- 1School of Biological Sciences, The University of Utah, Salt Lake City, UT, United States
- 2Department of Microbiology, Weber State University, Ogden, UT, United States
- 3Earth-Life Science Institute, Tokyo Institute of Technology, Tokyo, Japan
- 4Department of Geosciences, Smith College, Northampton, MA, United States
- 5School of Marine and Atmospheric Sciences, Stony Brook University, Stony Brook, NY, United States
- 6Department of Biology, University of Naples “Federico II”, Naples, Italy
- 7Center for Sustainable Resource Science, RIKEN, Saitama, Japan
Strýtan Hydrothermal Field (SHF) is a submarine system located in Eyjafördur in northern Iceland composed of two main vents: Big Strýtan and Arnarnesstrýtan. The vents are shallow, ranging from 16 to 70 m water depth, and vent high pH (up to 10.2), moderate temperature (Tmax ∼70°C), anoxic, fresh fluids elevated in dissolved silica, with slightly elevated concentrations of hydrogen and methane. In contrast to other alkaline hydrothermal vents, SHF is unique because it is hosted in basalt and therefore the high pH is not created by serpentinization. While previous studies have assessed the geology and geochemistry of this site, the microbial diversity of SHF has not been explored in detail. Here we present a microbial diversity survey of the actively venting fluids and chimneys from Big Strýtan and Arnarnesstrýtan, using 16S rRNA gene amplicon sequencing. Community members from the vent fluids are mostly aerobic heterotrophic bacteria; however, within the chimneys oxic, low oxygen, and anoxic habitats could be distinguished, where taxa putatively capable of acetogenesis, sulfur-cycling, and hydrogen metabolism were observed. Very few archaea were observed in the samples. The inhabitants of SHF are more similar to terrestrial hot spring samples than other marine sites. It has been hypothesized that life on Earth (and elsewhere in the solar system) could have originated in an alkaline hydrothermal system, however all other studied alkaline submarine hydrothermal systems to date are fueled by serpentinization. SHF adds to our understandings of hydrothermal vents in relationship to microbial diversity, evolution, and possibly the origin of life.
Introduction
Alkaline submarine hydrothermal vents have been proposed as potential sites for the origin of life on Earth (and potentially elsewhere in the solar system) because they promote strong proton and redox gradients and facilitate the abiotic synthesis of organic molecules (Russell, 2009; Russell et al., 2010; Price et al., 2017). Specifically, the geochemistry of these systems appears to provide a template for autotrophic metabolic pathways (McCollom and Seewald, 2007; Proskurowski et al., 2008; Hudson et al., 2020), such as the Wood-Ljungdahl pathway shared by methanogens, acetogens, and sulfate-reducers (Martin and Russell, 2007; Adam et al., 2018; Hudson et al., 2020), which may be one of the most ancient metabolic pathways (Ward and Shih, 2019). Previous work on submarine alkaline hydrothermal vents has focused on systems fueled by serpentinization, such as Lost City (Kelley et al., 2005; Lang and Brazelton, 2020; Brazelton et al., 2022), Prony (Monnin et al., 2014; Quéméneur et al., 2014; Postec et al., 2015), and Old City hydrothermal fields (Lecoeuvre et al., 2021). While incredibly valuable, these studies have left many open questions about how opportunities for microbial life may differ in alkaline hydrothermal vents with different geological bases.
Strýtan Hydrothermal Field (SHF), while highly alkaline, is not a serpentinizing system, providing a point of comparison to other alkaline sites. Fresh groundwater is heated, enriched in silica, and brought to high pH before venting into the bottom of the marine Eyjafördur. As the hydrothermal and surrounding marine waters mix, they precipitate magnesium silicate minerals which form chimneys rising up to 55 meters from the seafloor (Figure 1) and create steep sodium ion gradients. Harnessing energy as ion gradients across membranes is as universal as the genetic code (Lane and Martin, 2012). As a result, environments with these types of gradients may be important for considering the origin of life (Price et al., 2017); the Na+ cell membrane pump is crucial for many ancient microbial lineages, including methanogens and acetogens, and is involved in adenosine triphosphate (ATP) synthesis and carbon assimilation in diverse organisms (Lane and Martin, 2012; Branscomb et al., 2017). Although not a focus of this study, it is fascinating to consider an energy source invoked in origin of life theory as possibly being operative in today’s microbial communities.
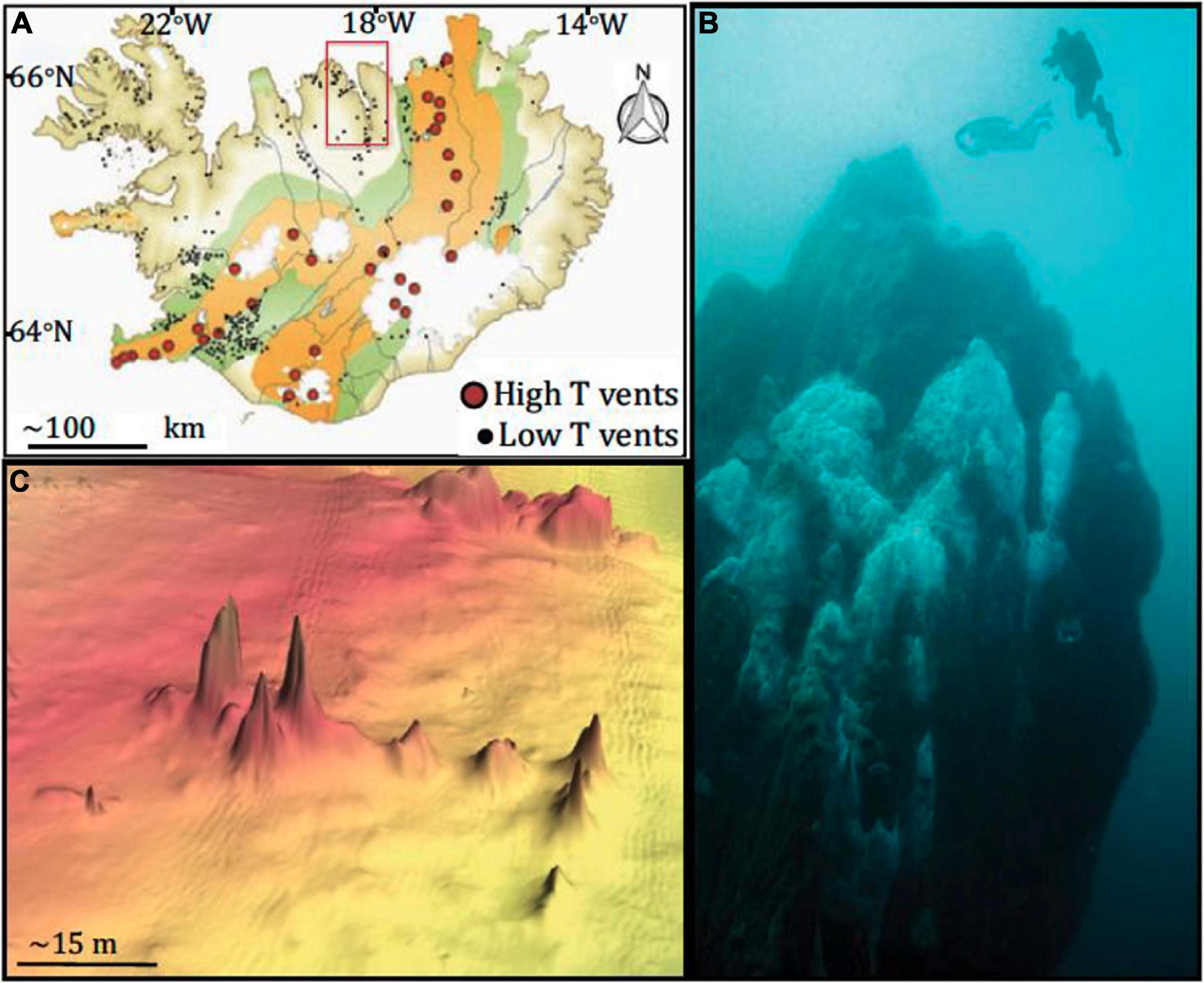
Figure 1. Map of sampling site and vents. (A) Map of Iceland, highlighting hydrothermal vents. Eyjafjördur, where Big Strýtan and Arnarnesstrýtan are located, is highlighted by the red box; (B) photograph of Big Strýtan chimney (courtesy of E. Bogason); (C) bathymetric map of Arnarnesstrýtan. Figure from Price et al. (2017). The figure is reprinted with permission under a Creative Commons CC-BY license.
Very little microbiological research has been conducted at SHF. In a study from 2001, researchers exploring vent fluids isolated Aquificales and Korarchaeota from clone libraries (Marteinsson et al., 2001b). However, a full census of microbial diversity at SHF has not been previously conducted. By characterizing the microbial diversity of vent fluids and mineral precipitates at SHF, we can better understand the ability of alkaline hydrothermal systems, and this unique site in particular, to support microbial communities and better constrain their potential roles in the origin of life.
Materials and methods
Sample collection
In August 2017, we performed a 7-day field-sampling campaign to SHF, where scientific SCUBA divers collected actively venting fluids from both Big Strýtan and Arnarnesstrýtan using 30 m of cleaned polypropylene tubing by swimming to the appropriate sampling location and holding the tubing inlet in the actively venting water. On the boat, the tubing was connected to two 12V inline electric galley pumps to pull water from the vent. During sample collection, pH and temperature were monitored to ensure the capture of vent fluids, rather than background seawater. Vent fluid samples for DNA analyses were collected in triple-rinsed 20 L carboys and background seawater samples were collected in sterilized 4 L carboys. A field station laboratory was established in the port dive shop, where collected fluid samples were filtered through 0.22 μm Sterivex cartridge filters (Millipore, MA, United States) using a peristaltic pump and flash-frozen in liquid N2.
Hydrothermal vent chimney samples from Big Strýtan and Arnarnesstrýtan were collected by scientific divers by breaking off small (∼15 cm × 5 cm) pieces into sterile Whirl-pak bags (Nasco, WI, United States). Back in the field-station laboratory, the chimney samples were subsampled into distinct chimney layers (Supplementary Figures 1, 2) using a flame-sterilized scalpel, and these solid samples were immediately frozen in liquid N2. All frozen filter and chimney samples were shipped back to the University of Utah in dry shippers and stored at –80°C until nucleic acid extraction.
DNA extraction
DNA was extracted from the Sterivex filter cartridges using a lab protocol previously developed for low-biomass alkaline fluid samples (Brazelton et al., 2022). Briefly, cell lysis was performed by alternating freeze/thaw cycles in an alkaline buffer, followed by purification with phenol-chloroform and precipitation with ethanol. Additionally, a laboratory extraction blank (ICEd062, Supplementary Table 1), consisting of a new Sterivex filter, was extracted alongside the fluid samples to track any potential laboratory contamination.
