- 1Co-Innovation Center for Sustainable Forestry in Southern China, College of Biology and the Environment, Nanjing Forestry University, Nanjing, China
- 2Jiangsu Key Laboratory for Biodiversity and Biotechnology, College of Life Sciences, Nanjing Normal University, Nanjing, China
The gut microbiome is vital to the physiological and biochemical functions of the host, and changes in the composition of these microbial communities may affect growth and adaptability to the environment. Pomacea canaliculata is an invasive freshwater snail which has become a serious agricultural pest. Temperature adaptation is considered an important reason for the widespread distribution of this species. To date, the contribution of the gut microbes to host fitness of P. canaliculata during long-term temperature stress is not well understood. In this study, the morphological changes and intestinal microbiome of P. canaliculata under long-term stress at low temperature (15°C) and high temperature (35°C) were investigated with laboratory experiments. Compared with control group (25°C), the alpha diversity increased and pathogenic bacteria enriched changed under high and low temperature stress. The effect of high temperature stress on the intestinal microbiome of P. canaliculata was more significant than that of low temperature stress. A sustained high temperature environment led to an increase in the abundance of pathogenic bacteria, such as Aeromonas and Enterobacter, and a decrease in the abundance of immune-related bacteria such as Bacteroidetes, Firmicutes, and Lactococcus. These intestine microbiome changes can increase the risk of diseases like intestinal inflammation, and lead to more deaths at high temperature environments. In addition, with the extension of stress time from 14 to 28 days, the beneficial bacteria such as Bacteroidetes, Firmicutes, and Lactococcus were significantly enriched, while potential pathogenic bacteria such as Pseudomonas, Acinetobacter, Shivalella, and Flavobacterium decreased, suggesting that intestinal microbiota may play an important role in host response to heat stress. These results are consistent with previously reported results that the survival rate of both male and female P. canaliculata no longer significantly reduced after 21 days of high temperature stress, suggesting that the surviving P. canaliculata had gradually adapted to high temperature environments under long-term high temperature stress.
Introduction
Pomacea canaliculata (Caenogastropoda: Ampullariidae) is an invasive freshwater snail originating from South America that has been introduced outside its native range on occasion to other locations (Hayes et al., 2008), including Asia, Europe, and both North and South America, and it has become a serious agricultural pest that can damage crops and wetland ecosystems (Cowie, 2002; Hayes et al., 2015). During the invasion process of P. canaliculata, a variety of ecological adaptation mechanisms have occurred to cope with various environmental stresses, among which temperature adaptation is an important cause of the widespread distribution of this species (Yusa et al., 2006). Previous studies have shown that tolerance to low and high temperature environments is a key factor for the adaptation of P. canaliculata to harsh environments and successful expansion of its range of activities (Zheng et al., 2012; Yoshida et al., 2014).
Temperature is an important abiotic factor that affects the physiological state of animals, especially for aquatic organisms (Sepulveda and Moeller, 2020). It is an important environmental variable that affects both the physiological and biochemical reactions of aquatic organisms, thereby controlling their metabolic reaction rate and affecting their growth and development (Kelley, 2014; Jiang et al., 2016; Sepulveda and Moeller, 2020). To date, it is widely recognized that several aspects of the life cycle of P. canaliculata, including growth, feeding, crawling, aerial respiration, reproduction and survival, can be affected by temperature (Matsukura and Wada, 2007; Seuffert et al., 2010). P. canaliculata died at low temperatures close to 0°C (Matsukura et al., 2009). During winter most P. canaliculata are inactive but not in a deep lethargic state, and at 13–15°C, half are active. Laboratory test showed that the active and feeding time of P. canaliculata increased significantly as the temperature increased from 10 to 30°C (Seuffert et al., 2010), and their activity level and feeding ability peaked between 25 and 32°C (Heiler et al., 2008; Seuffert et al., 2010). Although P. canaliculata had no mortality at 15 and 20°C, their growth rate was low, whereas at 25, 30, and 35°C the snails grew faster but displayed a reduction in survival (Seuffert and Martín, 2013). At temperatures above 35°C, however, the growth rate of P. canaliculata decreased significantly (Seuffert and Martín, 2013). The optimal temperature for the hatching of P. canaliculata eggs is about 30°C, with no eggs are laid above 35°C, and no reproductive activities are carried out below 15°C (Seuffert and Martín, 2017). The frequency and duration of side crawling of P. canaliculata significantly decreased when the water temperature was decreased from 25 to 20°C or 15°C, while the ventilation behavior and food consumption increased when the water temperature was increased from 25 to 30°C (Bae et al., 2021).
The gut microbiome, also known as the “animal second genome,” has co-evolved with animals, interacts with each other, and is capable of significantly affecting the physiology, development and health of its hosts (McFall-Ngai et al., 2013). The community structure and diversity of the gut microbiome are affected by the host’s own physiological conditions. The gut microbiome can also participate in the digestion and absorption of host nutrients as well as energy metabolism, and can function to regulate the expression of host genes, material metabolism, and tissue and organ development (Flint et al., 2008; Hall et al., 2017). The gut microbiota is in a dynamic balance, and various host and environmental factors can affect its diversity and community structure (Zhao et al., 2021), such as host gender (Ma et al., 2018), health status (Chandler et al., 2014), diet (Cardoso et al., 2012), season (Pierce et al., 2016), and temperature (Li et al., 2019; Sepulveda and Moeller, 2020).
Temperature is considered one of the main factors affecting the gut microbial community (Sepulveda and Moeller, 2020) and long-term seasonal changes in temperature may lead to adaptive changes in the composition of the gut microbiome. The gut and pallial fluid microbiome of the American oyster (Crassostrea virginica) are affected significantly by water temperature, which might be attributed the decreased water temperatures in winter that leads to decreased metabolic activity in many bacteria and oysters due to reduced physical activity (Pierce et al., 2016). Gut microbial communities may also be affected by temperature on shorter timescales, such as extreme weather events (heat waves, cold snaps, etc.) (Gao et al., 2012). Studies showed that the composition of the gut microbial community of the red-backed salamander (Plethodon cinereus) differed under the three temperatures (10, 15, and 20°C). High temperature stress led to a decrease in the relative abundance of disease-resistant bacteria and an increase in the relative abundance of pathogenic bacteria (Fontaine et al., 2018). If the gut microbiota of some species is more plastic than others, they may exhibit greater phenotypic plasticity in new environments, and such a feature is often found in invasive organisms (Davidson et al., 2011). Studies have shown that ambient temperature can alter gut microbial diversity and composition in ectothermic animals (Bestion et al., 2017; Huyben et al., 2018; Fontaine and Kohl, 2020).
In this study, we investigated the effects of high temperature and low temperature stress on the growth and development of P. canaliculata along with the response of the intestinal microbiome to the long-term temperature stress. We hope that revealing the ability of invasive snails to quickly adapt to environmental changes and the mechanism of successful invasion will provide new ideas and a scientific basis for the prevention and effective management of invasive organisms.
Materials and methods
Sample collection
The eggs of P. canaliculata were collected from Taihu Wetland Park located in Kunshan (N 30.916°, E 120.403°), Jiangsu, China, in June, 2021. These eggs were brought back to the lab and hatched using a climate chamber (25°C with a cycle of 14 h-light: 10 h-dark; the humidity was 80%).
Species were identified based on the morphological characteristics of the egg mass combined with molecular experiments. Two to three eggs were randomly selected from each egg mass to extract genomic DNA, amplify the mitochondrial cytochrome c oxidase subunit I (COI) gene fragment (Yang et al., 2018), and sequence the target PCR product. After the eggs were confirmed to be P. canaliculata, the eggs masses were placed in an incubator to hatch. The hatchlings used in the experiment were put into plastic boxes (36 × 25 × 12 cm) containing aerated water and kept at 25°C. During the course of the experiment, P. canaliculata were fed with lettuce leaves every day, the aerated water was changed every 2 days.
Temperature treatment and morphometric measurement
Pomacea canaliculata of the same age (three and half a month) with similar length (female: 26.47 ± 0.96 mm; male: 25.96 ± 1.16 mm) were selected for temperature stress experiments using different temperatures: high temperature stress (35°C), low temperature stress (15°C) and control (25°C). Female and male P. canaliculata were divided to create a total of six experimental groups including high temperature group (HF), low temperature group (LF), and control group (CF) for females, and high temperature group (HM), low temperature group (LM), and control group (CM) for males. Three parallel repetitions were performed in each group, with 20 snails were placed in each repetition. The temperature stress experiment was conducted in an artificial climate box with a 14 h-light: 10 h-dark photoperiod and an 80% humidity. During the experiment, aerated water was used and changed every 2 days, with replacement water placed into a corresponding climate box 1 day in advance to make temperature consistent until the end of the experiment. Fresh lettuce leaves were fed every day.
All P. canaliculata were marked with a marking pen for morphological data measurement of the snails during the experiment. From the beginning of the experiment (0 days) to the end of the experiment (28 days), the behavior and activities of P. canaliculata were observed at a fixed time three times a day. The number of dead snails was recorded and these dead individuals were removed in a timely manner. Acupuncture and rehydration methods were used to identify the survival, suspended animation, and death (Bernatis et al., 2016). Morphological data measurements were performed every 7 days. The wet weight of the snails was measured and recorded using an electronic balance (Mettler Toledo, 0.001 g). Vernier calipers were used (Mitutoyo, 0.01 mm) to measure and record the length and width of the snail shells. The relevant formulas are as follows:
(1) Survival rate (%) = (Number of surviving snails/total number of snails) × 100% (Seuffert and Martín, 2013)
(2) Specific growth rate (%) = [(lnW2-lnW1)/(t2-t1)] × 100% (Ruppert et al., 2016)
(3) Weight gain rate (%) = (W2-W1)/W1 × 100% (Júnior et al., 2013)
(4) Growth rate of snail length (%) = (SL2-SL1)/SL1 × 100% (Bernatis et al., 2016)
In the above formula, W1 and W2 are the wet weights of P. canaliculata at time t1 and t2, SL1 and SL2 are the shell lengths of P. canaliculata at time t1 and t2, respectively.
Sampling
On the 14th day (14 days) and 28th day (28 days) after the start of the temperature stress experiment, five snails were randomly selected from the parallel replicates of the six temperature groups, for a total of 60 snails. The coiled gut content was extracted from the P. canaliculata according to Chen L. et al. (2021). The foot muscle of each of the sampling snails was isolated to further identify the P. canaliculata by amplifying COI gene using LCO1490/HCO2198 as described by Hayes et al. (2009). PCR amplification products were sequenced by Shanghai Sangon Biological Engineering Technology & Services Co., Ltd (Shanghai, China).
The group information of the 60 snails was as follows. The samples collected on the 14th day (14 days) included the female snail high temperature group (HF14), female snail low temperature group (LF14), female snail control group (CF14), male snail high temperature group (HM14), male snail low temperature group (LM14), and male snail control group (CM14). The samples collected on the 28th day (28 days) included the female snail high temperature group (HF28), female snail low temperature group (LF28), female snail control group (CF28), male snail high temperature group (HM28), male snail low temperature group (LM28), and male snail control group (CM28). Each group contained five snails.
Total DNA was extracted from the intestinal contents of 60 snails in small tubes using the FastDNA® Kit (MP 149 Biomedicals, Santa Ana, CA, United States). The experiments were carried out using universal primers in the V3-4 region of the 16S rRNA gene. The specific primer sequences were as follows: 341F (5′-CCTAYGGGRBGCASCAG-3′), 806R (5′-GGACTACNNGGGTATCTAAT-3′) (Dao et al., 2021). PCR products were detected using 2% agarose gel. The TruSeq Nano DNA LT Library Prep Kit (Illumina, United States) was used to build the library according to the manufacturer’s protocol. Then the PCR products were quantified using the Agilent High Sensitivity DNA Kit (Beijing, China). After quality assessment and quantification, amplicons were pooled and sequenced according to standard protocols using the Illumina Novaseq platform by Novo-gene Co. Ltd. (Beijing, China).
Data analysis
Microbiome bioinformatics analysis was performed using QIIME2 (version 2019.4)1 (Bolyen et al., 2019). Qiime cutadapt trim-paired was used to excise the primer fragment of the sequence and remove the sequences that did not match the primer. DADA2 was selected based on the qiime dada2 denoise-paired for quality control, denoising, splicing, and removing chimeras in the sequence (Callahan et al., 2016). After denoising all the libraries, the amplicon sequence variants (ASVs) signature sequences and ASV tables were merged, and singleton ASVs were removed. The sequences were aligned and species were annotated against the SILVA SSU rRNA database (version 132)2 using RDP Native Bayesian Classifier (version 2.11) (Quast et al., 2013; Bokulich et al., 2018).
