- 1Hunan Key Laboratory of Economic Crops Genetic Improvement and Integrated Utilization, Hunan University of Science and Technology, Xiangtan, China
- 2Department of Chemical Engineering, Xiangtan University, Xiangtan, China
Gray mold caused by Botrytis cinerea, a necrotrophic plant pathogen, is one of the most damaging diseases of tomato, resulting in both pre- and post-harvest losses. (E)-2-Hexenal dose-dependently inhibited the mycelial growth of B. cinerea, and caused distortion of mycelia and loss of the cytoplasm content, thus altering the morphology of B. cinerea hyphae. To understand molecular processes in response to (E)-2-hexenal, transcriptome sequencing was carried out using RNA-Seq technology. RNA-Seq results revealed that a total of 3,893 genes were differentially expressed in B. cinerea samples treated with (E)-2-hexenal fumigation. Among these genes, 1,949 were upregulated and 1,944 were downregulated. Moreover, further analysis results showed 2,113 unigenes were mapped onto 259 pathways in Kyoto Encyclopedia of Genes and Genomes (KEGG). Moreover, (E)-2-hexenal stress affected the expression of genes involved in the pathways of cell wall, cell membrane, and energy metabolism. KEGG pathway analysis showed that the terpenoid backbone biosynthesis and steroid biosynthesis were the most enriched in ergosterol biosynthetic process transcriptome data. Particularly, (E)-2-hexenal fumigation had influenced ergosterol biosynthetic gene expression levels (e.g., ERG1, ERG3, ERG4, ERG7, ERG12, ERG13, ERG24, ERG25, ERG26, and ERG27), which were in good agreement with the experimental measurement results, and the ergosterol content decreased. Collectively, the results of this study increase our current understanding of (E)-2-hexenal inhibition mechanisms in B. cinerea and provide relevant information on postharvest shelf life extension and preservation of fruits and vegetables.
Introduction
Botrytis cinerea, a kind of plant necrotrophic pathogen, causes one of the most serious post-harvest diseases that parasitizes on senescent or dead plant tissues and produces gray mold and fruit softening (Blanco-Ulate et al., 2015). Its hyphae can infiltrate plant organs or tissues by means of natural openings or wounds, spread from dead tissues, and then colonize in healthy tissues (Kumar and Kirti, 2015).
It was presumed that a thin or defective cuticle prompted plants to activate defense mechanisms constitutively and secrete antifungal ingredients on their surface, consequently restraining B. cinerea development (AbuQamar et al., 2016; Sun et al., 2017). Moreover, cuticle metabolic pathways might immediately regulate interactions of the plant and pathogen via intricate lipid signaling pathways (Huysmans et al., 2017) or hormone-regulated defense pathways, resulting in hypersensitive cell death (Mang et al., 2009; Ni et al., 2016).
Ergosterol (ERG) is known as the primary sterol of fungi, except for certain rust fungi, and is of vital importance in cell membranes (Gasecka et al., 2018). Ergosterol takes part in many biological functions, such as regulation, membrane fluidity, cell cycle, and activity (Alcazar-Fuoli and Mellado, 2013; Hokken et al., 2019). About 20 enzymes are involved in the biosynthesis of ergosterol, and its pathway synthesizes squalene from mevalonate gradually (Long et al., 2017). The hydrophobic properties of essential oils (EOs) allows them to easily to enter the lipids of the cell membrane and mitochondrial membrane, thus destabilizing the cell structure, damaging membrane integrity, and increasing cellular membrane permeability (Oliveira et al., 2020). Green leaf volatiles (GLV) are produced in large amounts by green plants, among which (E)-2-hexenal is a natural substitute for the antifungal substance with a defensive reaction (Scala et al., 2013), which has a great application prospect in preserving agricultural products (Sun et al., 2022). (E)-2-Hexenal, a kind of plant volatile compound, has been well recorded that it can improve plant resistance to pathogens by stimulating accumulation of antifungal substances including PDF1.2, PR-3, and camalexin (Kunishima et al., 2016). It has been proved experimentally that (E)-2-hexenal with a concentration of 369 μL/L exerts the highest antifungal effect in all volatile substances (Menniti et al., 2010).
Prost et al. (2005) suggested that trans-2-hexenal has 100% growth inhibition against B. cinerea at 100 mM after 24 h of treatment and severely destroys fungal membranes and cell walls. Previous in vitro experiments showed that several volatile ingredients in tomato leaves showed inhibitory effects on B. cinerea in Lycopersicon esculentum (Zhang et al., 2018). Natural plant compounds including citral, benzoic acid, and cuminic acid could hinder respiration enzymatic activity including ATP synthesis and condensation reaction of acetyl-CoA in some fungi (Zheng et al., 2015; Wang et al., 2016). However, the molecular mechanisms of these processes remain unclear and require extensive investigation. Preparation work must be carried out to provide a starting point for in-depth research, particularly into complex mechanisms of (E)-2-hexenal on the inhibitory effect of B. cinerea. In many biological species, RNA-Seq is used for whole-genome expression analysis and is an increasingly attractive method for reliable and efficient transcriptome sequencing.
Thus far, the molecular mechanism of resistance of (E)-2-hexenal to mycelial growth of B. cinerea is not fully understood. In this study, the efficacies of (E)-2-hexenal in controlling B. cinerea infestation and its spore germination were evaluated. To further elucidate the inhibitory mechanism of (E)-2-hexenal against B. cinerea, changes in global gene expression profiles in B. cinerea mycelia between control and (E)-2-hexenal treatment were evaluated by transcriptomic analysis, and their impact on the spore germination of B. cinerea was analyzed. Physiological and biochemical validation experiments confirmed the transcriptional changes observed. This study provides evidence for identifying genes involved in or some crucial metabolic pathways and developing a safe and effective method for controlling B. cinerea infestation in agricultural products using (E)-2-hexenal.
Materials and methods
Chemicals, fungal culture, and spore suspension preparation
(E)-2-Hexenal (purity 99%; CAS: 6728-26-3) was purchased from Sigma-Aldrich (Shanghai, China) and stored in accordance with the manufacturer’s specifications. Propidium iodide (PI) was also acquired from Sigma-Aldrich (Shanghai, China). Isopropanol and trichloromethane were purchased from Sinopharm Group Co., Ltd., (Beijing, China).
Botrytis cinerea spores were cultured on potato dextrose agar (PDA) medium (1 L of an infusion from potatoes, 15 g/L agar, and 20 g/L glucose) for 5 days at 28°C. Spores were collected from the B. cinerea culture dish with 0.1% (v/v) Tween 80 saline, and then the mycelium was filtered out. The suspension of B. cinerea spores was adjusted to 1.0 × 106 spores/mL measured using a hemocytometer.
Determination of antifungal activity of (E)-2-hexenal
Effects of (E)-2-hexenal on the mycelial growth of B. cinerea were measured in vitro by using the agar dilution method (Yahyazadeh et al., 2008). Primarily, high-concentration storage solution (50 μL/mL) of liquid (E)-2-hexenal was prepared by dissolving it in anhydrous ethanol and then filtered by filter sterilization. PDA (20 mL) was poured into sterile Petri dishes (90 mm diameter), and the determined amounts of (E)-2-hexenal were added to PDA mediums (plus 0.05% Tween 80) to obtain desired concentrations of 0, 10, 20, 40, 80, 160, and 320 μL/L. Inocula with a 6-mm-diameter disk were cut from the PDA plates using a punching bear and then were placed at the center of each new Petri plate. After that, the plates were incubated at 28 + 2°C for 48 h. Each treatment was carried out in triplicate. The percentage of inhibition of mycelial growth (MGI) was computed using the following formula:
where dc (cm) is the average colony diameter of the control group and dt (cm) is the average colony diameter of the treatment group. The lowest concentration that completely restrained the growth of the fungus was regarded as the minimum inhibitory concentration (MIC). The minimum fungicidal concentration (MFC) was considered as the lowest concentration that restrained the growth of the pathogen in a fresh PDA plate after a 72-h incubation at 28 + 2°C, manifesting more than 99.5% killing of the initial inoculum (Talibi et al., 2012).
