slurry, fresh manure, aged manure), and plants (wheat grain and lettuce) was analyzed by high-throughput qPCR. The structure of soil prokaryotic communities was determined by 16S rRNA amplicon sequencing and qPCR (Jauregi et al., 2021). A higher ARGs abundance was found in slurry vs. fresh or aged manure, in soil vs. plant samples, and in wheat grain vs. lettuce (Jauregi et al., 2021; Zalewska et al., 2021).
Manure treatment impacts on persistence, selection, enrichment and dissemination of AMR
The available manure processing technologies are currently not taking into consideration, in terms of treatment performance, the removal of antibiotics, ARG and ARB, although evidence exists that ARB and ARG are not completely eliminated during manure treatments, contributing to the environmental resistome (Hou et al., 2017; Figure 5).
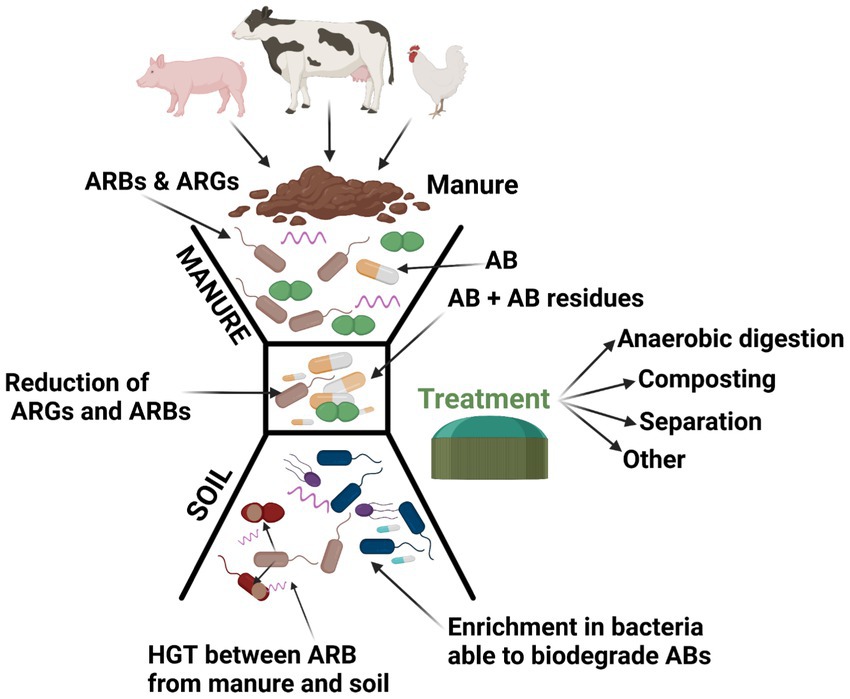
Figure 5. Effects of manure treatment on ABs and ARB, ARGs. The figure is created using Biorender.com.
In a recent review, it has been shown that the uncovered lagoons are the least effective removing tetracycline resistance gene; however, their performance could be gradually improved if covered lagoons and biofiltration are sequentially added. Further, mesophilic digestion was not effective, in contrast with thermophilic AD. Aerobic thermophilic composting and postdigestion composting reduced ARGs by >80% (Agga et al., 2022). These results suggest that different manure management practices have different efficiencies in removing antibiotics, ARB and ARG. In the following sections of the manuscript, we will focus on the efficiency of anaerobic digestion (AD) and composting techniques.
Liquid (with a dry matter content of 6%–12% or less if flushing is applied) or solid (with a dry matter content of 20%–65%) animal manure are treated by different technologies to obtain organic fertilizer products. In the first step, the liquid manure is separated into two fractions, i.e., the liquid (3%–6% dried matter content), which is generally applied as fertilizer and the solid one.
The tetracycline residues are largely removed from the liquid fraction, the antibiotic being absorbed onto the solid fraction of manure. Thus reduction of the antibiotic load in the liquid fraction is an important first step in manure treatment (Wallace et al., 2018). On the contrary, to their poor absorption in the animal gut and/or reversible metabolization, sulfonamides are excreted in significant amounts and are persisting in the liquid manure and then entering the environment. In the groundwater samples from Lower Saxony agricultural areas, sulfonamides were detected in concentrations up to 100 ng L−1 (Spielmeyer et al., 2017).
The solid fraction can be further composted, dried and eventually pelletized or incinerated, while the liquid fraction can be used as fertilizer (eventually contributing to the dissemination of antibiotic residues, ARG and zoonotic bacteria into the environment) or further treated by different procedures (Lyngsø et al., 2011; Dungan et al., 2018; Van den Meersche et al., 2019; Oliver et al., 2020).
Both liquid and solid manure can be used for biogas production in AD systems, which are the most frequent treatments in Europe, being applied to treat 6.4% of the total manure production in 2010 (LIFE+MANEV, 2015).
Another common treatment of manure is represented by composting (microbial decomposition of organic matter under controlled aerobic conditions), the resulting product being more suitable for direct soil application. The aerobic composting process begins with the activity of mesophilic organisms, at 20–45°C, which will generate the appropriate conditions for the activity of thermophilic fungi and bacteria, with optimum growth temperature of 50–70°C. Regular turning of the pile will speed up the decomposition process, from several months in case of extensive outdoor composting in windrows with limited turning to 1–2 weeks in intensive tunnel composting with forced aeration (Melse and de Buisonjé, 2020).
Effect of AD and composting on antibiotic residues
Anaerobic digestion
The rates of degradation of antibiotics during AD are dependent on their chemical structure, but also on manure characteristics and type, the reported degradation rates differing from study to study (Arikan et al., 2009; Alvarez et al., 2010; Kasumba et al., 2020; Lee et al., 2020).
Tetracycline and sulfonamide antibiotics were shown to persist in the animal manure (swine, cattle, and poultry) after AD, which can potentially lead to the emergence and persistence of tetracycline resistant bacteria in the environment when the AD byproducts are applied on soils for crop production (Spielmeyer et al., 2014, 2015, 2017; Kasumba et al., 2020). In case of tetracyclines, the DT50 during AD reported in different studies varied from >300 to 3.8 days in comparison with composting (Figure 6).
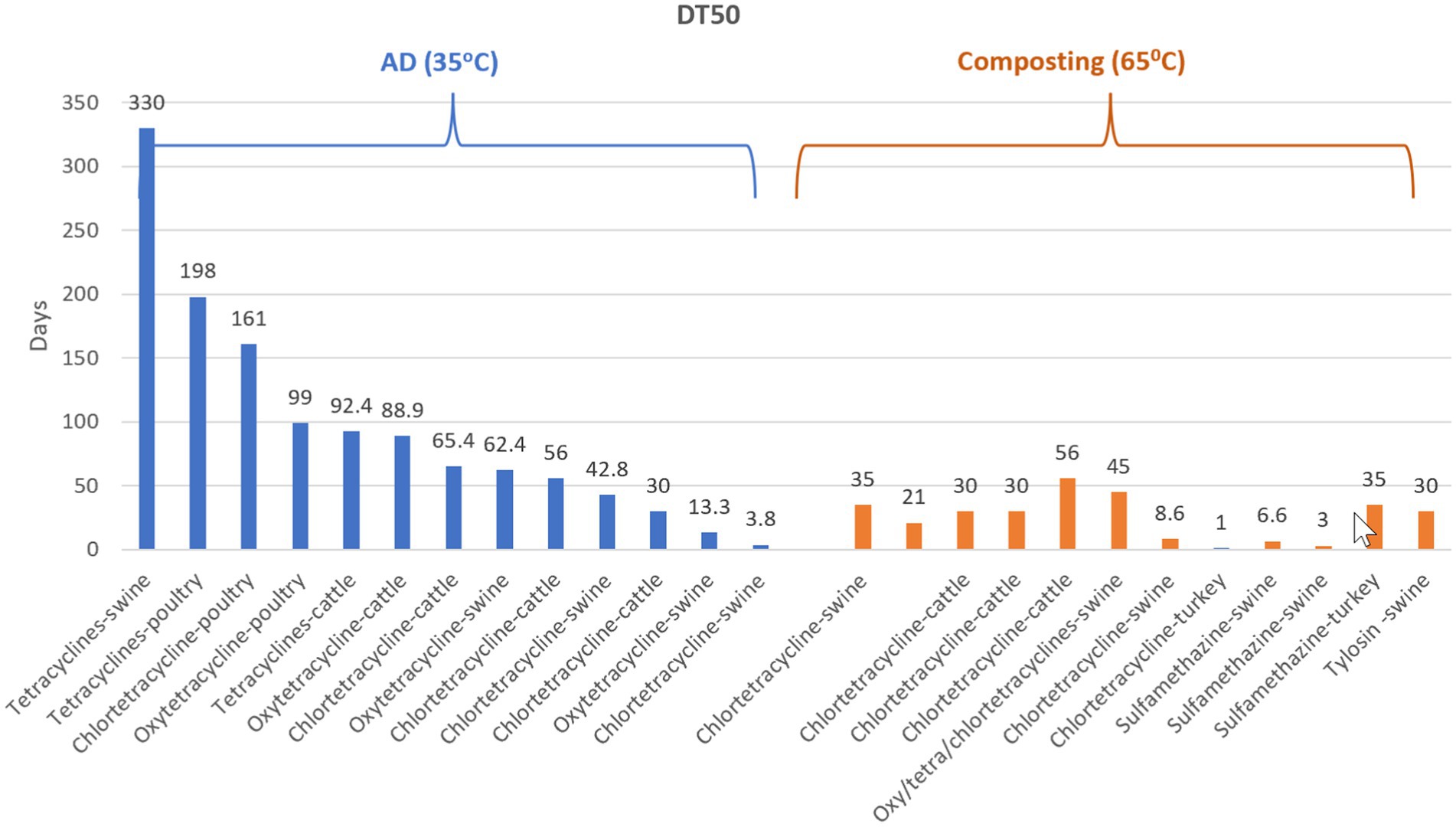
Figure 6. Comparative representation of the DT50 of different antibiotics during AD and composting of different types of manure (poultry, swine, cattle, turkey), reported in different studies (Osman et al., 2006; Dolliver et al., 2008; Alvarez et al., 2010; Hu et al., 2011; Kim et al., 2012; Selvam et al., 2012; Ray et al., 2017; Kasumba et al., 2020; Lee et al., 2020). The figure is created using Biorender.com.