Sequence data analysis was mainly performed using QIIME2 and R package (version 3.2.0). The ASV table of QIIME2 was used to calculate the alpha diversity index of the ASV level, including the Shannon-wiener diversity index, Simpson diversity index, Chao1 richness estimator, observed species, and Goods_coverage index, the data are expressed in the form of a box plot. The alpha diversity indices were compared using the Kruskal–Wallis H, and P < 0.05 indicates a significant difference.
Non-metric multidimensional scaling (NMDS) and unweighted pair-group method with arithmetic means (UPGMA) were used for beta diversity analysis. Analysis of similarity (ANOSIM) (Clarke, 1993; Warton et al., 2012) was used to assess the significance of microbial community structure differentiation between groups. Mantel tests based on spearman’s correlation analysis were used to determine the correlations between temperature, stress time and microbiota variation, respectively. NMDS ordinations was analyzed based on the Bray-Curtis dissimilarity matrices in the “vegan” package in R (Dixon, 2003).
Morphological data were analyzed with the SPSS 25.0 statistical package, and Kolmogorov–Smirnov and Bartlett tests were used to test data normality and homogeneity respectively. Two-way repeated-measures ANOVA was used to analyze the effects of temperature and sex on the growth rate of snail length, weight gain rate and specific growth rate of P. canaliculata, with temperature treatments and sex as the fixed factors, and time as the within-subject factor. The relative abundances of important dominant bacterial phyla in the high temperature group, low temperature group and control group were compared based on one-way ANOVA. The differences between treatments in multiple samples were tested using Duncan’s multiple comparisons. All descriptive statistical values are expressed as mean ± standard error (SE), and the significance level was set at α = 0.05.
Results
Effect of temperature on the growth and development of Pomacea canaliculata
After 28 days of temperature stress, the survival rate of male and female P. canaliculata was highest in the control group and the lowest in the high-temperature group (Figure 1 and Supplementary Table 1) with one male high temperature groups experienced mass death of snails. Both 35 and 15°C caused the survival rate of snails to decrease, and the negative effect of high temperature on the survival rate of snails was more significant.
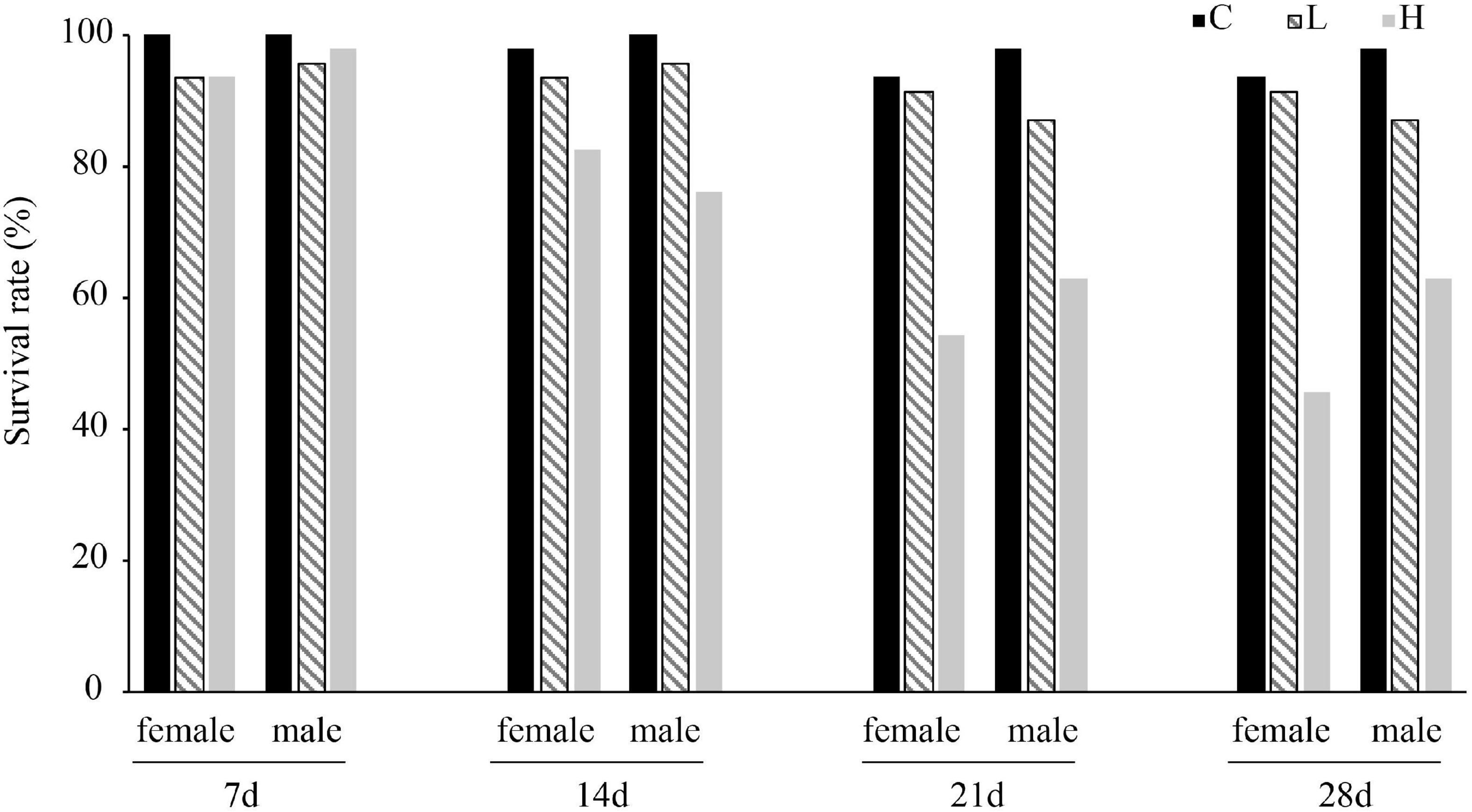
Figure 1. Survival rate of female and male Pomacea canaliculata at different temperature and treated time.
Two-way repeated-measures ANOVA showed that stress time had a significant effect on the growth rate of snail length (F1,114 = 26.907, P < 0.001) (Tables 1, 2). Specifically, the interaction of stress time and temperature had a significant effect on both the growth rates (F2,114 = 29.867, P < 0.001) and specific growth rates (F2,114 = 15.079, P < 0.001) of the snails of P. canaliculata (Tables 1, 2). The interaction of stress time and sex had a significant effect on the growth rates of snail length and weight gain (F1,114 = 29.677, P < 0.001) (Tables 1, 2), but no significant effect was observed on the specific growth rate. The interaction of stress time, temperature and sex had a significant effect on the growth rate of snail length (F2,114 = 30.085, P < 0.001) (Table 2), but no significant effect was observed on the weight gain rate or the specific growth rate.
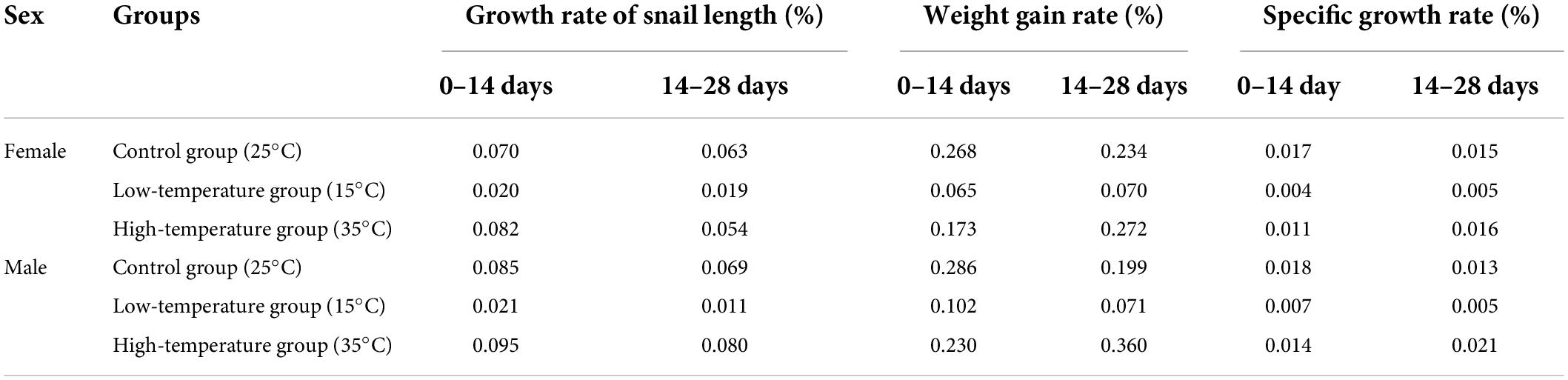
Table 1. Growth rate of snail length, weight gain rate, and specific growth rate of female and male Pomacea canaliculata at different temperature and treated time.

Table 2. Results of two-way repeated measures ANOVA to test the effect of temperature and sex as fixed factors, with treated time as within-subject factor, during different period on growth rate of morphological index.
Gut microbial amplicon sequence variant and alpha diversity index
After preliminary filtering, the deduplicated sequences were clustered at 97% similarity level, and 57,537 ASVs were then obtained. According to the species annotation results, bacteria were classified into 54 phyla, 182 classes, 366 orders, 619 families, and 1,370 genera. The rarefaction curves of each group tended to be flat, suggesting that the sequencing results could represent the real species composition in the samples (Supplementary Figure 1).
The Goods coverage index of each group was above 98%, indicating a high level of diversity coverage. The Kruskal–Wallis H-test was used to analyze the abundance and diversity of the intestinal microbiota of P. canaliculata. Since there was no significant difference in the alpha diversity of intestinal microbiota between female and male P. canaliculata (P > 0.05), the data of same sex were combined for alpha diversity analysis. The female and male samples were combined for each of the temperature and stress time groups for analysis.
After 14 days of temperature stress treatment, the order of Chao1 index and observed species index from high to low was the low temperature group, high temperature group, and control group (Figure 2A). No significant difference was observed after 14 days of temperature stress treatment. The results of the Chao1 index and observed species index after both 14 and 28 days temperature stress treatment were consistent (Figure 2B). However, the observed species index of the high temperature group was significantly greater than that of the control group after the 28-days temperature stress treatment (P < 0.05) (Figure 2B). The order of Shannon index and Simpson index from high to low was: the high temperature group, low temperature group and control group after both 14 and 28 days treatment. The Simpson index in both 14 and 28 days treatment groups was significantly higher than that in the control group (P < 0.05) (Figures 2A,B). After 14 or 28 days of temperature stress treatment, the high temperature group had the greatest richness and diversity index for intestinal microbiome, followed by the low temperature group and then the control group (Figures 2A,B).
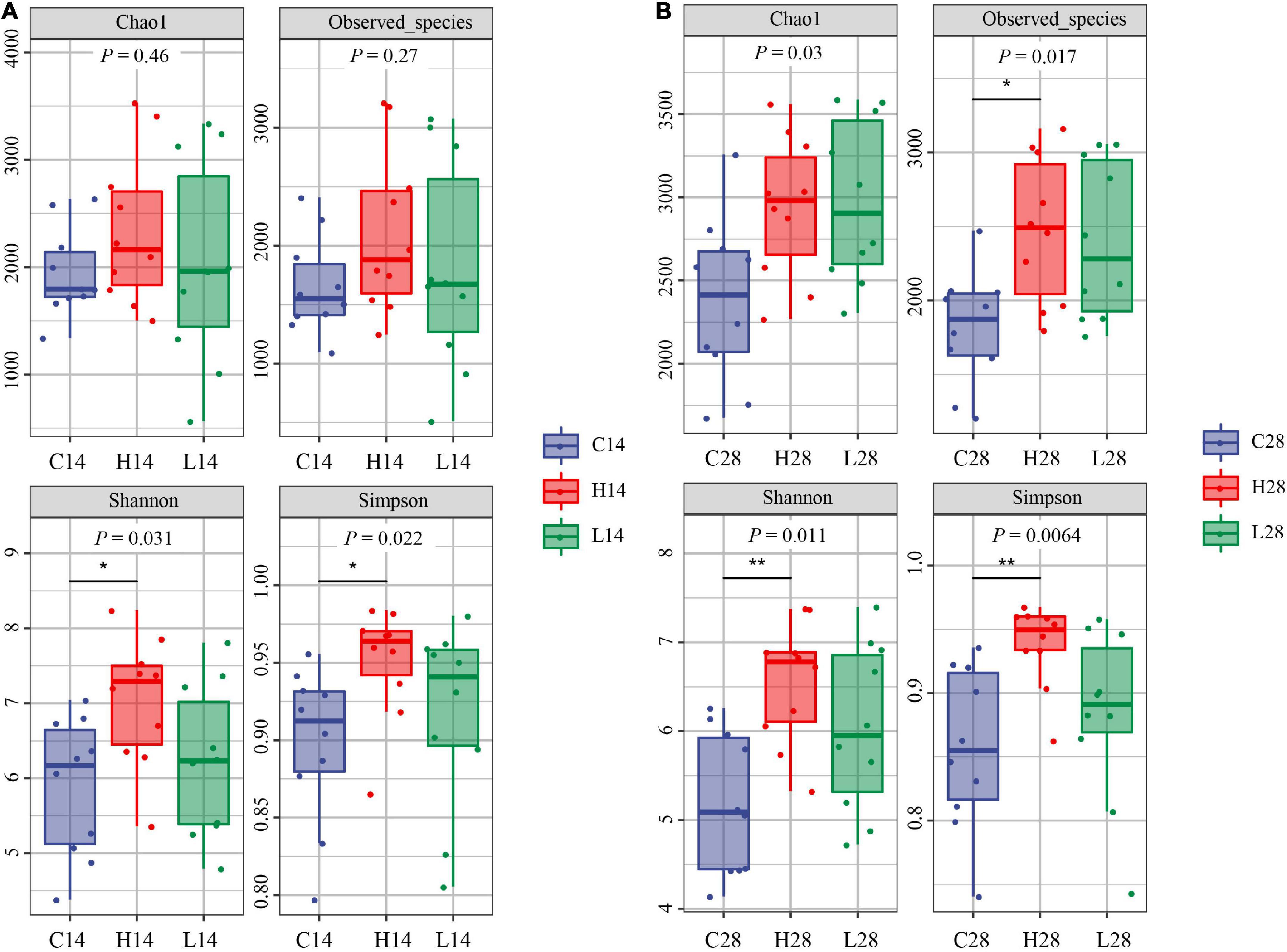
Figure 2. Comparison of intestinal microbiome alpha diversity index of different temperatures on 14 days (A) and 28 days (B). C14, H14, and L14 represent the control group, high and low temperature groups treated for 14 days, respectively. C28, H28, and L28 represent the control group, high and low temperature groups treated for 28 days, respectively. *P < 0.05 and <0.01 and **P < 0.01 represented the differences that were significant.
