- 1Key Laboratory of Dairy Science, Ministry of Education, Department of Food Science, Northeast Agricultural University, Harbin, China
- 2Hyproca Nutrition Co., Ltd., Changsha, China
- 3Hunan Provincial Key Laboratory of Food Science and Biotechnology, Changsha, China
Treatments targeted for gut microbial regulation are newly developed strategies in constipation management. In this study, the alleviating effects of gut micro-ecologically regulatory treatments on constipation in mice were investigated. Male BALB/c mice were treated with loperamide to induce constipation, and then the corresponding intervention was administered in each group, respectively. The results showed that administration of mixed probiotics (MP), a 5-fold dose of postbiotics (P5), both synbiotics (S and S2), as well as mixed probiotics and postbiotics (MPP) blend for 8 days shortened the time to the first black stool, raised fecal water content, promoted intestinal motility, and increased serum motilin level in loperamide-treated mice. Furthermore, these treatments altered gut microbial composition and metabolism of short-chain fatty acids (SCFA). Based on linear regression analysis, SCFA was positively correlated with serum motilin except for isobutyrate. It suggested gut microbial metabolites affected secretion of motilin to increase gastrointestinal movement and transportation function and thus improved pathological symptoms of mice with constipation. In conclusion, the alteration of gut micro-ecology is closely associated with gastrointestinal function, and it is an effective way to improve constipation via probiotic, prebiotic, and postbiotic treatment.
Introduction
Constipation, a worldwide digestive disorder, impacts around 14% of people in different regions (Black and Ford, 2018). Patients with constipation usually show symptoms like reduced frequency of defecation, hard or lumpy stools, a sensation of incomplete evacuation, and abdominal bloating and pain (Mearin et al., 2016). Chronic constipation not only hurts patients’ health but also increases the burden on the social health care system (Sharma and Rao, 2017). Conventionally, laxative drugs are widely used for the treatment of astriction, which can prompt defecation soon after administration, but the effects are less likely to exist when the drug use is ceased (Smith, 1973; Corazziari, 1999; Wang et al., 2020). Thus, laxative abuse probably leads to drug dependence and may deteriorate the symptom of constipation. Understanding the mechanisms of astriction has become the key aspect of developing new therapies.
Studies in recent years have shown the role of the gut microbiome in the pathogenesis of constipation. Zoppi et al. (1998) compared the composition of the fecal sample from children with constipation as well as their healthy counterparts and found that clostridia were more abundant in the constipated subjects (Zoppi et al., 1998). Clostridium difficile is the main cause to induce nosocomial diarrhea in children (Sadeghifard et al., 2010). Furthermore, in a children cohort with autism spectrum disorder (ASD), the proportion of Clostridium spp. including Clostridium paraputri, Clostridium bolteae, and Clostridium perfringens in the feces of was significantly higher than in neurotypical children, and especially Clostridium diffiicile and Clostridium clostridiioforme were not found in neurotypical children (Kandeel et al., 2020). This suggests that Clostridium spp. play an important role in affecting the development of diseases including neurological and intestinal disorders during childhood. Adult patients with functional constipation presented lower abundances of Bacteroides, Roseburia, and Coprococcus compared with healthy volunteers (Shin et al., 2019), and a pediatric study showed that the genus Prevotella decreased in constipated obese children compared with non-constipated obese children, while Blautia, Coprococcus, and Ruminococcus increased (Zhu et al., 2014). Animal experiments reported a reduced abundance of Bacteroides, Lactobacillus, Desulfovibrio, and Methylobacterium, and enriched Clostridium and Akkermansia (Cao et al., 2017; Wang et al., 2017a; Yi et al., 2019). Cao et al. transferred the feces of constipated patients to the intestine of germ-free mice and the recipient formed constipation-like symptoms, including reduced intestinal peristalsis and fecal water content (Cao et al., 2017). Although the character of the gut microbiome in constipated subjects differs across different researches, gut microbial dysbiosis probably contributes to the pathogenesis of constipation.
Intestinal health lays a great reliance on the gut microbiome (Sekirov et al., 2010). Gut microbiota affects the development of constipation via modulating the intestinal functions and communication with the central nervous system (Simon et al., 2021). Regulating gut microecology has been a newly developed strategy for constipation treatment. Probiotics, prebiotics, and synbiotics have been reported as dietary supplements to regulate immune responses, gut microbial composition, and metabolisms (Ştefãnuţ et al., 2015; Kamiński et al., 2020), and especially microbial metabolites contribute to the improvement of gastrointestinal functions and interaction between the gut and central system via cell signaling pathways (Mitrea et al., 2022). Probiotics supplement is one of the most effective measures to regulate gut microbiota. Many studies have concluded that probiotic consumption helps to change the gut microbial structure and alleviate constipation in humans (Dimidi et al., 2014; Martinez-Martinez et al., 2017; Huang et al., 2018) and animal models (Wang et al., 2017a,2020; Inatomi and Honma, 2021; Wang R. et al., 2021). Meanwhile, prebiotics, which can promote the growth of beneficial bacteria in the intestine, has been reported to improve constipation-associated disorders and partly change the gut microbial composition in adults (Yu et al., 2017; Chu et al., 2019). Present literature has demonstrated postbiotics consist of cell corpses, cell components, cell-wall fractions, cellular secretions, and metabolites, that are from probiotics and if received in sufficient quantity possess health effects on the host (Sabahi et al., 2022). In this research, the effects of mixed probiotics, postbiotics, synbiotics, and probiotics plus postbiotics on loperamide-induced constipation in BALB/c mice were compared, and their influence on the hormonal level, fecal short-chain fatty acids (SCFA), as well as gut microbial composition were sequenced to explore the potential targets of their constipation-alleviating function.
Materials and methods
Preparation of experimental formulation
The experimental formulation used in this study was a mixture of probiotics, prebiotics, postbiotics, and other nutritional components. Probiotics, prebiotics, and postbiotics were all purchased from Aunulife Biotechnology Co., Ltd. (Changsha, China). All these powdered products were resuspended or dissolved in saline before use. MP consisted of 6 strains (Bifidobacterium animalis subsp. animalis BB-115, B. longum subsp. infantis BLI-02, Lacticaseibacillus rhamnosus MP108, Ligilactobacillus salivarius AP-32, L. rhamnosus F-1, and Lactiplantibacillus plantarum LPL28) with a total colony-forming unit of 2.0 × 1010. The prebiotics contained lactitol, fructooligosaccharides, inulin, and stachyose, and postbiotics consisted of isolated soy protein and 4 strains including L. plantarum LPL28, L. salivarius AP-32, B. longum subsp. infantis BLI-02, and Lactobacillus acidophilus TYCA06. After fermentation, the supernatant of 4 strains was extracted via centrifuging, and then it was mixed with isolated soy protein after heat-kill sterilization. The mixture was made with postbiotics after heat-kill sterilization and spay-drying. Other components used as nutritional supplements were lactose, vitamin C, psyllium seed husk, and some fruit and vegetable powder.