Measurement of extracellular pH and extracellular conductivity
According to the previous method, the extracellular pH and conductivity of B. cinerea cells (B05.10), which were obtained from the China General Microbiological Culture Collection Center (Preservation No. CGMCC 3.3790, Beijing, China), were measured using a Delta 320 pH Meter (Mettler-Toledo, Greifensee, Switzerland) and a DDS-12DW Conductivity Meter (Bante Instrument Co., Ltd. Shanghai, China) in accordance with the specifications, respectively (Shao et al., 2013). After the addition of (E)-2-hexenal, the extracellular pH and conductivity of B. cinerea cells treated at a minimal inhibitory concentration (MIC) or minimal fungicidal concentration (MFC) concentration were selected at 0, 30, 60, and 120 min for treatment. The control did not have any (E)-2-hexenal. Results were presented as pH in the growth medium and amount of extracellular conductivity (μs/cm) at each interval of incubation.
DNA leakage determination
According to the previous method, cell constituents were released into the supernatants and determined with minor modifications (Paul et al., 2011). In brief, B. cinerea cells were centrifuged at 4,000 g for 20 min and collected from 100 mL potato dextrose broth (PDB), and the particles were eluted three times with sterile water, which were resuspended in phosphate-buffered solution (100 mL, pH 7.0). The suspensions were treated with various concentrations (0, 1 × MIC, and 1 × MFC) of (E)-2-hexenal for 0, 30, 60, and 120 min. Subsequently, test specimens (2 mL) were collected and centrifuged for 2 min at 12,000 g. In order to measure the concentration of released components, the absorbance of these supernatant solutions (1 mL) was determine at 260 nm by using the UV-2450 UV/Vis Spectrophotometer (SHIMADZU international trade Co. Ltd., Shanghai, China).
Potassium ions efflux
The amount of the potassium ions was determined according to the method described previously (Tao et al., 2014). After fluid solutions containing B. cinerea were exposed to (E)-2-hexenal at 1 × MIC or 1 × MFC for a short-term period (0, 30, 60, and 120 min), free potassium ions in suspensions of fungal cells were measured. At each preset interval, the concentration of extracellular potassium was determined by flame atomic absorption spectroscopy following a photometric procedure (Shimadzu AA6300, Japan). Moreover, control in flasks with no (E)-2-hexenal was measured. The final results were presented as micrograms per milliliter free potassium ions (μg/mL) in the culture medium in each incubation interval.
Scanning electron microscopy
The fungi (6-day-old) cultured on potato dextrose agar (PDA) plates were handled with (E)-2-hexenal at three concentrations (0, 1 × MIC, 1 × MFC, 8 × MFC, and 16 × MFC) for 2 h and were observed by utilizing scanning electron microscopy (SEM) (Helal et al., 2007; Yahyazadeh et al., 2008). The segments (5 × 10 mm) were removed from PDA plates, quickly placed into 0.05 M phosphate buffer (pH 6.8) containing 3% (v/v) glutaraldehyde, and then stored at 4°C. The samples were stored in this solution for 48 h to fix and then washed with distilled water for three times and 20 min each. Next, they were dehydrated in a series of ethanol solutions (30, 50, 70, and 95%, v/v) for 20 min, respectively, and finally handled with pure ethanol for 45 min. The samples were then dried at the critical point of liquid carbon dioxide. The segments of B. cinerea were dried in desiccators until further use. After drying, the prepared samples were placed on 1/2 in SEM stubs with double-slide adhesive labels, and gold–palladium particles were sprayed by a Polaron SEM Coating System sputter coater. The test specimens were observed under a JSM-6360 LV SEM (JEOL, Ltd, Tokyo, Japan), which was operated at 25 kV at 3000 × magnification. All the tests were performed in triplicate.
Plasma membrane integrity of Botrytis cinerea spore assay
Membrane integrity assay was carried out according to the procedure described by Shao et al. (2015). B. cinerea spores (5 × 105 spores/mL) were handled with different dosages of (E)-2-hexenal (0, 8 × MFC, 16 × MFC). After 2 h of incubation on a rotary shaker which was operated at 25°C at 200 rpm, spores were centrifuged and collected at 8,000 × g. Finally, they were stained for 5 min at 30°C with 10 μg/mL propidium iodide (Sigma-Aldrich, Saint Louis, MO, United States) (Qin et al., 2011; Shao et al., 2015). B. cinerea spores were then centrifuged and collected, sodium phosphate buffer (pH 7.0) was used, and the spores were eluted twice to remove residual dye. The stained spores were viewed under an Axioskop 40 fluorescence microscope (Zeiss, Oberkochen, Germany) with a separate fluorescein filter set (excitation BP 546/12 nm, emission LP 590 nm, Zeiss no. 15). A total of three different fields from each slide which were no less than 20 spores were randomly chosen, and the spore number in the bright field was regarded as the total spore number.
RNA sequencing and transcriptomic data processing
Transcriptomic analyses were performed using (E)-2-hexenal-treated and untreated B. cinerea samples. Hyphae were incubated with 1 × MFC of (E)-2-hexenal for 4 h, collected, and frozen in liquid nitrogen for RNA extraction. In brief, total RNA was extracted from control and (E)-2-hexenal-treated groups using TRIzol® reagent (Thermo Fisher Scientific Co., Ltd., Shanghai, China) according to the manufacturer’s instructions. RNA integrity was assessed using an RNA Nano 6000 Assay Kit of the Bioanalyzer 2100 system (Agilent Technologies, Palo Alto, CA, United States). Total RNA, 3 μg per sample, was applied as the initial material for RNA sample preparation. The high-quality RNA samples were subsequently used to construct cDNA libraries for sequencing on an Illumina HiSeq platform (Genepioneer Biotechnology Company, Nanjing, China). The raw reads were first processed by a Hiseq2000 system and filtered to eliminate low-quality reads to gain clean reads. The nucleotide genome sequence of B. cinerea had been deposited at GenBank, with accession numbers CP009805.1- CP009822.1. The Supplementary Files and transcriptome information had been deposited at the GenBank Sequence Read Archive (SRA) database, with accession number SRR17272254.
Differential expression analysis and functional enrichment
In order to distinguish these DEGs between the two diverse samples, the expression levels of each transcript were computed through the fragments per kilobase per million mapped fragments (FPKM) method. EdgeR was applied for the differential expression analysis (Lun et al., 2016). The DEGs were chosen according to the following criteria: | log2 FC| ≥ 1, the false discovery rate (FDR) less than 0.05, and the FC (fold change) calculated by separating the expression level (FPKM) of each gene in SE1 by that in SE0.
To annotate the function of these differentially expressed genes (DEGs), Gene Ontology (GO) analysis was conducted by using the GOseq R software package (Young et al., 2010). They contained three main categories: cellular component, biological process, and molecular function. The identified DEGs were used as biological pathways enriched through KEGG pathway analyses which were performed by KOBAS software (Mao et al., 2005).
Real-time fluorescence quantitative PCR
After exposure to (E)-2-hexenal at 1 × MFC for 4 h, RNA was extracted from B. cinerea cells with TRIzol reagent (Invitrogen, Carlsbad, CA, United States) in accordance with the manufacturer’s instructions. cDNA was synthesized from 2 μg of DNA-free RNA using Moloney Murine Leukemia Virus (M-MLV) Synthesis Kit (Promega, Madison, WI, United States) with oligo dT18. Real-time fluorescence quantitative PCR (RT-PCR) was conducted using a FastStart Universal SYBR Green Master (Roche, Switzerland) on a BIO-RAD CFX Connect real-time PCR detection system. Primers for ergosterol biosynthetic gene which were designed were performed by using Primer Premier for Windows, version 5.0 (Premier Biosoft International, Palo Alto, CA, United States). All primer pairs for expression assays are listed in Table 1. The reaction procedure of RT-PCR was as follows: 95°C for 10 min, followed by 40 cycles of 95°C for 15 s and 60°C for 1 min. The relative expression level was calculated based on the 2–ΔΔCT method using actin gene as an internal reference (Livak and Schmittgen, 2001). In total, three biological replicates were carried out for all qPCR experiments.
Determination of ergosterol content in cell membranes
After B. cinerea cells were exposed to (E)-2-hexenal at the MFC for 0, 30, 60, and 120 min, total ergosterol contents were measured by using the previous method with slight alterations (Tao et al., 2014). A measure of 0.0050 g ergosterol standard sample was accurately weighed and dissolved in 95% ethanol. The volume was then constant to final volume of 50 mL, and the concentration of ergosterol liquid storage solution was 0.1 mg mL–1. The absorbance value A was determined at 282 nm using a TU-19 UV/VIS spectrophotometer (Purkinje General Instrument Co, Ltd. Beijing, China). According to the absorbance value A obtained, the mass concentration of solution C was determined by the standard curve. Control was the samples without (E)-2-hexenal treatment.