Both the richness index and diversity index had no significant difference after 14 and 28 days in control groups (Figure 3A). The Chao1 index of 28 days high and low temperature groups was significantly higher than that of the 14 days groups (P < 0.05), and no significant difference was observed in other indexes (Figures 3B,C). These results indicated that under the stress of low and high temperature, the abundance of intestinal microbiota of P. canaliculata increased significantly with the extension of time (P < 0.05).
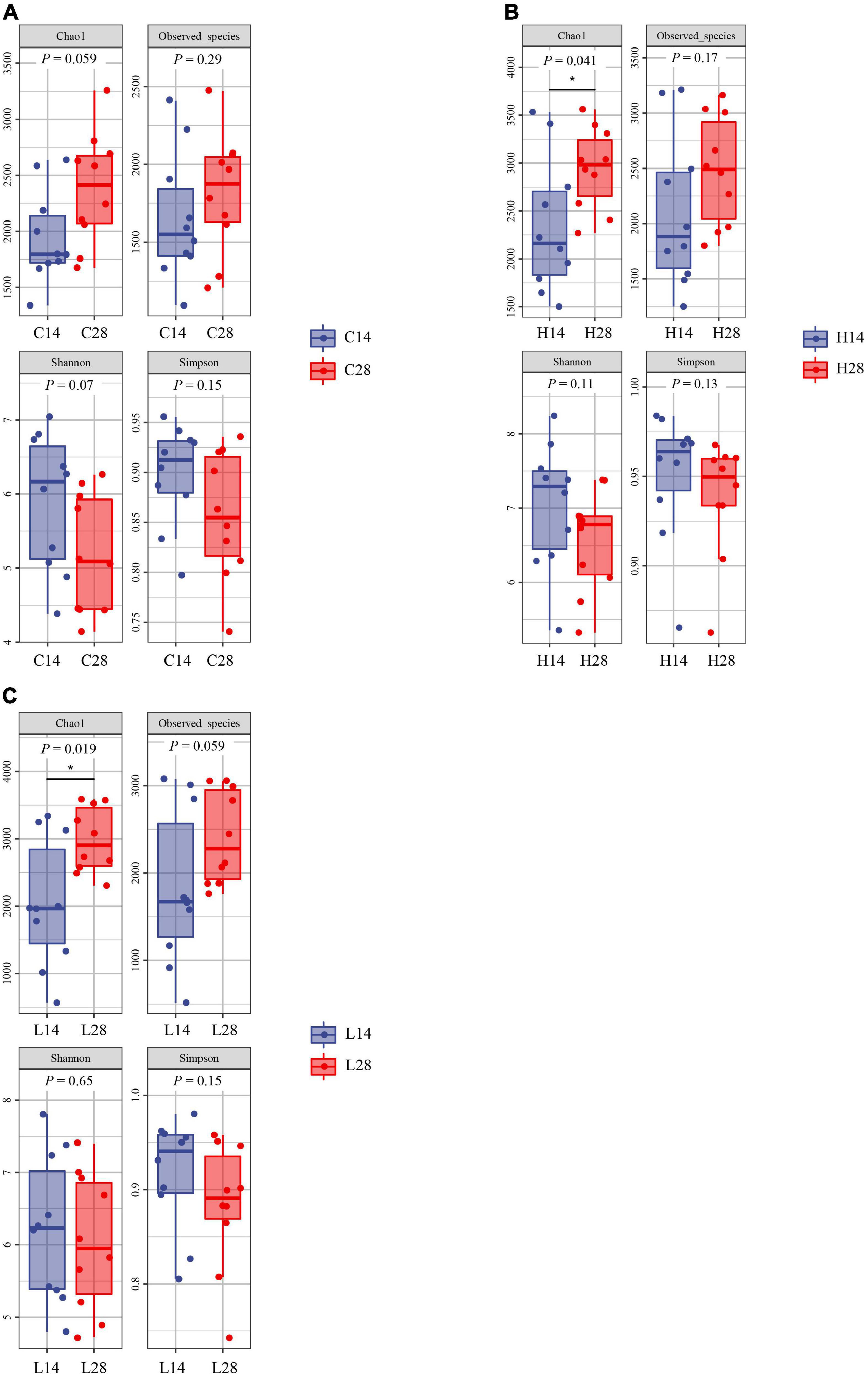
Figure 3. Comparison of intestinal microbiome alpha diversity index of different experimental time points at the control group (A), high temperature group (B), and low temperature group (C). The * denotes a significant difference (P < 0.05 and <0.01) between groups.
Composition of intestinal microbial community of Pomacea canaliculata under different temperature stress
At the phylum level, the intestinal microbiome of P. canaliculata in the high-temperature group was mainly composed of Proteobacteria, Firmicutes, Bacteroidetes, and Fusobacteria, with a relative proportion of 32.96, 30.03, 23.68, and 10.80%, respectively. In the control group, the relatively high abundant microbiome included Proteobacteria (66.73%), Bacteroidetes (15.76%), Firmicutes (11.40%), Fusobacteria (1.35%), and Actinobacteria (1.06%). In the low temperature group, the relatively high abundant microbiome included Proteobacteria (54.31%), Bacteroidetes (24.84%), Firmicutes (14.11%), Actinobacteria (1.15%), Cyanobacteria (1.24%), and Spirochetes (1.18%) (Figure 4A).
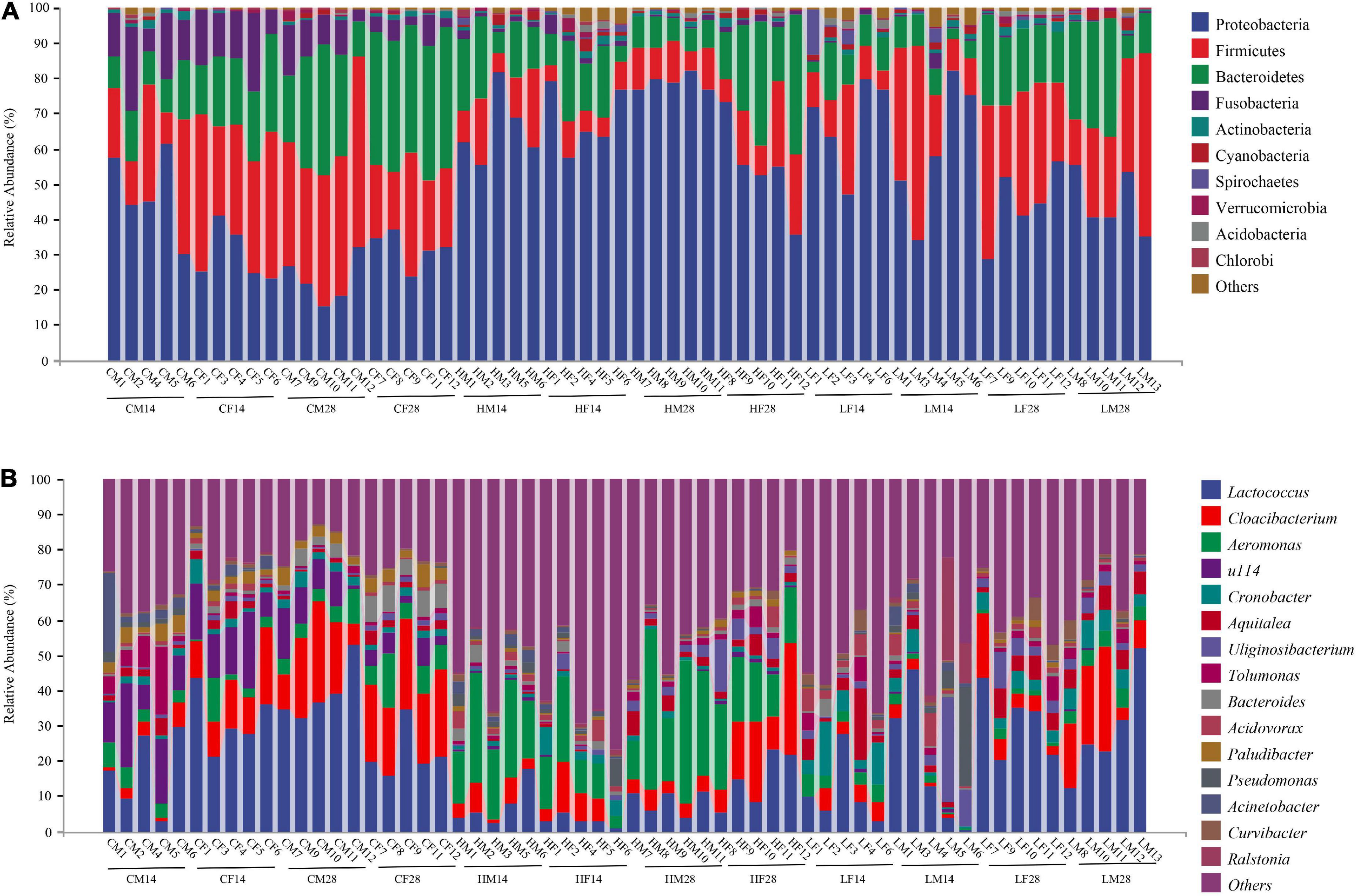
Figure 4. Abundance at the phylum (A) and genus (B) level of intestine microbiota in Pomacea canaliculata treated in different temperatures (mean relative abundance > 1%).
At the genus level, the dominant genera in the intestinal microbiome of the snails in the high temperature group were Aeromonas, Lactococcus, and Cloacibacterium, accounting for 20.21, 8.24, and 20.24%, respectively. The intestinal microbiome in the control group was mainly composed of Lactococcus (27.42%), Cloacibacterium (14.00%), u114 (10.36%), and Aeromonas (5.58%). In the low temperature group, the intestinal microbiome of the snail was mainly composed of Lactococcus (22.22%), Cloacibacterium (7.44%), Cronobacter (5.05%), Aquitalea (4.71%), Uliginosibacterium (4.56%), and Aeromonas (2.82%) (Figure 4B).
Analysis of beta-diversity of the intestinal microbiome of Pomacea canaliculata under different temperature stress
According to the Bray-Curtis distance algorithm, all samples were clustered at the genus level. The results showed that the samples in the high temperature group were first placed into one cluster, while the low temperature and control groups were not divided into two clusters but confusingly classified. The cluster of the high temperature group was further divided into two clusters: the 14 days treatment groups and the 28 days treatment groups (Figure 5A). Because the clustering of the samples in control group and low temperature groups was relatively chaotic, to further study the effects of low temperature and stress time on the intestinal microbiome of P. canaliculata, NMDS and ANOSIM analyses were performed based on the Bray-Curtis distance algorithm. The samples were aggregated based on three temperatures and the two stress times, and the results showed that both temperature and stress time had a significant impact on the intestinal microbiome of P. canaliculata (R = 0.6052, P < 0.001) (Figure 5B).