DNA was extracted from ∼0.5 to 1.0 g of homogenized chimney samples using the MP Biomedical FastDNA Spin kit (CA, United States), per the manufacturer’s instructions, with the exception of the use of a Mini-Beadbeater-16 (Biospec Products, OK, United States) in place of the recommended FastPrep Instrument. Technical replicates were pooled, to a total starting mass of 1.5–3.5 g per chimney sample, using an Amicon Ultra-2 Centrifugal Filter Unit with Ultracel-30 membrane (Millipore, Darmstadt, Germany). All DNA samples were purified using AMPure XP speedbeads (Rohland and Reich, 2012) and quantified via the Qubit high-sensitivity DNA assay (Invitrogen, CA, United States). A list of all DNA samples included in the study can be found in Supplementary Table 1.
16S rRNA sequencing and data analysis
DNA samples were submitted to the Michigan State University Research and Technology Support Facility Genomics Core for sequencing of the V4 region of the 16S rRNA gene on an Illumina MiSeq platform using the universal duel-indexed Illumina fusion primers 515F-806R (Kozich et al., 2013). Amplicon concentrations were normalized and pooled using an Invitrogen SequalPrep DNA Normalization Plate. After library quality control and quantitation, the pool was loaded on an Illumina MiSeq v2 flow cell and sequenced using a standard 500 cycle reagent kit. Base calling was performed by Illumina Real Time Analysis (RTA) software v1.18.54. Output of RTA was demultiplexed and converted to fastq files using Illumina Bcl2fastq v1.8.4.
16S rRNA gene amplicon sequences were processed with cutadapt v. 1.15 (Martin, 2011) and DADA2 v. 1.10.1 (Callahan et al., 2016), including quality trimming and filtering of reads, removal of chimeras, and inference of amplicon sequence variants (ASVs). Taxonomic classification of all ASVs was performed with DADA2 using the SILVA reference alignment (SSURef v138) and taxonomy outline (Pruesse et al., 2012) with the default minimum bootstrap value of 50 for taxonomic assignments (Callahan et al., 2016).
Quality control of sequence data was performed by removing putative contaminant sequences from the dataset. For vent fluid and background seawater samples, which were collected by filtration through Sterivex filters, a laboratory extraction blank (ICEd062; Supplementary Table 1) was sequenced and the 73 ASVs detected in that extraction blank were removed from the 8 vent fluid samples and 2 background seawater samples. Chimney samples were extracted via a DNA extraction kit, and 203 ASVs belonging to the genera outlined in Sheik et al. (2018) as putative kit contaminants were removed from the 12 chimney samples. A detailed list of all taxa removed from the dataset can be found in Supplementary Table 2. Additionally, any sequences classified to Domain “NA” or “Eukaryota” and all Bacteria classified as “Chloroplast” or “Mitochondria” were removed from the dataset.
After removing potential contaminant sequences, raw counts were converted to proportions to normalize for variations in sequencing depth among samples. The proportional abundances of all 872 unique ASVs among all seawater, vent fluid, and chimney samples were used to calculate the Morisita-Horn community dissimilarity between each pair of samples. Similar results were obtained with other metrics of dissimilarity (e.g., Bray-Curtis, Sørensen). The multi-dimensional scaling (MDS) plot was generated from a table of ASV proportional abundances across all sample categories (vent fluids, chimneys, and background seawater) using the distance, ordinate, and plot_ordination commands in the R package phyloseq v.1.26.1 (McMurdie and Holmes, 2013). All sequence data is available in the NCBI Sequence Read Archive (SRA) under BioProject PRJNA861241.
Comparison to other high pH vents
We cross-referenced the taxa detected in previous studies of alkaline submarine vents (Quéméneur et al., 2014; Postec et al., 2015; Lecoeuvre et al., 2021; Brazelton et al., 2022) and alkaline hot springs (Reysenbach et al., 1994; Marteinsson et al., 2001a; Blank et al., 2002; Nakagawa and Fukui, 2003; Purcell et al., 2007; Boomer et al., 2009; Meyer-Dombard et al., 2011; Tobler and Benning, 2011; Meyer-Dombard and Amend, 2014; López-López et al., 2015; Martinez et al., 2019) with the abundant (>1% of any sample) taxa detected in this study (Supplementary Table 3). For studies conducted with clone-libraries, we included all reported taxa (Reysenbach et al., 1994; Marteinsson et al., 2001a; Blank et al., 2002; Nakagawa and Fukui, 2003; Purcell et al., 2007; Boomer et al., 2009; Meyer-Dombard et al., 2011; Tobler and Benning, 2011; Meyer-Dombard and Amend, 2014; Quéméneur et al., 2014; Postec et al., 2015), while for studies conducted with amplicon and/or metagenomic sequencing, we included taxa representing a relative abundance of >1% of any sample (López-López et al., 2015; Martinez et al., 2019; Lecoeuvre et al., 2021; Brazelton et al., 2022). Similarities between phylum through genus for Archaeon and class through genus for Bacteria were included with the lowest common taxonomic level reported in Supplementary Table 3.
Additionally, the only previous microbiological study of SHF was published in 2001 (Marteinsson et al., 2001b) using Sanger sequencing of clone libraries. Given that microbial taxonomy is ever evolving, in order to compare the taxonomy of these previously detected microorganisms to our current data, we classified the sequences reported in Marteinsson et al. (2001a) by searching them against the current (November 2021) NCBI nr database using the BLASTn function (Altschul et al., 1990). Data originally reported in Marteinsson et al. (2001a) and the updated taxonomic assignments can be found in Supplementary Table 4.
Aqueous geochemistry
Fluid samples were collected directly from the tubes through which microbial samples were taken. Temperature was measured in situ with a Thermo Fisher Scientific temperature probe in an underwater housing constructed at the Max Planck Institute for Marine Microbiology. pH was measured in the field just after surfacing from each dive using a Hanna HALO Bluetooth pH meter with temperature compensation. Samples for major anion concentrations were preserved in the field by 0.2 μm syringe filtered into acid washed HDPE Nalgene® bottles and frozen until analysis. Analyses were performed in the Wehrmann Biogeochemistry Laboratory, School of Marine and Atmospheric Sciences, Stony Brook University using a Metrohm 930 compact ion chromatograph with matrix elimination. Samples for major cation concentrations were preserved in the field by 0.2 μm syringe filtered into acid washed HDPE Nalgene® bottles, acidified to 0.1% acid with ultrapure nitric acid, and frozen until analysis. Analyses were performed in the Black Paleooceanography Laboratory, School of Marine and Atmospheric Sciences, Stony Brook University using a Horiba Ultima 2C inductively coupled plasma–optical emission spectrometer (ICP-OES).
Results
Geochemistry of Strýtan Hydrothermal Field
Strýtan Hydrothermal Field is located in Eyjafördur in northern Iceland and consists of two main vent sites: Big Strýtan and Arnarnesstrýtan (Figure 1). Each site consists of several large (up to 55 m tall) chimneys in shallow water (<60 m depth) composed of saponite, a magnesium silicate material with basaltic host rock (Geptner et al., 2002; Price et al., 2017). The chimneys vent pH 10.2 freshwater that is enriched in dissolved silicate and slightly elevated in H2 (0.1–5.2 μM) and methane (0.5–1.4 μM) (Price et al., 2017). In contrast to other alkaline hydrothermal vents, the Strýtan Hydrothermal Field is hosted in basalt and therefore the high pH is not created by serpentinization but rather a combination of (1) plagioclase hydrolysis, coupled with calcite precipitation, and (2) hydration of Mg in pyroxene and olivine in basalt (Geptner et al., 2002; Price et al., 2017). SHF vent fluids are sourced in groundwater, and the steep sodium ion gradients from the fluids at SHF (from <3 to 468 mM), may be important for understanding processes involved in the origin of life (Lane and Martin, 2012; Price et al., 2017).
Five types of samples were collected during our expedition: (1) vent fluids from Big Strýtan vent, (2) chimney samples from Big Strýtan vent, (3) vent fluids from Arnarnesstrýtan vent, (4) chimney samples from Arnarnesstrýtan vent, and (5) background seawater samples, each of which harbored distinct microbial communities (Figure 2). The chimney samples from the two vents, while distinct from one another, were more similar to each other than to any of the fluid samples and all the vent and chimney samples were distinct from the background seawater (Figure 2).
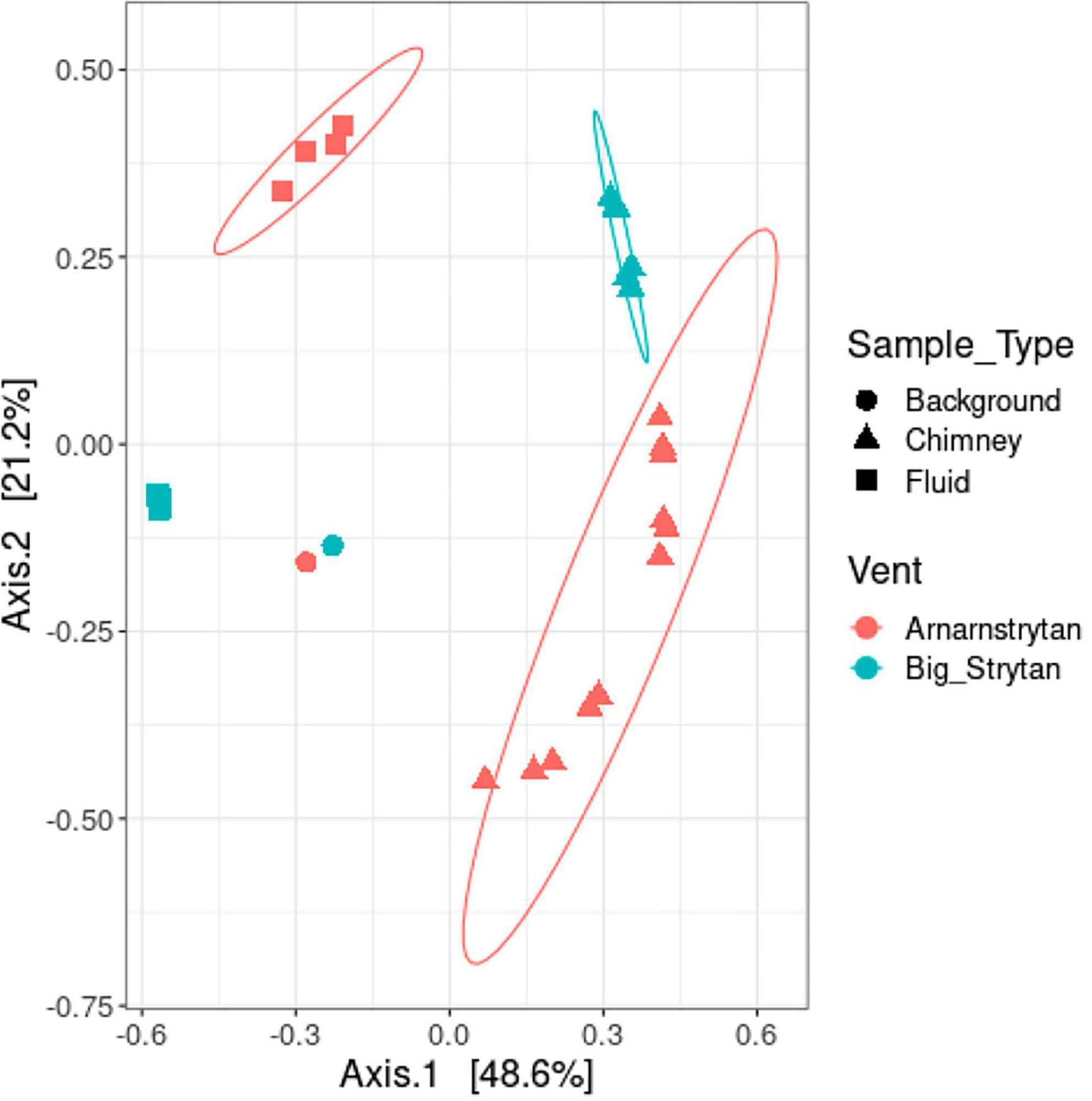
Figure 2. Distinct microbial communities between sample types (background seawater, chimney, and vent fluids) and different vents (Big Strýtan, pink; Arnarnesstrýtan, teal). Multi-dimensional scaling plot of community dissimilarity, calculated with the Morisita-Horn dissimilarity index.