Statistical analysis
All data were represented as mean values in triplicate and + standard deviation (S.D.) and were analyzed using Excel 2007 (Microsoft Co., Redmond, WA, United States) to produce the figures. Analysis of variance (ANOVA), followed by Duncan’s multiple range test, was employed for statistical evaluation. The data were processed using SPSS (version 18.0; IBM, Chicago, IL, United States).
Results
Extracellular conductivity
The results of extracellular conductivity from B. cinerea cells exposed to (E)-2-hexenal (0, 1 × MIC, and 1 × MFC) for a period of 0–120 min are shown in Figure 1A. When exposed to (E)-2-hexenal at 1 × MIC and 1 × MFC for 30 min, the values of extracellular conductivity were 70.0 and 76.5 μs/cm, respectively, significantly higher than those of the control (62.0 μs/cm) (P < 0.05). The extracellular conductivity increased with the increase in the treatment concentration and exposure time. At 120 min of exposure, the extracellular conductivity of B. cinerea suspensions with (E)-2-hexenal at 1 × MIC and 1 × MFC obviously increased, with conductivity reaching 89.5 μs/cm for 1 × MIC and 104.0 μs/cm for 1 × MFC, respectively. However, the change in extracellular conductivity for control (64.0 μs/cm) was almost unchanged.
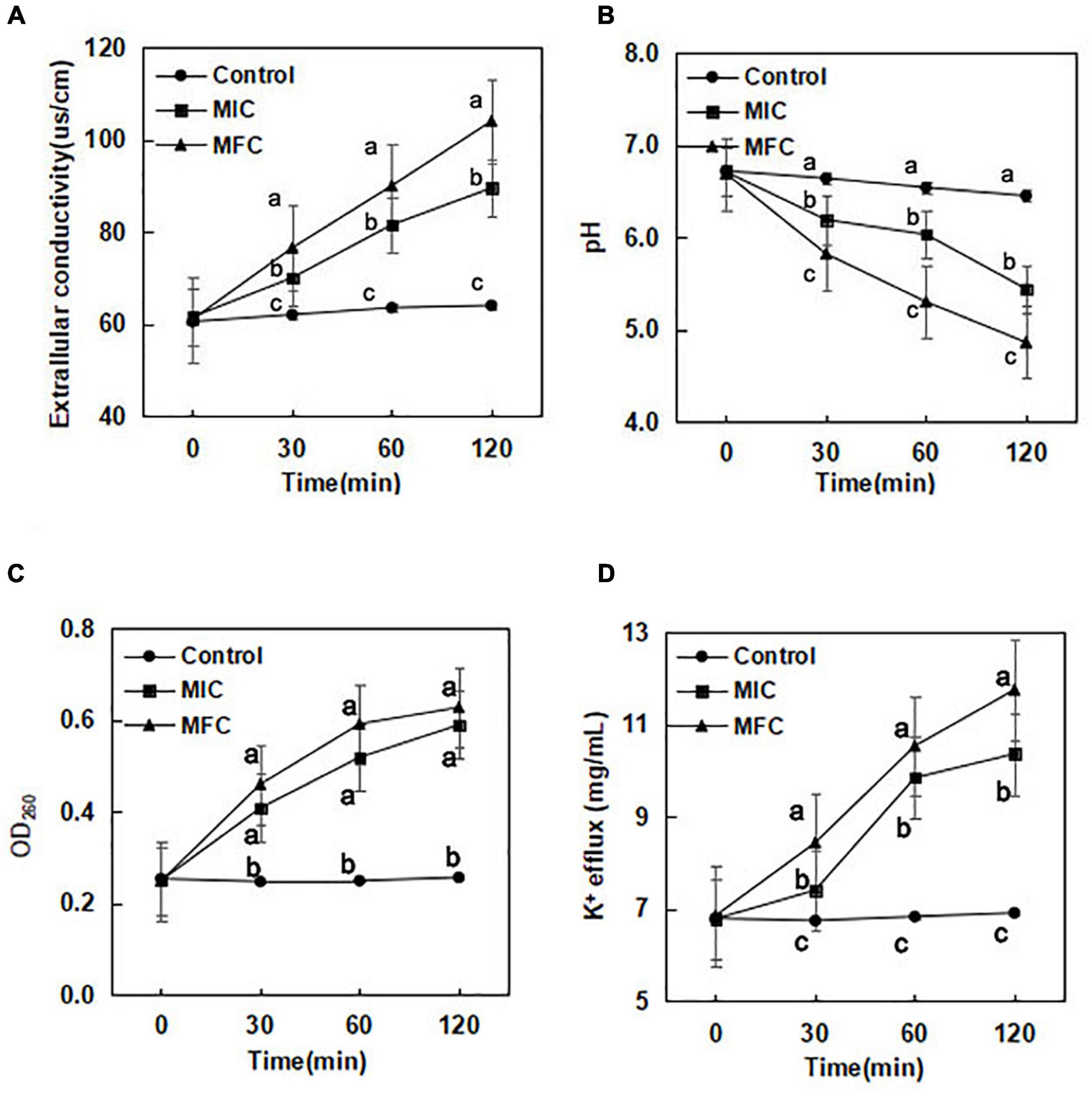
Figure 1. Effect of (E)-2-hexenal on extracellular conductivity (A), extracellular pH (B), 260 nm absorbing material release (C), and K+ efflux (D) of B. cinerea [(◆):1 × MIC; (■):1 × MFC; (●): control]. Data presented are the means of pooled data. Error bars indicate the SDs of the means (n = 6). Symbols with different letters at each time point indicate significant differences according to Duncan’s multiple range test (p < 0.05).
Extracellular pH
The extracellular pH of B. cinerea cells exposed to (E)-2-hexenal is presented in Figure 1B. A downward trend in extracellular pH was observed for the control, 1 × MIC, and 1 × MFC. A sharp decline in extracellular pH occurred at 1 × MFC and 1 × MIC. After incubation with (E)-2-hexenal at 1 × MIC and 1 × MFC for 30 min, the extracellular pH values in B. cinerea suspensions were 6.19 and 5.82, respectively, which were obviously lower than those of control (6.64) (P < 0.05). The extracellular pH values in B. cinerea suspensions after incubation with (E)-2-hexenal at 1 × MIC and 1 × MFC for 120 min were 5.42 and 4.82, respectively, which were significantly lower than those of control (6.45) (P < 0.05).
DNA release
The results of the release of DNA when B. cinerea was treated with (E)-2-hexenal at three different concentrations (0, 1 × MIC, and 1 × MFC) for 0, 30, 60, and 120 min, respectively, are shown in Figure 1C. After B. cinerea cells were treated with (E)-2-hexenal at different concentrations, an immediately significant (P < 0.05) increase in the release of DNA was observed. The OD260 values in B. cinerea suspensions without (E)-2-hexenal remained constant throughout the treatment period. The OD260 value in the B. cinerea suspensions treated with (E)-2-hexenal at 1 × MIC for 120 min was 0.59, the value was much higher (P < 0.05) than that of control (0.26) and slightly lower than that at 1 × MFC (0.63).
Potassium ion efflux
Potassium ions (K+) leaked from B. cinerea mycelia when incubated with (E)-2-hexenal (Figure 1D). (E)-2-Hexenal at 1 × MFC could significantly induce K+ release during the initial 30 min of treatment course, and the potassium ion concentration was 8.43 μg/mL after (E)-2-hexenal at 1 × MFC was treated for 30 min. When the processing time reached 120 min, K+ release at 1 × MFC of (E)-2-hexenal continuously increased and reached 11.75 μg/mL. By comparison, treatment with (E)-2-hexenal at 1 × MIC (10.36 μg/mL) resulted in more K+ release than control (6.91 μg/mL) after B. cinerea mycelia was incubated for 120 min.
Effect of (E)-2-hexenal on the mycelium and spores of surface morphology of Botrytis cinerea
Scanning electron microscopy images showed treatment with (E)-2-hexenal at 8 × MFC or 16 × MFC for 2 h obviously altered the surface morphology of B. cinerea spores (Figure 2A). The use of high concentrations of (E)-2-hexenal (8 × MFC and 16 × MFC) was determined by repeated verification using tomato inoculation experiments (data not shown). The effects of (E)-2-hexenal on the surface morphology of B. cinerea mycelia could be obtained by observing the surface ultrastructure by SEM (Figure 2B). Particularly, compared with control, when treated with (E)-2-hexenal at 8 × MFC, each spore displayed depressed and shrunken spore surfaces. By contrast, (E)-2-hexenal at 16 × MFC disposed B. cinerea spores after 2 h of exposure, which appeared severely collapsed, and most of these spores had ruptured because of the lack of cytoplasm. At the same time, compared with control, B. cinerea hyphae subjected to (E)-2-hexenal at 1 × MIC for 2 h displayed some changes in surface morphology, showing a little shrinkage; however, it was still smooth and full. However, when the hyphae was treated with 1 × MFC of (E)-2-hexenal, the severe morphological changes have occurred, the hyphae had twisted, shrunk, and even ruptured.