Composting
Composting reduces or eliminates many antibiotic residues in manure mainly by temperature dependent abiotic processes such as adsorption and/or degradation rather than biotic processes (Dolliver et al., 2008; Arikan et al., 2009; Wu et al., 2011; Kim et al., 2012; Ray et al., 2017; Liu et al., 2018; Cheng et al., 2019). For most antibiotics the calculated half-lives during composting ranged from 0.9 to 16 days (Oliver et al., 2020; Figure 6). In the case of cow manure stockpiles, the degradation of antibiotics takes place in weeks, months or longer and is dependent on the type of antibiotics, and interaction between different antibiotics (Oliver et al., 2020). For example, chlortetracycline was found to decline rapidly, with <1% of the starting concentration detectable after day 17, in stockpiled cattle manure. However, the degradation rate slowed down to 56 days when chlortetracycline and sulfamethazine were both present in a stockpile (Sura et al., 2014). These results suggest that when multiple antibiotics are present, some of them could inhibit the bacteria able to degrade other antibiotics (Oliver et al., 2020). However, in case of other antibiotics associations, the degradation rates are enhanced probably due to the selection of compost microbial associations able to act on multiple compounds (Storteboom et al., 2007; Arikan et al., 2009).
Effects of AD and composting on ARB and ARG
Anaerobic digestions
The efficiency of AD in the removal of ARB and ARG appears to be dependent on the digester operating temperature. Anaerobic digestion at thermophilic conditions seems to be better than mesophilic AD at reducing ARB and ARG (Sun et al., 2016; Wallace et al., 2018; Gros et al., 2019; Huang et al., 2019; Zou et al., 2020). Zou et al. (2020) found that during thermophilic AD of pig manure, ARB numbers (sulfonamide / tetracycline resistant bacteria) were reduced by 4-log CFUs per gram dry manure, but only by approximately 1-log CFU at mesophilic temperature. Sun et al. (2016) observed that tetracycline resistance genes decreased when the operating temperature was in the thermophilic range. Eight out of 10 detected ARG declined at 55°C, but only five out of 10 and four out of 10 ARG decreased at 35°C and 20°C, respectively (Sun et al., 2016). However, Huang et al. (2019) found that thermophilic AD of swine manure did not achieve a better removal of abundance of total ARG compared to mesophilic AD.
Mesophilic AD seems to work only for certain ARB/ARG. For example, Wallace et al. (2018) noticed a significant reduction in sulfonamide resistance genes during mesophilic AD of cow manure, but no reduction effect on tetracycline resistance genes. Moreover, some other studies showed even an increase of the abundance of some ARG after mesophilic AD treatment such as sulfonamide, amphenicol and tetracycline resistance genes (Chen et al., 2010; Pu et al., 2018). Gros et al. (2019) observed that the mesophilic AD treatment, even though it was followed by solid–liquid separation, has reduced only modestly the abundance of ARG for quinolones, tetracyclines, macrolides, sulfonamide and β-lactams, as the copy numbers detected in the solid and liquid digestate fractions were similar to those quantified in slurry and sludge (Gros et al., 2019).
The different efficiency in removing ARG between mesophilic and thermophilic AD was linked to the microbial community composition of the sludge AD process (Agga et al., 2020). The microbial communities may decrease the antibiotic concentration during the mesophilic AD process, the subinhibitory antibiotic concentrations potentially acting as selection factors and promoting the propagation of ARB that could explain the increased abundance of ARG (Agga et al., 2020).
However, further studies for better understanding the ARG evolution under different setups are needed.
Although lab-scale AD has been demonstrated to reduce the abundance of ARG, there are only few data from commercial farms. The impact of on-farm AD on the decrease of enteric bacteria ARG, as well as of the frequency of horizontal transfer potential of ARG was evaluated in six commercial dairy farms in Ontario, Canada (Tran et al., 2021). Anaerobic digestion significantly decreased the viable coliform counts, the frequency of the horizontal transfer of ESBL genes as well as the abundance of sulphonamides, macrolides and beta-lactam resistance genes (Tran et al., 2021).
Composting techniques
Composting can reduce populations of ARB as well as ARG more effectively than other manure treatment processes, such as AD or simple manure stockpiling, but this depends on the type of manure and composting duration (Wang et al., 2015; Youngquist et al., 2016). In short-term cow manure stockpiles, the prevalence of antibiotic-resistant E. coli and Enterococcus spp. was not changed during 3 days (Walczak and Xu, 2011). In exchange, the composting of pig manure for 48 days led to a drastic decrease of ARB (Acinetobacter sp., Pseudomonas sp.) by 4–7 log units in cultivable erythromycin-resistant and tetracycline-resistant bacteria (especially for Acinetobacter sp. strains) and of associated ARG (Wang et al., 2015). Hartmann and colleagues detected ESBL producing E. coli that had an identical rep-PCR pattern in animal fecal samples, composted manure and the environment of farms including cultivated and pasture fertilized soils, suggesting that ARB may persist in finished composts and facilitate their dissemination in fertilized soils (Hartmann et al., 2012).
Composting of the solid fraction of swine manure resulted in a reduction of different ARG concentrations, i.e.: tet (Q), tet (W), tet (C), tet (G), tet (Z), tet (Y), tet (M), tet (W), tet (O), tet (T), erm (A), erm (C), erm (F), erm (T), erm (X), sul1, sul2, dfrA1, dfrA7, gyrA and parC (Selvam et al., 2012; Wang et al., 2012). The broiler chicken litter was evaluated before and after composting for the abundance of 10 gene targets associated with antibiotic resistance or horizontal gene transfer (qPCR) and the results were correlated with the composition of the bacterial communities (16S rRNA gene amplicon sequencing) and the abundance of viable enteric bacteria (viable plate count; Subirats et al., 2020). Composting significantly reduced the abundance of enteric bacteria, including those carrying antibiotic resistance in litter from broiler chickens fed both with antibiotic supplemented diet and with antibiotic-free diet; the absolute abundance of all of the target genes decreased after composting except sul1, intI1, incW and erm(F) that remained stable (Subirats et al., 2020).
However, composting could lead to an apparently limited decrease in different representative ARG. For example, it has been shown that the chicken litter from broilers fed with bacitracin methylene disalicylate supplemented diet had an increased abundance of some ARGs, maintained after composting (Subirats et al., 2020). Le Devendec et al. (2016) indicated that, even after 6 weeks of composting or storage, resistance plasmids could still be transferred, suggesting that, in these conditions, composting may be insufficient to completely eliminate the risk of spreading AMR through chicken manure. Other studies have also shown that many ARGs persist after composting, with over 50 of these ARGs detectable, for example, in finished cattle manure composts (Qian et al., 2016). Co-composting of pig and chicken manure did not reduce the diversity of ARG, and a total of 19 ARG subtypes and two transposon genes were still persistent (Gao et al., 2019).
Several studies have indicated that temperature is a critical factor to limit the proliferation and activity of ARB and ARG (He et al., 2014; Wang et al., 2017). It has been shown that thermophilic composting has a higher efficiency for ARG removal than mesophilic composting (Sun et al., 2015; Qian et al., 2016). Pu et al. observed a decline in ARB, ARG and transposons abundance after aerobic co-composting of pig and chicken manures. However, the diversity and abundance of ARG was increased at the temperature-decreasing stage (55°C–25°C, 14–20 days), compared with the temperature-increasing stage (55°C–60°C, 3–14 days). Three genes conferring resistance to amphenicol macrolide-lincosamide-streptogramin B and vancomycin were highly enriched (101-fold, 420-fold, and 250-fold) at temperature decreasing stage (Pu et al., 2019).
Conclusions and perspectives
The four major knowledge gaps related to the environmental dimensions of AMR proposed by Larsson et al. (2018), i.e., (Accinelli et al., 2007) the relative contributions of AMR from different sources (Agga et al., 2020) the role of the environment in the spread of AMR (Agga et al., 2022) the risk of human exposure to AMR in the environment, and (Alexy et al., 2004) the development of mitigation strategies, are all applicable to livestock production and manure as sources of antibiotic residues and AMR (Jutkina et al., 2018).
Fertilization with natural products is one of the main routes responsible for the introduction of antibiotic residues, ARG and zoonotic bacteria from animals to soil and, for the possible further dissemination into drinking water systems. Thus, proper handling, treatment and storage of manure prior to land application are key aspects to control the dissemination of AMR into the environment.
However, additional research is needed for understanding the fate of ARG during different types of manure treatment, such as anaerobic digestion and composting and to determine the optimal conditions for removal of antibiotic residues, ARB and ARG, allowing for the development of specific best practices for livestock manure treatment plants. It must also be determined if different manure treatments are really removing or just diluting resistance. Relevant criteria should be developed and/or modified according to research results for achieving a high-level efficiency in microorganisms removal during manure treatment and processing.