Animals and experimental design
Eight-week-old male BALB/c mice (n = 64) were purchased from Vital River Laboratory Animal Technology Co., Ltd. (Beijing, China). Mice were fed in a specific pathogen-free environment at a room temperature of 25°C ± 2°C and humidity of 50% ± 5% with a 12-h light–dark cycle, having free access to drinking water and standard chow. After 1 week of adaptation, mice were randomly divided into 8 groups (n = 8/group), containing the control group (control), model group (model), six constipated groups treated with mixed probiotics (MP), postbiotics (P and a 5-fold dose of P, P5), synbiotics (S and a 2-fold dose of S, S2), and probiotics plus postbiotics (MPP), and details of the experimental design were listed in Table 1. The Control group was orally treated only with saline per day during the experimental period by gavage, while the other groups were treated every day with loperamide (10 mg/kg body weight), and with corresponding products 1 h after loperamide administration. The treatments were carried out by gavage and lasted for 8 days. After the intervention period, animals were anesthetized with pentobarbital (50 mg/kg body weight) (Wang et al., 2010) and were then sacrificed by exsanguination. All protocols for the animal experiment were approved by the Ethics Committee of Jiangnan University [JN. No20201130b0720301(355)] and were in accord with the European Community guidelines (directive 2010/63/EU).
Defecation test
Defecation status was represented by first defecation time and fecal water content. Mice were treated with activated carbon meal 1 h after treatments on the eighth day, and then the time to the first black stool of each mouse was measured according to Wang et al. (Cao et al., 2017). Feces were then collected in individual sterile EP tubes on ice and the water content was determined as described by Lee et al. (2012).
Determination of intestinal propulsion rate
Gastrointestinal (GI) motility was determined as previously described with a modification (Verdu et al., 2009). Mice were fasted from 6:00 p.m. for 18 h on the eighth day and were then treated with loperamide (treated groups) or saline (control group), and activated carbo meal, after which mice were anesthetized with pentobarbital (50 mg/kg body weight) and executed by exsanguination (Wang et al., 2010). The blood sample was collected immediately centrifuged at 3000 × g, 4°C for 15 min to obtain serum. The entire intestine was carefully removed out of the abdomen and put on blotting paper. The distance from the pylorus to the end of the darkened intestine as well as the entire length of the intestine was measured. The intestinal propulsion rate was calculated by the ratio of the length of the black intestine to the entire intestinal length.
Serum cytokine detection
Serum samples were divided into 2 to 3 individual sterile EP tubes and then stored at −80°C. Cytokines containing IFN-α, TGF-β1, TNF-α, IL-1β, IL-6, IL-10, and IL-17A were determined using a MILLIPLEX MAP Kit Mouse Cytokine/Chemokine Magnetic Bead Panel (EMD Millipore Corporation, Billerica, MA, United States) according to the manufacturer’s instruction and performed with a Luminex MAGPIX System (Luminex, Austin, TX, United States).
Quantification of fecal short-chain fatty acids
Fecal samples (∼50 mg) were soaked with saturated NaCl solution and acidified with sulfuric acid (10%). Total SCFAs extracted with diethyl ether. Determination of the concentration of SCFA was carried out through a gas chromatography-mass spectrometry system (GCMS-QP2010 Ultra system, Shimadzu Corporation, Japan) according to Wang et al. (2017a). The concentration of total SCFA was calculated as the sum of acetic, propionic, butyric, isobutyric, valeric, and isovaleric acids.
Analysis of fecal microbiome
Total DNA was extracted from fecal samples with a FastDNA Spin Kit for Soil (MP Biomedicals, catalog no. 6560-200) following the manufacturer’s instructions. The V4 region of the 16S rRNA gene was amplified using a specific primer (forward primer, 5′-AYT GGG YDT AAA GNG-3′; reverse primer, 5′-TAC NVG GGT ATC TAA TCC-3′) by polymerase chain reaction (PCR) as previously described. Then the PCR products were purified, quantified, and sequenced as previously described by Zhao et al. (2018). Afterward, the 16S rRNA sequence data were analyzed using the QIIME2 pipeline as previously described (Zhao et al., 2018).
Statistical analysis
Results were all expressed as mean ± SD. One-way ANOVA and Dunnett’s multiple comparison test were applied for the comparison between groups. Comparisons and linear regression analysis were carried out using GraphPad Prism version 8.0.2 (GraphPad Software Inc., United States). Values with p < 0.05 were considered significantly different. Pearson’s correlation analysis was conducted in MetaboAnalyst 5.0.1
Results
The synbiotics improved defecation status and gastrointestinal transit
As shown in Figure 1A, compared with the control group, a 2.3-fold increase in the time to the first black stool was observed in the model group (p < 0.0001), suggesting the formation of constipation due to loperamide treatment. The figure for S and S2 treatments decreased to approximately 50% of that of the model group. MP, P5, and MPP treatments partly shortened the defecation time, while P treatment showed little effect on it. Fecal water content in the model group decreased in comparison with the control group, which was nearly restored in S and S2 groups (Figure 1B). MP, P5, and MPP partly raised fecal water content, while the effect of P treatment was less significant than other treatments (Figure 1B). As shown in Figure 1C, the intestinal propulsion rate in the model group was significantly lower than that in the control group. Administration of S2 restored the decrease in intestinal propulsion rate, while the treatment with S partly inverted the decrease. MP, P5, and MPP slightly promoted the intestinal propulsion rate. In contrast, P treatment did not affect the intestinal propulsion rate in constipated mice.
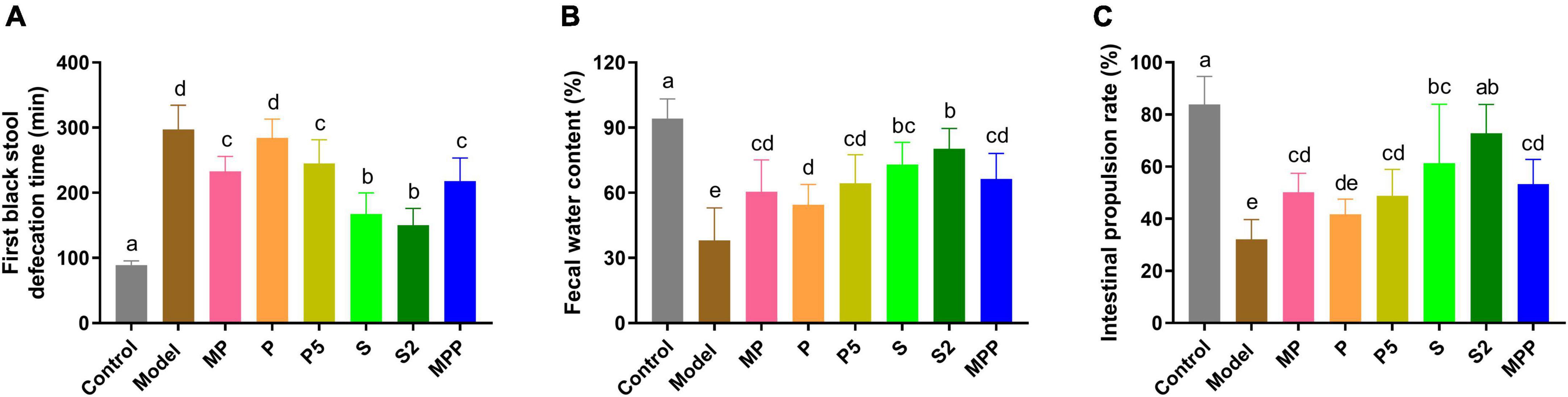
Figure 1. The differences in (A) time to the first black stool, (B) fecal water content, and (C) intestinal propulsion rate among groups. Values are expressed as mean ± SEM (n = 8). Results were compared by one-way ANOVA followed Duncan’s multiple comparison test. Different letters represent significant differences (p < 0.05).