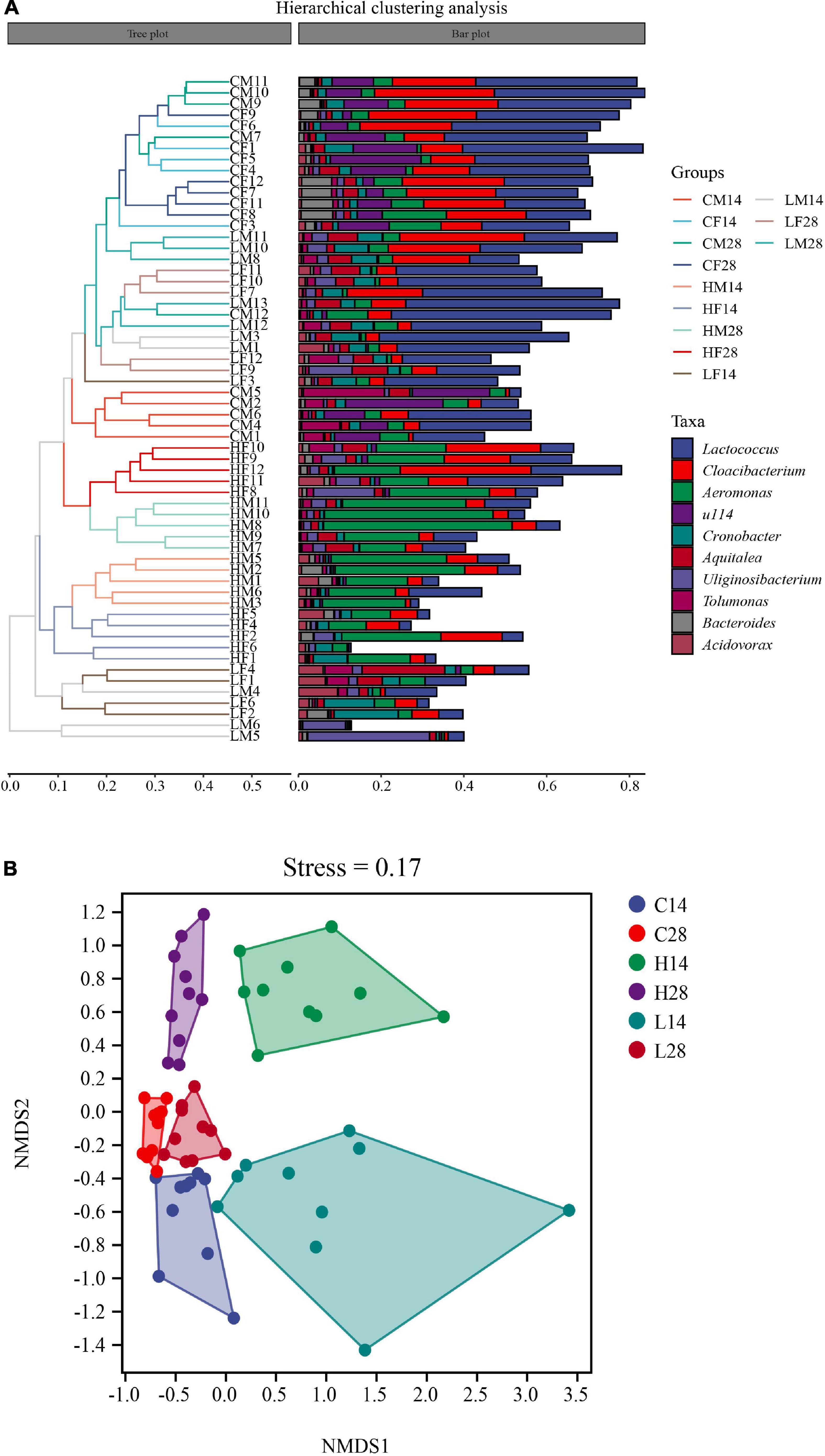
Figure 5. The UPGMA cluster analysis of samples in Bray-Curtis distances (A); NMDS analysis based on Bray-Curtis distances of intestine microbiome (B).
The results of mantel test showed the similar trend that temperature and stress time were significantly correlated with the intestinal microbiome of P. canaliculata (P < 0.05). Temperature treatment was significantly correlated with intestine microbial communities of P. canaliculata in both sexes (P < 0.05) (Supplementary Tables 2–4).
Differences in the intestinal microbial structure of Pomacea canaliculata under different temperature stress
Non-metric multidimensional scaling and ANOSIM analyses based on the Bray-Curtis distance showed that the structure of the gut microbiota of female snails was significantly different after 14 days stress at different temperatures (R = 0.868, P < 0.001) (Figure 6A). Similar phenomenon was observed for male snails (R = 0.644, P < 0.001) (Figure 6B). There were still significant differences in the intestinal community composition of female snails under different temperatures for 28 days (R = 0.876, P < 0.001) (Figure 6C) and again, similar phenomenon was observed for male snails (R = 0.892, P < 0.001) (Figure 6D). After both 14 and 28 days of temperature stress treatment, there were significant differences in the gut microbiota structure of P. canaliculata under different temperature treatments, indicating that temperature stress had a significant effect on the structure of the intestinal microbiome of P. canaliculata.
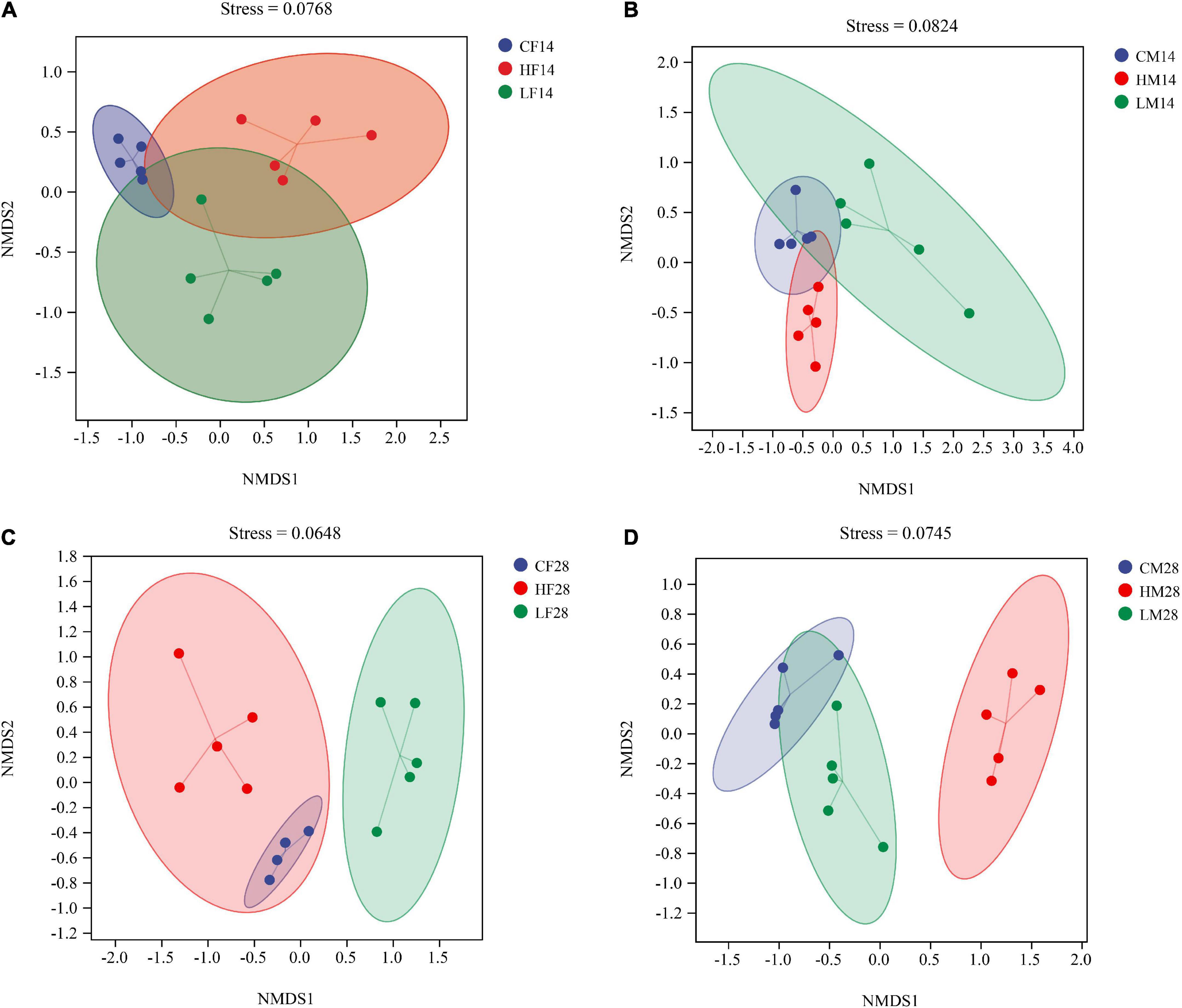
Figure 6. Non-metric multidimensional scaling (NMDS) analysis based on Bray-Curtis distances plots of Pomacea canaliculata intestine microbial communities at the different temperature [(A) 14 days female; (B) 14 days male; (C) 28 days female; (D) 28 days male].
Differences in the intestinal microbial structure of Pomacea canaliculata with different stress times between different sexes
The NMDS and ANOSIM analysis based on the unweighted-UniFrac distance algorithm showed that the structure of the intestinal microbiome in female P. canaliculata varied significantly over time in both high and low temperature groups (P < 0.05) (Table 3 and Supplementary Figure 2), indicating that the duration of temperature stress had a significant effect on the intestinal bacteria in female P. canaliculata. This result was also supported by NMDS and ANOSIM analysis based on the weighted-UniFrac distance algorithm (Table 3 and Supplementary Figure 2). For males, the duration of temperature stress also had a significant effect on the structure of the gut microbiota (Table 3 and Supplementary Figure 2).

Table 3. Analysis of similarity (ANOSIM) analyses based on unweighted-UniFrac and weighted-UniFrac distances of Pomacea canaliculata intestine microbial communities at the different experimental time points.
The ANOSIM analysis based on the Bray-Curtis distance algorithm also showed significant difference in the intestinal microbiome between female and male P. canaliculata. Significant difference was also observed between 14 and 28 days treatment groups and between high and low temperature groups (R > 0.5, P < 0.05) (Figure 6).
Differential analysis of the gut microbiome at different temperatures, different stress times, and different sexes
It can be seen from the Venn diagram that the high, low temperature and control groups had a total of 3,758 ASVs, of which the high temperature group had 18,233 ASVs (13.92%), the low temperature group had 17,580 ASVs (14.67%), and the control group had 11,433 ASVs (19.81%) (Figure 7A).
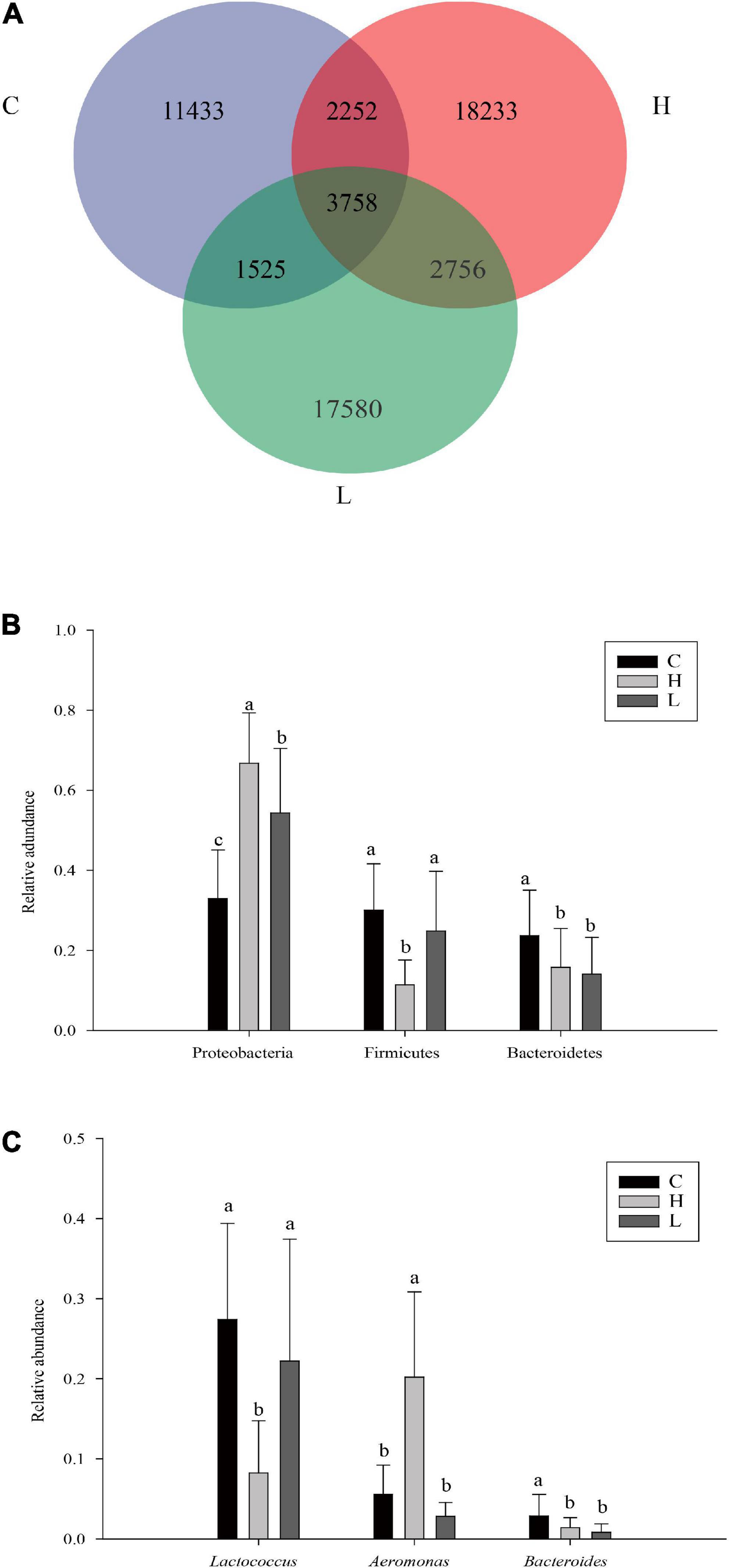
Figure 7. Venn analysis of samples from different temperature (A). The relative abundances of dominant phylum (B) and genus (C) of Pomacea canaliculata intestine microbiome at different temperature group (Duncan’s post hoc test, a = 0.05; a>b>c). The C, H, and L represented the control group, high and low temperature groups treated for 14 and 28 days, respectively.