The background seawater sample exhibited chemical characteristics typical of seawater (e.g., pH 8, mM concentrations of ions; Table 1; Pinet, 2019). The chemistry of the vent fluids from Big Strýtan and Arnarnesstrýtan were similar, with high pH (∼10) and generally low in dissolved ions. This is in agreement with previous studies (Marteinsson et al., 2001b; Price et al., 2017). The sample from Arnarnesstrýtan had slightly higher dissolved ions, likely as a result of mixing with seawater, either deeper in the hydrothermal edifice or during sampling. Of particular interest, H2S and Fe were slightly elevated in the vent fluids relative to seawater. While the relative contributions to the H2S content of microbial SO42– reduction and mobilization from subsurface basalts during water-rock reactions has not been confirmed, the abundance of known dissimilatory sulfate reducing microorganisms in the vent fluids (such as Thermodesulfovibrionales, e.g., Sekiguchi et al., 2008; Figure 3) does suggests a role for biological sulfur cycling.
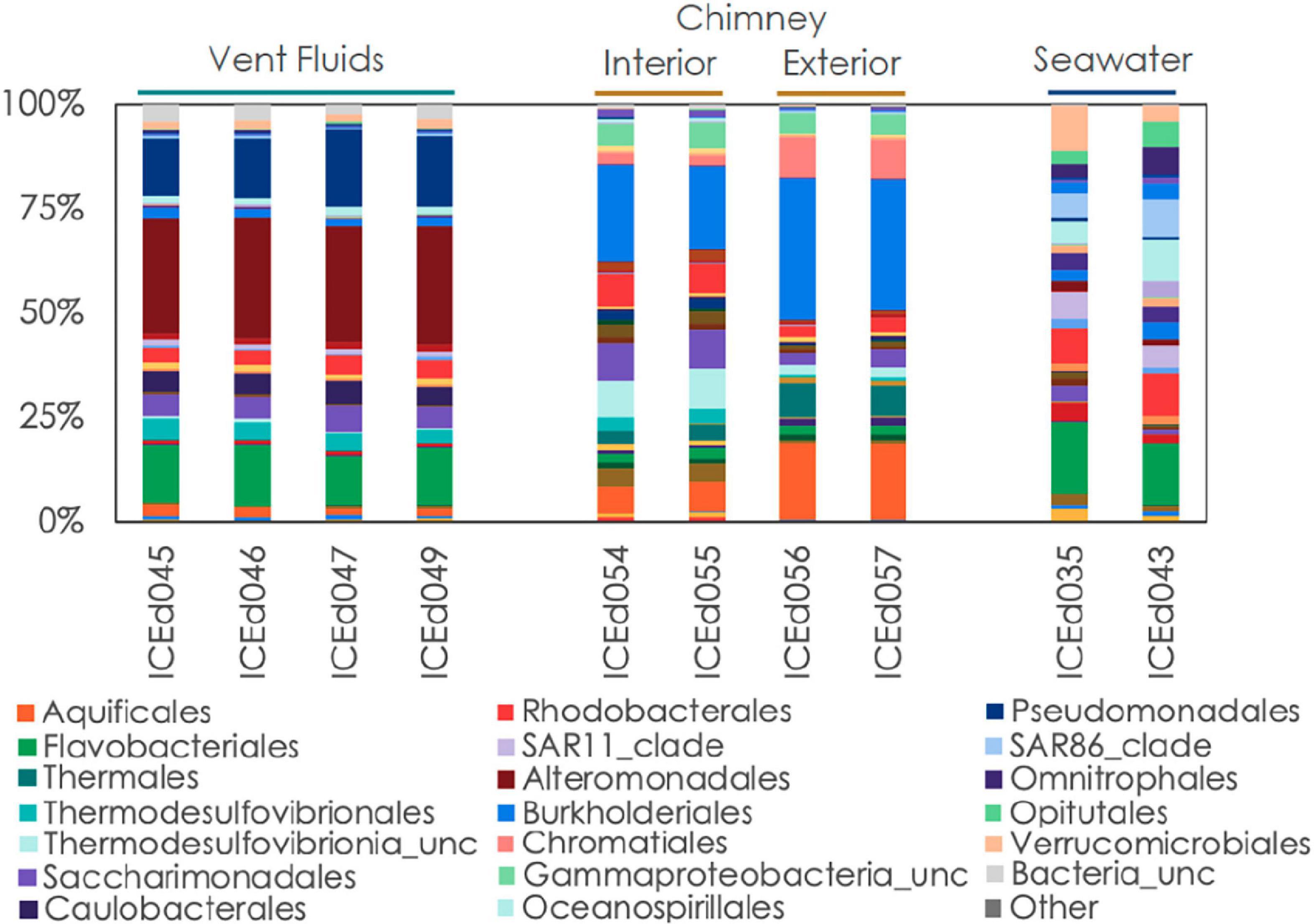
Figure 3. Relative abundance of microbial communities in Big Strýtan vent fluids, chimneys, and background seawater at the order level.
Microbial community of Big Strýtan vent
The microbial communities between background seawater, vent fluids, and chimney material from the Big Strýtan site are distinct (Figure 3). Vent fluids from Big Strýtan were dominated by members of the orders Alteromonadales (29.6%), Pseudomonadales (19.1%), Flavobacteriales (14.9%), Saccharimonadales (6.4%), Caulobacterales (6.0%), and Thermodesulfovibrionales (5%; Figure 3). Two of the three most abundant ASVs in Big Strýtan vent fluids both belonged to the genus Pseudoalteromonas within the class Alteromonadales. The best hit for ASV sq4, which made up 18–21% of the vent fluid community, was Pseudoalteromonas carrageenovora, isolated from extremely deep marine sediments from the Challenger Deep (Chen et al., 2021; Table 2). ASV sq11, classified as Pseudoalteromonas and comprising 5–7% of the vent fluids, had a best hit from a recently formed, circumneutral hydrothermal vent (García-Davis et al., 2021; Table 2). There were five ASVs belonging to the Pseudomonadales in Big Strýtan vent fluids (Supplementary Table 3). The third most abundant ASV in Big Strýtan vent fluids was sq31 (7.8%), which was classified as Acinetobacter sp. and has 100% similarity to a sequence from a subsurface aquifer (Table 2; Espín et al., 2020). Two other ASVs belonging to the Pseudomonadales were classified at least the genus level and their best hits were also from hydrothermal vents: ASV sq63 (3.3%) is 100% similar to Psychrobacter sp. found at the recently formed vent referenced above (García-Davis et al., 2021) and ASV sq113 (2.2%) is 99.6% similar to Acinetobacter johnsonii (unpublished; Supplementary Table 3). The two ASVs of Flavobacterium spp. had best hits that originated in Arctic sediments (F. petrolei, Chaudhary et al., 2019) or volcanic soil samples (F. degerlachei, Zeglin et al., 2016; Supplementary Table 3). Two ASVs (sq28, sq112) classified as Thermodesulfovibrio were close matches to sequences found in terrestrial alkaline hot spring samples from Iceland (Marteinsson et al., 2001a). While the orders Saccharimonadales and Caulobacterales made up 6.4 and 6.0% of the vent fluids, respectively (Figure 3), no individual ASV belonging to these orders was >1% of any sample.
We subsampled a piece of chimney from Big Strýtan into interior and exterior sections for microbial analyses (Supplementary Figure 1). The interior of the Big Strýtan chimney samples, which were in direct contact with actively venting fluids, were dominated by members of the Burkholderiales (22.1%), Thermodesulfovibrionales (9.1%), Saccharimonadales (8.9%), Rhodobacteriales (7.4%), and Aquificales (6.8%) (Figure 3). The exterior of the chimney samples, which were in contact with surrounding seawater, were dominated by Burkholderiales (33.4%), Aquificales (18.2%), and Chromatiales (9.7%) (Figure 3). The most abundant ASV was sq2_Sutterellaceae (Burkholderiales), which accounted for 16.6% of the inner layers and 25.6% of the outer layers of the chimney (Table 2) and had a best hit match from a circumneutral hot spring in Hungary (Anda et al., 2014). ASV sq3, identified as Thermocrinis albus (Aquificales) and first isolated from Hvergerthi in Iceland (Eder and Huber, 2002), was 6.3% and 17.4% of the interior and exterior of Big Strýtan chimney samples, respectively. ASV sq16 was classified to the class Thermodesulfovibrionia and was only 95.7% similar to the best hit match in the NCBI SRA database, which was a sequence from an alkaline spring at Yellowstone National Park (Boomer et al., 2009; Table 2). Finally, ASV sq17, comprising 9.6% of the exterior chimney, was classified as Candidatus Thiobios (Chromatiales) and had a best hit to a sequence from marine sediments (Table 2).
The background seawater samples, which were collected on two separate dives (Supplementary Table 1) shared marked similarities in their community composition and were dominated by members of the Flavobacteriales (17.4%), Rhodobacterales (10.4%), Verrucomicrobiales (10.7%), Oceanospirillales (10.3%), SAR86 (9.5%), Opitutales (6.6%), Omnitrophales (6.6%), and SAR11 (6.5%) (Figures 3, 4). It is important to note that the genera within these orders were distinct from members found in the vent or chimney samples (Supplementary Table 3).