Another key intervention necessary to curb the further AMR emergence and spread and to maintain the efficiency of antibiotics is to limit their use, emphasizing the need for a prudent – cautious – use of antibiotics on farm animals to avoid unnecessary selective pressure (Hölzel et al., 2010). The proposed EU legislative framework, which will be enforced by 2022, bans the use of human reserve antibiotics in veterinary medicine and the use of unprescribed animal antimicrobials. Due to MDR organisms it is recommended that the “last resort” antibiotics and other clinically important antibiotic classes should be reserved for treatment of confirmed or suspected infections. An encouraging result of this measure is the decrease in the prevalence of colistin resistance genes in pig farm environments was reported in China after banning the use of colistin as an animal feed additive (Gao et al., 2019). Additionally, in order to safeguard the future use of antibiotics for treatment of bacterial infections, both in animals and humans, prudent use centered on correct diagnosis, correct choice and use of antimicrobials, along with appropriate susceptibility testing needs to be further strengthened (Silley and Stephan, 2017). On the other hand, it should be considered that antibiotic use in livestock is a prerequisite of animal welfare, consumer protection and cost efficiency of animal production. Further limiting the use of antibiotics on these farms might have effects on animal welfare and therefore might be difficult to implement (Wallace et al., 2018). However, a reduction of the consumption of quinolones and third-and fourth generation cephalosporins in veterinary medicine has been confirmed by the first joint report of the European Centre for Disease Prevention and Control (ECDC), the European Food Safety Authority (EFSA) (2018) and the European Medicines Agency (EMA) (2019) on antibiotics consumption and antibiotic resistance in humans and food-producing animals (Anon, 2015).
Further research should be also conducted to explore the potential dissemination routes of ARG among soil microorganisms and then to vegetables, to be able to take effective measures for controlling the persistence and dissemination of AMR in the vegetable production chain (Carballo et al., 2013). Additional research should follow to elucidate which microorganisms participate in the ARG transmission in a certain environment and quantify them.
The answers to these questions are pending on the characterization of land microbiome before and after manure application, assessing the risk of different raw and pre-treated manure types with regards to AR dissemination, as well as the maintenance and rate of pathogen transfer from grassland to animals. This is an essential component of agri-food research to fully assess the risk that manure land spreading has on the transfer of AR pathogens into the food chain and to humans.
Most imperative is that stakeholders help advise these research efforts so that scientific findings are more easily translated into practical on-farm management decisions and support to best equip the involved actors with the resources and tools needed to respond to the global AMR crisis.
Author contributions
MC and LM: conceptualization. LM, MP, MJ, NM, CP, and PS: writing—original draft preparation. GG, PS, HS, ET, SG, PB, FB, and PK: writing—review and editing. MC: supervision. AR: funding acquisition. All authors contributed to the article and approved the submitted version.
Funding
This research was funded by COFUND-JPI-EC-AMR-ARMIS, Antimicrobial Resistance Manure Intervention Strategies, grant number 40/2018; CNFIS-FDI-2022-0675, UEFISCDI - PN-III-P4-PCE2021-1797 and the Ministry of Research, Innovation and Digitalization through Program 1—Development of the national R&D system, Subprogram 1.2—Institutional performance—Financing projects for excellence in RDI, Contract no. 41 PFE/30.12.2021.
Conflict of interest
The authors declare that the research was conducted in the absence of any commercial or financial relationships that could be construed as a potential conflict of interest.
Publisher’s note
All claims expressed in this article are solely those of the authors and do not necessarily represent those of their affiliated organizations, or those of the publisher, the editors and the reviewers. Any product that may be evaluated in this article, or claim that may be made by its manufacturer, is not guaranteed or endorsed by the publisher.
References
Aarestrup, F. M. (2015). The livestock reservoir for antimicrobial resistance: a personal view on changing patterns of risks, effects of interventions and the way forward. Phil. Trans. R. Soc. 370:20140085. doi: 10.1098/rstb.2014.0085
Accinelli, C., Koskinen, W. C., Becker, J. M., and Sadowsky, M. J. (2007). Environmental fate of two sulfonamide antimicrobial agents in soil. J. Agric. Food Chem. 55, 2677–2682. doi: 10.1021/jf063709j
Agga, G. E., Couch, M., Parekh, R. R., Mahmoudi, F., Appala, K., Kasumba, J., et al. (2022). Lagoon, anaerobic digestion, and composting of animal manure treatments impact on tetracycline resistance genes. Antibiotics 11:391. doi: 10.3390/antibiotics11030391
Agga, G. E., Kasumba, J., Loughrin, J. H., and Conte, E. D. (2020). Anaerobic digestion of tetracycline spiked livestock manure and poultry litter increased the abundances of antibiotic and heavy metal resistance genes. Front. Microbiol. 11:614424. doi: 10.3389/fmicb.2020.614424
Alexy, R., Kümpel, T., and Kümmerer, K. (2004). Assessment of degradation of 18 antibiotics in the closed bottle test. Chemosphere 57, 505–512. doi: 10.1016/j.chemosphere.2004.06.024
Alvarez, J. A., Otero, L., Lema, J. M., and Omil, F. (2010). The effect and fate of antibiotics during the anaerobic digestion of pig manure. Bioresour. Technol. 101, 8581–8586. doi: 10.1016/j.biortech.2010.06.075
Amador, P., Fernandes, R., Prudêncio, C., and Duarte, I. (2019). Prevalence of antibiotic resistance genes in multidrug-resistant Enterobacteriaceae on Portuguese livestock manure. Antibiotics 8:23. doi: 10.3390/antibiotics8010023
Anon (2015). “Committee for medicinal products for veterinary use (CVMP),” in European Medicines Agency Veterinary Medicines and Inspections. Available at: http://www.ema.europa.eu/docs/en_GB/document_library/Scientific_guideline/2010/02/WC500070670.pdf
Arikan, O. A., Mulbry, W., and Rice, C. (2009). Management of antibiotic residues from agricultural sources: use of composting to reduce chlortetracycline residues in beef manure from treated animals. J. Hazard. Mater. 164, 483–489. doi: 10.1016/j.jhazmat.2008.08.019
Baquero, F., Martínez, J. L., and Cantón, R. (2008). Antibiotics and antibiotic resistance in water environments. Curr. Opin. Biotechnol. 19, 260–265. doi: 10.1016/j.copbio.2008.05.006
Bengtsson-Palme, J., Kristiansson, E., and Larsson, D. G. J. (2018). Environmental factors influencing the development and spread of antibiotic resistance. FEMS Microbiol. Rev. 42:fux053. doi: 10.1093/femsre/fux053
Berendsen, B. J., Wegh, R. S., Memelink, J., Zuidema, T., and Stolker, L. A. (2015). The analysis of animal faeces as a tool to monitor antibiotic usage. Talanta 132, 258–268. doi: 10.1016/j.talanta.2014.09.022
Boeckel, T. P. V., Glennon, E. E., Chen, D., Gilbert, M., Robinson, T. P., Grenfell, B. T., et al. (2017). Reducing antimicrobial use in food animals. Science 357, 1350–1352. doi: 10.1126/science.aao1495
Buta-Hubeny, M., Korzeniewska, E., Hubeny, J., Zieliński, W., Rolbiecki, D., Harnisz, M., et al. (2022). Structure of the manure resistome and the associated mobilome for assessing the risk of antimicrobial resistance transmission to crops. Sci. Total Environ. 808:152144. doi: 10.1016/j.scitotenv.2021.152144
Campagnolo, E. R., Johnson, K. R., Karpati, A., Rubin, C. S., Kolpin, D. W., Meyer, M. T., et al. (2002). Antimicrobial residues in animal waste and water resources proximal to large-scale swine and poultry feeding operations. Sci. Total Environ. 299, 89–95. doi: 10.1016/S0048-9697(02)00233-4
Cao, J., Yang, G., Mai, Q., Zhuang, Z., and Zhuang, L. (2020). Co-selection of antibiotic-resistant bacteria in a paddy soil exposed to As(III) contamination with an emphasis on potential pathogens. Sci. Total Environ. 725:138367. doi: 10.1016/j.scitotenv.2020.138367
Carballo, M., Aguayo, S., González, M., Esperon, F., and Torre, A. (2016). Environmental assessment of Tetracycline’s residues detected in pig slurry and poultry manure. J. Environ. Prot. 7, 82–92. doi: 10.4236/jep.2016.71008
Carballo, M., Esperón, F., Sacristán, C., González, M., Vázquez, B., Aguayo, S., et al. (2013). Occurrence of tetracycline residues and antimicrobial resistance in gram negative bacteria isolates from cattle farms in Spain. Adv. Biosci. Biotechnol. 4, 295–303. doi: 10.4236/abb.2013.42A040
Carlson, J. C., and Mabury, S. A. (2006). Dissipation kinetics and mobility of chlortetracycline, tylosin, and monensin in an agricultural soil in Northumberland County, Ontario, Canada. Environ. Toxicol. Chem. 25, 1–10. doi: 10.1897/04-657R.1