At the phylum level, Proteobacteria, Firmicutes, and Bacteroidetes were the dominant phyla shared by these groups. The relative abundance of important dominant bacterial phyla in the high, low temperature and control groups was compared based on one-way analysis of variance. The results showed that the relative abundance of Proteobacteria in the high temperature group was the largest (66.73%), which was significantly higher than that of the low temperature group (54.31%) and control group (32.96%) (F2,57 = 31.05, P < 0.001). Firmicutes also had significant differences among different temperature groups, and its relative abundance in the high temperature group (11.40%) was significantly lower than that in the low temperature (24.84%) and control groups (30.03%) (F2,57 = 14.04, P < 0.001). Furthermore, the relative abundance of Bacteroidetes decreased sequentially in the control (23.68%), high (15.76%), and low temperature (14.11%) groups. The relative abundance of Bacteroidetes in the control group was significantly greater than that in the high and low temperature groups (F2,57 = 5.14, P = 0.0089) (Figure 7B).
At the genus level, Lactococcus, Aeromonas, and Bacteroides were the dominant genera shared by the high, low temperature and control groups. The relative abundance of Lactococcus in the high temperature group (8.24%) was significantly lower than that in the low temperature (22.22%) and control (27.42%) groups (F2,57 = 14.14, P < 0.001). The relative abundance of Aeromonas in the high temperature group (20.21%) was significantly higher than that in the low temperature (2.82%) and control (5.58%) groups (F2,57 = 40.43, P < 0.001). Additionally, the relative abundance of Bacteroides decreased successively in the high, low temperature and control groups, and their relative abundances were 1.41, 0.83, and 2.84%, respectively. The relative abundance of Bacteroides in the high and low temperature groups was significantly lower than in the control group (F2,57 = 6.38, P = 0.003) (Figure 7C).
To further explore the potential biomarkers with statistically significant differences between the high, low temperature and control groups, LEfSe analysis was performed on the samples with different stress times and sex (| LDA| > 3.5). There were one phylum, two classes, two orders, four families, and six genera in the microbiota with different abundances in the high temperature group, and Proteobacteria, Rikenellaceae, Rhodospirillaceae, Aeromonas, Blvii28, Enterobacter, Novispirillum, and Vogesella were significantly enriched (Figure 8). There were one phylum, three classes, seven orders, six families, and eight genera in the low temperature group, and Cyanobacteria, Neisseriales, Enterobacteriaceae, Neisseriaceae, Oxalobacteraceae, Cronobacter, Uliginosibacterium, Aquitalea, Acidovorax, and Methylotenera were significantly enriched (Figure 8). There were three phyla, four classes, four orders, five families, and six genera in control group, and Firmicutes, Fusobacteria, Bacteroidetes, Streptococcaceae, Fusobacteriaceae, Porphyromonadaceae, Bacteroidaceae, Lactococcus, Cloacibacterium, and Bacteroides were significantly enriched (Figure 8).
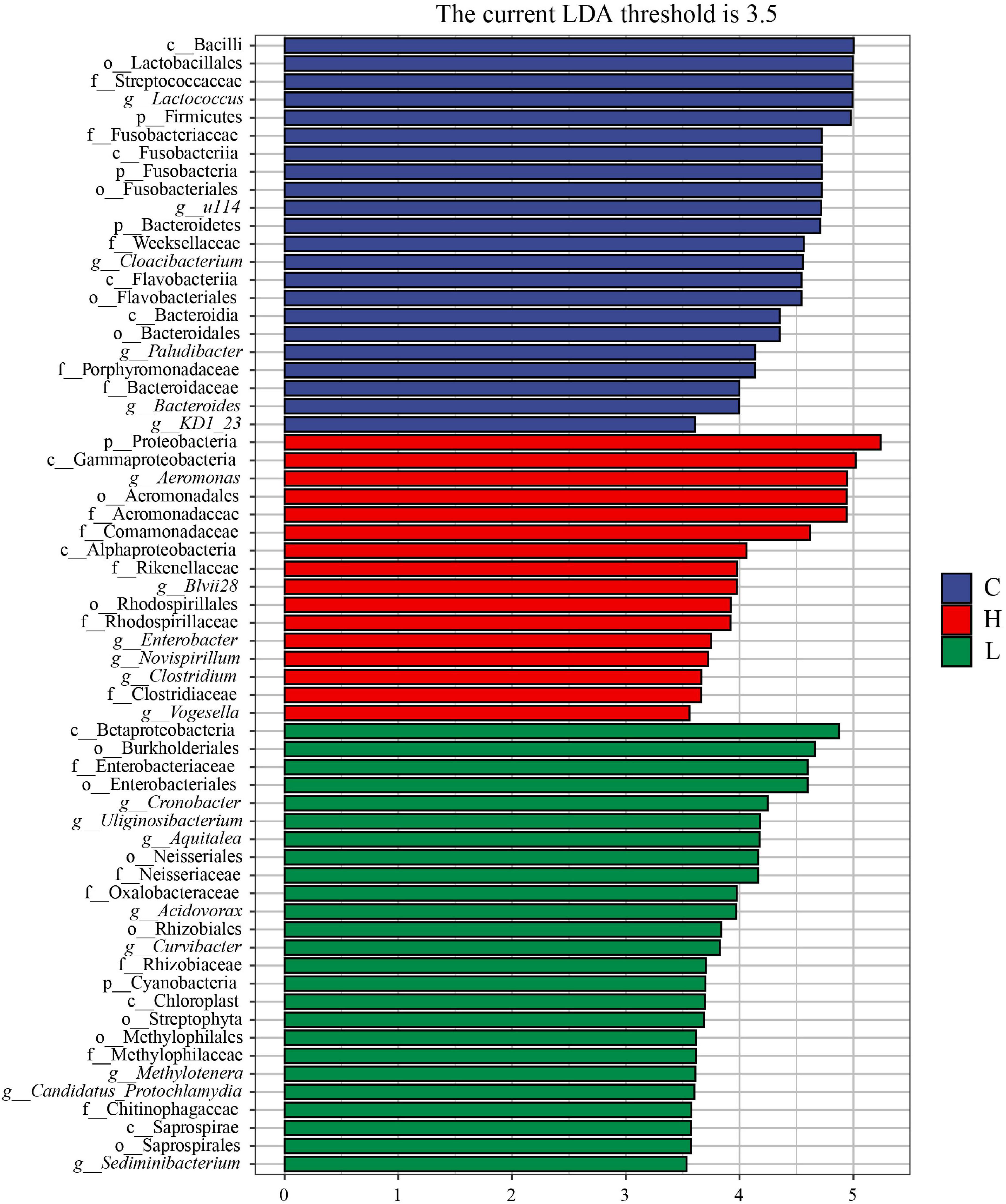
Figure 8. LEfSe analysis of Pomacea canaliculata intestinal microbiota composition under different temperature. The c, g, f, o, and p represented class, genus, family, order, phylum, respectively.
Furthermore, there were two phyla, four classes, five orders, five families, and four genera with different abundances in the intestinal microbiome of P. canaliculata under 14-days low temperature stress, including Proteobacteria, Fusobacteria, Lachnospiraceae, Bacteriovoracaceae, Ralstonia, Pseudomonas, and Acidovorax. There were two phyla, two classes, four orders, four families, and four genera significantly enriched in the 28-days low temperature stress group, including Bacteroidetes and Firmicutes, Lactococcus, and Chryseobacterium (Supplementary Figure 3A).
There were three classes, seven orders, 10 families, and seven genera with different abundances in the 14-days high temperature stress group, including Spirochetes, Comamonadaceae, Rhodospirillaceae, Oxalobacteraceae, Lachnospiraceae, Pseudomonas, Shewanella, Acinetobacter, Acidovorax, and Flavobacterium. There were one class, three orders, three families, and six genera with significant differences in abundance in the 28-days high temperature stress group, including Streptococcaceae, Neisseriaceae, Lactococcus, Enterobacter, and Aquitalea (Supplementary Figure 3B).
In the 28-days low temperature group (L28), Actinobacteria, Acidobacteria, and Comamonas were significantly enriched in the female samples, while Aeromonas, Cronobacter, and Enterobacter were significantly enriched in the male samples (Supplementary Figure 4A). In the 28-days high temperature group (H28), Bacteroidetes, Fusobacteria, and Bacteroides were significantly enriched in the female samples, while Proteobacteria, Enterobacteriaceae, and Sulfurospirillum were significantly enriched in the male samples (Supplementary Figure 4B). In the 28-days control group (C28), Bacteroidetes, Proteobacteria, Bacteroides, Enterobacter, and Aquitalea were significantly enriched in the female samples, while Fusobacteria and Lactococcus were significantly enriched in the male samples (Supplementary Figure 4C).
Discussion
The intestinal microbiome is involved in various functions, such as immune regulation, gastrointestinal motility, and energy metabolism regulation (Paaijmans et al., 2013). In this study, we used the Illumina Novaseq sequencing technology of the 16S rRNA gene to investigate the response of the intestinal microbiome of P. canaliculata to temperature stress. The results showed that the alpha diversity and community structure of the intestinal microbiome of P. canaliculata changed significantly under high temperature (35°C) and low temperature (15°C) stress.
Effect of temperature stress on the growth and development of Pomacea canaliculata
Previous studies have shown that the survival rate of P. canaliculata is negatively correlated with temperature, and the survival rate is the lowest at 35°C (Seuffert and Martín, 2013). In the high temperature range (25, 30, and 35°C), the survival rate of P. canaliculata decreased with increasing temperature, while in the low temperature range (0 and 15°C), the survival rate increased with decreasing temperature (Seuffert and Martín, 2013). The present study showed that the survival rate of P. canaliculata was the lowest in the high temperature group (54.35%), followed by low temperature group and control group (Figure 1 and Supplementary Table 1). In addition, after 21 days of high temperature stress, the survival rates of female and male P. canaliculata were no longer significantly reduced, indicating that P. canaliculata may have gradually adapted to the high temperature environment over a long-term treatment.
This study showed that the growth rate of snail length in the control group was significantly greater than that in the high and low temperature groups, and the growth rate and specific growth rate for snail weight in the high temperature group and control group were significantly greater than those in the low temperature group, (Tables 1, 2), consistent with previous studies (Seuffert et al., 2010). In addition, the increased growth rate of snail length of female P. canaliculata in the low temperature group was significantly greater than that of male P. canaliculata (Tables 1, 2), which may be closely related to the higher plant digestibility and foraging rate of female snails (Tamburi and Martín, 2009; Xu et al., 2011).
Effect of temperature on the intestinal microbiome of Pomacea canaliculata
The structural composition of the gut microbiota of aquatic organisms is affected by ambient temperature (Hagi et al., 2004; Pierce et al., 2016). The survival rate of the mussel Mytilus galloprovincialis significantly decreased under high temperature stress at 27°C, while the diversity of its gut microbiota increased significantly (Li et al., 2019). In our study, both high and low temperature stress led to an increase in the diversity index of the intestinal microbiome of P. canaliculata (Figures 2A,B). It is important to note that the increased diversity of the intestinal microbiome is often beneficial for animals to cope with changes in the external environment and maintain the dynamic balance of the intestinal microenvironment (Sommer et al., 2017), indicating that the increase in the alpha diversity index may be related to the adaptation to the stress environment of P. canaliculata.