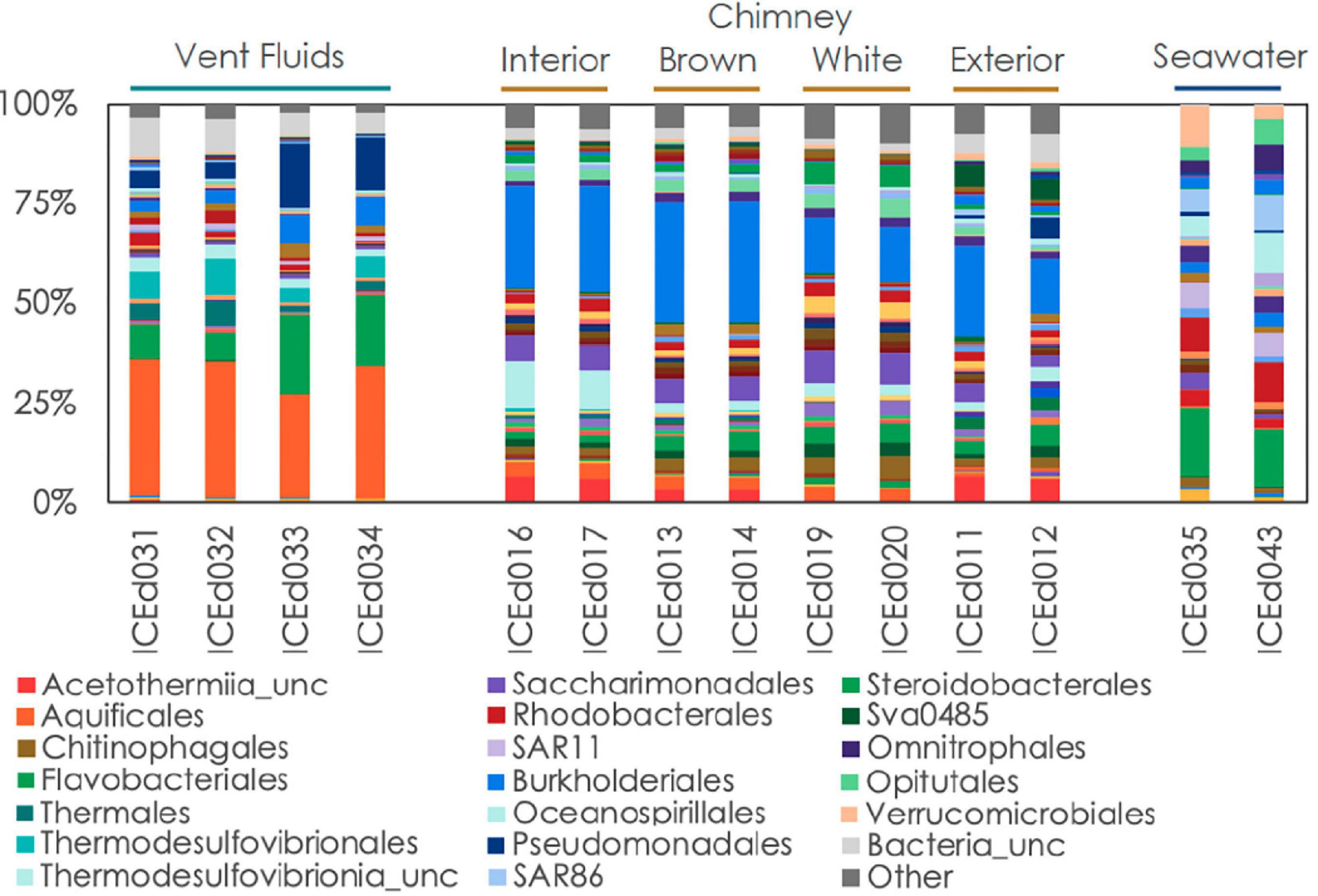
Figure 4. Relative abundance of microbial communities in Arnarnesstrýtan vent fluids, chimneys, and seawater at the order level.
Microbial community of Arnarnesstrýtan vent
Even at the order level of taxonomy, distinct communities were observed in Arnarnesstrýtan vent fluids, chimney samples, and background seawater (Figure 4). Vent fluids from Arnarnesstrýtan were dominated by members of the orders Aquificales (33.9%), Flavobacteriales (19.5%), Pseudomonadales (16.3%), Thermodesulfovibrionales (9%), Burkholderiales (7.5%), and Thermales (6.4%), as well as a significant proportion of unclassified Bacteria (9.7%) (Figure 4). There were six ASVs belonging to the Aquificales, two of which were “uncultured” Thermocrinis sp. and four classified as Thermocrinis albus (Table 3). The best hits of all six sequences were from previous studies conducted in alkaline Icelandic hot springs (Marteinsson et al., 2001a; Wirth et al., 2010; Tobler and Benning, 2011), though not specifically at SHF. Three ASVs of Flavobacterium spp. all had best hits that originated in Arctic sediments (F. petrolei, Chaudhary et al., 2019) or thawing permafrost samples (F. psychrolimnae, Aszalós et al., 2020 and F. degerlachei, Zeglin et al., 2016) (Table 3 and Supplementary Table 3). Pseudomonas extremaustralis (sq55) was also detected in Arnarnesstrýtan vent fluids and has previously been observed in other alkaline hot springs (Table 3; Wang and Pecoraro, 2021). Furthermore, this ASV (Sq55_Pseduomonas) was not detected in background seawater samples. There were two ASVs of Thermodesulfovibrio (sq28, sq60), both of which were previously detected in alkaline hot springs in Iceland (Marteinsson et al., 2001a; Supplementary Table 3). ASV sq14 was classified as Thermus islandicus and was 100% similar to the type strain, isolated from a hot spring in southern Iceland (Bjornsdottir et al., 2009).
The Arnarnesstrýtan chimney exhibited distinct color zones and was subsampled to assess the microbial diversity of each zone independently (Supplementary Figure 2). The interior-most zone (gray) was in direct contact with venting fluids and expected to be a more anoxic environment than the exterior-most zone (yellow), which was in direct contact with the surrounding seawater (Supplementary Figure 2). The interior Arnarnesstrýtan chimney samples were dominated by members of the Burkholderiales (25.9%), Thermodesulfovibrionia (11.6%), Acetothermiia (6.5%), and Saccharimonadales (5.8%) (Figure 4). Meanwhile, the exterior Arnarnesstrýtan chimney samples were dominated by Burkholderiales (13.7%), Acetothermiia (6.5%), Sva0485 (5.4%), and Pseudomonadales (5.0%) (Figure 4). Similar to the vent fluids, there was a significant proportion of unclassified Bacteria (7%) found in Arnarnesstrýtan chimney samples (Figure 4). Five ASVs from the class Burkholderiales were detected in the chimney samples. The most abundant was ASV sq2_Sutterellaceae, which accounted for 10–20% of the inner layers and 2–3% of the outer layers of the chimney (Table 3). ASV sq66 was identified as Hydrogenophaga and was abundant in the exterior layers (white, yellow; Supplementary Table 3). ASV sq7_Hydrogenophilaceae was detected at high abundance (4–7%) in all layers of the chimney sample (Table 3). Thermodesulfovibrio spp. were detected in Arnarnesstrýtan vent fluids and in the inner most chimney sample there were three ASVs of unclassified Thermodesulfovibrionia (same Class) that accounted for 7–10% of the replicate samples (Figure 4 and Table 3). Two of those ASVs (sq16, sq134) had sequence similarity of 95% with their closest BLAST hits, both of which were from an alkaline hot spring in Yellowstone National Park (Boomer et al., 2009; Supplementary Table 3). There were two ASVs identified as uncultured Acetothermiia. ASV sq32 was most abundant (4.5%) in the interior layer, while ASV sq41, the closest BLAST hit of which is also from alkaline vents in Iceland (Tobler and Benning, 2011), was abundant (5.9%) in the exterior layer of the chimney (Table 3 and Supplementary Table 3). Finally, an ASV classified as Sva0485 (phylum) was 6% of the exterior (yellow) layer and found in other studies looking at microbialite formation (Table 3; Corman et al., 2016; Couradeau et al., 2017). While the orders Saccharimonadales and Pseudomonadales made up 5.8 and 5.0% of the chimney samples, respectively (Figure 4), no individual ASV belonging to these orders was >1% of any sample. Only one ASV belonging to the domain Archaea accounted for >1% of any sample in the study. This was ASV sq175_Candidatus Nitrosopumilus, present in exterior Arnarnesstrýtan chimneys (Supplementary Table 3).
Discussion
Microbial ecology of Strýtan Hydrothermal Field
The vent fluids from both Big Strýtan and Arnarnesstrýtan contain an abundance of Flavobacterium, Pseudoalteromonas, Psychrobacter, Acinetobacter, and Pseudomonas species (Tables 2, 3 and Supplementary Table 3). Type strain members of these genera are all aerobic, heterotrophic bacteria (Akagawa-Matsushita et al., 1992; Bozal et al., 2003; Van Trappen et al., 2004, 2005; Chaudhary et al., 2019), with the exception of Pseudomonas extremaustralis, which is capable of nitrate-reduction (López et al., 2009, 2017). Interestingly, almost all of the species detected exclusively in vent fluids are also found in Arctic and Antarctic samples (Bozal et al., 2003; Van Trappen et al., 2004, 2005; López et al., 2009; Chaudhary et al., 2019). None of these ASVs were detected in background seawater samples, so it is unlikely that the samples were “contaminated” by seawater. Instead, the vent fluid samples from Big Strýtan and Arnarnesstrýtan represent a mixing zone within the chimney, where deeply sourced hydrothermal fluids, enriched in H2, CH4, and H2S, come into contact with oxygenated seawater, creating a niche habitat for aerobic to microaerophilic organisms capable of H2 and H2S-oxidation.
Two ASVs were abundant in both vent fluids from Arnarnesstrýtan and exterior chimney samples from Big Strýtan, but not detected in the other samples. These belonged to Thermus islandicus (sq14) and Thermocrinis albus (sq3; Tables 2, 3 and Supplementary Table 3). Additional ASVs of Thermocrinis albus (sq15, sq20) were detected only in Arnarnesstrýtan vent fluids (Supplementary Table 3). The best hit matches for these four ASVs all originally come from alkaline geothermal vents in Iceland (Marteinsson et al., 2001a; Tobler and Benning, 2011). Thermus islandicus is a microaerophilic, mixotrophic, sulfur-oxidizer, originally isolated from a pH 6 hot spring in southern Iceland (Bjornsdottir et al., 2009). Thermocrinis albus belongs to the phylum Aquificota and is a microaerophilic chemolithoautotroph that uses the reverse-TCA cycle and is able to utilize H2, S0, and thiosulfate as electron donors (Eder and Huber, 2002; Hügler et al., 2007; Wirth et al., 2010). These microaerophilic, sulfur-oxidizing organisms, are likely inhabiting an oxic/anoxic mixing zone within Arnarnesstrýtan fluids and a low-oxygen cavity within the exterior of the Big Strýtan chimney. The chemistry of the fluids suggest that seawater was entrained slightly more for Arnarnesstrýtan compared to Big Strýtan. It is possible that this occurred prior to sampling, deeper within the chimney edifice. If true, then the entire edifice might have elevated O2 concentrations from seawater, which would help explain the presence of more aerobic metabolisms in Arnarnesstrýtan fluids compared to Big Strýtan.