Ceccarelli, D., Hesp, A., van der Goot, J., Joosten, P., Sarrazin, S., Wagenaar, J. A., et al. (2020). Antimicrobial resistance prevalence in commensal Escherichia coli from broilers, fattening turkeys, fattening pigs and veal calves in European countries and association with antimicrobial usage at country level. J. Med. Microbiol. 69, 537–547. doi: 10.1099/jmm.0.001176
Chantziaras, I., Boyen, F., Callens, B., and Dewulf, J. (2013). Correlation between veterinary antimicrobial use and antimicrobial resistance in food-producing animals: a report on seven countries. J. Antimicrob. Chemother. 69, 827–834. doi: 10.1093/jac/dkt443
Chen, J., Michel, F. C., Sreevatsan, S., Morrison, M., and Yu, Z. (2010). Occurrence and persistence of erythromycin resistance genes (erm) and tetracycline resistance genes (tet) in waste treatment systems on swine. Farms. Microb. Ecol. 60, 479–486. doi: 10.1007/s00248-010-9634-5
Chen, Z., Zhang, W., Yang, L., Stedtfeld, R. D., Peng, A., Gu, C., et al. (2019). Antibiotic resistance genes and bacterial communities in cornfield and pasture soils receiving swine and dairy manures. Environ. Pollut. 248, 947–957. doi: 10.1016/j.envpol.2019.02.093
Cheng, W., Chen, H., Su, C., and Yan, S. (2013). Abundance and persistence of antibiotic resistance genes in livestock farms: a comprehensive investigation in eastern China. Environ. Int. 61, 1–7. doi: 10.1016/j.envint.2013.08.023
Cheng, D. M., Feng, Y. Y., Liu, Y. W., Xue, J. M., and Li, J. Z. (2019). Dynamics of oxytetracycline, sulfamerazine, and ciprofloxacin and related antibiotic resistance genes during swine manure composting. J. Environ. Manag. 230, 102–109. doi: 10.1016/j.jenvman.2018.09.074
Collignon, P. J., and McEwen, S. A. (2019). One health-its importance in helping to better control antimicrobial resistance. Trop. Med. Infect. Dis. 4:22. doi: 10.3390/tropicalmed4010022
Couce, A., and Blázquez, J. (2009). Side effects of antibiotics on genetic variability. FEMS Microbiol. Rev. 33, 531–538. doi: 10.1111/j.1574-6976.2009.00165.x
Dandachi, I., Sokhn, E. S., Dahdouh, E. A., Azar, E., El-Bazzal, B., Rolain, J. M., et al. (2018). Prevalence and characterization of multi-drug-resistant gram-negative bacilli isolated from Lebanese poultry: A nationwide study. Front Microbiol. 9:550. doi: 10.3389/fmicb.2018.00550
De Liguoro, M., Cibin, V., Capolongo, F., Halling-Sørensen, B., and Montesissa, C. (2003). Use of oxytetracycline and tylosin in intensive calf farming: evaluation of transfer to manure and soil. Chemosphere 52, 203–212. doi: 10.1016/S0045-6535(03)00284-4
Doi, Y. (2019). Treatment options for carbapenem-resistant gram-negative bacterial infections. Clin. Infect. Dis. 69, S565–S575. doi: 10.1093/cid/ciz830
Dolliver, H., Gupta, S., and Noll, S. (2008). Antibiotic degradation during manure composting. J. Environ. Qual. 37, 1245–1253. doi: 10.2134/jeq2007.0399
Dungan, R. S., McKinney, C. W., and Leytem, A. B. (2018). Tracking antibiotic resistance genes in soil irrigated with dairy wastewater. Sci. Total Environ. 635, 1477–1148. doi: 10.1016/j.scitotenv.2018.04.020
Durso, L. M., and Cook, K. L. (2019). One health and antibiotic resistance in Agroecosystems. EcoHealth 16, 414–419. doi: 10.1007/s10393-018-1324-7
Duygan, B. O., Gaille, C., Fenner, K., and van der Meer, J. (2021). Assessing antibiotics biodegradation and effects at sub-inhibitory concentrations by quantitative microbial community Deconvolution. Front. Environ. Sci. 9:737247. doi: 10.3389/fenvs.2021.737247
Elliott, K. A., Kenny, C., and Madan, J. (2017). “A global treaty to reduce antimicrobial use in livestock,” in CGD Policy Paper (Washington, DC: Center for Global Development).
European Food Safety Authority (EFSA) (2018). The European Union summary report on antimicrobial resistance in zoonotic and indicator bacteria from humans, animals and food in 2016. Contract No.: 5182.
European Medicines Agency (EMA) (2019). Analysis of antimicrobial consumption and resistance (‘JIACRA’ reports). Available at: https://www.ema.europa.eu/en/veterinary-regulatory/overview/antimicrobial-resistance/analysis-antimicrobial-consumption-resistance-jiacra-reports#report-on-2013%E2%80%9315-(jiacra-ii)-section
Fahrenfeld, N., Knowlton, K., Krometis, L. A., Hession, W. C., Xia, K., Lipscomb, E., et al. (2014). Effect of manure application on abundance of antibiotic resistance genes and their attenuation rates in soil: field-scale mass balance approach. Environ. Sci. Technol. 48, 2643–2650. doi: 10.1021/es404988k
Fang, H., Wang, H. F., Cai, L., and Yu, Y. (2015). Prevalence of antibiotic resistance genes and bacterial pathogens in long-term manured greenhouse soils as revealed by metagenomic survey. Environ. Sci. Technol. 49, 1095–1104. doi: 10.1021/es504157v
FAO and WHO (2019). Joint FAO/WHO Expert Meeting in collaboration with OIE on Foodborne Antimicrobial Resistance: Role of the Environment, Crops and Biocides – Meeting report. Microbiological Risk Assessment Series no. 34. Rome. Available at: http://www.fao.org/3/ca6724en/ca6724en.pdf
FDA (2019). Summary Report on Antimicrobials Sold or Distributed for Use in Food-Producing Animals. Available at: https://www.fda.gov/animal-veterinary/cvm-updates/fda-releases-annual-summary-report-antimicrobials-sold-or-distributed-2018-use-food-producing
FDA (2020). Summary Report on Antimicrobials Sold or Distributed for Use in Food-Producing Animals. Available at: https://www.fda.gov/media/144427/download
Feßler, A. T., and Schwarz, S. (2017). Antimicrobial resistance in Corynebacterium spp., Arcanobacterium spp., and Trueperella pyogenes. Microbiol. Spectr. 5. doi: 10.1128/microbiolspec.ARBA-0021-2017
Friese, A., Schulz, J., Laube, H., von Salviati, C., Hartung, J., and Roesler, U. (2013). Faecal occurrence and emissions of livestock-associated methicillin-resistant Staphylococcus aureus (laMRSA) and ESBL/AmpC-producing E. coli from animal farms in Germany. Berl. Munch. Tierarztl. Wochenschr. 126, 175–180.
Furtula, V., Farrell, E., Diarrassouba, F., Rempel, H., Pritchard, J., and Diarra, M. (2010). Veterinary pharmaceuticals and antibiotic resistance of Escherichia coli isolates in poultry litter from commercial farms and controlled feeding trials. Poult. Sci. 89, 180–188. doi: 10.3382/ps.2009-00198
Gao, Y., Lu, C., Shen, D., Liu, J., Ma, Z., Yang, B., et al. (2019). Elimination of the risks of colistin resistance gene (mcr-1) in livestock manure during composting. Environ. Int. 126, 61–68. doi: 10.1016/j.envint.2019.02.015
Gou, M., Hu, H.-W., Zhang, Y.-J., Wang, J.-T., Hayden, H., Tang, Y.-Q., et al. (2018). Aerobic composting reduces antibiotic resistance genes in cattle manure and the resistome dissemination in agricultural soils. Sci. Total Environ. 612, 1300–1310. doi: 10.1016/j.scitotenv.2017.09.028
Graham, J. P., Evans, S. L., Price, L. B., and Silbergeld, E. K. (2009). Fate of antimicrobial-resistant enterococci and staphylococci and resistance determinants in stored poultry litter. Environ. Res. 109, 682–689. doi: 10.1016/j.envres.2009.05.005
Graham, D. W., Knapp, C. W., Christensen, B. T., McCluskey, S., and Dolfing, J. (2016). Appearance of β-lactam resistance genes in agricultural soils and clinical isolates over the 20th century. Sci. Rep. 6:21550. doi: 10.1038/srep21550
Grave, K., Torren-Edo, J. A. J., and Mackay, D. (2010). Comparison of the sales of veterinary antimicrobial agents between 10 European countries. J. Antimicrob. Chemother. 65, 2037–2040. doi: 10.1093/jac/dkq247
Gros, M., Marti, E., Balcázar, J. L., Boy-Roura, M., Busquets, A., Colón, J., et al. (2019). Fate of pharmaceuticals and antibiotic resistance genes in a full-scale on-farm livestock waste treatment plant. J. Hazard. Mater. 378:120716. doi: 10.1016/j.jhazmat.2019.05.109
Haller, M. Y., Muller, S. R., Mc Ardell, C. S., Alder, A. C., and Suter, M. J.-F. (2002). Quantification of veterinary antibiotics (sulfonamides and trimethoprim) in animal manure by liquid chromatography–mass spectrometry. J. Chromat. A 952, 111–120. doi: 10.1016/S0021-9673(02)00083-3
Halling-Sørensen, B., Jacobsen, A. M., Jensen, J., Sengelov, G., Vaclavik, E., and Ingerslev, F. (2005). Dissipation and effects of chlortetracycline and tylosin in two agricultural soils: A field-scale study in southern Denmark. Environ. Toxicol. Chem. 24, 802–810. doi: 10.1897/03-576.1
Hamscher, G., Pawelzick, H., Höper, H., and Nau, H. (2005). Different behavior of Tetracyclines and sulfonamides in Sandy soils after repeated fertilization with liquid manure. Environ. Toxicol. Chem./SETAC 24, 861–868. doi: 10.1897/04-182R.1
Han, X.-M., Hu, H. W., Chen, Q. L., Yang, L.-Y., Li, H. L., Zhu, Y.-G., et al. (2018). Antibiotic resistance genes and associated bacterial communities in agricultural soils amended with different sources of animal manures. Soil Biol. Biochem. 126, 91–102. doi: 10.1016/j.soilbio.2018.08.018
Harms, K., and Bauer, J. (2012). “Detection and occurrence of antibiotics and their metabolites in pig manure in Bavaria (Germany),” in Antimicrobial Resistance in the Environment. eds. P. L. Keen and M. H. M. M. Montforts (New Jersey: John Wiley & Sons, Inc.), 293–307.