The gut microbiome of aquatic animals is quite susceptible to temperature (Sepulveda and Moeller, 2020). The gut bacterial community structure of Mytilus coruscus was significantly different at 27 and 31°C, and the main bacterial species were strongly affected by temperature (Li et al., 2018). When comparing the gut microbiota of Chanos chanos during 3 weeks of control (26°C) and elevated temperature (33°C) treatments, significant difference was observed soon after the temperature was increased, but tended to become similar, and no significant difference was observed at the end of the experiment. Changes in the gut microbiota due to heat stress can contribute to host adaptation to high temperatures (Hassenrück et al., 2020). In this study, temperature also had a significant effect on the gut microbiota structure of P. canaliculata. The hierarchical clustering analysis of samples based on the Bray-Curtis distance algorithm showed that the high temperature group was clustered alone and separated from the low temperature and control groups (Figure 5A). While there was no obvious separation between the low temperature and control group, which may be due to the low temperature stress of 15°C having no obvious effect on P. canaliculata (Pan et al., 2008). However, there were significant differences in the gut microbiota structure of P. canaliculata after 14 days (Figures 6A,B) and 28 days (Figures 6C,D) of stress at different temperatures. Similar results reported in the sea urchin (Echinometra sp. EZ) with gut microbiota varying across thermally variable habitats and displaying temporal shifts correlated with temperature (Ketchum et al., 2021).
Although temperature caused significant changes in the gut microbiota structure of P. canaliculata, the composition of its core microbiota was not greatly affected, and only their relative abundances were changed. The dominant core microbiota at the phylum level in all samples included Proteobacteria, Bacteroidetes, and Firmicutes (Figure 4A). The dominant core microbiota at the genus level included Aeromonas, Lactococcus, and Cloacibacterium (Figure 4B), suggesting that the core microbiota play a critical role in maintaining the basic physiological functions of the snail.
At the phylum level, most bacteria in Proteobacteria are facultative anaerobes and primarily pathogenic bacteria, which may lead to intestinal inflammation (Lupp et al., 2007). The increased abundance of Proteobacteria is often closely related to energy imbalance and instability in the host gut microbiota, which usually occur in the process of host metabolic disorders (Shin et al., 2015). In a study of the insect gut microbiota, elevated temperature was found to be associated with an increase in the relative abundance of Proteobacteria (Sepulveda and Moeller, 2020). For example, in the intestinal microbiota of Porcellio scaber, the relative abundance of Actinobacteria decreased with increasing temperature, and the relative abundance of Proteobacteria increased with increasing temperature (Horváthová et al., 2019). In contrast to insects, a positive correlation between the relative abundance of Proteobacteria and temperature has rarely been observed in mollusks (Sepulveda and Moeller, 2020). In this study, the relative abundance of Proteobacteria in the high temperature group was significantly greater than that in the low temperature group, and the relative abundance of Proteobacteria in the low temperature group was significantly greater than that in the control group (Figure 7B), indicating that the high and low temperature environments can lead to a stress response in P. canaliculata, resulting in the enrichment of pathogenic bacteria and a change in the intestinal microbiota structure. Bacteroidetes are mainly responsible for promoting carbohydrate fermentation and are involved in a variety of functions, such as polysaccharide, bile acid, and steroid metabolism as well as the maintenance of the intestinal microbiota balance (Sears, 2005). Inflammation of the gut is often accompanied by a significant reduction in the abundance of Bacteroidetes (Scaldaferri et al., 2013). This result was also confirmed in the study of the gut microbiota of the rainbow trout (Oncorhynchus mykiss) (Zhou et al., 2022) and mussel (M. galloprovincialis) (Li et al., 2019) under acute temperature stress. The relative abundance of Bacteroidetes in the high and low temperature groups in this study was significantly lower than that in the control group (Figure 7C), indicating that high and low temperature had adverse effects on the health status of P. canaliculata and could affect the maintenance of intestinal homeostasis and the development of host immunity (Colston and Jackson, 2016). Previous studies showed that increasing temperature tends to reduce the abundance of Firmicutes (Bestion et al., 2017; Zhu et al., 2019). A similar effect was observed in Lithobates pipiens, where the relative abundance of Firmicutes decreased significantly when the temperature increased from 18 to 28°C (Kohl et al., 2016). The mechanism by which the relative abundance of Firmicutes is negatively correlated with temperature is still unclear. It is speculated that the animal host may maintain the number of Firmicutes in the gut through active metabolism. Therefore, a metabolic disorder in the host caused by heat stress may lead to the prevalence of this phylum’s reduction (Sepulveda and Moeller, 2020). In this study, the relative abundance of Firmicutes in the high temperature group was significantly lower than that in the low temperature group and control group (Figure 7B), which is similar to previous conclusions and indicates that the sharp increase in temperature is likely to inhibit the growth of Firmicutes in the intestinal tract, causing the occurrence of inflammation and resulting in the death of P. canaliculata.
Lactococcus was found to be one of the dominant genera in the intestinal lactic acid microbiota of Cornu aspersum and Oncomelania hupensis (Koleva et al., 2014; Hao et al., 2020). Furthermore, the abundance of lactic acid bacteria (especially Lactococcus) in the gut microbiota of O. mykiss reduced significantly after 6 weeks of treatment under heat stress at 18°C (Huyben et al., 2018). This result is consistent with our results in this study that the relative abundance of Lactococcus in the high temperature group was significantly lower than that in the low temperature group and control group, presumably due to the increased temperature inhibiting the growth of Lactococcus (Figure 7C). Additionally, Clostridium has the ability to degrade cellulose and cellobiose (Kohl et al., 2016). In this study, this genus was significantly enriched in the high temperature group, which may play an important role in promoting the digestion and absorption of food by the host.
Temperature-induced changes in the gut microbiota may also lead to increase in pathogenic microbiota (Fontaine et al., 2018). Aeromonas is a gram-negative facultative anaerobic bacterium, most of which have been identified as pathogenic to some organisms, including fish (Dar et al., 2016) and shellfish (Chen J. S. et al., 2021; De Silva et al., 2021). The relative abundance of Aeromonas in the high temperature group was significantly higher than that in the low temperature and the control groups (Figure 7C). Enterobacter was also significantly enriched in the high temperature group. When the temperature changes sharply, the intestinal microbiota is disordered, and the abundance of pathogenic bacteria increases, which, to a certain extent, can explain the higher mortality rate of the snails in the high temperature group. Massive proliferation of opportunistic pathogens such as Vibrio and Arcobacter under heat stress conditions could increase the susceptibility of the host to disease and result in the mass death of mussels (Li et al., 2019).
Effect of temperature stress time on the intestinal microbiome of Pomacea canaliculata
The plasticity of gut microbial communities further helps species respond to new ecological challenges and adapt to new environments (Alberdi et al., 2016). Studies have shown that the gut microbiota of the invasive bullfrog (Lithobates catesbeianus) is more plastic than that of the green frog (Lithobates clamitans). Bullfrogs gut microbiota changes after only hours of warmer temperatures, whereas in green frogs this change occurs significantly after days (Fontaine and Kohl, 2020).
There were significant differences in the gut microbiota structure of P. canaliculata after 14 days of stress at different temperatures, and the differences in the structure of the gut microbiota were more obvious after 28 days of stress. According to the results of the LEfSe analysis, many pathogenic bacteria including Pseudomonas, Acinetobacter, Shewanella, and Flavobacterium were enriched after 14 days of temperature stress, which were significantly enriched in the high temperature group (H14) (Supplementary Figure 3B). Pathogenic bacteria such as Proteobacteria, Pseudomonas, and Bacteriovoracaceae were significantly enriched in the low temperature group (L14) (Supplementary Figure 3A). Some strains of Flavobacterium sp. have pathogenic effects on aquatic animals such as freshwater fish and marine fish (Bernardet, 2011), and the mortality rate of diseased fish can be as high as 100%, with symptoms such as surface ulcers, gill rot, and tissue necrosis.
After 28-days of temperature stress, the number of pathogenic bacteria decreased, and many beneficial bacteria were enriched, such as Lactococcus, Bacteroidetes, Firmicutes, etc. As common probiotics, they were significantly enriched in the later stages of the high and low temperature stress groups (H28 and L28) (Supplementary Figures 3A,B). Bacteroidetes are mainly involved in the degradation of carbohydrates, metabolism of sugars, and metabolism of substances such as bile acids, which are closely related to host health (Ghanbari et al., 2015). Firmicutes contribute to the maintenance of intestinal homeostasis and the development of host immunity (Colston and Jackson, 2016), and are more efficient than Bacteroidetes as an energy source, promoting caloric absorption and nutrient transport, thereby leading to weight gain (Zhu et al., 2021). The decrease of pathogenic bacteria and enrichment of a large number of probiotics in the later stage of temperature stress were highly consistent with the significant increase in the abundance of the intestinal microbiota of P. canaliculata over time. This finding indicates that the intestinal microbiome may have begun to adapt to the stress environment at the later stage of temperature stress. A similar trend was observed in the morphological analysis of P. canaliculata. After 21 days of high temperature stress, the survival rates of both female and male P. canaliculata were no longer significantly reduced, indicating that the surviving P. canaliculata may have gradually adapted to the high temperature environment under long-term treatment. Whether this is due to changes in the host’s physiological growth state caused by changes in the gut microbiota or the effect of the gut microbiota response to temperature on the health of the host remains unclear. Further studies are needed to clarify the relationship between the host and gut microbiome as it pertains to temperature stress response.
Differences in the intestinal microbiome between male and female Pomacea canaliculata
Previous studies have shown that sex is also one of the important factors affecting the gut microbiome of animals. The gut microbiota diversity of the male large-finned mudskipper (Periophthalmus magnuspinnatus) was significantly higher than that of females (Ma et al., 2018). Also, the microbial communities of male and female blackhead minnows (Pimephales promelas) were significantly segregated according to beta diversity metrics (Debofsky et al., 2020). When comparing with the effects of temperature and stress time on the intestinal microbiome of P. canaliculata, the differences in the intestinal microbiota between male and female P. canaliculata were smaller, but still significant. There was no significant difference in the alpha diversity index of the intestinal microbiota between female and male P. canaliculata (P > 0.05), but the ANOSIM analysis results based on the Bray-Curtis distance algorithm still showed significant differences between females and males in the high, low temperature and control groups.
According to the LEfSe analysis results, Enterobacter and Aeromonas were significantly enriched in male P. canaliculata after 28 days of low temperature stress (Supplementary Figure 4A). Proteobacteria and Enterobacteriaceae were also significantly enriched in male P. canaliculata after 28 days of high temperature stress (Supplementary Figure 4B). Proteobacteria, Enterobacteriaceae, Enterobacter, and Aeromonas are all opportunistic pathogens. The increased numbers of these opportunistic pathogens greatly increase the risk of bacterial infection in male P. canaliculata, which may be a reason why male snails are more susceptible to heat stress (Song et al., 2014). In addition, Bacteroidetes, Fusobacterium, Bacteroides and other species closely related to cellulose decomposition bacteria were significantly enriched in female P. canaliculata in high temperature and control groups, which may be closely related to the higher plant digestion ability and foraging rate of female P. canaliculata (Tamburi and Martín, 2009; Xu et al., 2011).
Conclusion
In this study, 16S rRNA high-throughput sequencing technology was used to study the growth, development and intestinal microbiome of P. canaliculata. The results showed that long-term high and low temperature stress had negative effects on the health status of P. canaliculata. Both high and low temperature stress had a significant impact on the intestinal microbiome of P. canaliculata, resulting in a significant increase in diversity and enrichment of pathogenic bacteria. The NMDS analysis showed that after 14 days of temperature stress, the intestinal microbiome structure of P. canaliculata was significantly changed to adapt to adverse environments including prolonged stress time, increased abundance of beneficial bacteria increased, and decreased opportunistic pathogens.
Data availability statement
The datasets presented in this study can be found in online repositories. The names of the repository/repositories and accession number(s) can be found in NCBI BioProject PRJNA843708.
Ethics statement
The animal study was reviewed and approved by the Animal Research Ethics Committee of Nanjing Normal University (Permit No. IACUC-20220267).
Author contributions
LC conceived the study. SL, ZQ, SG, and WS performed the experiments. SL and ZQ analyzed the data and drafted the manuscript under guidance of LC and HL. XL, HL, and LC revised the manuscript. All authors reviewed the manuscript.
Funding
This work was supported by the National Natural Science Foundation of China (No.32170434), Postgraduate Research and Practice Innovation Program of Jiangsu Province (No. KYCX22_1613), and the Priority Academic Program Development of Jiangsu Higher Education Institutions (PAPD).