Taxa with putatively diverse metabolisms were detected within the chimney samples from both vents. Interior chimney samples from both Big Strýtan and Arnarnesstrýtan had an abundance of Thermodesulfovibrionia (sq13, sq16, sq84, sq110, sq117, and sq134) and Sutterellaceae (sq2, sq38, sq78, sq87, sq92, and sq146; Tables 2, 3 and Supplementary Table 3). Members of both these groups are anaerobes and Thermodesulfovibrionia are capable of nitrate- and sulfate-reduction (Morotomi, 2014; Arshad et al., 2017; Umezawa et al., 2021). The exterior chimney samples from both vents contained Acetothermia (sq41; Table 3 and Supplementary Table 3), a phylum that is also referred to OP1 and Bipolaricaulota in the literature. Genomes of Acetothermia contain the complete Wood-Ljungdahl pathway, allowing for potential acetogenesis in these samples (Youssef et al., 2019). Various taxa putatively capable of carbon-fixation via the Calvin-Bassam-Benson cycle were detected in exterior samples from Big Strýtan (Ca. Thiobios) and Arnarnesstrýtan (Hydrogenophilaceae, Hydrogenophaga) chimney (Tables 2, 3 and Supplementary Table 3; Willems et al., 1989; Rinke et al., 2009; Orlygsson and Kristjansson, 2014). The apparent transition from Wood-Ljungdahl dominated autotrophy in interior samples to CBB-driven carbon fixation in the exterior may reflect the different sensitivities of these pathways to O2 concentration (e.g., Berg, 2011) across the chimney/seawater boundary. Additionally, the sulfate-reducing Ca. Desulforudis (sq149) was detected in exterior Arnarnesstrýtan chimney samples (Chivian et al., 2008). This organism has previously been shown to be capable of carbon fixation via the O2-sensitive Wood-Ljungdahl pathway (Karnachuk et al., 2019), suggesting low-oxygen conditions extended into at least some distal regions of the chimney interior. Interestingly, no phototrophic bacteria were detected, even in exterior chimney samples, despite the shallow water depth.
Our subsampling of the chimney samples (Supplementary Figures 1, 2) have allowed for us to distinguish oxic, low oxygen, and anoxic habitats within the chimneys. The inferred metabolisms suggest that O2 and perhaps SO42– are important electron acceptors, along with NO32–, whereas H2 and H2S may be the most important electron donors. It appears that sulfur metabolisms may play a crucial role. With the observation that O2 and SO42– may be entrained deeper within the system, H2S observed in the vent fluids may be produced by SO4 (or thiosulfate) reduction with H2. Support for this observation comes from Rucker et al. (2022), who conducted thermodynamic calculations of Gibbs free energy to evaluate the highest energy yielding reactions as a proxy for microbial metabolism. The most energetically favorable reactions in all mixing schemes for Strýtan includes CO2 or O2 reduction with H2, or O2 reduction with H2S oxidation. Numerous NO3– reduction reactions are also favorable, and NO3– reduction with H2S oxidation is among the “top five most” energetically yielding reactions.
As noted above, the only previous microbiological study conducted at SHF was published in 2001 and used cultivation-dependent and clone library methodologies (Marteinsson et al., 2001b). Of the ∼100 bacteria described in Marteinsson et al. (2001b), accession numbers were only available for eight of them. To compare the taxonomy of those eight sequences from 2001 to our current dataset, we performed a BLASTn search of the sequences against the current (Nov. 2021) NCBI SRA database (Supplementary Table 4). Most notable is the presence of Thermocrinis albus (referred to as Aquificales in Marteinsson et al., 2001b), which dominated clone-libraries in the 2001 study and accounted for 19 and 34% of the amplicon libraries from Big Strýtan chimney and Arnarnesstrýtan vent fluids, respectively, in the present study (Supplementary Table 3, 4). We similarly detected members of the Actinobacteriota, Nitrospirota, Caulobacteraceae, and Burkholderiales at SHF (Marteinsson et al., 2001b).
It should be noted that 16S rRNA derived discussion of metabolic capacity may not accurately represent what is happening in the natural environment. Future study of metagenomic and metranscriptomic data from this site will help clarify the metabolic strategies employed at SHF and the current work is a first insight.
Comparison of Strýtan Hydrothermal Field to other alkaline hydrothermal vent sites
To put the unique nature of Strýtan Hydrothermal Field into context, we compared the microbial composition reported here to the two most similar ecosystem classes–alkaline submarine hydrothermal vents and alkaline terrestrial hot springs. Aside from SHF, the only other studied alkaline submarine hydrothermal vents are hosted in ultramafic rocks, rather than basalt, and are fueled by serpentinization, which creates high pH and millimolar concentrations of hydrogen and methane (Table 4). Prony hydrothermal field (PHF), located in New Caledonia is a shallow vent (16–47 m) where freshwater fluids influenced by serpentinization mix with seawater (Table 4; Quéméneur et al., 2014; Postec et al., 2015). The Lost City and Old City hydrothermal fields, also fueled by serpentinization, are much deeper (700–900 m and 3,100 m, respectively) and the source water for the vents is marine, rather than freshwater (Table 4; Kelley et al., 2005; Lecoeuvre et al., 2021). While elevated compared to seawater, the H2 and CH4 concentrations at SHF are in the micromolar range and therefore not as elevated as is found at serpentinite-hosted sites (Table 4). Therefore, high pH is the strongest geochemical similarity between SHF and serpentinite-hosted hydrothermal vents.
Similarities between phylum through genus for the single observed Archeon and class through genus for Bacteria were included with the lowest common taxonomic level reported in Supplementary Table 3, with the closest matches highlighted in bright colors and higher (more distant) matches in faint colors. Commonalities between PHF and SHF include Acetothermia, Thermus, and Rhodocyclaceae (Gammaproteobacteria), as well as an unclassified bacterium, with 99% sequence similarity. Lost City and Old City hydrothermal fields shared Nitrosopumilaceae, Acetothermia, Thermodesulfovibrionia, Thiomicrospiraceaea, and various families belonging to the Bacteroidota and Gammaproteobacteria within SHF (Figure 5 and Supplementary Table 3). Notably, Flavobacterium and Thermocrinis abundant in SHF were absent in other alkaline submarine systems.
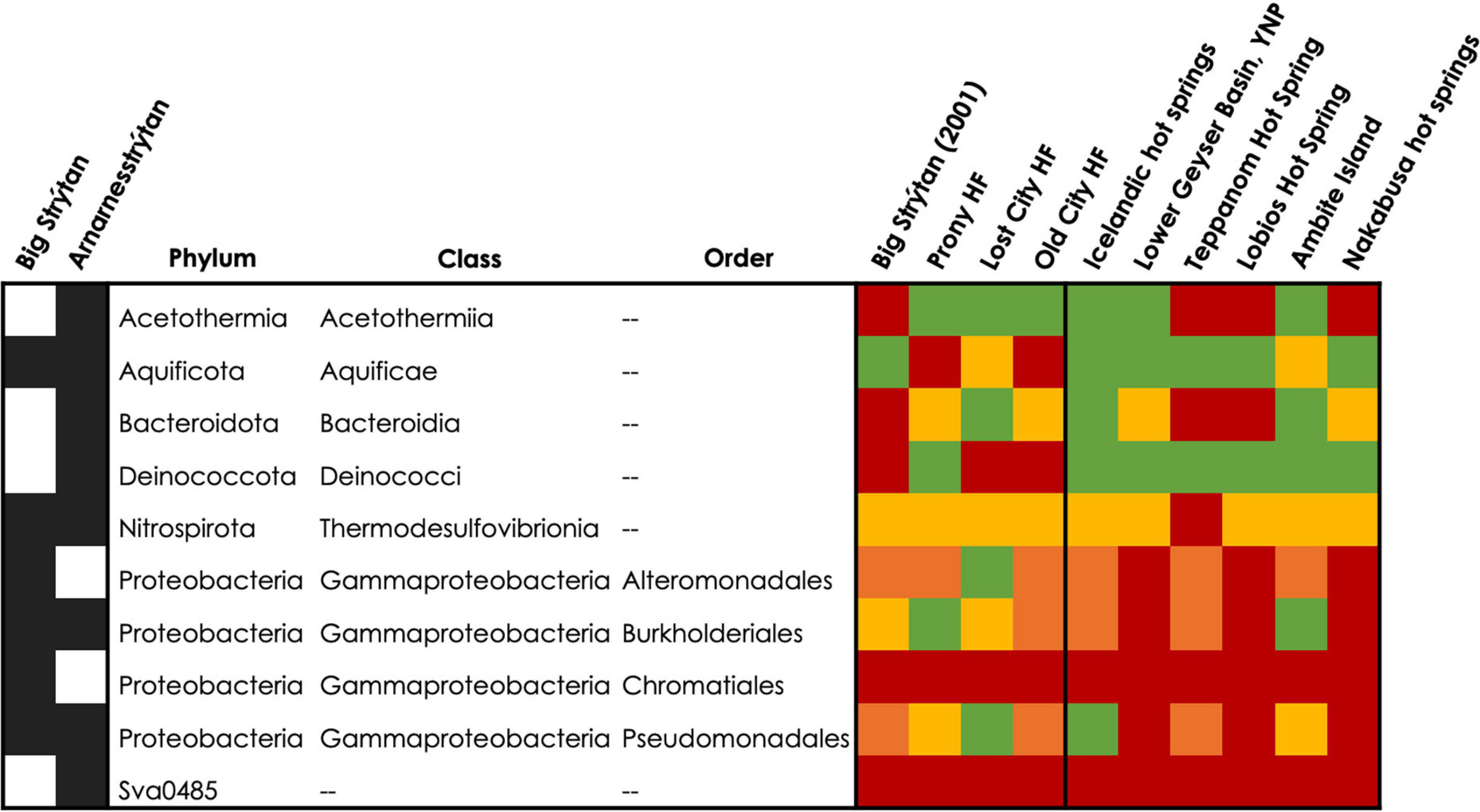
Figure 5. Detection of abundant taxa from SHF at other alkaline submarine hydrothermal vents and hot springs. Abundant ASVs (>5%) are listed at the class level, or order level for Proteobacteria. In the heatmap, the colors represent: red = not present, orange = phylum present (or class for Proteobacteria), yellow = class present (or order for Proteobacteria), and green = detected at a lower taxonomic level or the same level as in the current study. Additional details about these abundant ASV’s can be found in Tables 2, 3 and Supplementary Table 3.