Hartmann, A., Locatelli, A., Amoureux, L., Depret, G., Jolivet, C., Gueneau, E., et al. (2012). Occurrence of CTX-M producing Escherichia coli in soils, cattle, and farm environment in France (Burgundy region). Front. Microbiol. 3:83. doi: 10.3389/fmicb.2012.00083
Hassell, J. M., Ward, M. J., Muloi, D., Bettridge, J. M., Robinson, T. P., Kariuki, S., et al. (2019)., et al.Clinically relevant antimicrobial resistance at the wildlife-livestock-human interface in Nairobi: an epidemiological study. Lancet Planet Health. 3, e259–e269.
Hastings, P. J., Rosenberg, S. M., and Slack, A. (2004). Antibiotic-induced lateral transfer of antibiotic resistance. Trends Microbiol. 12, 401–404. doi: 10.1016/j.tim.2004.07.003
He, L. Y., Liu, Y. S., Su, H. C., Zhao, J. L., Liu, S. S., Chen, J., et al. (2014). Dissemination of antibiotic resistance genes in representative broiler feedlots environments: identification of indicator ARGs and correlations with environmental variables. Environ. Sci. Technol. 48, 13120–13129. doi: 10.1021/es5041267
Heuer, H., Solehati, Q., Zimmerling, U., Kleineidam, K., Schloter, M., Muller, T., et al. (2011). Accumulation of sulfonamide resistance genes in arable soils due to repeated application of manure containing sulfadiazine. Appl. Environ. Microbiol. 77, 2527–2530. doi: 10.1128/AEM.02577-10
Hoeksma, P., Aarnink, A. J. A., de Buisonjé, F. E., En Rutjes, S. A., and Blaak, H. (2015). Effect van processtappen op overleving van micro-organismen bij mestverwerking. Wageningen Livestock Research, Rapport. 893:blz. 29.
Hoeksma, P., de Koeijer, T., Wipfler, E. L., Aarnink, A. J. A., Blokland, P. W., Rakonjac, N., et al. (2020). Milieurisico van Antibiotica in Mest voor Bodem en Grondwater Mogelijk Beperkt. Vereniging van milieuprofessionals, Tijdschrift Milieu, 46–52.
Hoeksma, P., Schmitt, H., de Buisonjé, F., Pishgar Komleh, H., and Ehlert, P., (2021). Composition of Mineral Concentrates. Results of monitoring installations of the pilot mineral concentrate. Wageningen Livestock Research, Report 1295.
Hölzel, C. S., Schwaiger, K., Harms, K., Küchenhoff, H., Kunz, A., Meyer, K., et al. (2010). Sewage sludge and liquid pig manure as possible sources of antibiotic resistant bacteria. Environ. Res. 110, 318–326. doi: 10.1016/j.envres.2010.02.009
Hou, Y., Velthof, G. L., Lesschen, J. P., Staritsky, I. G., and Oenema, O. (2017). Nutrient recovery and emissions of ammonia, nitrous oxide, and methane from animal manure in Europe: effects of manure treatment technologies. Environ. Sci. Technol. 51, 375–383. doi: 10.1021/acs.est.6b04524
Hu, H. W., Han, X. M., Shi, X. Z., Wang, J. T., Han, L. L., Chen, D., et al. (2016). Temporal changes of antibiotic-resistance genes and bacterial communities in two contrasting soils treated with cattle manure. FEMS Microbiol. Ecol. 92:fiv169. doi: 10.1093/femsec/fiv169
Hu, Z., Liu, Y., Chen, G., Gui, X., Chen, T., and Zhan, X. (2011). Characterization of organic matter degradation during composting of manure-straw mixtures spiked with tetracyclines. Bioresour. Technol. 102, 7329–7334. doi: 10.1016/j.biortech.2011.05.003
Hu, Y., Yang, X., Li, J., Lv, N., Liu, F., Wu, J., et al. (2016). The bacterial mobile resistome transfer network connecting the animal and human microbiomes. Appl. Environ. Microbiol. 82, 6672–6681. doi: 10.1128/AEM.01802-16
Huang, X., Zheng, J., Tian, S., Liu, C., Liu, L., Wei, L., et al. (2019). Higher temperatures do not always achieve better antibiotic resistance gene removal in anaerobic digestion of swine manure. Appl. Environ. Microbiol. 85:e02878–18. doi: 10.1128/AEM.02878-18
Huijbers, P. M., Blaak, H., de Jong, M. C., Graat, E. A., Vandenbroucke-Grauls, C. M., and de Roda Husman, A. M. (2015). Role of the environment in the transmission of antimicrobial resistance to humans: a review. Environ. Sci. Technol. 49, 11993–12004. doi: 10.1021/acs.est.5b02566
Huijbers, P. M. C., Flach, C. F., and Larsson Joakim, D. G. (2019). A conceptual framework for the environmental surveillance of antibiotics and antibiotic resistance. Environ. Int. 130:104880. doi: 10.1016/j.envint.2019.05.074
Huygens, J., Daeseleire, E., Mahillon, J., Van Elst, D., Decrop, J., Meirlaen, J., et al. (2021). Presence of antibiotic residues and antibiotic resistant bacteria in cattle manure intended for fertilization of agricultural fields: a one health perspective. Antibiotics 10:410. doi: 10.3390/antibiotics10040410
Jauregi, L., Epelde, L., Alkorta, I., and Garbisu, C. (2021). Antibiotic resistance in agricultural soil and crops associated to the application of cow manure-derived amendments From conventional and organic livestock farms. Front. Vet. 8:633858. doi: 10.3389/fvets.2021.633858
Jutkina, J., Marathe, N. P., Flach, C. F., and Larsson, D. G. J. (2018). Antibiotics and common antibacterial biocides stimulate horizontal transfer of resistance at low concentrations. Sci. Total Environ. 616–617, 172–178. doi: 10.1016/j.scitotenv.2017.10.312
Jutkina, J., Rutgersson, C., Flach, C. F., and Joakim Larsson, D. G. (2016). An assay for determining minimal concentrations of antibiotics that drive horizontal transfer of resistance. Sci. Total Environ. 548–549, 131–138. doi: 10.1016/j.scitotenv.2016.01.044
Kasumba, J., Appala, K., Agga, G. E., Loughrin, J. H., and Conte, E. D. (2020). Anaerobic digestion of livestock and poultry manures spiked with tetracycline antibiotics. J. Environ. Sci. Health B 55, 135–147. doi: 10.1080/03601234.2019.1667190
Kemper, N. (2008). Veterinary antibiotics in the aquatic and terrestrial environment. Ecol. Indic. 8, 1–13. doi: 10.1016/j.ecolind.2007.06.002
Kim, K. R., Owens, G., Ok, Y. S., Park, W. K., Lee, D. B., and Kwon, S. I. (2012). Decline in extractable antibiotics in manure-based composts during composting. Waste Manag. 32, 110–116. doi: 10.1016/j.wasman.2011.07.026
Kyselková, M., Jirout, J., Chroňáková, A., Vrchotová, N., Bradley, R., Schmitt, H., et al. (2013). Cow excrements enhance the occurrence of tetracycline resistance genes in soil regardless of their oxytetracycline content. Chemosphere 93, 2413–2418. doi: 10.1016/j.chemosphere.2013.08.058
Kyselková, M., Jirout, J., Vrchotová, N., Schmitt, H., and Elhottová, D. (2015). Spread of tetracycline resistance genes at a conventional dairy farm. Front. Microbiol. 6:536. doi: 10.3389/fmicb.2015.00536
Laconi, A., Mughini-Gras, L., Tolosi, R., Grilli, G., Trocino, A., Carraro, L., et al. (2021). Microbial community composition and antimicrobial resistance in agricultural soils fertilized with livestock manure from conventional farming in northern Italy. Sci. Total Environ. 760:143404. doi: 10.1016/j.scitotenv.2020.143404
Landers, T. F., Cohen, B., Wittum, T. E., and Larson, E. L. (2012). A review of antibiotic use in food animals: perspective, policy, and potential. Public Health Rep. 127, 4–22. doi: 10.1177/003335491212700103
Larsson, G. G. J., Andremont, A., Bengtsson-Palme, J., Brandt, K. K., Roda Husman, A. M., Fagerstedt, P., et al. (2018). Critical knowledge gaps and research needs related to the environmental dimensions of antibiotic resistance. Environ. Int. 117, 132–138. doi: 10.1016/j.envint.2018.04.041
Le Devendec, L., Mourand, G., Bougeard, S., Léaustic, J., Jouy, E., Keita, A., et al. (2016). Impact of colistin sulfate treatment of broilers on the presence of resistant bacteria and resistance genes in stored or composted manure. Vet. Microbiol. 194, 98–106. doi: 10.1016/j.vetmic.2015.11.012
Leal, R. M. P., Figueira, R. F., Tornisielo, V. L., and Regitano, J. B. (2012). Occurrence and sorption of fluoroquinolones in poultry litters and soils from São Paulo State, Brazil. Sci Total Environ. 432, 344–349. doi: 10.1016/j.scitotenv.2012.06.002
Lee, C., Jeong, S., Ju, M., and Kim, J. Y. (2020). Fate of chlortetracycline antibiotics during anaerobic degradation of cattle manure. J. Hazard. Mater. 386:121894. doi: 10.1016/j.jhazmat.2019.121894
Li, B., Yang, Y., Ma, L., Ju, F., Guo, F., Tiedje, J. M., et al. (2015). Metagenomic and network analysis reveal wide distribution and co-occurrence of environmental antibiotic resistance genes. ISME J. 9, 2490–2502. doi: 10.1038/ismej.2015.59
Li, Y. X., Zhang, X. L., Li, W., Lu, X. F., Liu, B., and Wang, J. (2013). The residues and environmental risks of multiple veterinary antibiotics in animal faeces. Environ. Monit. Assess. 185, 2211–2220. doi: 10.1007/s10661-012-2702-1
LIFE+MANEV (2015). Evaluation of manure management and treatment technology for environmental protection and sustainable livestock farming in Europe (LIFE09 ENV/ES/000453).