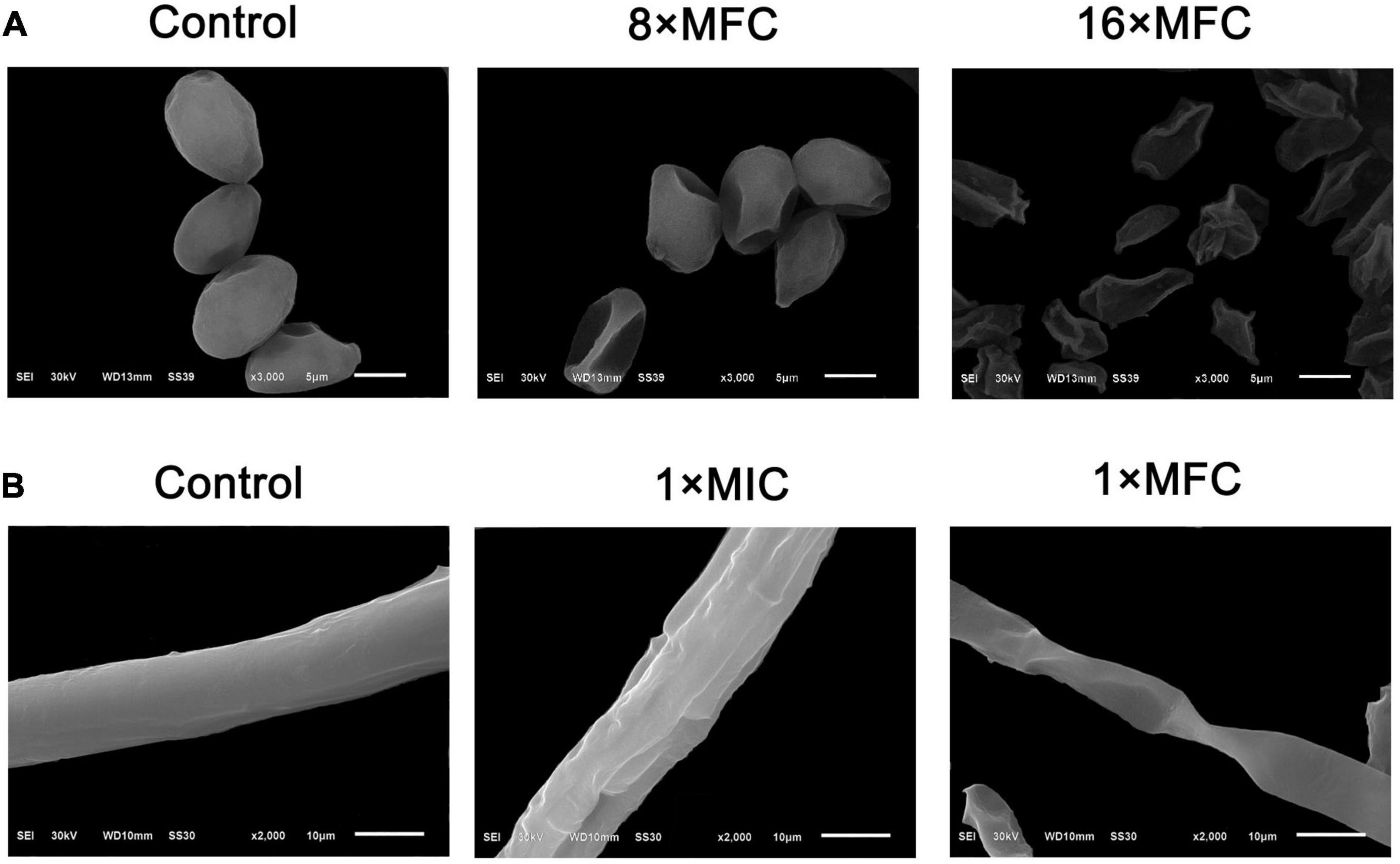
Figure 2. Scanning electron microphotography of B. cinerea spores and hyphae after treatment with (E)-2-hexenal. (A) Spores of untreated, 8 × MFC, and 16 × MFC (E)-2-hexenal; (B) Mycelia of untreated, 1 × MIC (160 μL/L), and 1 × MFC (320 μL/L) (E)-2-hexenal.
Effect of (E)-2-hexenal on the plasma membrane integrity of Botrytis cinerea spores
In order to evaluate the action mode of (E)-2-hexenal on B. cinerea spores, the integrity of B. cinerea spore cell membranes was measured using an Axioskop 40 fluorescence Zeiss microscope (Zeiss, Oberkochen, Germany). Their plasma membranes were distinctly destroyed by (E)-2-hexenal. These were positively correlated with reagent concentration and processing time (Figure 3). Untreated control emerged to have intact plasma membranes, whereas macroscopic observation by using a fluorescence microscope showed that all the spore plasma membranes with (E)-2-hexenal at 16 × MFC were destroyed. However, several spore plasma membranes with (E)-2-hexenal at 8 × MFC were damaged. The experimental results of PI staining and SEM showed that the integrity of the B. cinerea plasma membrane distinctly decreased with the increase in reagent concentration (Figures 2, 3).
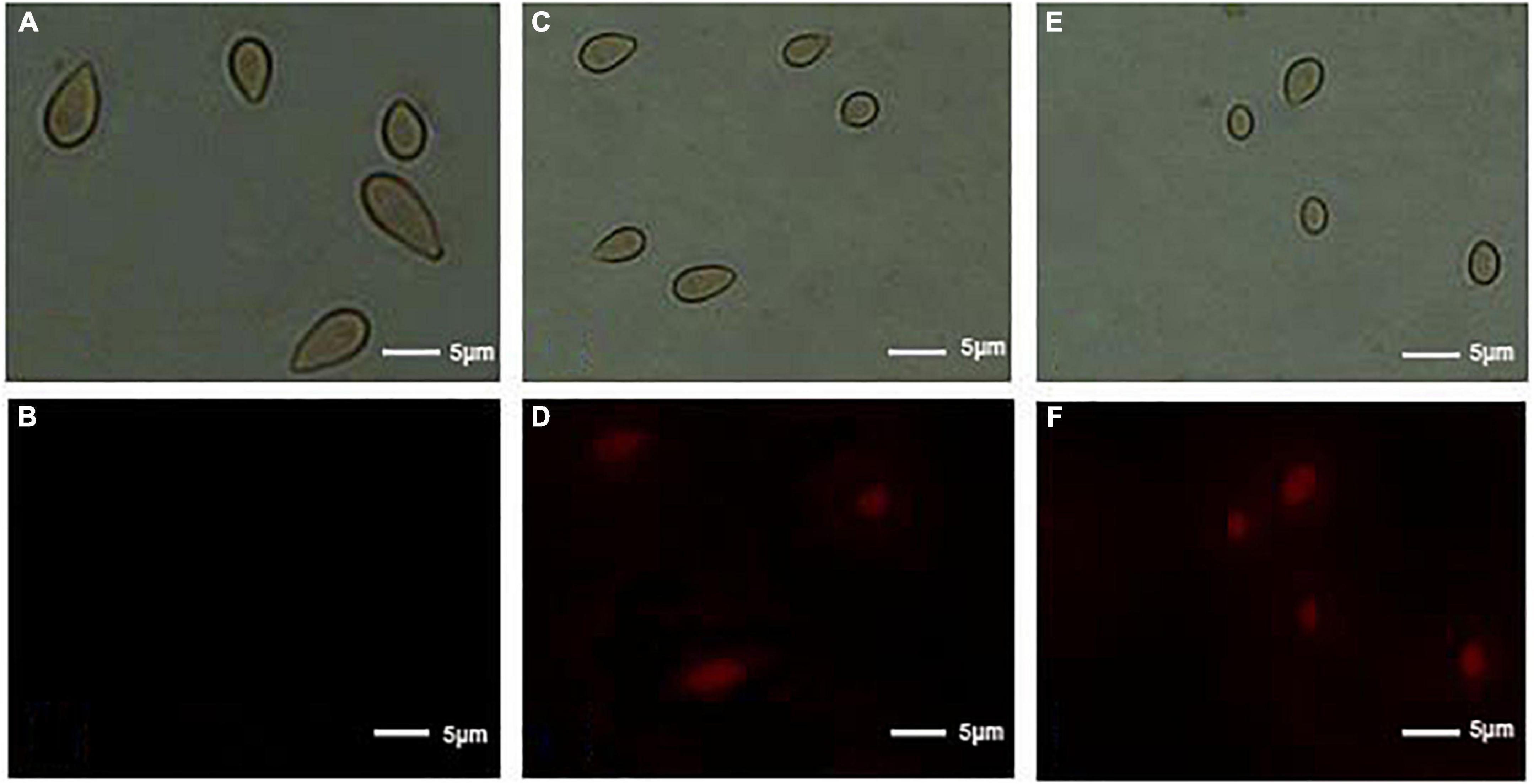
Figure 3. Effect of control, 8 × MFC, and 16 × MFC (E)-2-hexenal on the membrane integrity of B. cinerea spores. Spores were cultured at 28 + 2°C in PDB medium supplemented with 0 (A, bright field; B, PI) or 8 × MFC (C, bright field; D, PI), and 16 × MFC (E, bright field; F, PI) for 2 h of incubation, and spores were stained with the PI and observed with a fluorescence microscope. The scale bar indicated the length of 5 μm.