Our BLASTn results of abundant (>5%) ASVs indicate that the inhabitants of SHF are actually more similar to terrestrial hot spring samples than to marine sites (Tables 2, 3). Therefore, we additionally compared our diversity data to alkaline terrestrial hot springs (Table 5 and Figure 5 and Supplementary Table 3), such as Lower Geyser Basin, Yellowstone National Park, United States, Teppanom Hot Spring in Thailand, Lobios Hot Spring, Spain, Ambitle Island in Papua New Guinea, Nakabusa Hot Springs, Japan, and geothermal springs from southern Iceland. Notably, SHF has a higher pH than these sites (with the exception of other Icelandic sites) and a potentially high energy mixing zone where geothermal waters come into contact with oxygenated seawater (Table 5).
Thermocrinis and Thermus are two hallmark genera of terrestrial hot spring systems and were detected in most of the hot spring sites compared here (Figure 5 and Supplementary Table 3; Reysenbach et al., 1994; Blank et al., 2002; Nakagawa and Fukui, 2002; 2003; Purcell et al., 2007; Boomer et al., 2009; Meyer-Dombard et al., 2011; Meyer-Dombard and Amend, 2014; López-López et al., 2015; Nishihara et al., 2018; Martinez et al., 2019). However the exact species detected at SHF, Thermocrinis albus and Thermus islandicus, were only previously found in other hot springs in Iceland (Figure 5 and Supplementary Table 3; Marteinsson et al., 2001a; Tobler and Benning, 2011). Additional taxa that were found in terrestrial hot springs and SHF include the Nitrosopumilus, Acetothermia, Thermodesulfovibrionia, and Desulfobacteriota. Abundant taxa that are unique to SHF, compared to alkaline submarine and terrestrial geothermal systems, include Thermoanaerobaculaceae, Hydrogenispora, Hydrogenophaga, Sutterellaceae, Ca. Alysiosphaera, Ca. Thiobios, SAR324, and Sva0485 (Figure 5 and Supplementary Table 3).
Conclusion
Strýtan Hydrothermal Field is a unique site, both geochemically and microbiologically. Close relatives to sequences found here have been described in submarine hydrothermal vents, terrestrial hot springs, Antarctica, the deep subsurface, and geothermal ground waters, suggesting it represents an amalgamation of various different environments and therefore a unique habitat. Community members from the vent fluids are mostly aerobic heterotrophic bacteria; however, within the chimneys we see taxa putatively capable of acetogenesis, sulfur-cycling, and hydrogen metabolism. Previous studies have hypothesized that alkaline vents, such as the ones described here could be a potential site for the origin of life on Earth (Russell, 2009; Russell et al., 2010). Additionally, the steep sodium gradient at SHF, which is not present at the other vents, could make it an even more likely analog for the cradle of life (Martin and Russell, 2007; Lane and Martin, 2012; Price et al., 2017). While the presence of O2 in seawater at SHF and other alkaline vents—and therefore a bioenergetically favorable oxygen gradient with reduced hydrothermal fluids—makes these sites inexact analogs for the early Earth, which was anoxic at the time of the origin of life (e.g., Farquhar et al., 2000; Johnson et al., 2014), this may actually position these systems as optimal analogs for habitable environments on other planets. In particular, both Mars (due to photochemistry and hydrogen escape) and Europa (due to radiolysis of surface ice followed by mixing with the subsurface ocean) possess now, and likely have for much of their history, O2 at concentrations that may be sufficient to support aerobic metabolisms (Hand et al., 2007; Lanza et al., 2016; Stamenković et al., 2018; Ward et al., 2019). Future metagenome-, metatranscriptome-, and culture-based work at SHF may therefore serve as an excellent natural laboratory to investigate the possibility of the origin and survival of life elsewhere in the solar system. Here we demonstrate that SHF is not only geochemically distinctive compared to other submarine hydrothermal vents, but also microbiologically unique. Future studies of the system may further help us to decipher the possible roles of both freshwater and marine alkaline hydrothermal systems in the origin of life.
Data availability statement
The data presented in this study are deposited in the NCBI SRA repository, accession number PRJNA861241.
Author contributions
KT, LW, WB, SM, RP, and DG contributed to the conception and design of the study. KT, LW, RP, DG, and SM participated in the sample collection and processing. KT, LW, ZK, AS, HP, RP, and WB contributed to the data analysis, curation, methodology, visualization, and validation. KT wrote the original draft. SM organized the field expedition and acquired the funding. All authors contributed to the interpretation, contributed to the review, editing, and approval of the final submitted version.
Funding
This research was supported in part by the ELSI Origins Network (EON), which was supported by a grant from the John Templeton Foundation and also partially supported by the NASA Astrobiology Institute Rock-Powered Life team and NASA Habitable Worlds program grant 80NSSC20K0228. SM acknowledges support from JSPS KAKENHI Grant No. JP20H00195 and RP received partial funding for this work from the Hanse-Wissenschaftskolleg Institute for Advanced Study, Delmenhorst, Germany.
Acknowledgments
Erlendur Bogason was instrumental in the work and we are grateful to the Strýtan Divecenter in Hjalkeyri, Iceland. Additionally, we thank Ryuhei Nakamura and Masahiro Yamamoto for their contributions during the field sampling expedition and Jake Lowe for his comments on the manuscript draft. We are grateful to the Icelandic National Energy Authority and Environment Agency for issuing permits for this work. We especially acknowledge members of the University of Akureyri for a warm welcome and laboratory support Profs. Hrei ar ór Valt sson, Jóhann Örlygsson, sta Margrét smundsdóttir, and Rannveig Björnsdóttir.
Conflict of interest
The authors declare that the research was conducted in the absence of any commercial or financial relationships that could be construed as a potential conflict of interest.
Publisher’s note
All claims expressed in this article are solely those of the authors and do not necessarily represent those of their affiliated organizations, or those of the publisher, the editors and the reviewers. Any product that may be evaluated in this article, or claim that may be made by its manufacturer, is not guaranteed or endorsed by the publisher.
Author disclaimer
The opinions expressed in this publication are those of the author(s) and do not necessarily reflect the views of EON or the John Templeton Foundation.
Supplementary material
The Supplementary Material for this article can be found online at: https://www.frontiersin.org/articles/10.3389/fmicb.2022.960335/full#supplementary-material
References
Adam, P. S., Borrel, G., and Gribaldo, S. (2018). Evolutionary history of carbon monoxide dehydrogenase/acetyl-CoA synthase, one of the oldest enzymatic complexes. Proc. Natl. Acad. Sci. U.S.A. 115, E1166–E1173. doi: 10.1073/pnas.1716667115
Akagawa-Matsushita, M., Matsuo, M., Koga, Y., and Yamasato, K. (1992). Alteromonas atlantica sp. nov. and Alteromonas carrageenovora sp. nov., bacteria that decompose algal polysaccharides. Int. J. Syst. Evol. Microbiol. 42, 621–627. doi: 10.1099/00207713-42-4-621
Altschul, S. F., Gish, W., Miller, W., Myers, E. W., and Lipman, D. J. (1990). Basic local alignment search tool. J. Mol. Biol. 215, 403–410. doi: 10.1016/S0022-2836(05)80360-2
Anda, D., Büki, G., Krett, G., Makk, J., Márialigeti, K., Erõss, A., et al. (2014). Diversity and morphological structure of bacterial communities inhabiting the Diana-Hygieia Thermal Spring (Budapest, Hungary). Acta Microbiol. Immunol. Hung. 61, 329–346. doi: 10.1556/amicr.61.2014.3.7
Arshad, A., Dalcin Martins, P., Frank, J., Jetten, M. S. M., Op den Camp, H. J. M., and Welte, C. U. (2017). Mimicking microbial interactions under nitrate-reducing conditions in an anoxic bioreactor: enrichment of novel Nitrospirae bacteria distantly related to Thermodesulfovibrio. Environ. Microbiol. 19, 4965–4977. doi: 10.1111/1462-2920.13977
Aszalós, J. M., Szabó, A., Megyes, M., Anda, D., Nagy, B., and Borsodi, A. K. (2020). Bacterial diversity of a high-altitude permafrost thaw pond located on ojos del salado (dry andes, altiplano-atacama region). Astrobiology 20, 754–765. doi: 10.1089/ast.2018.2012
Berg, I. A. (2011). Ecological aspects of the distribution of different autotrophic CO2 fixation pathways. Appl. Environ. Microbiol. 77, 1925–1936. doi: 10.1128/AEM.02473-10
Bjornsdottir, S. H., Petursdottir, S. K., Hreggvidsson, G. O., Skirnisdottir, S., Hjorleifsdottir, S., Arnfinnsson, J., et al. (2009). Thermus islandicus sp. nov., a mixotrophic sulfur-oxidizing bacterium isolated from the Torfajokull geothermal area. Int. J. Syst. Evol. Microbiol. 59, 2962–2966. doi: 10.1099/ijs.0.007013-0
Blank, C. E., Cady, S. L., and Pace, N. R. (2002). Microbial composition of near-boiling silica-depositing thermal springs throughout Yellowstone National Park. Appl. Environ. Microbiol. 68, 5123–5135. doi: 10.1128/AEM.68.10.5123-5135.2002
Boomer, S. M., Noll, K. L., Geesey, G. G., and Dutton, B. E. (2009). Formation of multilayered photosynthetic biofilms in an alkaline thermal spring in Yellowstone National Park, Wyoming. Appl. Environ. Microbiol. 75, 2464–2475. doi: 10.1128/AEM.01802-08