The synbiotics changed serum hormones relevant to gastrointestinal motility
Motilin, a peptide hormone released from enterochromaffin cells, promotes GI contraction and digestive motility (Kamerling et al., 2003). A lack of motilin is associated with both normal transit constipation and slow transit constipation (Wang Y. Y. et al., 2021). As shown in Figure 2, compared with the control group, a significant decrease in serum motilin level was recorded in the model group (p < 0.0001). All the interventions except for P restored the serum motilin level in constipated mice. Furthermore, all the interventions did not change the level of proinflammatory cytokines in serum, including IFN-α, TGF-β1, TNF-α, IL-1β, IL-6, IL-10, and IL-17A (Table 2).
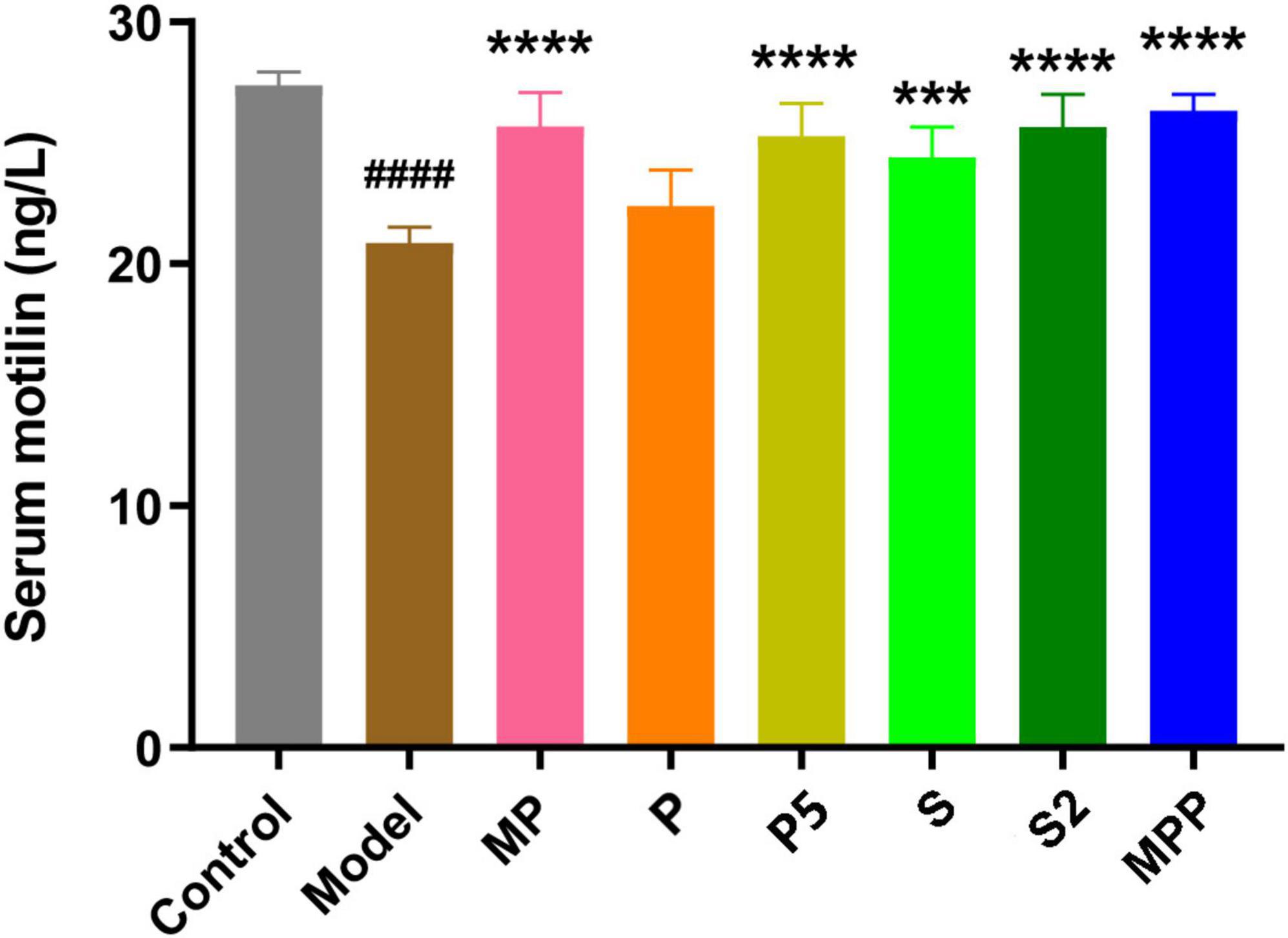
Figure 2. The differences in serum motilin level among groups. Values are expressed as mean ± SEM (n = 8). Results were compared by one-way ANOVA and Dunnett’s multiple comparison test: ***p < 0.001, ****p < 0.0001 compared with the model group; ####p < 0.0001 compared with the control group.
The synbiotics changed gut microbial composition in mice
16S rRNA sequencing results showed that Firmicutes and Bacteroidetes were the two dominating phyla in fecal samples. As shown in Figure 3, after loperamide treatment, the relative abundance of Actinobacteria decreased from 2.26 to 0.91%, and the figure for Patescibacteria dropped from 6.77 to 1.59%, while Bacteroidetes increased from 32.38 to 40.50%. MP administration upregulated Actinobacteria, Patescibacteria, and Firmicutes in constipated mice, and down-regulated Bacteroidetes. The proportion of Verrucomicrobia and Bacteroidetes increased in the P group compared with the model group, while Firmicutes decreased. A significant increase in Actinobacteria was observed in S and MPP groups.
No significant change was observed between the control and model groups from the alpha-diversity index, including Chao1, Simpson, Shannon, as well as observed OTUs (Figures 4A–D). Treatments other than the synbiotics increased the Chao1 index and observed OTUs in mice (Figures 4A,B). Mice in the treated groups except for MP and S2 groups exhibited an obvious increase in Shannon and Simpson indexes (Figures 4C,D).