Lima, T., Domingues, S., and Da Silva, G. J. (2020). Manure as a potential hotspot for antibiotic resistance dissemination by horizontal gene transfer events. Vet Sci. 7:110. doi: 10.3390/vetsci7030110
Liu, G., Bogaj, K., Bortolaia, V., Olsen, J. E., and Thomsen, L. E. (2019). Antibiotic-induced, increased conjugative transfer is common to diverse naturally occurring ESBL plasmids in Escherichia coli. Front. Microbiol. 10:2119. doi: 10.3389/fmicb.2019.02119
Liu, Y. W., Feng, Y., Cheng, D. M., Xue, J. M., Wakelin, S., and Li, Z. J. (2018). Dynamics of bacterial composition and the fate of antibiotic resistance genes and mobile genetic elements during the co-composting with gentamicin fermentation residue and lovastatin fermentation residue. Bioresour. Technol. 261, 249–256. doi: 10.1016/j.biortech.2018.04.008
Liu, C., Tan, L., Zhang, L., Tian, W., and Ma, L. (2021). A review of the distribution of antibiotics in water in different regions of China and current antibiotic degradation pathways. Front. Environ. Sci. 9:692298. doi: 10.3389/fenvs.2021.692298
Looft, T., Johnson, T. A., Allen, H. K., Bayles, D. O., Alt, D. P., Stedtfeld, R. D., et al. (2011). In-feed antibiotic effects on the swine intestinal microbiome. PNAS 109, 1691–1696. doi: 10.1073/pnas.1120238109
Lopatkin, A. J., Sysoeva, T. A., and You, L. (2016). Dissecting the effects of antibiotics on horizontal gene transfer: analysis suggests a critical role of selection dynamics. BioEssays 38, 1283–1292. doi: 10.1002/bies.201600133
Lopatto, E., Choi, J., Colina, A., Ma, L., Howe, A., and Hinsa-Leasure, S. (2019). Characterizing the soil microbiome and quantifying antibiotic resistance gene dynamics in agricultural soil following swine CAFO manure application. PLoS One 14:e0220770. doi: 10.1371/journal.pone.0220770
Lyngsø, F. H., Flotats, X., Blasi, A. B., Palatsi, J., Magri, A., and Schelde, K. M. (2011). Inventory of manure processing activities in Europe. Technical Report N° I Concerning “Manure Processing Activities in Europe” to the European Commission, Directorate-General Environment. Project reference: ENV.B.1/ETU/2010/0007.
MARAN (2018). Monitoring of Antimicrobial Resistance and Antibiotic Usage in Animals in the Netherlands. Available at: https://www.wur.nl/en/Research-Results/Research-Institutes/Bioveterinary-Research/In-the-spotlight/Antibiotic-resistance/MARAN-reports.htm
Marti, E., Jofre, J., and Balcazar, J. L. (2013). Prevalence of antibiotic resistance genes and bacterial community composition in a river influenced by a wastewater treatment plant. PLoS One 8:e78906. doi: 10.1371/journal.pone.0078906
Marti, R., Tien, Y. C., Murray, R., Scott, A., Sabourin, L., and Topp, E. (2014). Safely coupling livestock and crop production systems: how rapidly do antibiotic resistance genes dissipate in soil following a commercial application of swine or dairy manure? Appl. Environ. Microb. 80, 3258–3265. doi: 10.1128/AEM.00231-14
Martínez-Carballo, E., González-Barreiro, C., Scharf, S., and Gans, O. (2007). Environmental monitoring study of selected veterinary antibiotics in animal manure and soils in Austria. Environ. Pollut. 148, 570–579. doi: 10.1016/j.envpol.2006.11.035
Massé, D. I., Saady, N. M., and Gilbert, Y. (2014). Potential of biological processes to eliminate antibiotics in livestock manure: an overview. Animals (Basel). 4, 146–163. doi: 10.3390/ani4020146
Melse, W., and de Buisonjé, F. E. (2020). Manure Treatment and Utilization Options. Netherlands: Wageningen Livestock Research.
Miguela-Villoldo, P., Moreno, M. A., Rodríguez-Lázaro, D., Gallardo, A., Hernández, M., Serrano, T., et al. (2022). Longitudinal study of the mcr-1 gene prevalence in Spanish food-producing pigs from 1998 to 2021 and its relationship with the use of polymyxins. Porc. Health Manag. 8:12. doi: 10.1186/s40813-022-00255-0
Munir, M., and Xagoraraki, I. (2011). Levels of antibiotic resistance genes in manure, biosolids, and fertilized soil. J. Environ. Qual. 40, 248–255. doi: 10.2134/jeq2010.0209
Nguyen, B. T., Chen, Q. L., He, J. Z., and Hu, H. W. (2020). Microbial regulation of natural antibiotic resistance: understanding the protist-bacteria interactions for evolution of soil resistome. Sci. Total Environ. 705:135882. doi: 10.1016/j.scitotenv.2019.135882
Nõlvak, H., Truu, M., Kanger, K., Tampere, M., Espenberg, M., Loit, E., et al. (2016). Inorganic and organic fertilizers impact the abundance and proportion of antibiotic resistance and integron-integrase genes in agricultural grassland soil. Sci. Total Environ. 562, 678–689. doi: 10.1016/j.scitotenv.2016.04.035
OIE (2018). OIE Annual Report on Antimicrobial Agents Intended for Use in Animals – Better Understanding of the Global Situation. Third Report. Available at: https://rr-africa.oie.int/wp-content/uploads/2019/09/annual_report_amr_3.pdf
Oliver, J. P., Gooch, C. A., Lansing, S., Schueler, J., Hurst, J. J., Sassoubre, L., et al. (2020). Invited review: fate of antibiotic residues, antibiotic-resistant bacteria, and antibiotic resistance genes in US dairy manure management systems. J. Dairy Sci. 103, 1051–1071. doi: 10.3168/jds.2019-16778
Osman, A. A., Sikora, L. J., Mulbry, W., Khan, S. U., Rice, C., and Foster, G. D. (2006). The fate and effect of oxytetracycline during the anaerobic digestion of manure from therapeutically treated calves. J. Dairy Sci. 41, 1637–1643. doi: 10.1016/j.procbio.2006.03.010
Pal, C., Bengtsson-Palme, J., Kristiansson, E., and Larsson, D. G. J. (2016). The structure and diversity of human, animal and environmental resistomes. Microbiome 4:54. doi: 10.1186/s40168-016-0199-5
Pérez-Valera, E., Kyselková, M., Ahmed, E., Sladecek, F. X. J., Goberna, M., and Elhottová, D. (2019). Native soil microorganisms hinder the soil enrichment with antibiotic resistance genes following manure applications. Sci. Rep. 9:6760. doi: 10.1038/s41598-019-42734-5
Pikkemaat, M. G., Yassin, H., van der Fels-Klerx, H. J., and Berendsen, B. J. A. (2016). Antibiotic Residues and Resistance in the Environment Wageningen. RIKILT Wageningen UR (University & Research centre), RIKILT report 2016.009.
Popa, L. I., Czobor Barbu, I., and Chifiriuc, M. C. (2018). Mobile genetic elements involved in the horizontal transfer of antibiotic resistance genes. ROAMI 77, 263–276.