Bozal, N., Montes, M. J., Tudela, E., and Guinea, J. (2003). Characterization of several Psychrobacter strains isolated from Antarctic environments and description of Psychrobacter luti sp. nov. and Psychrobacter fozii sp. nov. Int. J. Syst. Evol. Microbiol. 53, 1093–1100. doi: 10.1099/ijs.0.02457-0
Branscomb, E., Biancalani, T., Goldenfeld, N., and Russell, M. (2017). Escapement mechanisms and the conversion of disequilibria; the engines of creation. Phys. Rep. 677, 1–60. doi: 10.1016/j.physrep.2017.02.001
Brazelton, W. J., McGonigle, J. M., Motamedi, S., Pendleton, H. L., Twing, K. I., Miller, B. C., et al. (2022). Metabolic strategies shared by basement residents of the Lost City hydrothermal field. Appl. Environ. Microbiol. [Epub ahead of print]. doi: 10.1101/2022.01.25.477282
Callahan, B. J., McMurdie, P. J., Rosen, M. J., Han, A. W., Johnson, A. J. A., and Holmes, S. P. (2016). DADA2: high-resolution sample inference from Illumina amplicon data. Nat. Methods 13, 581–583. doi: 10.1038/nmeth.3869
Chaudhary, D. K., Kim, D.-U., Kim, D., and Kim, J. (2019). Flavobacterium petrolei sp. nov., a novel psychrophilic, diesel-degrading bacterium isolated from oil-contaminated Arctic soil. Sci. Rep. 9:4134. doi: 10.1038/s41598-019-40667-7
Chen, P., Zhou, H., Huang, Y., Xie, Z., Zhang, M., Wei, Y., et al. (2021). Revealing the full biosphere structure and versatile metabolic functions in the deepest ocean sediment of the Challenger Deep. Genome Biol. 22:207. doi: 10.1186/s13059-021-02408-w
Chivian, D., Brodie, E. L., Alm, E. J., Culley, D. E., Dehal, P. S., DeSantis, T. Z., et al. (2008). Environmental genomics reveals a single-species ecosystem deep within Earth. Science 322, 275–278. doi: 10.1126/science.1155495
Corman, J. R., Poret-Peterson, A. T., Uchitel, A., and Elser, J. J. (2016). Interaction between lithification and resource availability in the microbialites of Río Mesquites, Cuatro Ciénegas, México. Geobiology 14, 176–189. doi: 10.1111/gbi.12168
Couradeau, E., Roush, D., Guida, B. S., and Garcia-Pichel, F. (2017). Diversity and mineral substrate preference in endolithic microbial communities from marine intertidal outcrops (Isla de Mona, Puerto Rico). Biogeosciences 14, 311–324. doi: 10.5194/bg-14-311-2017
Eder, W., and Huber, R. (2002). New isolates and physiological properties of the Aquificales and description of Thermocrinis albus sp. nov. Extremophiles 6, 309–318. doi: 10.1007/s00792-001-0259-y
Espín, Y., Aranzulla, G., Álvarez-Ortí, M., and Gómez-Alday, J. J. (2020). Microbial community and atrazine-degrading genetic potential in deep zones of a hypersaline lake-aquifer system. Appl. Sci. 10:7111. doi: 10.3390/app10207111
Farquhar, J., Bao, H., and Thiemens, M. (2000). Atmospheric influence of Earth’s earliest sulfur cycle. Science 289, 756–758. doi: 10.1126/science.289.5480.756
García-Davis, S., Reyes, C. P., Lagunes, I., Padrón, J. M., Fraile-Nuez, E., Fernández, J. J., et al. (2021). Bioprospecting antiproliferative marine microbiota from submarine Volcano Tagoro. Front. Mar. Sci. 8:687701. doi: 10.3389/fmars.2021.687701
Geptner, A., Kristmannsdóttir, H., Kristjansson, J., and Marteinsson, V. (2002). Biogenic saponite from an active submarine hot spring, Iceland. Clays Clay Miner. 50, 174–185. doi: 10.1346/000986002760832775
Hand, K. P., Carlson, R. W., and Chyba, C. F. (2007). Energy, chemical disequilibrium, and geological constraints on Europa. Astrobiology 7, 1006–1022. doi: 10.1089/ast.2007.0156
Hudson, R., de Graaf, R., Strandoo Rodin, M., Ohno, A., Lane, N., McGlynn, S. E., et al. (2020). CO2 reduction driven by a pH gradient. Proc. Natl. Acad. Sci. U.S.A. 117, 22873–22879. doi: 10.1073/pnas.2002659117
Hügler, M., Huber, H., Molyneaux, S. J., Vetriani, C., and Sievert, S. M. (2007). Autotrophic CO2 fixation via the reductive tricarboxylic acid cycle in different lineages within the phylum Aquificae: evidence for two ways of citrate cleavage. Environ. Microbiol. 9, 81–92. doi: 10.1111/j.1462-2920.2006.01118.x
Johnson, J. E., Gerpheide, A., Lamb, M. P., and Fischer, W. W. (2014). O2 constraints from Paleoproterozoic detrital pyrite and uraninite. GSA Bull. 126, 813–830. doi: 10.1130/B30949.1
Karnachuk, O. V., Frank, Y. A., Lukina, A. P., Kadnikov, V. V., Beletsky, A. V., Mardanov, A. V., et al. (2019). Domestication of previously uncultivated Candidatus Desulforudis audaxviator from a deep aquifer in Siberia sheds light on its physiology and evolution. ISME J. 13, 1947–1959. doi: 10.1038/s41396-019-0402-3
Kelley, D. S., Karson, J. A., Früh-Green, G. L., Yoerger, D. R., Shank, T. M., Butterfield, D. A., et al. (2005). A serpentinite-hosted ecosystem: the Lost City hydrothermal field. Science 307, 1428–1434. doi: 10.1126/science.1102556
Kozich, J. J., Westcott, S. L., Baxter, N. T., Highlander, S. K., and Schloss, P. D. (2013). Development of a dual-index sequencing strategy and curation pipeline for analyzing amplicon sequence data on the MiSeq illumina sequencing platform. Appl. Environ. Microbiol. 79, 5112–5120. doi: 10.1128/AEM.01043-13
Lane, N., and Martin, W. F. (2012). The origin of membrane bioenergetics. Cell 151, 1406–1416. doi: 10.1016/j.cell.2012.11.050
Lang, S. Q., and Brazelton, W. J. (2020). Habitability of the marine serpentinite subsurface: a case study of the Lost City hydrothermal field. Philos. Trans. R. Soc. Math. Phys. Eng. Sci. 378:20180429. doi: 10.1098/rsta.2018.0429
Lanza, N. L., Wiens, R. C., Arvidson, R. E., Clark, B. C., Fischer, W. W., Gellert, R., et al. (2016). Oxidation of manganese in an ancient aquifer, Kimberley formation, Gale crater, Mars. Geophys. Res. Lett. 43, 7398–7407. doi: 10.1002/2016GL069109
Lecoeuvre, A., Ménez, B., Cannat, M., Chavagnac, V., and Gérard, E. (2021). Microbial ecology of the newly discovered serpentinite-hosted Old City hydrothermal field (southwest Indian ridge). ISME J. 15, 818–832. doi: 10.1038/s41396-020-00816-7
López, G., Diaz-Cárdenas, C., Shapiro, N., Woyke, T., Kyrpides, N. C., David Alzate, J., et al. (2017). Draft genome sequence of Pseudomonas extremaustralis strain USBA-GBX 515 isolated from Superparamo soil samples in Colombian Andes. Stand. Genomic Sci. 12:78. doi: 10.1186/s40793-017-0292-9
López, N. I., Pettinari, M. J., Stackebrandt, E., Tribelli, P. M., Põtter, M., Steinbüchel, A., et al. (2009). Pseudomonas extremaustralis sp. nov., a Poly(3-hydroxybutyrate) producer isolated from an antarctic environment. Curr. Microbiol. 59, 514–519. doi: 10.1007/s00284-009-9469-9
López-López, O., Knapik, K., Cerdán, M.-E., and González-Siso, M.-I. (2015). Metagenomics of an alkaline hot spring in galicia (Spain): microbial diversity analysis and screening for novel lipolytic enzymes. Front. Microbiol. 6:1291. doi: 10.3389/fmicb.2015.01291
Marteinsson, V. T., Kristjánsson, J. K., Kristmannsdóttir, H., Dahlkvist, M., Sæmundsson, K., Hannington, M., et al. (2001b). Discovery and description of giant submarine smectite cones on the Seafloor in Eyjafjordur, Northern Iceland, and a novel thermal microbial habitat. Appl. Environ. Microbiol. 67, 827–833. doi: 10.1128/AEM.67.2.827-833.2001
Marteinsson, V. T., Hauksdóttir, S., Hobel, C. F. V., Kristmannsdóttir, H., Hreggvidsson, G. O., and Kristjánsson, J. K. (2001a). Phylogenetic diversity analysis of subterranean hot springs in Iceland. Appl. Environ. Microbiol. 67, 4242–4248. doi: 10.1128/AEM.67.9.4242-4248.2001
Martin, M. (2011). Cutadapt removes adapter sequences from high-throughput sequencing reads. EMBnet J. 17, 10–12. doi: 10.14806/ej.17.1.200
Martin, W., and Russell, M. J. (2007). On the origin of biochemistry at an alkaline hydrothermal vent. Philos. Trans. R. Soc. B Biol. Sci. 362, 1887–1926. doi: 10.1098/rstb.2006.1881
Martinez, J. N., Nishihara, A., Lichtenberg, M., Trampe, E., Kawai, S., Tank, M., et al. (2019). Vertical distribution and diversity of phototrophic bacteria within a hot spring microbial mat (Nakabusa Hot Springs, Japan). Microb. Environ. 34, 374–387. doi: 10.1264/jsme2.ME19047
McCollom, T. M., and Seewald, J. S. (2007). Abiotic synthesis of organic compounds in deep-sea hydrothermal environments. Chem. Rev. 107, 382–401. doi: 10.1021/cr0503660
McMurdie, P. J., and Holmes, S. (2013). phyloseq: an R package for reproducible interactive analysis and graphics of microbiome census data. PLoS One 8:e61217. doi: 10.1371/journal.pone.0061217
Meyer-Dombard, D. R., and Amend, J. P. (2014). Geochemistry and microbial ecology in alkaline hot springs of Ambitle Island, Papua New Guinea. Extremophiles 18, 763–778. doi: 10.1007/s00792-014-0657-6
Meyer-Dombard, D. R., Swingley, W., Raymond, J., Havig, J., Shock, E. L., and Summons, R. E. (2011). Hydrothermal ecotones and streamer biofilm communities in the Lower Geyser Basin, Yellowstone National Park. Environ. Microbiol. 13, 2216–2231. doi: 10.1111/j.1462-2920.2011.02476.x
Monnin, C., Chavagnac, V., Boulart, C., Ménez, B., Gérard, M., Gérard, E., et al. (2014). The low temperature hyperalkaline hydrothermal system of the Prony bay (New Caledonia). Astrobiol. Exobiol. 11, 6221–6267. doi: 10.5194/bgd-11-6221-2014
Morotomi, M. (2014). “The Family Sutterellaceae,” in The Prokaryotes: Alphaproteobacteria and Betaproteobacteria, eds E. Rosenberg, E. F. DeLong, S. Lory, E. Stackebrandt, and F. Thompson (Berlin: Springer), 1005–1012.
Nakagawa, T., and Fukui, M. (2003). Molecular characterization of community structures and sulfur metabolism within microbial streamers in Japanese Hot Springs. Appl. Environ. Microbiol. 69, 7044–7057. doi: 10.1128/AEM.69.12.7044-7057.2003
Nakagawa, T., and Fukui, M. (2002). Phylogenetic characterization of microbial mats and streamers from a Japanese alkaline hot spring with a thermal gradient. J. Gen. Appl. Microbiol. 48, 211–222.