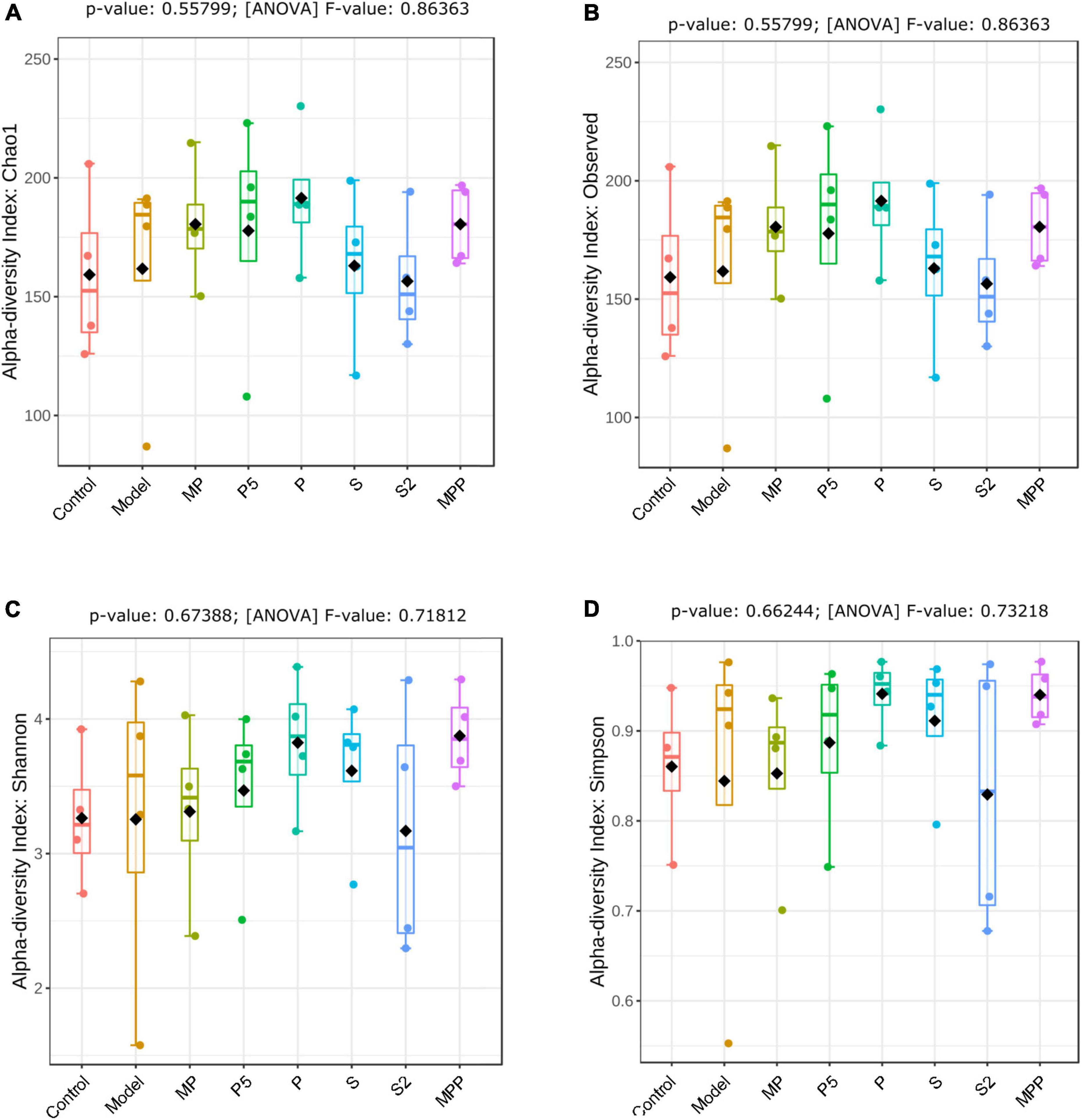
Figure 4. The differences in (A) Chao1 index, (B) observed OTUs, (C) Shannon index, and (D) Simpson index of fecal microbiome among groups. Results were compared by one-way ANOVA and Dunnett’s multiple comparison test.
At the genus level, no apparent difference was found between the model and control groups except the increased Akkermansia in the model group (Figure 5). Ruminiclostridium 6, Ruminiclostridium 9, and Eubacterium xylanophilum group were more abundant in MP and MPP groups, in comparison with the model group, while the abundance of Alistipes was much lower (Figure 5). There was a rise in Ruminiclostridium 6, Ruminiclostridium 9, Ruminococcus 1, Ruminiclostridium, and Bacteroides, as well as a decrease in Alistipes in the P, P5, S, and S2 groups compared with the model group (Figure 5).
The synbiotics changed fecal short-chain fatty acids concentration in mice
The concentration of fecal SCFA is an important implication of intestinal motility. As shown in Table 3, loperamide administration decreased the level of fecal acetate in mice, and this decrease was reversed in the MP, P5, S, S2, and MPP groups. While the decline in fecal propionate was restored in all these groups. Butyrate in stool was less in the model group than in the control group, which was reversed in the MP, P5, S, and S2 groups. A significant decrease in fecal valerate could be found in the model group, and this reduction was reversed by MP, S2, and MPP treatments. No obvious difference in fecal isobutyrate and isovalerate was observed between the control and model groups. Meanwhile, the level of isovalerate in stool increased in the MP, S, and S2 groups.
To explore the relationship between fecal SCFA and gut microbial genera, Pearson’s correlation analysis was carried out. As shown in Figure 6, fecal acetate was positively correlated with Ruminiclostridium 9 and negatively associated with Lactobacillus. Fecal propionate level was positively related to six genera, including Ruminococcus 1 and Blautia, while negatively correlated with Streptococcus. Butyrate was just positively associated with Eubacterium nodatum group and Erysipelatoclostridium, while isobutyrate was positively correlated with five genera like Prevotellaceae UCG 001, Ruminococcaceae UCG 013, and Ruminococcaceae UCG 014. In contrast, valerate was positively related to Lactobacillus and Enterorhabdus, while negatively associated with Muribaculum. A positive correlation was observed between isovalerate and two genera: Lactobacillus and Enterorhabdus, while a negative one was recorded between isovalerate and five genera, including Muribaculum, Intestinimonas, Lachnospiraceae NK4A136 group, Alistipes, and GCA 900066225, respectively.