Overall transcriptome profiles Botrytis cinerea
In order to determine their transcriptome profiles of B. cinerea in CK and (E)-2-hexenal-treated groups, a congregated cDNA specimen from each group was sequenced separately using the Illumina sequencing platform. The concrete contents in sequence assembly and information annotation are presented in Table 2. After the raw reads were filtered, 23.6 and 23.1 million clean reads were accessible from CK and (E)-2-hexenal, respectively. A total of 288,357 unigenes were entirely utilized for functional annotation. Among them, 107,120 unigenes were annotated to the GO database. The total number of identified DEGs were 3,893 between CK and (E)-2-hexenal, which included 1,949 upregulated and 1,944 downregulated genes (Table 2). The number of upregulated genes was almost equal to that of downregulated genes.
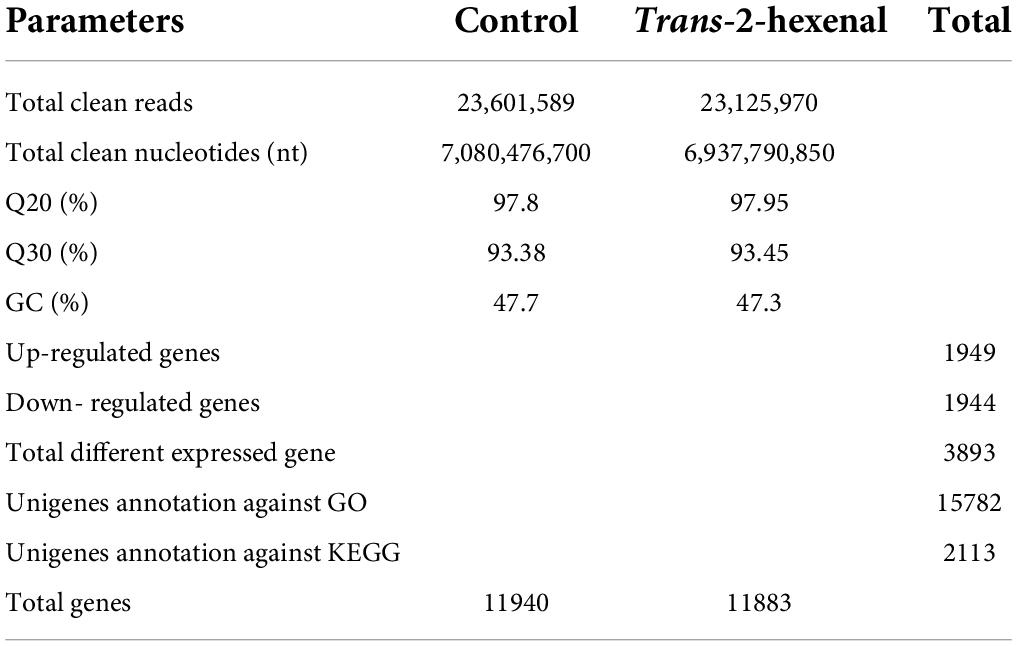
Table 2. Summary data of reads in control (CK) and (E)-2-hexenal-treated B. cinerea mycelia transcriptomes.
Web Gene Ontology Annotation Plot (WEGO) was utilized to visualize and plot the GO annotation. Annotated unigenes were classified by GO assignments into three ontologies with large amounts of cellular component (6,927), followed by biological process (5,366), and molecular function (2,889) (Figure 4). In metabolic process, single-organism metabolic process, phosphate-containing compound metabolic process, and phosphorus metabolic process are the most enriched functional groups with 583, 179, and 188 unigenes, respectively. In molecular function, binding and catalytic activities were the top two groups. Cell part, cell, and organelle in cellular components compose the highest percentage, together with unigenes abundance in the cytoplasm (943) maximally, membrane (786), integral component of membrane (679), nucleus (611), etc.
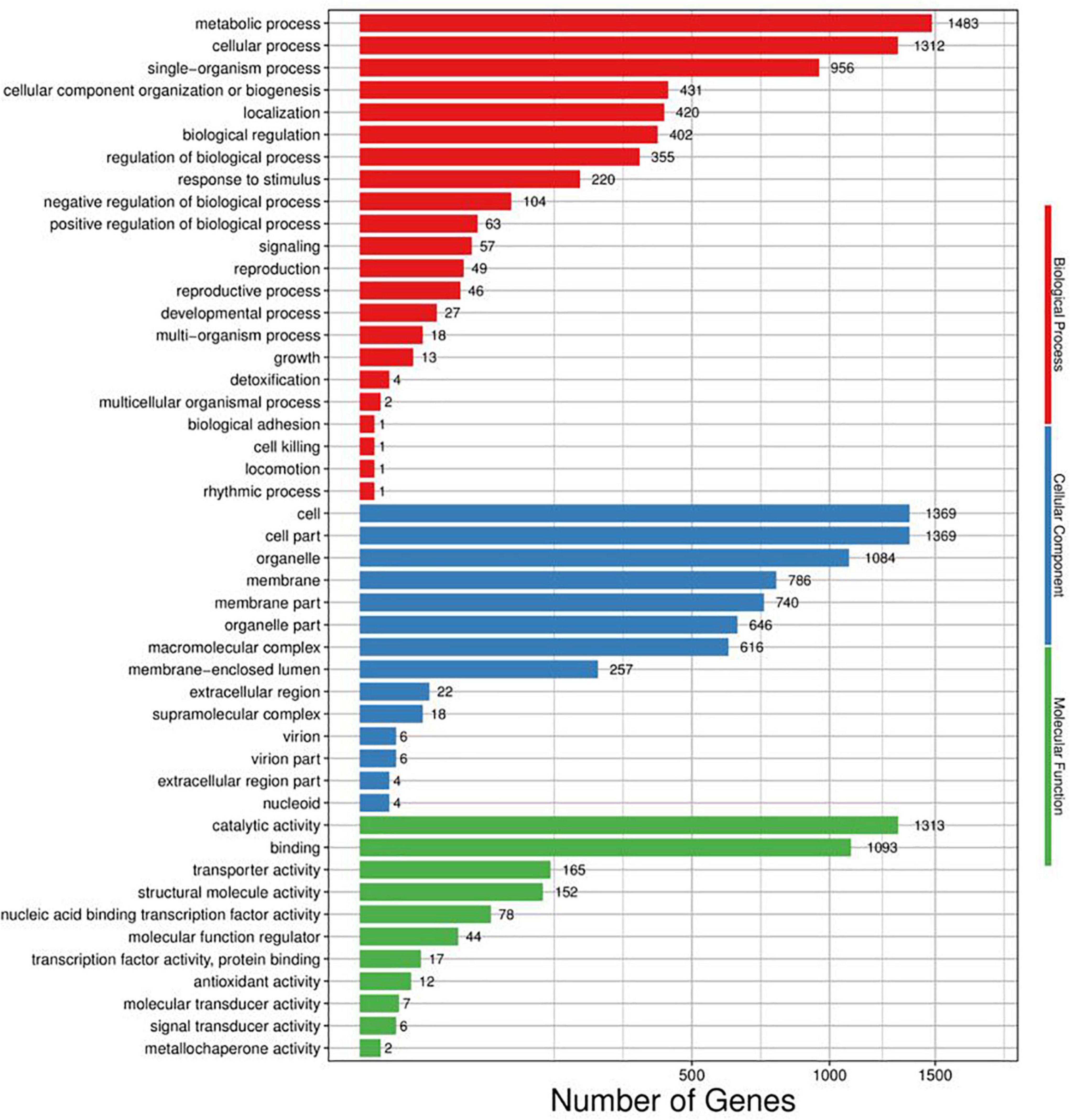
Figure 4. Distribution of genes differentially regulated in response to (E)-2-hexenal in the three main categories. The number of DEGs belonging to the most represented categories and the P-values of gene ontology enrichment are shown.