Pornsukarom, S., and Thakur, S. (2016). Assessing the impact of manure application in commercial swine farms on the transmission of antimicrobial resistant salmonella in the environment. PLoS One 11:e0164621. doi: 10.1371/journal.pone.0164621
Pruden, A., Larsson, D., Amézquita, A., Collignon, P., Brandt, K. K., Graham, D. W., et al. (2013). Management options for reducing the release of antibiotics and antibiotic resistance genes to the environment. Environ. Health Perspect. 121, 878–885. doi: 10.1289/ehp.1206446
Pu, C., Liu, H., Ding, G., Sun, Y., Yu, X., Chen, J., et al. (2018). Impact of direct application of biogas slurry and residue in fields: in situ analysis of antibiotic resistance genes from pig manure to fields. J. Hazard. Mater. 344, 441–449. doi: 10.1016/j.jhazmat.2017.10.031
Pu, C., Yu, Y., Diao, J., Gong, X., Li, J., and Sun, Y. (2019). Exploring the persistence and spreading of antibiotic resistance from manure to biocompost, soils and vegetables. Sci. Total Environ. 688, 262–269. doi: 10.1016/j.scitotenv.2019.06.081
Qian, X., Sun, W., Gu, J., Wang, X. J., Zhang, Y. J., Duan, M. L., et al. (2016). Reducing antibiotic resistance genes, integrons, and pathogens in dairy manure by continuous thermophilic composting. Bioresour. Technol. 220, 425–432. doi: 10.1016/j.biortech.2016.08.101
Rahman, M. M., Shan, J., Yang, P., Shang, X., Xia, Y., and Yan, X. (2018). Effects of long-term pig manure application on antibiotics, abundance of antibiotic resistance genes (ARGs), anammox and denitrification rates in paddy soils. Environ. Pollut. 240, 368–377. doi: 10.1016/j.envpol.2018.04.135
Rasschaert, G., Elst, D. V., Colson, L., Herman, L., Ferreira, H. C. C., Dewulf, J., et al. (2020). Antibiotic residues and antibiotic-resistant bacteria in pig slurry used to fertilize agricultural fields. Antibiotics 9:34. doi: 10.3390/antibiotics9010034
Ray, P., Chen, C., Knowlton, K. F., Pruden, A., and Xia, K. (2017). Fate and effect of antibiotics in beef and dairy manure during static and turned composting. J. Environ. Qual. 46, 45–54. doi: 10.2134/jeq2016.07.0269
Ross, J., and Topp, E. (2015). Abundance of antibiotic resistance genes in bacteriophage following soil fertilization with dairy manure or municipal biosolids, and evidence for potential transduction. Appl. Environ. Microbiol. 81, 7905–7913. doi: 10.1128/AEM.02363-15
Ruuskanen, M., Muurinen, J., Meierjohan, A., Pärnänen, K., Tamminen, M., Lyra, C., et al. (2016). Fertilizing with animal manure disseminates antibiotic resistance genes to the farm environment. J. Environ. Qual. 45, 488–493. doi: 10.2134/jeq2015.05.0250
Sandberg, K. D., and LaPara, T. M. (2016). The fate of antibiotic resistance genes and class 1 integrons following the application of swine and dairy manure to soils. FEMS Microbiol. Ecol. 10:fiw001. doi: 10.1093/femsec/fiw001
Schauss, T., Glaeser, S. P., Gütschow, A., Dott, W., and Kämpfer, P. (2015). Improved detection of extended spectrum beta-lactamase (ESBL)-producing Escherichia coli in input and output samples of German biogas plants by a selective pre-enrichment procedure. PLoS One 10:e0119791. doi: 10.1371/journal.pone.0119791
Schmitt, H. K., Stoob, G., Hamscher, E. S., and Seinen, W. (2006). Tetracyclines and tetracycline resistance in agricultural soils: microcosm and field studies. Microb. Ecol. 51, 267–276. doi: 10.1007/s00248-006-9035-y
Scott, H. M., Acuff, G., Bergeron, G., Bourassa, M. W., Gill, J., Graham, D. W., et al. (2019). Critically important antibiotics: criteria and approaches for measuring and reducing their use in food animal agriculture. Ann. N. Y. Acad. Sci. 1441, 8–16. doi: 10.1111/nyas.14058
Selvam, A., Xu, D., Zhao, Z., and Wong, J. W. C. (2012). Fate of tetracycline, sulfonamide and fluoroquinolone resistance genes and the changes in bacterial diversity during composting of swine manure. Bioresour. Technol. 126, 383–390. doi: 10.1016/j.biortech.2012.03.045
Shober, A. M., and Maguire, R. O. (2018). Manure Management. Reference Module in Earth Systems and Environmental Sciences. Elsevier.
Silley, P., and Stephan, B. (2017). Prudent use and regulatory guidelines for veterinary antibiotics-politics or science? J. Appl. Microbiol. 123, 1373–1380. doi: 10.1111/jam.13553
Spielmeyer, A., Ahlborn, J., and Hamscher, G. (2014). Simultaneous determination of 14 sulfonamides and tetracyclines in biogas plants by liquid-liquid-extraction and liquid chromatography tandem mass spectrometry. Anal. Bioanal. Chem. 406, 2513–2524. doi: 10.1007/s00216-014-7649-3
Spielmeyer, A., Breier, B., Groißmeier, K., and Hamscher, G. (2015). Elimination patterns of worldwide used sulfonamides and tetracyclines during anaerobic fermentation. Bioresour. Technol. 193, 307–314. doi: 10.1016/j.biortech.2015.06.081
Spielmeyer, A., Höper, H., and Hamscher, G. (2017). Long-term monitoring of sulfonamide leaching from manure amended soil into groundwater. Chemosphere 177, 232–238. doi: 10.1016/j.chemosphere.2017.03.020
Storteboom, H., Arabi, M., Davis, J. G., Crimi, B., and Pruden, A. (2010). Tracking antibiotic resistance genes in the South Platte river basin using molecular signatures of urban, agricultural, and pristine sources. Environ. Sci. Technol. 44, 7397–7404. doi: 10.1021/es101657s
Storteboom, H. N., Kim, S. C., Doesken, K. C., Carlson, K. H., Davis, J. G., and Pruden, A. (2007). Response of antibiotics and resistance genes to high-intensity and low-intensity manure management. J. Environ. Qual. 36, 1695–1703. doi: 10.2134/jeq2007.0006
Subirats, J., Murray, R., Scott, A., Lau, C. H., and Topp, E. (2020). Composting of chicken litter from commercial broiler farms reduces the abundance of viable enteric bacteria, Firmicutes, and selected antibiotic resistance genes. Sci. Total Environ. 746:141113. doi: 10.1016/j.scitotenv.2020.141113
Sun, W., Qian, X., Gu, J., Wang, X. J., and Duan, M. L. (2016). Mechanism and effect of temperature on variations in antibiotic resistance genes during anaerobic digestion of dairy manure. Sci. Rep. 6:30237. doi: 10.1038/srep30237
Sun, M., Ye, M., Wu, J., Feng, Y., Wan, J., Tian, D., et al. (2015). Positive relationship detected between soil bioaccessible organic pollutants and antibiotic resistance genes at dairy farms in Nanjing. Eastern China. Environ Pollut. 206, 421–428. doi: 10.1016/j.envpol.2015.07.022
Sura, S., Degenhardt, D., Cessna, A. J., Larney, F. J., Olson, A. F., and McAllister, T. A. (2014). Dissipation of three veterinary antimicrobials in beef cattle feedlot manure stockpiled over winter. J. Environ. Qual. 43, 1061–1070. doi: 10.2134/jeq2013.11.0455
Tiseo, K., Huber, L., Gilbert, M., Robinson, T. P., and Van Boeckel, T. P. (2020). Global trends in antimicrobial use in food animals from 2017 to 2030. Antibiotics (Basel) 9:918. doi: 10.3390/antibiotics9120918
Topp, E., Chapman, R., Devers-Lamrani, M., Hartmann, A., Marti, R., Martin-Laurent, F., et al. (2013). Accelerated biodegradation of veterinary antibiotics in agricultural soil following long-term exposure, and isolation of a Sulfamethazine-degrading sp. J. Environ. Qual. 42, 173–178. doi: 10.2134/jeq2012.0162
Topp, E., Larsson, D. G. J., Miller, D. N., Van den Eede, C., and Virta, M. P. J. (2018). Antimicrobial resistance and the environment: assessment, advances, gaps and recommendations for agriculture, aquaculture and pharmaceutical manufacturing. FEMS Microbiol. Ecol. 94:185. doi: 10.1093/femsec/fix185
Tran, T. T., Scott, A., Tien, Y. C., Murray, R., Boerlin, P., Pearl, D. L., et al. (2021). On-farm anaerobic digestion of dairy manure reduces the abundance of antibiotic resistance-associated gene targets, and the potential for plasmid transfer. Appl. Environ. Microbiol. 87:e0298020. doi: 10.1128/AEM.02980-20
Tyrrell, C., Burgess, C. M., Brennan, F. P., and Walsh, F. (2019). Antibiotic resistance in grass and soil. Biochem. Soc. Trans. 47, 477–486. doi: 10.1042/BST20180552
Tzavaras, I., Siarkou, V. I., Zdragas, A., Kotzamanidis, C., Vafeas, G., Bourtzi-Hatzopoulou, E., et al. (2012). Diversity of vanA-type vancomycin-resistant enterococcus faecium isolated from broilers, poultry slaughterers and hospitalized humans in Greece. J. Antimicrob. Chemother. 67, 1811–1818. doi: 10.1093/jac/dks166
Udikovic-Kolic, N., Wichmann, F., Broderick, N. A., and Handelsman, J. (2014). Bloom of resident antibiotic-resistant bacteria in soil following manure fertilization. PNAS 111, 15202–15207. doi: 10.1073/pnas.1409836111
Van den Meersche, T., Rasschaert, G., Haesebrouck, F., Coillie, E. V., Herman, L., Van Weyenberg, S., et al. (2019). Presence and fate of antibiotic residues, antibiotic resistance genes and zoonotic bacteria during biological swine manure treatment. Ecotoxicol. Environ. Saf. 175, 29–38. doi: 10.1016/j.ecoenv.2019.01.127