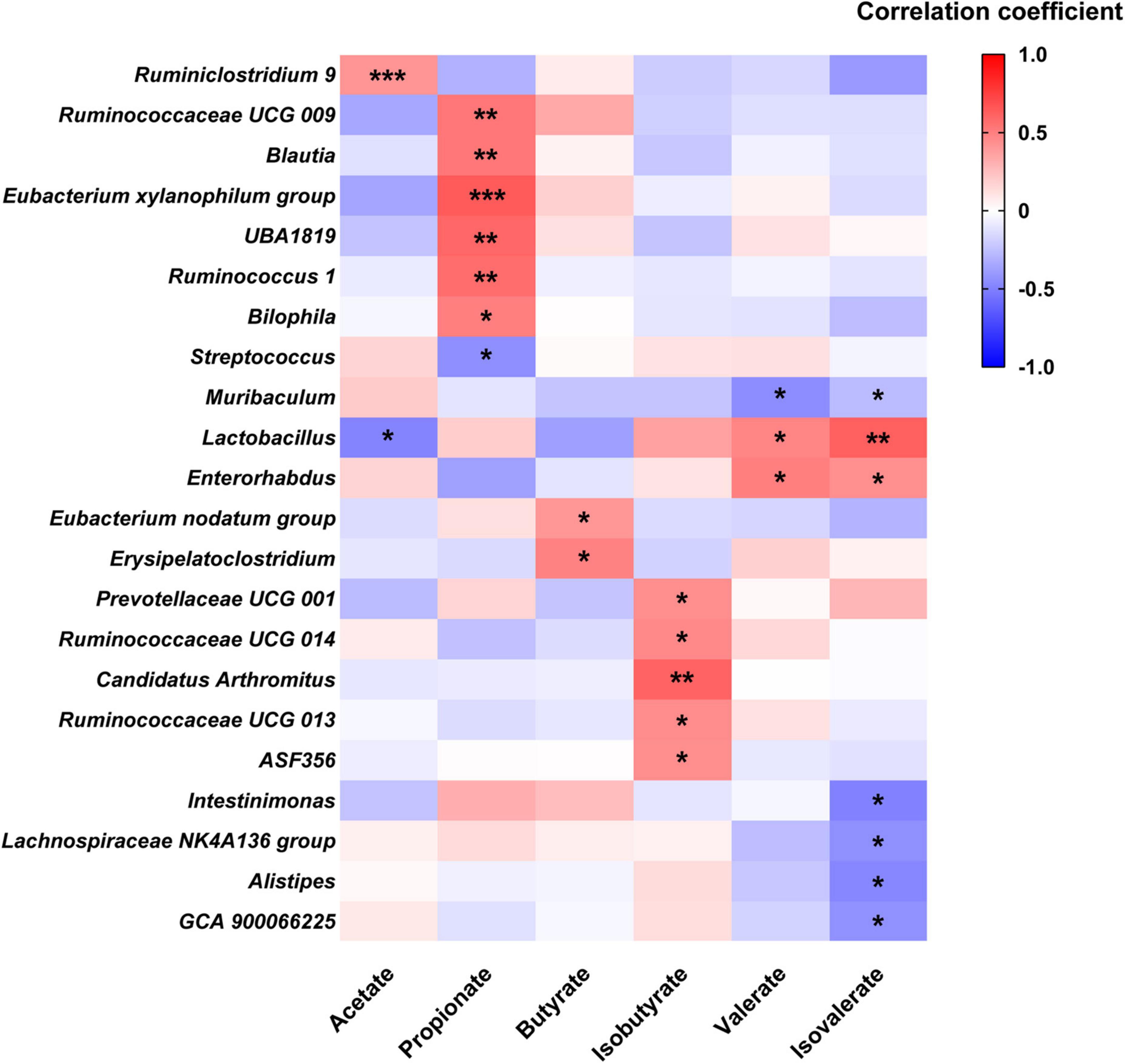
Figure 6. Heatmap of Pearson’s correlation analysis between SCFA and fecal microbial genera. *p < 0.05, ** p < 0.01, *** p < 0.001 statistical significance of Pearson’s correlation analysis.
Linear regression analysis was applied to analyze the correlation between serum motilin and fecal SCFA. Among these SCFA, acetate, propionate, butyrate, valerate, and isovalerate exhibited positive correlations with serum motilin levels, while isobutyrate was not statistically correlated with that (Figure 7).
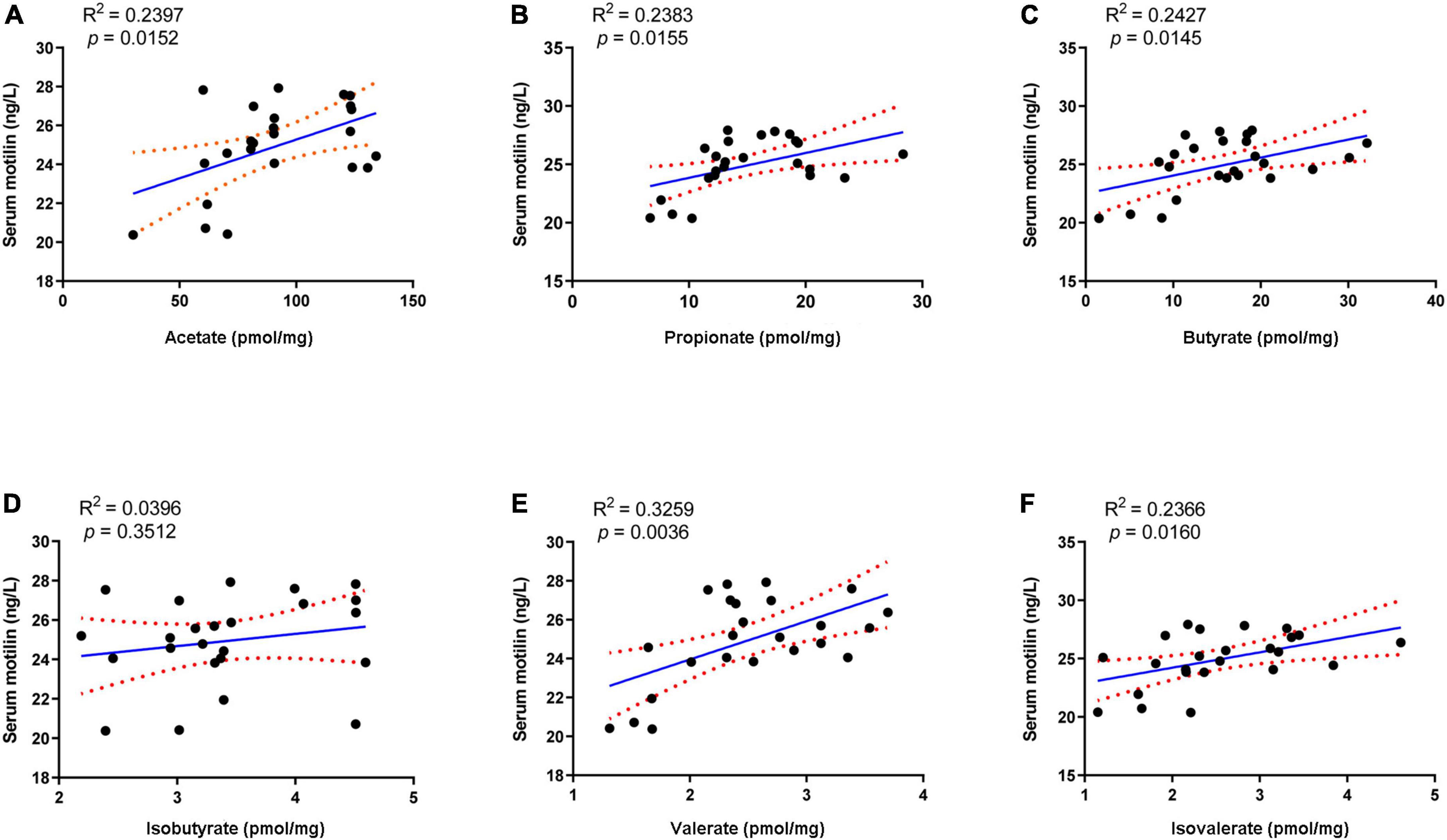
Figure 7. Linear regression analysis between serum motilin level and (A) acetate, (B) propionate, (C) butyrate, (D) isobutyrate, (E) valerate, and (F) isovalerate.