Among these DEGs, 2,113 DEGs in total were mapped to the KEGG database and added up to 259 pathways (Figure 5). The concrete pathways related to environmental information processing, such as ABC transporters (2, 0.09%), plant–pathogen interaction (1, 0.05%), MAPK signaling pathway (29, 1.37%), and phosphatidylinositol signaling (6, 0.28%); some pathways associated with the cell membrane, such as steroid biosynthesis (9, 0.43%), fatty acid biosynthesis (6, 0.28%), and amino acid biosynthesis (62, 2.93%); some pathways related to energy, citrate cycle (4, 0.19%), starch and sucrose metabolism (17, 0.80%), oxidative phosphorylation (23, 1.09%), and glycolysis/gluconeogenesis (17, 0.80%); and some pathways involved in genetic information, such as RNA degradation (22, 1.04%), RNA polymerase (11, 0.52%), ribosome biogenesis in eukaryotes (13, 0.62%), ribosomes (97, 4.59%), and aminoacyl-tRNA biosynthesis (30, 1.42%), were all impacted by the treatment of (E)-2-hexenal (Supplementary Table S1).
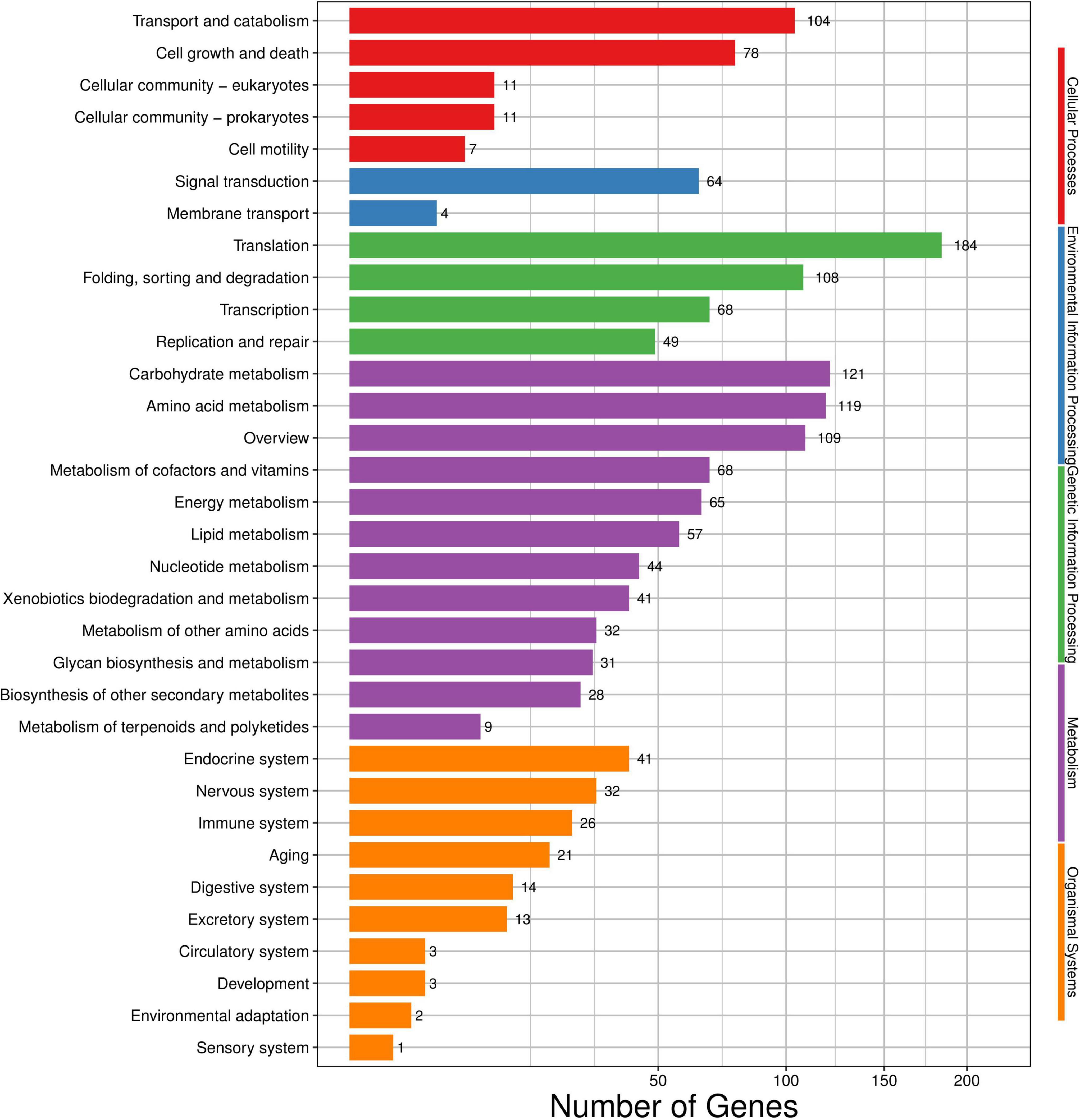
Figure 5. Kyoto encyclopedia of genes and genomes (KEGG) categories mapped from the annotated proteins. The vertical axis lists the names of pathways in the KEGG database, and the horizontal axis shows the proportion of annotated genes in each pathway.
Genes associated with cell wall biosynthesis
The cell wall is the outer layer of the cell membrane which protects the cell from osmotic strength and mechanical injures and establishes the cell shape. We discovered that several pathways related to cell wall biosynthesis (Supplementary Table S2), such as nucleotide sugar and amino sugar metabolism, and sucrose and starch metabolism, were prominently affected by (E)-2-hexenal. Chitin synthase 1 (CHS1), chitin synthase 5 (CHS5), and 1,3-beta-glucan synthase 1 (FKS1), three crucial cell wall-related genes which encoded for 1,3-β-glucan synthase and chitin synthase, respectively, were predominantly activated. The results obtained in this experiment were in accord with other previous research.
Genes associated with the cell membrane
Several genes were involved in pathways associated with cell membrane metabolism (Supplementary Table S3), such as fatty acid biosynthesis and metabolism, inositol phosphate metabolism, steroid biosynthesis, and unsaturated fatty acid biosynthesis, and their expression levels were altered after exposed to (E)-2-hexenal. Among them, inositol monophosphate 1-phosphatase (IMPA), phosphatidylinositol 3-kinase VPS34 (PIK3C3), acetyl-CoA acetyltransferase 1 (ACAA1), enoyl-[acyl-carrier-protein] reductase 1 (NRBF1), lanosterol synthase (ERG7), and 3-keto-steroid reductase (ERG27) were downregulated, indicating that the integrity of the cell membrane might be destructed. In the current study, (E)-2-hexenal severely disrupted ergosterol biosynthesis in B. cinerea cells (Supplementary Table S4). These results demonstrate that these plasma membranes were a crucial antifungal target for (E)-2-hexenal. Remarkably, the expression quantities of four genes in the biosynthetic pathway of ergosterol, which encoded for lanosterol synthase (ERG7), mevalonate kinase (ERG12), hydroxymethylglutaryl-CoA synthase (ERG13), and 3-keto-steroid reductase (ERG27), were downregulated 6. 3-, 5. 0-, 3. 7-, and 2.9-fold, respectively. On the contrary, expression quantity of 3-ketoacyl-(acyl-carrier-protein) reductase (fabG) was enhanced 2.91-fold.