Conflict of interest
The authors declare that the research was conducted in the absence of any commercial or financial relationships that could be construed as a potential conflict of interest.
Publisher’s note
All claims expressed in this article are solely those of the authors and do not necessarily represent those of their affiliated organizations, or those of the publisher, the editors and the reviewers. Any product that may be evaluated in this article, or claim that may be made by its manufacturer, is not guaranteed or endorsed by the publisher.
Supplementary material
The Supplementary Material for this article can be found online at: https://www.frontiersin.org/articles/10.3389/fmicb.2022.961502/full#supplementary-material
Supplementary Figure 1 | Rarefaction Curve for all sample. HF14, LF14, CF14, HM14, LM14, and CM14 represent the female high temperature group, female low temperature group, female control group, male high temperature group, male low temperature group, and male control group treated for 14 days, respectively. HF28, LF28, CF28, HM28, LM28, and CM28 represent the female high temperature group, female low temperature group, female control group, male high temperature group, male low temperature group, and male control group treated for 28 days, respectively.
Supplementary Figure 2 | Non-metric multidimensional scaling (NMDS) analysis based on (A,C,E) unweighted-UniFrac distances and (B,D,F) weighted-UniFrac distances plots of female Pomacea canaliculata intestine microbial communities at the different stress time in control group, high and low temperature groups, respectively. NMDS analysis based on (G,I,K) unweighted-UniFrac distances and (H,J,L) weighted-UniFrac distances plots of male P. canaliculata intestine microbial communities at the different stress time in control group, high and low temperature groups, respectively.
Supplementary Figure 3 | LEfSe analysis of Pomacea canaliculata intestinal microbiota composition at different experimental time points under low (A) and high temperature (B) stress.
Supplementary Figure 4 | LEfSe analysis of female and male Pomacea canaliculata intestine microbiome under (A) high temperature, (B) low temperature, and (C) control.
Supplementary Table 1 | Survival rate of female and male Pomacea canaliculata at different temperature and treated time.
Supplementary Table 2 | Mantel tests for the correlations between Pomacea canaliculata intestine microbiota and the different temperature groups using Spearman’s coefficients. The C, H, and L represented the control group, high and low temperature groups treated for 14 and 28 days, respectively. C14, H14, and L14 represent the control group, high and low temperature groups treated for 14 days, respectively. C28, H28, and L28 represent the control group, high and low temperature groups treated for 28 days, respectively.
Supplementary Table 3 | Mantel tests for the correlations between Pomacea canaliculata intestine microbiota and the different experimental time points using Spearman’s coefficients. C14, H14, and L14 represent the control group, high and low temperature groups treated for 14 days, respectively. C28, H28, and L28 represent the control group, high and low temperature groups treated for 28 days, respectively.
Supplementary Table 4 | Mantel tests for the correlations between female, male Pomacea canaliculata intestine microbiota and the different temperature groups using Spearman’s coefficients. CF, HF, and LF represent the female control group, female high temperature group and female low temperature group treated for 14 and 28 days, respectively. CM, HM, and LM represent the male control group, male high temperature group and male low temperature group treated for 14 and 28 days, respectively.
Footnotes
References
Alberdi, A., Aizpurua, O., Bohmann, K., Zepeda-Mendoza, M. L., and Gilbert, M. T. P. (2016). Do vertebrate gut metagenomes confer rapid ecological adaptation? Trends Ecol. Evol. 31, 689–699. doi: 10.1016/j.tree.2016.06.008
Bae, M. J., Kim, E. J., and Park, Y. S. (2021). Comparison of invasive apple snail (Pomacea canaliculata) behaviors in different water temperature gradients. Water 13:1149. doi: 10.3390/w13091149
Bernardet, J. F. (2011). “Family I. Flavobacteriaceae Reichenbach 1992,” in Bergey’s Manual of Systematic Bacteriology, 2nd Edn, ed. W. Whitman (Baltimore: The Williams & Wilkins Co.), 106–111.
Bernatis, J. L., Mcgaw, I. J., and Cross, C. L. (2016). Abiotic tolerances in different life stages of apple snails Pomacea canaliculata and Pomacea maculata and the implications for distribution. J. Shellfish Res. 35, 1013–1025. doi: 10.2983/035.035.0424
Bestion, E., Jacob, S., Zinger, L., Di Gesu, L., Richard, M., White, J., et al. (2017). Climate warming reduces gut microbiota diversity in a vertebrate ectotherm. Nat. Ecol. Evol. 1:161. doi: 10.1038/s41559-017-0161
Bokulich, N. A., Kaehler, B. D., Rideout, J. R., Dillon, M., Bolyen, E., Knight, R., et al. (2018). Optimizing taxonomic classification of marker-gene amplicon sequences with QIIME 2’s q2-feature-classifier plugin. Microbiome 6:90. doi: 10.1186/s40168-018-0470-z
Bolyen, E., Rideout, J. R., Dillon, M. R., Bokulich, N. A., Abnet, C. C., Al-Ghalith, G. A., et al. (2019). Reproducible, interactive, scalable and extensible microbiome data science using QIIME2. Nat. Biotechnol. 37, 852–857. doi: 10.1038/s41587-019-0209-9
Callahan, B. J., Mcmurdie, P. J., Rosen, M. J., Han, A. W., Johnson, A. J. A., and Holmes, S. P. (2016). Dada2: High-resolution sample inference from Illumina amplicon data. Nat. Methods 13, 581–583. doi: 10.1038/nmeth.3869
Cardoso, A. M., Cavalcante, J. J. V., Cantão, M. E., Thompson, C. E., Flatschart, R. B., Glogauer, A., et al. (2012). Metagenomic analysis of the microbiota from the crop of an invasive snail reveals a rich reservoir of novel genes. PLoS One 7:e48505. doi: 10.1371/journal.pone.0048505
Chandler, J. A., James, P. M., Jospin, G., and Lang, J. M. (2014). The bacterial communities of Drosophila suzukii collected from undamaged cherries. PeerJ 2:e474. doi: 10.7717/peerj.474
Chen, J. S., Hsu, G. J., Hsu, B. M., Yang, P. Y., Kuo, Y. J., Wang, J. L., et al. (2021). Prevalence, virulence-gene profiles, antimicrobial resistance, and genetic diversity of human pathogenic Aeromonas spp. from shellfish and aquatic environments. Environ. Pollut. 287:117361. doi: 10.1016/j.envpol.2021.117361
Chen, L., Li, S. X., Xiao, Q., Lin, X. X., Qu, Y. F., Wu, G. G., et al. (2021). Composition and diversity of gut microbiota in Pomacea canaliculata in sexes and between developmental stages. BMC Microbiol. 21:200. doi: 10.1186/s12866-021-02259-2
Clarke, K. R. (1993). Non-parametric multivariate analyses of changes in community structure. Aust. J. Ecol. 18, 117–143. doi: 10.1111/j.1442-9993.1993.tb00438.x
Colston, T. J., and Jackson, C. R. (2016). Microbiome evolution along divergent branches of the vertebrate tree of life: What is known and unknown. Mol. Ecol. 25, 3776–3800. doi: 10.1111/mec.13730
Cowie, R. H. (2002). “Apple snails (Ampullariidae) as agricultural pests: their biology, impacts and management,” in Molluscs as Crop Pests, ed. G. M. Barker (Wallingford: CAB International), 145–189.
Dao, R., Wu, D. X., Wang, H., Jin, H., Li, L., Fu, X. Q., et al. (2021). Exploration of the characteristics of intestinal microbiota and metabolomics in different rat models of Mongolian medicine. Evid. Complement. Alternat. Med. 2021:5532069. doi: 10.1155/2021/5532069
Dar, G. H., Dar, S. A., Kamili, A. N., Chishti, M. Z., and Ahmad, F. (2016). Detection and characterization of potentially pathogenic Aeromonas sobria isolated from fish Hypophthalmichthys molitrix (Cypriniformes: Cyprinidae). Microb. Pathog. 91, 136–140. doi: 10.1016/j.micpath.2015.10.017
Davidson, A. M., Jennions, M., and Nicotra, A. B. (2011). Do invasive species show higher phenotypic plasticity than native species and, if so, is it adaptive? A meta-analysis. Ecol. Lett. 14, 419–431. doi: 10.1111/j.1461-0248.2011.01596.x
De Silva, L. A. D. S., Wickramanayake, M. V. K. S., and Heo, G. J. (2021). Virulence and antimicrobial resistance potential of Aeromonas spp. associated with shellfish. Lett. Appl. Microbiol. 73, 176–186. doi: 10.1111/lam.13489
Debofsky, A., Xie, Y. W., Grimard, C., Alcaraz, A. J., Brinkmann, M., Hecker, M., et al. (2020). Differential responses of gut microbiota of male and female fathead minnow (Pimephales promelas) to a short-term environmentally-relevant, aqueous exposure to benzo[a]pyrene. Chemosphere 252:126461. doi: 10.1016/j.chemosphere.2020.126461
Dixon, P. (2003). VEGAN, a package of R functions for community ecology. J. Veg. Sci. 14, 927–930. doi: 10.1111/j.1654-1103.2003.tb02228.x
Flint, H. J., Bayer, E. A., Rincon, M. T., Lamed, R., and White, B. A. (2008). Polysaccharide utilization by gut bacteria: Potential for new insights from genomic analysis. Nat. Rev. Microbiol. 6, 121–131. doi: 10.1038/nrmicro1817
Fontaine, S. S., and Kohl, K. D. (2020). Gut microbiota of invasive bullfrog tadpoles responds more rapidly to temperature than a noninvasive congener. Mol. Ecol. 29, 2449–2462. doi: 10.1111/mec.15487
Fontaine, S. S., Novarro, A. J., and Kohl, K. D. (2018). Environmental temperature alters the digestive performance and gut microbiota of a terrestrial amphibian. J. Exp. Biol. 221:jeb187559. doi: 10.1242/jeb.187559
Gao, Y., Fu, J. S., Drake, J. B., Liu, Y., and Lamarque, F. J. (2012). Projected changes of extreme weather events in the eastern United States based on a high resolution climate modeling system. Environ. Res. Lett. 7:044025. doi: 10.1088/1748-9326/7/4/044025
Ghanbari, M., Kneifel, W., and Domig, K. J. (2015). A new view of the fish gut microbiome: Advances from next-generation sequencing. Aquaculture 448, 464–475. doi: 10.1016/j.aquaculture.2015.06.033
Hagi, T., Tanaka, D., Iwamura, Y., and Hoshino, Y. (2004). Diversity and seasonal changes in lactic acid bacteria in the intestinal tract of cultured freshwater fish. Aquaculture 234, 335–346. doi: 10.1016/j.aquaculture.2004.01.018
Hall, A. B., Tolonen, A. C., and Xavier, R. J. (2017). Human genetic variation and the gut microbiome in disease. Nat. Rev. Genet. 18, 690–699. doi: 10.1038/nrg.2017.63
Hao, Y. W., Guan, W., Wu, H. N., Li, L. H., Abe, E. M., Xue, J. B., et al. (2020). Intestinal microbiome profiles in Oncomelania hupensis in mainland China. Acta. Trop. 201:105202. doi: 10.1016/j.actatropica.2019.105202
Hassenrück, C., Reinwald, H., Kunzmann, A., Tiedemann, I., and Gärdes, A. (2020). Effects of Thermal stress on the gut microbiome of juvenile milkfish (Chanos chanos). Microorganisms 9:5. doi: 10.3390/microorganisms9010005
Hayes, K. A., Burks, R. L., Castro-Vazquez, A., Darby, P. C., Heras, H., Martín, P. R., et al. (2015). Insights from an integrated view of the biology of apple snails (Caenogastropoda: Ampullariidae). Malacologia 58, 245–302. doi: 10.4002/040.058.0209
Hayes, K. A., Cowie, R. H., and Thiengo, S. C. (2009). A global phylogeny of apple snails: Gondwanan origin, generic relationships, and the influence of outgroup choice (Caenogastropoda: Ampullariidae). Biol. J. Linn. Soc. 98, 61–76. doi: 10.1111/j.1095-8312.2009.01246.x
Hayes, K. A., Joshi, R. C., Thiengo, S. C., and Cowie, R. H. (2008). Out of South America: Multiple origins of non-native apple snails in Asia. Divers. Distributions 14, 701–712. doi: 10.1111/j.1472-4642.2008.00483.x
Heiler, K. C. M., Von Oheimb, P. V., Ekschmitt, K., and Albrecht, C. (2008). Studies on the temperature dependence of activity and on the diurnal activity rhythm of the invasive Pomacea canaliculata (Gastropoda: Ampullariidae). Mollusca 26, 73–81.