Discussion
Constipation is a functional GI disorder that is conventionally recognized by a decrease in defecation frequency, fecal water content, as well as GI motility. In recent years, the strategies based on gut microecological regulation, such as the supplementation of probiotics, prebiotics, and synbiotics, have drawn more attention. In this study, the effects of probiotics, postbiotics, and synbiotics were investigated in a mice model of spastic constipation, which was induced by loperamide administration (Salminen et al., 1998).
Loperamide, an agonist of μ-opioid receptors, prevents the release of acetylcholine and prostaglandin, thus inhibiting intestinal peristalsis and leading to prolonged retention of intestinal contents (Wang et al., 2017b). Our results showed that loperamide treatment caused typical symptoms of constipation, including an apparent increase in the time to the first black stool defecation and an obvious decrease in fecal water content. Administration of MP, P5, S, S2, and MPP treatments shortened the time to the first black stool and increased fecal water content in loperamide-treated mice, suggesting alleviations of these interventions. Constipation in mice was further confirmed by the decrease in intestinal propulsion rate. These treatments promoted intestinal propulsion rate in constipated mice, indicating an improvement in GI motility. In contrast, P treatment slightly improved fecal water content and GI motility but failed to shorten the time to the first black stool. Therefore, P treatment was less potent in relieving constipation than P5, and this might be due to the differences in dose.
Motilin is secreted by endocrine cells in the duodenum, jejunum, and antrum (Polak et al., 1975), and plays an important role in maintaining GI motility by stimulating its receptors (Kamerling et al., 2003). In this study, a significant decrease in serum motilin was recorded in constipated mice in contrast to healthy mice. This helped to explain the reduction in GI motility in this mice model. All interventions except for P restored serum motilin levels in constipated mice. Thus, MP, P5, S, S2, and MPP treatments might contribute to the stability of intestinal motility by sustaining motilin levels.
Alterations in the fecal microbiome were detected in constipated control mice, including the decreased Actinobacteria and Patescibacteria as well as the raised Bacteroidetes at the phylum level, suggesting gut microbial alterations due to loperamide treatment. Treatment with MP upregulated Firmicutes and downregulated Bacteroidetes in constipated mice. It was previously reported that increased Bacteroidetes and decreased Firmicutes were associated with constipation in rodents (Wang et al., 2017b). Thus, the probiotics applied in this study might help to reduce the risk of constipation by regulating the ratio of Firmicutes to Bacteroidetes. As for diversity, treatments increased different alpha-diversity indicators, suggesting that these interventions contribute to stabilizing the gut microbiome in the host.
Apart from regulating gut microbiota, probiotics, prebiotics, and synbiotics can influence the fermentation products in the gut, such as SCFA (Wang et al., 2017b; Lan et al., 2020). SCFA is mainly formed by the bacterial fermentation of indigestible carbohydrates in the large intestine and can stimulate intestine contraction, thereby promoting GI motility (Niwa et al., 2002). Previous studies revealed an association between improved constipation and fecal SCFA concentration, especially those using microbial regulating strategies like supplementation of probiotics (Wang et al., 2017b), prebiotics (Lan et al., 2020), and dietary fiber (Niwa et al., 2002; Zhuang et al., 2019). Our results showed that fecal acetate, propionate, butyrate, and valerate decreased after loperamide treatment, and these decreases were inverted by the administration of MP (Table 3). MPP treatment restored the changes in acetate, propionate, and valerate during constipation. Both S and S2 treatments restored the reduction in acetate, propionate, and butyrate, and increased isovalerate in the feces, while S2 upregulated fecal valerate in constipated mice. In contrast, P5 treatment raised acetate, propionate, and butyrate in stool, while P treatment failed to change fecal SCFA profile in constipated mice. Meanwhile, the correlation analysis showed that the SCFA other than isobutyrate was positively correlated with serum motilin, indicating that increased intestinal SCFA may contribute to upregulated motilin synthesis. Therefore, the alterations in SCFA in the gut after the interventions except for P might contribute to promoting GI motility and alleviating constipation.
Alterations in gut microbial genera probably lead to changes in fecal SCFA profile. In this study, the relative abundances of Ruminiclostridium 9 and Eubacterium xylanophilum group were increased after MPP administration, respectively, while Alistipes was reduced. Pearson’s correlation analysis suggested that fecal acetate concentration was positively associated with Ruminiclostridium 9, and propionate was positively associated with the Eubacterium xylanophilum group, while Alistipes negatively related to isovalerate. Ruminiclostridium 9 and the Eubacterium xylanophilum group were previously reported to be SCFA producers in the gut (Guo et al., 2018). Hence, the rise in these two genera in the gut might be favorable for improving SCFA production, thus promoting intestinal movement after MPP intervention. Treatments with P, P5, S, and S2 upregulated genera Ruminiclostridium 9, Ruminiclostridium, Ruminococcus 1, and Bacteroides in feces. Ruminococcus 1, which was identified as an SCFA producer (Louis and Flint, 2017), positively correlated with propionate in our results. According to Jacobson et al., upregulation of Bacteroides may help to raise propionate production, although fecal propionate did not show a significant correlation in this study (Jacobson et al., 2018). Accordingly, increases in Ruminiclostridium 9, Ruminiclostridium, Ruminococcus 1, and Bacteroides induced by P, P5, S, and S2 administrations might contribute to stimulating GI motility and ameliorating constipation.
Conclusion
The present study showed that the MP, P5, S, S2, and MPP treatments shortened the time to the first black stool, increased fecal water content and GI motility, and raised serum motilin levels in mice. Meanwhile, these interventions changed the gut microbial structure and increased fecal SCFA concentration, which contributed to their alleviating effects on constipation.
Data availability statement
The original contributions presented in this study are publicly available. This data can be found here: https://www.ncbi.nlm.nih.gov/bioproject/PRJNA842449; accession number: PRJNA842449.
Ethics statement
The animal study was reviewed and approved by the Ethics Committee of Jiangnan University [JN. No. 20201130b0720301 (355)].