Effect of (E)-2-hexenal on gene expression levels in ergosterol biosynthesis
In order to determine the reliability of transcriptome expression levels, the ergosterol-related biosynthetic genes were selected for qRT-PCR analysis. These candidate genes play indispensable roles in the biosynthetic pathway of ergosterol (Figure 6). The terpenoid backbone underwent a series of enzymatic reactions by regulating distinct downstream targets to form ergosterol under the catalysis of ERG gene families.
As shown in Figure 7, the candidate genes were either activated or inhibited; however, their expression levels were different in control and the treated samples with (E)-2-hexenal. ERG7 and ERG27 gene expressions in control and the processed samples were repressed, with a higher expression level found in control than that in the processed samples. By contrast, delta(7)-sterol 5(6)-desaturase (ERG3) and methylsterol monooxygenase (ERG25) gene expressions were markedly induced by (E)-2-hexenal, and their expression levels were dramatically increased compared to those in control groups, with 3.14- and 2.93-fold, respectively (Figure 7). Most results were in concordance with RNA-Seq data, which manifesting that RNA-Seq analysis was valid, although the fold change of several detected unigenes by qRT-PCR and sequencing did not exactly match. The expression levels of ERG3 and ERG25 by qRT-PCR analysis were upregulated, whereas the levels were inhibited in RNA-Seq data. Taken together, it can be inferred that (E)-2-hexenal is able to impact the expression quantities of ergosterol biosynthetic gene pathways in B. cinerea cells.
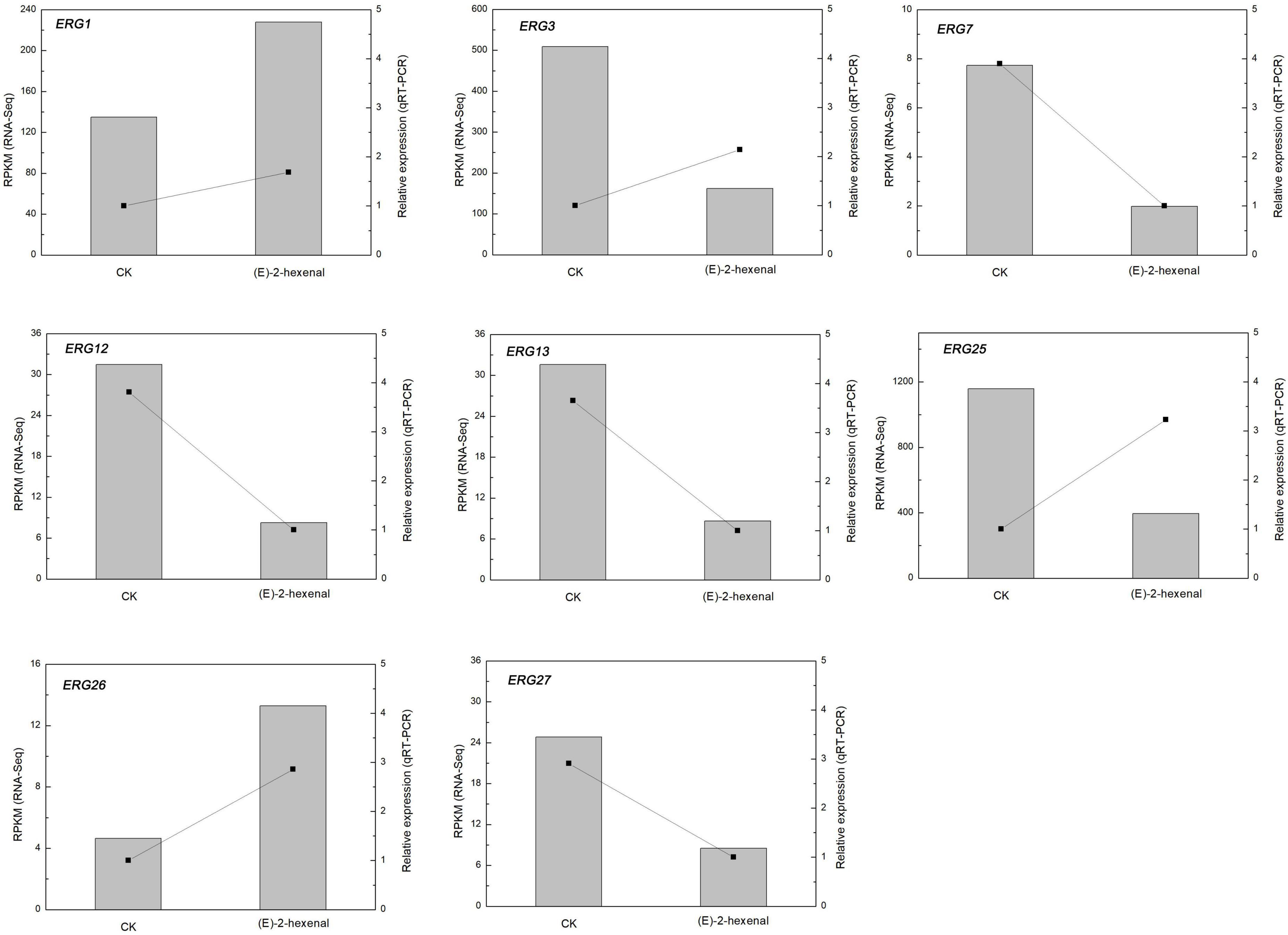
Figure 7. Changes in the expression of ergosterol biosynthesis genes of B. cinerea mycelia treated by CK and 1 × MFC (E)-2-hexenal for 2 h. Data from qRT-PCR were normalized relative to the geometric average of BcRPL5. The relative expression level of each gene in CK was set at 1, and the values are the mean + SD of three replications. The normalized expression level (RPKM) of RNA-Seq is indicated on the y-axis.
Effect of (E)-2-hexenal on ergosterol contents
To verify RNA-Seq analysis and to account for the influence of (E)-2-hexenal on ergosterol biosynthesis further, the ergosterol contents of B. cinerea were determined by using a UV/VIS spectrophotometer. According to the ergosterol standard curve, the ergosterol content in the control and 1 × MFC (E)-2-hexenal-treated samples was determined by measuring the absorbance value at 282 nm. The ergosterol contents of B. cinerea cells treated with (E)-2-hexenal continuously decreased throughout the period, whereas those in the untreated cells were almost constant (Figure 8). The ergosterol content of B. cinerea cells exposed to 1 × MFC of (E)-2-hexenal for 30 min was 3.62 + 0.05 mg/g DW, which was significantly lower than that of control (4.05 + 0.04 mg/g DW). The difference increased with the extension of treatment time. When the incubation time was 120 min, the ergosterol content in treatment cells was 3.08 + 0.04 mg/g DW, as compared to 3.88 + 0.10 mg/g DW in control cells.