Horváthová, T., Babik, W., Kozłowski, J., and Bauchinger, U. (2019). The loss of actinobacterial symbionts at elevated temperatures. J. Therm. Biol. 82, 222–228. doi: 10.1016/j.jtherbio.2019.04.015
Huyben, D., Sun, L., Moccia, R., Kiessling, A., Dicksved, J., and Lundh, T. (2018). Dietary live yeast and increased water temperature influence the gut microbiota of rainbow trout. J. Appl. Microbiol. 124, 1377–1392. doi: 10.1111/jam.13738
Jiang, W. W., Li, J. Q., Gao, Y. P., Mao, Y. Z., Jiang, Z. J., Du, M. R., et al. (2016). Effects of temperature change on physiological and biochemical responses of Yesso scallop, Patinopecten yessoensis. Aquaculture 451, 463–472. doi: 10.1016/j.aquaculture.2015.10.012
Júnior, E. S., De Barros, J. C. N., Paresque, K., and De Freitas, R. R. (2013). The effect of stocking density on the growth of apple snails native Pomacea bridgesii and exotic Pomacea lineata (Mollusca, Gastropoda). An. Acad. Bras. Ciênc. 85, 753–760. doi: 10.1590/S0001-37652013000200019
Kelley, A. L. (2014). The role thermal physiology plays in species invasion. Conserv. Physiol. 2:cou045. doi: 10.1093/conphys/cou045
Ketchum, R. N., Smith, E. G., Vaughan, G. O., McParland, D., Ai-Mansoori, N., Burt, J. A., et al. (2021). Unraveling the predictive role of temperature in the gut microbiota of the sea urchin Echinometra sp. EZ across spatial and temporal gradients. Mol. Ecol. 30, 3869–3881. doi: 10.1111/mec.15990
Kohl, K. D., Stengel, A., and Dearing, M. D. (2016). Inoculation of tannin-degrading bacteria into novel hosts increases performance on tannin-rich diets. Environ. Microbiol. 18, 1720–1729. doi: 10.1111/1462-2920.12841
Koleva, Z., Dedov, I., Kizheva, J., Lipovanska, R., Moncheva, P., and Hristova, P. (2014). Lactic acid microflora of the gut of snail Cornu aspersum. Biotechnol. Biotechnol. Equip. 28, 627–634. doi: 10.1080/13102818.2014.947071
Li, Y. F., Xu, J. K., Chen, Y. W., Ding, W. Y., Shao, A. Q., Liang, X., et al. (2019). Characterization of gut microbiome in the mussel Mytilus galloprovincialis in response to thermal stress. Front. Physiol. 10:1086. doi: 10.3389/fphys.2019.01086
Li, Y. F., Yang, N., Liang, X., Yoshida, A., Osatomi, K., Power, D., et al. (2018). Elevated seawater temperatures decrease microbial diversity in the gut of Mytilus coruscus. Front. Physiol. 9:839. doi: 10.3389/fphys.2018.00839
Lupp, C., Robertson, M. L., Wickham, M. E., Sekirov, I., Champion, O. L., Gaynor, E. C., et al. (2007). Host-Mediated Inflammation disrupts the intestinal microbiota and promotes the overgrowth of Enterobacteriaceae. Cell Host Microbe 2, 119–129. doi: 10.1016/j.chom.2007.08.002
Ma, R. R., Wang, S. K., Zhao, F., Xu, C., Ji, Y., Song, C., et al. (2018). Comparative study on intestinal bacterial communities of Boleophthalmus pectinirostris and Periophthalmus magnuspinnatus with different sexes and feeding strategies. Ann. Microbiol. 68, 123–133. doi: 10.1007/s13213-018-1324-4
Matsukura, K., and Wada, T. (2007). Environmental factors affecting the increase in cold hardiness in the apple snail Pomacea canaliculata (Gastropoda: Ampullariidae). Appl. Entomol. Zool. 42, 533–539. doi: 10.1303/aez.2007.533
Matsukura, K., Tsumuki, H., Izumi, Y., and Wada, T. (2009). Physiological response to low temperature in the freshwater apple snail, Pomacea canaliculata (Gastropoda: Ampullariidae). J. Exp. Biol. 212, 2558–2563. doi: 10.1242/jeb.031500
McFall-Ngai, M., Hadfield, M. G., Bosch, T. C. G., Carey, H. V., Domazet-Loso, T., and Douglas, A. E. (2013). Animals in a bacterial world, a new imperative for the life sciences. Proc. Natl. Acad. Sci. U.S.A. 110, 3229–3236. doi: 10.1073/pnas.1218525110
Paaijmans, K. P., Heinig, R. L., Seliga, R. A., Blanford, J. I., Blanford, S., Murdock, C. C., et al. (2013). Temperature variation makes ectotherms more sensitive to climate change. Glob. Change Biol. 19, 2373–2380. doi: 10.1111/gcb.12240
Pan, Y. Y., Dong, S. Z., and Yu, X. P. (2008). Effects of temperature stress on development, feeding and survival of the apple snail, Pomacea canaliculata (Lamarck). Acta Phytophylacica Sin. 35, 239–244. doi: 10.13802/j.cnki.zwbhxb
Pierce, M. L., Ward, J. E., Holohan, B. A., Zhao, X., and Hicks, R. (2016). The influence of site and season on the gut and pallial fluid microbial communities of the eastern oyster, Crassostrea virginica (Bivalvia, Ostreidae): Communitylevel physiological profiling and genetic structure. Hydrobiologia 765, 97–113. doi: 10.1007/s10750-015-2405-z
Quast, C., Pruesse, E., Yilmaz, P., Gerken, J., Schweer, T., Yarza, P., et al. (2013). The SILVA ribosomal RNA gene database project: Improved data processing and web-based tools. Nucleic Acids Res. 41, 590–596. doi: 10.1093/nar/gks1219
Ruppert, K., Geiβ, C., Ostermann, S., Theis, C., and Oehlmann, J. (2016). Comparative sensitivity of juvenile and adult Potamopyrgus antipodarum (Mollusca: Hydrobiidae) under chronic exposure to cadmium and tributyltin. J. Environ. Sci. Health A Tox. Hazard. Subst. Environ. Eng. 51, 736–743. doi: 10.1080/10934529.2016.1170443
Scaldaferri, F., Gerardi, V., Lopetuso, L. R., Del Zompo, F., Mangiola, F., Boškoski, I., et al. (2013). Gut microbial flora, prebiotics, and probiotics in IBD: Their current usage and utility. Biomed. Res. Int. 2013:435268. doi: 10.1155/2013/435268
Sears, C. L. (2005). A dynamic partnership: Celebrating our gut flora. Anaerobe 11, 247–251. doi: 10.1016/j.anaerobe.2005.05.001
Sepulveda, J., and Moeller, A. H. (2020). The effects of temperature on animal gut microbiomes. Front. Microbiol. 11:384. doi: 10.3389/fmicb.2020.00384
Seuffert, M. E., and Martín, P. R. (2013). Juvenile growth and survival of the apple snail Pomacea canaliculata (Caenogastropoda: Ampullariidae) reared at different constant temperatures. Springerplus 2:312. doi: 10.1186/2193-1801-2-312
Seuffert, M. E., and Martín, P. R. (2017). Thermal limits for the establishment and growth of populations of the invasive apple snail Pomacea canaliculata. Biol. Invasions 19, 1169–1180. doi: 10.1007/s10530-016-1305-0
Seuffert, M. E., Burela, S., and Martín, P. R. (2010). Influence of water temperature on the activity of the freshwater snail Pomacea canaliculata (Caenogastropoda: Ampullariidae) at its southernmost limit (Southern Pampas, Argentina). J. Therm. Biol. 35, 77–84. doi: 10.1016/j.jtherbio.2009.11.003
Shin, N. R., Whon, T. W., and Bae, J. W. (2015). Proteobacteria: Microbial signaturc of dysbiosis in gut microbiota. Trends Biotechnol. 33, 496–503. doi: 10.1016/j.tibtech.2015.06.011
Sommer, F., Anderson, J. M., Bharti, R., Raes, J., and Rosenstiel, P. (2017). The resilience of the intestinal microbiota influences health and disease. Nat. Rev. Microbiol. 15, 630–638. doi: 10.1038/nrmicro.2017.58
Song, H. M., Mu, X. D., Gu, D. E., Luo, D., Yang, Y. X., Xu, M., et al. (2014). Molecular characteristics of the HSP70 gene and its differential expression in female and male golden apple snails (Pomacea canaliculata) under temperature stimulation. Cell Stress Chaperones 9, 579–589. doi: 10.1007/s12192-013-0485-0
Tamburi, N. E., and Martín, P. R. (2009). Feeding rates and food conversion efficiencies in the apple snail Pomacea canaliculata (Caenogastropoda: Ampullariidae). Malacologia 51, 221–232. doi: 10.4002/040.051.0201
Warton, D. I., Wright, S. T., and Wang, Y. (2012). Distance-based multivariate analyses confound location and dispersion effects. Methods Ecol. Evol. 3, 89–101. doi: 10.1111/j.2041-210X.2011.00127.x
Xu, W. B., Zhong, Q. H., Li, L. F., Zhang, J. E., Luo, M. Z., Zhuo, B. L., et al. (2011). Differences of female and male Pomacea canaliculata in foraging and heterosexual choice. Chin. J. Ecol. 30, 2528–2533. doi: 10.13292/j.1000-4890.2011.0374
Yang, Q. Q., Liu, S. W., He, C., and Yu, X. P. (2018). Distribution and the origin of invasive apple snails, Pomacea canaliculata and P. maculata (Gastropoda: Ampullariidae) in China. Sci. Rep. 8:1185. doi: 10.1038/s41598-017-19000-7
Yoshida, K., Matsukura, K., Cazzaniga, N. J., and Wada, T. (2014). Tolerance to low temperature and desiccation in two invasive apple snails, Pomacea canaliculata and P. maculata (Caenogastropoda: Ampullariidae), collected in their original distribution area (northern and central Argentina). J. Mollus. Stud. 80, 62–66. doi: 10.1093/mollus/eyt042
Yusa, Y., Sugiura, N., and Wada, T. (2006). Predatory potential of freshwater animals on an invasive agricultural pest, the apple snail Pomacea canaliculata (Gastropoda: Ampullariidae), in southern Japan. Biol. Invasions 8, 137–147. doi: 10.1007/s10530-004-1790-4
Zhao, R., Symonds, J. E., Walker, S. P., Steiner, K., Carter, C. G., Bowman, J. P., et al. (2021). Effects of feed ration and temperature on Chinook salmon (Oncorhynchus tshawytscha) microbiota in freshwater recirculating aquaculture systems. Aquaculture 543:736965. doi: 10.1016/j.aquaculture.2021.736965
Zheng, G., Dong, S., Hou, Y., Yang, K., and Yu, X. (2012). Molecular characteristics of HSC70 gene and its expression in the golden apple snails, Pomacea canaliculata (Mollusca: Gastropoda). Aquaculture 15, 41–49. doi: 10.1016/j.aquaculture.2012.06.002
Zhou, C. Q., Yang, S. W., Ka, W., Gao, P., Li, Y. L., Long, R. J., et al. (2022). Association of gut microbiota with metabolism in rainbow trout under acute heat stress. Front. Microbiol. 13:846336. doi: 10.3389/fmicb.2022.846336
Zhu, L., Liao, R., Wu, N., Zhu, G., and Yang, C. (2019). Heat stress mediates changes in fecal microbiome and functional pathways of laying hens. Appl. Microbiol. Biotechnol. 103, 461–472. doi: 10.1007/s00253-018-9465-8
Keywords: Pomacea canaliculata, temperature, 16S rRNA, microbiome, invasive snail
Citation: Li S, Qian Z, Gao S, Shen W, Li X, Li H and Chen L (2022) Effect of long-term temperature stress on the intestinal microbiome of an invasive snail. Front. Microbiol. 13:961502. doi: 10.3389/fmicb.2022.961502
Received: 10 June 2022; Accepted: 28 July 2022;
Published: 29 August 2022.
Edited by:
Huan Li, Lanzhou University, ChinaReviewed by:
Lin Zhang, Hubei University of Chinese Medicine, ChinaDeli Xu, Qufu Normal University, China
Yanfeng Sun, Agricultural University of Hebei, China
Copyright © 2022 Li, Qian, Gao, Shen, Li, Li and Chen. This is an open-access article distributed under the terms of the Creative Commons Attribution License (CC BY). The use, distribution or reproduction in other forums is permitted, provided the original author(s) and the copyright owner(s) are credited and that the original publication in this journal is cited, in accordance with accepted academic practice. No use, distribution or reproduction is permitted which does not comply with these terms.
*Correspondence: Hong Li, bmpsaWhvbmdAb3V0bG9vay5jb20=; Lian Chen, Y2hlbmxpYW5fMjAwNEAxNjMuY29t
†These authors have contributed equally to this work