Author contributions
YQZ conceived the project. YMZ completed the experiments and wrote the manuscript. QL and YH edited the manuscript. All authors contributed to the article and approved the submitted version.
Funding
This work was funded by Hyproca Nutrition Co., Ltd.
Acknowledgments
We would like to acknowledge Hyproca Nutrition Co., Ltd (Hunan, China) for funding this study.
Conflict of interest
All authors were employed by Hyproca Nutrition Co. Ltd. The authors declare that this study received funding from Hyproca Nutrition Co. Ltd. The funder was involved with study design.
Publisher’s note
All claims expressed in this article are solely those of the authors and do not necessarily represent those of their affiliated organizations, or those of the publisher, the editors and the reviewers. Any product that may be evaluated in this article, or claim that may be made by its manufacturer, is not guaranteed or endorsed by the publisher.
Footnotes
References
Black, C. J., and Ford, A. C. (2018). Chronic idiopathic constipation in adults: Epidemiology, pathophysiology, diagnosis and clinical management. Med. J. Aust. 209, 86–91. doi: 10.5694/mja18.00241
Cao, H., Liu, X., An, Y., Zhou, G., Liu, Y., Xu, M., et al. (2017). Dysbiosis contributes to chronic constipation development via regulation of serotonin transporter in the intestine. Sci. Rep. 7:10322. doi: 10.1038/s41598-017-10835-8
Chu, J. R., Kang, S. Y., Kim, S. E., Lee, S. J., Lee, Y. C., and Sung, M. K. (2019). Prebiotic UG1601 mitigates constipation-related events in association with gut microbiota: A randomized placebo-controlled intervention study. World J. Gastroenterol. 25, 6129–6144. doi: 10.3748/wjg.v25.i40.6129
Corazziari, E. (1999). Need of the ideal drug for the treatment of chronic constipation. Ital. J. Gastroenterol. Hepatol. 31, S232–S233.
Dimidi, E., Christodoulides, S., Fragkos, K. C., Scott, S. M., and Whelan, K. (2014). The effect of probiotics on functional constipation in adults: A systematic review and meta-analysis of randomized controlled trials. Am. J. Clin. Nutr. 100, 1075–1084. doi: 10.3945/ajcn.114.089151
Guo, J. R., Dong, X. F., Liu, S., and Tong, J. M. (2018). High-throughput sequencing reveals the effect of Bacillus subtilis CGMCC 1.921 on the cecal microbiota and gene expression in ileum mucosa of laying hens. Poult. Sci. 97, 2543–2556. doi: 10.3382/ps/pey112
Huang, L., Zhu, Q., Qu, X., and Qin, H. (2018). Microbial treatment in chronic constipation. Sci. China Life Sci. 61, 744–752. doi: 10.1007/s11427-017-9220-7
Inatomi, T., and Honma, M. (2021). Effects of probiotics on loperamide-induced constipation in rats. Sci. Rep. 11:24098. doi: 10.1038/s41598-021-02931-7
Jacobson, A., Lam, L., Rajendram, M., Tamburini, F., Honeycutt, J., Pham, T., et al. (2018). A gut commensal-produced metabolite mediates colonization resistance to salmonella infection. Cell Host Microbe 24, 296–307.e7. doi: 10.1016/j.chom.2018.07.002
Kamerling, I. M., Van Haarst, A. D., Burggraaf, J., Schoemaker, R. C., Biemond, I., Heinzerling, H., et al. (2003). Motilin effects on the proximal stomach in patients with functional dyspepsia and healthy volunteers. Am. J. Physiol. Gastrointest. Liver Physiol. 284, G776–G781. doi: 10.1152/ajpgi.00456.2002
Kamiński, M., Skonieczna-Żydecka, K., Łoniewski, I., Koulaouzidis, A., and Marlicz, W. (2020). Are probiotics useful in the treatment of chronic idiopathic constipation in adults? A review of existing systematic reviews, meta-analyses, and recommendations. Prz. Gastroenterol. 15, 103–118. doi: 10.5114/pg.2019.86747
Kandeel, W. A., Meguid, N. A., Bjørklund, G., Eid, E. M., Farid, M., Mohamed, S. K., et al. (2020). Impact of Clostridium Bacteria in Children with Autism Spectrum Disorder and Their Anthropometric Measurements. J. Mol. Neurosci. 70, 897–907. doi: 10.1007/s12031-020-01482-2
Lan, J., Wang, K., Chen, G., Cao, G., and Yang, C. (2020). Effects of inulin and isomalto-oligosaccharide on diphenoxylate-induced constipation, gastrointestinal motility-related hormones, short-chain fatty acids, and the intestinal flora in rats. Food Funct. 11, 9216–9225. doi: 10.1039/d0fo00865f
Lee, H. Y., Kim, J. H., Jeung, H. W., Lee, C. U., Kim, D. S., Li, B., et al. (2012). Effects of Ficus carica paste on loperamide-induced constipation in rats. Food Chem. Toxicol. 50, 895–902. doi: 10.1016/j.fct.2011.12.001
Louis, P., and Flint, H. J. (2017). Formation of propionate and butyrate by the human colonic microbiota. Environ. Microbiol. 19, 29–41. doi: 10.1111/1462-2920.13589
Martinez-Martinez, M. I., Calabuig-Tolsa, R., and Cauli, O. (2017). The effect of probiotics as a treatment for constipation in elderly people: A systematic review. Arch. Gerontol. Geriatr. 71, 142–149. doi: 10.1016/j.archger.2017.04.004
Mearin, F., Lacy, B. E., Chang, L., Chey, W. D., Lembo, A. J., Simren, M., et al. (2016). Bowel disorders. Gastroenterology [Epub ahead of print]. doi: 10.1053/j.gastro.2016.02.031
Mitrea, L., Nemeş, S. A., Szabo, K., Teleky, B. E., and Vodnar, D. C. (2022). Guts imbalance imbalances the brain: A review of gut microbiota association with neurological and psychiatric disorders. Front. Med. 9:813204. doi: 10.3389/fmed.2022.813204
Niwa, T., Nakao, M., Hoshi, S., Yamada, K., Inagaki, K., Nishida, M., et al. (2002). Effect of dietary fiber on morphine-induced constipation in rats. Biosci. Biotechnol. Biochem. 66, 1233–1240. doi: 10.1271/bbb.66.1233
Polak, J. M., Pearse, A. G., and Heath, C. M. (1975). Complete identification of endocrine cells in the gastrointestinal tract using semithin-thin sections to identify motilin cells in human and animal intestine. Gut 16, 225–229. doi: 10.1136/gut.16.3.225
Sabahi, S., Homayouni Rad, A., Aghebati-Maleki, L., Sangtarash, N., Ozma, M. A., Karimi, A., et al. (2022). Postbiotics as the new frontier in food and pharmaceutical research. Crit. Rev. Food Sci. Nutr. 29, 1–28. doi: 10.1080/10408398.2022.2056727
Sadeghifard, N., Salari, M. H., Ghassemi, M. R., Eshraghi, S., and Amin Harati, F. (2010). The incidence of nosocomial toxigenic clostridium difficile associated diarrhea in Tehran tertiary medical centers. Acta Med. Iran. 48, 320–325.