Van den Meersche, T., Rasschaert, G., Vanden Nest, T., Haesebrouck, F., Herman, L., Van Coillie, E., et al. (2020). Longitudinal screening of antibiotic residues, antibiotic resistance genes and zoonotic bacteria in soils fertilized with pig manure. Environ. Sci. Pollut. Res. Int. 27, 28016–28029. doi: 10.1007/s11356-020-09119-y
van den Meersche, T., Van Pamel, E., Van Poucke, C., Herman, L., Heyndrickx, M., Rasschaert, G., et al. (2016). Development, validation and application of an ultra-high performance liquid chromatographic-tandem mass spectrometric method for the simultaneous detection and quantification of five different classes of veterinary antibiotics in swine manure. J. Chromatogr. A 1429, 248–257. doi: 10.1016/j.chroma.2015.12.046
Van Epps, A., and Blaney, L. (2016). Antibiotic residues in animal waste: occurrence and degradation in conventional agricultural waste management practices. Curr. Pollution Rep. 2, 135–155. doi: 10.1007/s40726-016-0037-1
Van Gompel, L., Luiken, R. E. C., Sarrazin, S., Munk, P., Knudsen, B. E., Hansen, R. B., et al. (2019). The antimicrobial resistome in relation to antimicrobial use and biosecurity in pig farming, a metagenome-wide association study in nine European countries. J. Antimicrob. Chemother. 74, 865–876. doi: 10.1093/jac/dky518
van Hoek, A. H., Mevius, D., Guerra, B., Mullany, P., Roberts, A. P., and Aarts, H. J. (2011). Acquired antibiotic resistance genes: an overview. Front. Microbiol. 2:203. doi: 10.3389/fmicb.2011.00203
Van, T. T. H., Yidana, Z., Smooker, P. M., and Coloe, P. J. (2020). Antibiotic use in food animals worldwide, with a focus on Africa: pluses and minuses. J. Global Antimicrob. Resist. 20, 170–177. doi: 10.1016/j.jgar.2019.07.031
Vikesland, P. J., Pruden, A., Alvarez, P. J. J., Aga, D., Bürgmann, H., Li, X. D., et al. (2017). Toward a comprehensive strategy to mitigate dissemination of environmental sources of antibiotic resistance. Environ. Sci. Technol. 51, 13061–13069. doi: 10.1021/acs.est.7b03623
Walczak, J. J., and Xu, S. P. (2011). Manure as a source of antibiotic-resistant Escherichia coli and enterococci: a case study of a Wisconsin, USA family dairy farm. Water Air Soil Pollut. 219, 579–589. doi: 10.1007/s11270-010-0729-x
Wallace, J. S., and Aga, D. S. (2016). Enhancing extraction and detection of veterinary antibiotics in solid and liquid fractions of manure. J. Environ. Qual. 45, 471–479. doi: 10.2134/jeq2015.05.0246
Wallace, J. S., Garner, E., Pruden, A., and Aga, D. S. (2018). Occurrence and transformation of veterinary antibiotics and antibiotic resistance genes in dairy manure treated by advanced anaerobic digestion and conventional treatment methods. Environ. Pollut. 236, 764–772. doi: 10.1016/j.envpol.2018.02.024
Walsh, F. (2013). The multiple roles of antibiotics and antibiotic resistance in nature. Front. Microbiol. 4:255. doi: 10.3389/fmicb.2013.00255
Wang, R., Chen, M. X., Feng, F., Zhang, J. Y., Sui, Q. W., Tong, J., et al. (2017). Effects of chlortetracycline and copper on tetracyclines and copper resistance genes and microbial community during swine manure anaerobic digestion. Bioresour. Technol. 238, 57–69. doi: 10.1016/j.biortech.2017.03.134
Wang, L., Gutek, A., Grewal, S., Michel, F. C. Jr., and Yu, Z. (2015). Changes in diversity of cultured bacteria resistant to erythromycin and tetracycline in swine manure during simulated composting and lagoon storage. Lett. Appl. Microbiol. 61, 245–251. doi: 10.1111/lam.12450
Wang, L., Oda, Y., Grewal, S., Morrison, M., Michel, F. C. Jr., and Yu, Z. (2012). Persistence of resistance to erythromycin and tetracycline in swine manure during simulated composting and lagoon treatments. Microb. Ecol. 63, 32–40. doi: 10.1007/s00248-011-9921-9
Watanabe, N., Bergamaschi, B. A., Loftin, K. A., Meyer, M. T., and Harter, T. (2010). Use and environmental occurrence of antibiotics in freestall dairy farms with manured forage fields. Environ. Sci. Technol. 44, 6591–6600. doi: 10.1021/es100834s
Wepking, C., Avera, B., Badgley, B., Barrett, J. E., Franklin, J., Knowlton, K. F., et al. (2017). Exposure to dairy manure leads to greater antibiotic resistance and increased mass-specific respiration in soil microbial communities. PNAS 284:20162233.
Wichmann, F., Udikovic-Kolic, N., Andrew, S., and Handelsman, J. (2014). Diverse antibiotic resistance genes in dairy cow manure. mBio 5, e01017–e01013. doi: 10.1128/mBio.01017-13
Woegerbauer, M., Bellanger, X., and Merlin, C. (2020). Cell-free DNA: an underestimated source of antibiotic resistance gene dissemination at the interface between human activities and downstream environments in the context of wastewater reuse. Front. Microbiol. 11:671. doi: 10.3389/fmicb.2020.00671
World Health Organization (WHO) (2015). The Global Antimicrobial Resistance Surveillance System (GLASS). Available at: https://www.who.int/antimicrobial-resistance/publications/global-action-plan/en/
World Health Organization (WHO) (2019). Critically Important Antimicrobials for Human Medicine, 6th Revision. Geneva: World Health Organization. Licence: CC BY-NC-SA 3.0 IGO.
World Health Organization (WHO) (2021). Antimicrobial Resistance. Available at: https://www.who.int/news-room/fact-sheets/detail/antimicrobial-resistance
Wu, X., Wei, Y., Zheng, J., Zhao, X., and Zhong, W. (2011). The behavior of tetracyclines and their degradation products during swine manure composting. Bioresour. Technol. 102, 5924–5931. doi: 10.1016/j.biortech.2011.03.007
Yang, Q., Tian, T., Niu, T., and Wang, P. (2017). Molecular characterization of antibiotic resistance in cultivable multidrug-resistant bacteria from livestock manure. Environ. Pollut. 229, 188–198. doi: 10.1016/j.envpol.2017.05.073
Youngquist, C. P., Mitchell, S. M., and Cogger, C. G. (2016). Fate of antibiotics and antibiotic resistance during digestion and composting: a review. J. Environ. Qual. 45, 537–545. doi: 10.2134/jeq2015.05.0256
Yuan, W., Tian, T., Yang, Q., and Riaz, L. (2020). Transfer potentials of antibiotic resistance genes in Escherichia spp. strains from different sources. Chemosphere 246:125736. doi: 10.1016/j.chemosphere.2019.125736
Zalewska, M., Błażejewska, A., Czapko, A., and Popowska, M. (2021). Antibiotics and antibiotic resistance genes in animal manure – consequences of its application in agriculture. Front. Microbiol. 12:610656. doi: 10.3389/fmicb.2021.610656
Zhang, Y., Gu, A. Z., Cen, T., Li, X., He, M., Li, D., et al. (2018). Sub-inhibitory concentrations of heavy metals facilitate the horizontal transfer of plasmid-mediated antibiotic resistance genes in water environment. Environ. Pollut. 237, 74–82. doi: 10.1016/j.envpol.2018.01.032
Zhao, L., Dong, Y. H., and Wang, H. (2010). Residues of veterinary antibiotics in manures from feedlot livestock in eight provinces of China. Sci. Total Environ. 408, 1069–1075. doi: 10.1016/j.scitotenv.2009.11.014
Zou, Y., Xiao, Y., Wang, H., Fang, T., and Dong, P. (2020). New insight into fates of sulfonamide and tetracycline resistance genes and resistant bacteria during anaerobic digestion of manure at thermophilic and mesophilic temperatures. J. Hazard. Mater. 384:121433. doi: 10.1016/j.jhazmat.2019.121433
Keywords: antimicrobial resistance, antibiotic resistant bacteria, antibiotic residues, antibiotic resistance genes, manure, anaerobic digestion
Citation: Marutescu LG, Jaga M, Postolache C, Barbuceanu F, Milita NM, Romascu LM, Schmitt H, de Roda Husman AM, Sefeedpari P, Glaeser S, Kämpfer P, Boerlin P, Topp E, Gradisteanu Pircalabioru G, Chifiriuc MC and Popa M (2022) Insights into the impact of manure on the environmental antibiotic residues and resistance pool. Front. Microbiol. 13:965132. doi: 10.3389/fmicb.2022.965132
Edited by:
Axel Cloeckaert, Institut National de recherche pour l’agriculture, l’alimentation et l’environnement (INRAE), FranceReviewed by:
Séamus Fanning, University College Dublin, IrelandJinshil Kim, Seoul National University, South Korea
Copyright © 2022 Marutescu, Jaga, Postolache, Barbuceanu, Milita, Romascu, Schmitt, de Roda Husman, Sefeedpari, Glaeser, Kämpfer, Boerlin, Topp, Gradisteanu Pircalabioru, Chifiriuc and Popa. This is an open-access article distributed under the terms of the Creative Commons Attribution License (CC BY). The use, distribution or reproduction in other forums is permitted, provided the original author(s) and the copyright owner(s) are credited and that the original publication in this journal is cited, in accordance with accepted academic practice. No use, distribution or reproduction is permitted which does not comply with these terms.
*Correspondence: Gratiela Gradisteanu Pircalabioru, Z3JhdGllbGEuZ3JhZGlzdGVhbnVAaWN1Yi51bmlidWMucm8=; Mariana Carmen Chifiriuc, Y2FybWVuLmNoaWZpcml1Y0BnbWFpbC5jb20=