Nishihara, A., Thiel, V., Matsuura, K., McGlynn, S. E., and Haruta, S. (2018). Phylogenetic diversity of nitrogenase reductase genes and possible nitrogen-fixing bacteria in thermophilic chemosynthetic microbial communities in nakabusa hot springs. Microb. Environ. 33, 357–365. doi: 10.1264/jsme2.ME18030
Onstott, T. C., McGown, D. J., Bakermans, C., Ruskeeniemi, T., Ahonen, L., Telling, J., et al. (2009). Microbial communities in subpermafrost saline fracture water at the Lupin Au Mine, Nunavut, Canada. Microb. Ecol. 58, 786–807. doi: 10.1007/s00248-009-9553-5
Orlygsson, J., and Kristjansson, J. K. (2014). “The Family Hydrogenophilaceae,” in The Prokaryotes: Alphaproteobacteria and Betaproteobacteria, eds E. Rosenberg, E. F. DeLong, S. Lory, E. Stackebrandt, and F. Thompson (Berlin: Springer), 859–868.
Païssé, S., Goñi-Urriza, M., Coulon, F., and Duran, R. (2010). How a bacterial community originating from a contaminated coastal sediment responds to an oil input. Microb. Ecol. 60, 394–405. doi: 10.1007/s00248-010-9721-7
Postec, A., Quéméneur, M., Bes, M., Mei, N., Benaïssa, F., Payri, C., et al. (2015). Microbial diversity in a submarine carbonate edifice from the serpentinizing hydrothermal system of the Prony Bay (New Caledonia) over a 6-year period. Front. Microbiol. 6:857. doi: 10.3389/fmicb.2015.00857
Price, R., Boyd, E. S., Hoehler, T. M., Wehrmann, L. M., Bogason, E., Valtısson, H. þ, et al. (2017). Alkaline vents and steep Na+ gradients from ridge-flank basalts—Implications for the origin and evolution of life. Geology 45, 1135–1138. doi: 10.1130/G39474.1
Proskurowski, G., Lilley, M. D., Seewald, J. S., Früh-Green, G. L., Olson, E. J., Lupton, J. E., et al. (2008). Abiogenic hydrocarbon production at lost city hydrothermal field. Science 319, 604–607. doi: 10.1126/science.1151194
Pruesse, E., Peplies, J., and Glöckner, F. O. (2012). SINA: accurate high-throughput multiple sequence alignment of ribosomal RNA genes. Bioinformatics 28, 1823–1829. doi: 10.1093/bioinformatics/bts252
Purcell, D., Sompong, U., Yim, L. C., Barraclough, T. G., Peerapornpisal, Y., and Pointing, S. B. (2007). The effects of temperature, pH and sulphide on the community structure of hyperthermophilic streamers in hot springs of northern Thailand. FEMS Microbiol. Ecol. 60, 456–466. doi: 10.1111/j.1574-6941.2007.00302.x
Quéméneur, M., Bes, M., Postec, A., Mei, N., Hamelin, J., Monnin, C., et al. (2014). Spatial distribution of microbial communities in the shallow submarine alkaline hydrothermal field of the Prony Bay, New Caledonia. Environ. Microbiol. Rep. 6, 665–674. doi: 10.1111/1758-2229.12184
Reysenbach, A. L., Wickham, G. S., and Pace, N. R. (1994). Phylogenetic analysis of the hyperthermophilic pink filament community in Octopus Spring, Yellowstone National Park. Appl. Environ. Microbiol. 60, 2113–2119. doi: 10.1128/aem.60.6.2113-2119.1994
Rinke, C., Schmitz-Esser, S., Loy, A., Horn, M., Wagner, M., and Bright, M. (2009). High genetic similarity between two geographically distinct strains of the sulfur-oxidizing symbiont “Candidatus Thiobios zoothamnicoli.”. FEMS Microbiol. Ecol. 67, 229–241. doi: 10.1111/j.1574-6941.2008.00628.x
Rohland, N., and Reich, D. (2012). Cost-effective, high-throughput DNA sequencing libraries for multiplexed target capture. Genome Res. 22, 939–946. doi: 10.1101/gr.128124.111
Rucker, H. R., Ely, T. D., LaRowe, D. E., Giovannelli, D., and Price, R. E. (2022). Quantifying the bioavailable energy in an ancient hydrothermal vent on Mars and a modern Earth-based analogue. bioRxiv [Preprint]. bioRxiv: 2022.09.09.507253.
Russell, M. (2009). The alkaline solution to the emergence of life: energy, entropy and early evolution. Acta Biotheor. 57, 389–394. doi: 10.1007/s10441-007-9026-5
Russell, M. J., Hall, A. J., and Martin, W. (2010). Serpentinization as a source of energy at the origin of life. Geobiology 8, 355–371. doi: 10.1111/j.1472-4669.2010.00249.x
Sekiguchi, Y., Muramatsu, M., Imachi, H., Narihiro, T., Ohashi, A., Harada, H., et al. (2008). Thermodesulfovibrio aggregans sp. nov. and Thermodesulfovibrio thiophilus sp. nov., anaerobic, thermophilic, sulfate-reducing bacteria isolated from thermophilic methanogenic sludge, and emended description of the genus Thermodesulfovibrio. Int. J. Syst. Evol. Microbiol. 58, 2541–2548. doi: 10.1099/ijs.0.2008/000893-0
Sheik, C. S., Reese, B. K., Twing, K. I., Sylvan, J. B., Grim, S. L., Schrenk, M. O., et al. (2018). Identification and removal of contaminant sequences from ribosomal gene databases: Lessons from the census of deep life. Front. Microbiol. 9:840. doi: 10.3389/fmicb.2018.00840
Shiraishi, F., Matsumura, Y., Chihara, R., Okumura, T., Itai, T., Kashiwabara, T., et al. (2019). Depositional processes of microbially colonized manganese crusts, Sambe hot spring, Japan. Geochim. Cosmochim. Acta 258, 1–18. doi: 10.1016/j.gca.2019.05.023
Stamenković, V., Ward, L. M., Mischna, M., and Fischer, W. W. (2018). O2 solubility in Martian near-surface environments and implications for aerobic life. Nat. Geosci. 11, 905–909. doi: 10.1038/s41561-018-0243-0
Tobler, D. J., and Benning, L. G. (2011). Bacterial diversity in five Icelandic geothermal waters: temperature and sinter growth rate effects. Extremophiles 15:473. doi: 10.1007/s00792-011-0378-z
Umezawa, K., Kojima, H., Kato, Y., and Fukui, M. (2021). Dissulfurispira thermophila gen. nov., sp. nov., a thermophilic chemolithoautotroph growing by sulfur disproportionation, and proposal of novel taxa in the phylum Nitrospirota to reclassify the genus Thermodesulfovibrio. Syst. Appl. Microbiol. 44:126184. doi: 10.1016/j.syapm.2021.126184
Van Trappen, S., Vandecandelaere, I., Mergaert, J., and Swings, J. (2004). Flavobacterium degerlachei sp. nov., Flavobacterium frigoris sp. nov. and Flavobacterium micromati sp. nov., novel psychrophilic bacteria isolated from microbial mats in Antarctic lakes. Int. J. Syst. Evol. Microbiol. 54, 85–92. doi: 10.1099/ijs.0.02857-0
Van Trappen, S., Vandecandelaere, I., Mergaert, J., and Swings, J. (2005). Flavobacterium fryxellicola sp. nov. and Flavobacterium psychrolimnae sp. nov., novel psychrophilic bacteria isolated from microbial mats in Antarctic lakes. Int. J. Syst. Evol. Microbiol. 55, 769–772. doi: 10.1099/ijs.0.03056-0
Wang, X., and Pecoraro, L. (2021). Diversity and co-occurrence patterns of fungal and bacterial communities from alkaline sediments and water of julong high-altitude hot springs at Tianchi Volcano, Northeast China. Biology 10:894. doi: 10.3390/biology10090894
Ward, L. M., and Shih, P. M. (2019). The evolution and productivity of carbon fixation pathways in response to changes in oxygen concentration over geological time. Free Radic. Biol. Med. 140, 188–199. doi: 10.1016/j.freeradbiomed.2019.01.049
Ward, L. M., Stamenković, V., Hand, K., and Fischer, W. W. (2019). Follow the oxygen: comparative histories of planetary oxygenation and opportunities for aerobic life. Astrobiology 19, 811–824. doi: 10.1089/ast.2017.1779
Willems, A., Busse, J., Goor, M., Pot, B., Falsen, E., Jantzen, E., et al. (1989). Hydrogenophaga, a new genus of hydrogen-oxidizing bacteria that includes Hydrogenophaga flava comb. nov. (Formerly Pseudomonas flava), Hydrogenophaga palleronii (Formerly Pseudomonas palleronii), Hydrogenophaga pseudoflava (Formerly Pseudomonas pseudoflava and “Pseudomonas carboxy do flava”), and Hydrogenophaga taeniospiralis (Formerly Pseudomonas taeniospiralis). Int. J. Syst. Evol. Microbiol. 39, 319–333. doi: 10.1099/00207713-39-3-319
Wirth, R., Sikorski, J., Brambilla, E., Misra, M., Lapidus, A., Copeland, A., et al. (2010). Complete genome sequence of Thermocrinis albus type strain (HI 11/12T). Stand. Genom. Sci. 2, 194–202. doi: 10.4056/sigs.761490
Youssef, N. H., Farag, I. F., Rudy, S., Mulliner, A., Walker, K., Caldwell, F., et al. (2019). The Wood–Ljungdahl pathway as a key component of metabolic versatility in candidate phylum Bipolaricaulota (Acetothermia, OP1). Environ. Microbiol. Rep. 11, 538–547. doi: 10.1111/1758-2229.12753
Keywords: hydrothermal vent, alkaline, Iceland, microbial diversity, 16S rRNA
Citation: Twing KI, Ward LM, Kane ZK, Sanders A, Price RE, Pendleton HL, Giovannelli D, Brazelton WJ and McGlynn SE (2022) Microbial ecology of a shallow alkaline hydrothermal vent: Strýtan Hydrothermal Field, Eyjafördur, northern Iceland. Front. Microbiol. 13:960335. doi: 10.3389/fmicb.2022.960335
Received: 02 June 2022; Accepted: 05 August 2022;
Published: 17 November 2022.
Edited by:
S. Emil Ruff, Marine Biological Laboratory (MBL), United StatesReviewed by:
Emma Bell, Swiss Federal Institute of Technology Lausanne, SwitzerlandLauren M. Seyler, Stockton University, United States
Copyright © 2022 Twing, Ward, Kane, Sanders, Price, Pendleton, Giovannelli, Brazelton and McGlynn. This is an open-access article distributed under the terms of the Creative Commons Attribution License (CC BY). The use, distribution or reproduction in other forums is permitted, provided the original author(s) and the copyright owner(s) are credited and that the original publication in this journal is cited, in accordance with accepted academic practice. No use, distribution or reproduction is permitted which does not comply with these terms.
*Correspondence: Katrina I. Twing, a2F0cmluYXR3aW5nQHdlYmVyLmVkdQ==