Salminen, S., Bouley, C., Boutron-Ruault, M. C., Cummings, J. H., Franck, A., Gibson, G. R., et al. (1998). Functional food science and gastrointestinal physiology and function. Br. J. Nutr. 80, S147–S171. doi: 10.1079/bjn19980108
Sekirov, I., Russell, S. L., Antunes, L. C., and Finlay, B. B. (2010). Gut microbiota in health and disease. Physiol. Rev. 90, 859–904. doi: 10.1152/physrev.00045.2009
Sharma, A., and Rao, S. (2017). Constipation: Pathophysiology and current therapeutic approaches. Handb. Exp. Pharmacol. 239, 59–74. doi: 10.1007/164_2016_111
Shin, A., Preidis, G. A., Shulman, R., and Kashyap, P. C. (2019). The gut microbiome in adult and pediatric functional gastrointestinal disorders. Clin. Gastroenterol. Hepatol. 17, 256–274. doi: 10.1016/j.cgh.2018.08.054
Simon, E., Cãlinoiu, L. F., Mitrea, L., and Vodnar, D. C. (2021). Probiotics, prebiotics, and synbiotics: Implications and beneficial effects against irritable bowel syndrome. Nutrients 13:2112. doi: 10.3390/nu13062112
Smith, B. (1973). Pathologic changes in the colon produced by anthraquinone purgatives. Dis. Colon Rectum 16, 455–458. doi: 10.1007/BF02588868
Ştefãnuţ, C., Mitrea, L., and Ognean, L. (2015). Effects of probiotics, prebiotics and synbiotics on some hematological and microbiological parameters in laboratory mice. Bull. Univ. Agric. Sci. Vet. Med. Cluj. Napoca. 72, 301–305. doi: 10.15835/buasvmcn-vm:11435
Verdu, E. F., Bercik, P., and Collins, S. M. (2009). Effect of probiotics on gastrointestinal function: Evidence from animal models. Therap. Adv. Gastroenterol. 2, 31–35. doi: 10.1177/1756283X09337645
Wang, G., Yang, S., Sun, S., Si, Q., Wang, L., Zhang, Q., et al. (2020). Lactobacillus rhamnosus strains relieve loperamide-induced constipation via different pathways independent of short-chain fatty acids. Front. Cell. Infect. Microbiol. 10:423. doi: 10.3389/fcimb.2020.00423
Wang, L. E., Cui, X. Y., Cui, S. Y., Cao, J. X., Zhang, J., Zhang, Y. H., et al. (2010). Potentiating effect of spinosin, a C-glycoside flavonoid of Semen Ziziphi spinosae, on pentobarbital-induced sleep may be related to postsynaptic 5-HT(1A) receptors. Phytomedicine 17, 404–409. doi: 10.1016/j.phymed.2010.01.014
Wang, L., Hu, L., Xu, Q., Jiang, T., Fang, S., Wang, G., et al. (2017a). Bifidobacteria exert species-specific effects on constipation in BALB/c mice. Food Funct. 8, 3587–3600. doi: 10.1039/c6fo01641c
Wang, L., Hu, L., Xu, Q., Yin, B., Fang, D., Wang, G., et al. (2017b). Bifidobacterium adolescentis exerts strain-specific effects on constipation induced by loperamide in balb/c mice. Int. J. Mol. Sci. 18:318. doi: 10.3390/ijms18020318
Wang, R., Sun, J., Li, G., Zhang, M., Niu, T., Kang, X., et al. (2021). Effect of Bifidobacterium animalis subsp. lactis MN-Gup on constipation and the composition of gut microbiota. Benef. Microbes. 12, 31–42. doi: 10.3920/BM2020.0023
Wang, Y. Y., Lu, R. Y., Shi, J., Zhao, S., Jiang, X., and Gu, X. (2021). CircORC2 is involved in the pathogenesis of slow transit constipation via modulating the signalling of miR-19a and neurotensin/motilin. J. Cell. Mol. Med. 25, 3754–3764. doi: 10.1111/jcmm.16211
Yi, R., Peng, P., Zhang, J., Du, M., Lan, L., Qian, Y., et al. (2019). Lactobacillus plantarum CQPC02-fermented soybean milk improves loperamide-induced constipation in mice. J. Med. Food 22, 1208–1221. doi: 10.1089/jmf.2019.4467
Yu, T., Zheng, Y. P., Tan, J. C., Xiong, W. J., Wang, Y., and Lin, L. (2017). Effects of prebiotics and synbiotics on functional constipation. Am. J. Med. Sci. 353, 282–292. doi: 10.1016/j.amjms.2016.09.014
Zhao, J., Tian, F., Yan, S., Zhai, Q., Zhang, H., and Chen, W. (2018). Lactobacillus plantarum CCFM10 alleviating oxidative stress and restoring the gut microbiota in d-galactose-induced aging mice. Food Funct. 9, 917–924. doi: 10.1039/c7fo01574g
Zhu, L., Liu, W., Alkhouri, R., Baker, R. D., Bard, J. E., Quigley, E. M., et al. (2014). Structural changes in the gut microbiome of constipated patients. Physiol. Genomics 46, 679–686. doi: 10.1152/physiolgenomics.00082.2014
Zhuang, M., Shang, W., Ma, Q., Strappe, P., and Zhou, Z. (2019). Abundance of probiotics and butyrate-production microbiome manages constipation via short-chain fatty acids production and hormones secretion. Mol. Nutr. Food Res. 63:e1801187. doi: 10.1002/mnfr.201801187
Keywords: constipation, probiotics, postbiotics, synbiotics, gut microbial regulation, short-chain fatty acids
Citation: Zhao Y, Liu Q, Hou Y and Zhao Y (2022) Alleviating effects of gut micro-ecologically regulatory treatments on mice with constipation. Front. Microbiol. 13:956438. doi: 10.3389/fmicb.2022.956438
Received: 30 May 2022; Accepted: 18 July 2022;
Published: 09 August 2022.
Edited by:
Vincenzina Fusco, Italian National Research Council, ItalyReviewed by:
Laura Mitrea, University of Agricultural Sciences and Veterinary Medicine of Cluj-Napoca, RomaniaWenyi Zhang, Inner Mongolia Agricultural University, China
Copyright © 2022 Zhao, Liu, Hou and Zhao. This is an open-access article distributed under the terms of the Creative Commons Attribution License (CC BY). The use, distribution or reproduction in other forums is permitted, provided the original author(s) and the copyright owner(s) are credited and that the original publication in this journal is cited, in accordance with accepted academic practice. No use, distribution or reproduction is permitted which does not comply with these terms.
*Correspondence: Yiqing Zhao, eWlxaW5nLnpoYW9AaHlwcm9jYS5jb20=