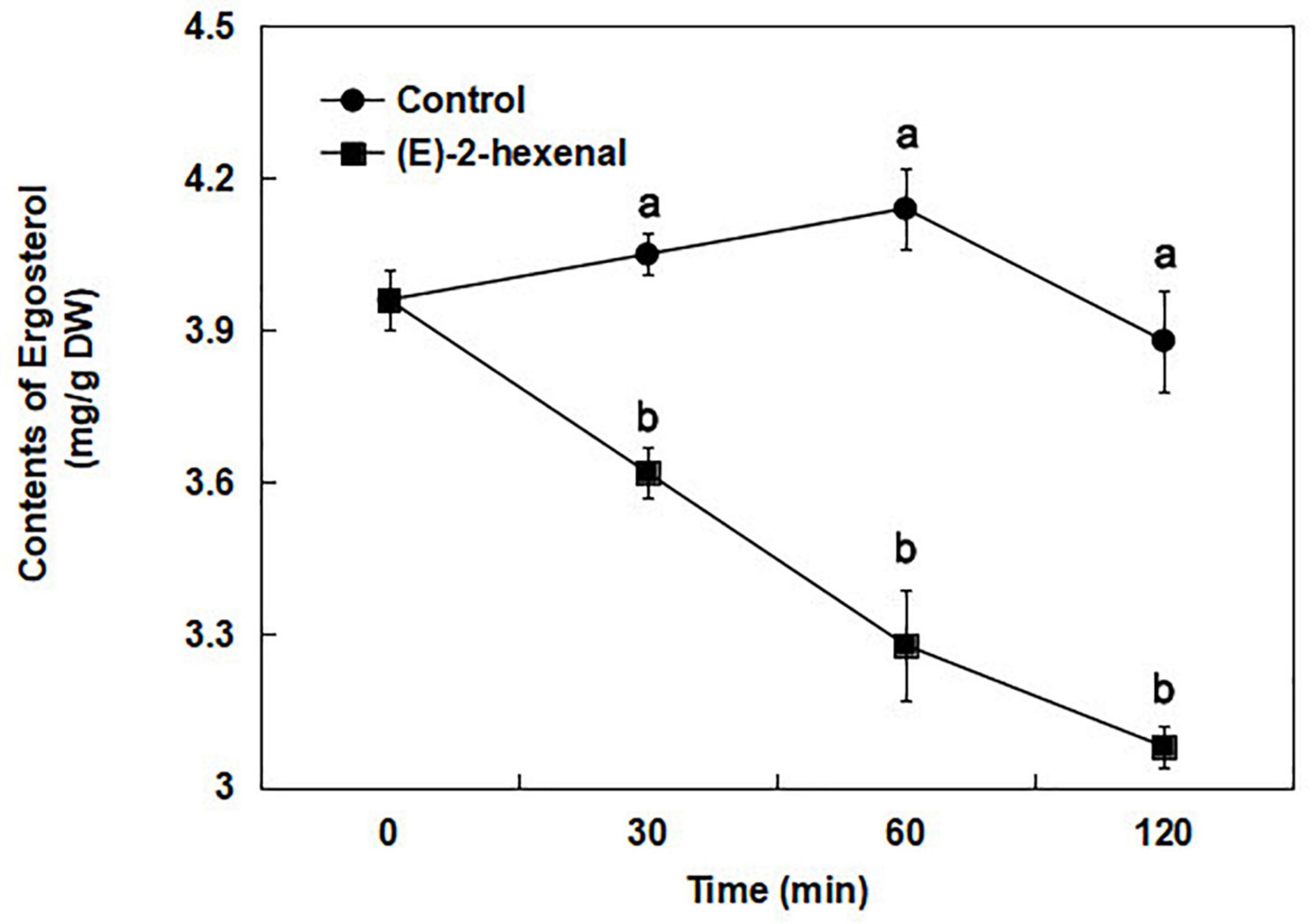
Figure 8. Total ergosterol contents in B. cinerea control (●) and 1 × MFC (E)-2-hexenal (■) treated cells, and values are the mean + SD of three measurements. Symbols with different letters at each time point indicate significant differences according to Duncan’s multiple range test (p < 0.05).
Gene expression associated with energy metabolism
In our study, most of pyruvate-related metabolic genes were downregulated after treatment with (E)-2-hexenal, implying that downstream pathways of pyruvate might be inhibited (Supplementary Table S5), such as pyruvate carboxylase (pyc), D-lactate dehydrogenase (LDHD), pyruvate kinase (pyk), alpha-isopropylmalate synthase (leuA), dihydrolipoamide succinyltransferase (pdhC), and glyoxalase (gloA). However, during the pyruvate metabolic process, individual genes are upregulated, such as malate dehydrogenase (maeB), phosphoenolpyruvate carboxykinase (pckA), and alpha-isopropylmalate synthase (LYS20).
Almost all the acetyl-CoA related to genes were decreased by (E)-2-hexenal, indicating that energy metabolism of B. cinerea in the mitochondria has been interrupted including acetyl-CoA acetyltransferase 1 (ACAA1), pyruvate dehydrogenase (pdhC), pyruvate carboxylase (pyc), biotin holocarboxylase synthetase (HLCS), and N-acetyl-gamma-glutamyl-phosphate reductase (ARG56). In this research, (E)-2-hexenal might also interrupt oxidative phosphorylation and the tricarboxylic acid cycle (TAC) pathway in B. cinerea, leading to the decrease in protein biosynthesis and the ATP content, which are indispensable for hyphal normal growth.
Furthermore, mitochondrial dehydrogenases, which comprise a variety of crucial dehydrogenases for ATP formation such as pyruvate dehydrogenase (pdhC), malate dehydrogenase (RPL28), aldehyde dehydrogenase (gabD), and succinate dehydrogenase (DDC), were immensely suppressed by (E)-2-hexenal. This also affords strong evidence of mitochondrial dysfunction.
Discussion
It was previously reported that (E)-2-hexenal, as a surfactant, is likely permeated by passive diffusion across the plasma membrane. Once (E)-2-hexenal enters cells, biologically crucial nucleophilic groups react with its α, β-unsaturated aldehyde moiety (Patrignani et al., 2015). However, the antimicrobial mechanisms of some other aldehydes, such as ortho-phthalaldehyde and glutaraldehyde, are likely to enhance its permeability and participate in interaction with the cytoplasmic membrane (Zi et al., 2018). Previous studies have shown that (E)-2-hexenal has antifungal activity with MIC 160 μL/L and MFC 320 μL/L, which may be relevant to the fungal membrane damage (Zhang et al., 2017).
Using the RNA-Seq method, Reeder et al. (2017) compared gene expression of Pseudogymnoascus destructans grown in the culture medium, and they were surprised to find that gene expression of the subtilisin protease family was significantly reduced during infection. Korn et al. (2021) suggested that trans-2-hexenal was a safe and known volatile compound that inhibit the growth of plant pathogens, and its presence led to the downregulation of many genes that were thought to be involved in the virulence of P. destructans.
Ergosterol is the key component of fungal lipid membranes and is responsible for maintaining the membrane fluidity and cell integrity (Tian et al., 2015). Commercial fungicides interrupt normal biosynthesis of sterol substances or markedly decrease the amount of ergosterol in Penicillium italicum (Ruan et al., 2017). Ergosterol has also the responsibility to sustain cell integrity and function (Tian et al., 2012; Xu et al., 2017). Fungi alleviate the damage cause by the ergosterol inhibitor to the cell membrane by upregulating the expression of ergosterol biosynthesis-related genes (Yang et al., 2020). ERG11 in A. fumigatus, which was one of genes associated with the ergosterol biosynthesis pathway, was upregulated under amphotericin B pressure (Gautam et al., 2008).
In the current study, several genes might be important candidate genes involved in ergosterol biosynthesis genes, such as ERG1, ERG3, ERG7, ERG12, ERG13, ERG25, ERG26, and ERG27, and most of them were suppressed (Figure 7). The qRT-PCR results of ERG3 and ERG25 were inconsistent with RNA-Seq sequencing results. The probable reasons were in connection with a number of factors, for instance, selection of reference genes and error in manual operation. These experimental results of the expression quantities in the biosynthetic pathway of ergosterol were associated with previous findings.
The decrease in energy metabolism may be a response to or a feedback adverse environmental condition to maintain cell viability (Wang et al., 2018). Natural phytochemicals not only destabilized the cell membranes but also impeded the energy transformation process of the fungus, causing ATP to leak from the fungal mycelium (Leyva Salas et al., 2017). To investigate the in-depth mechanism of possible antifungal actions of (E)-2-hexenal, RNA sequencing was performed to determine its effect on B. cinerea.
Gene ontology term annotation and KEGG pathway enrichment analysis showed that a number of significantly enriched DEGs may be related to the stimulation of concomitant mitochondrial dysfunction and early apoptosis, such as disruption of pyruvate metabolism and reduced ATP synthesis and acetyl-CoA and dehydrogenase activity (Supplementary Tables S3–S5). These results depicted the process of cell membrane damage was enslaved to energy deficit of B. cinerea caused by (E)-2-hexenal treatment, which is thought of as the possible antifungal mechanism of (E)-2-hexenal against B. cinerea by distraction of the TAC of hyphal cells, leading to energy deficiency and cell membrane damage, ultimately accelerating cell death.
In order to survive from extreme environmental changes, eucaryons must swiftly modulate their gene expression patterns to produce a battery of defense mechanisms (Legay et al., 2011; Li et al., 2011). In this research, (E)-2-hexenal caused significant changes in gene expression amounts associated with defense, detoxification, and cell rescue. At the same time, (E)-2-hexenal is proved to effectively inhibit the mycelial growth of pathogenic fungi in a dose-dependent manner. Genes related to the over-represented pathway, such as mRNA surveillance pathway, ribosome biogenesis in eukaryotes, RNA transport and aminoacyl-tRNA biosynthesis, and RNA polymerase, were incompletely depressed, manifesting a harm in these cellular translational activities (Supplementary Table S6). Furthermore, one gene liable for mitochondrial ribosomal protein (MRPS5) was found to be downregulated, indicating a block in mitochondrial dysfunction and mitochondrial translation (Leeuwen et al., 2013).
In the same way, an interruption in energy-associated pathways including TCA, oxidative phosphorylation, gluconeogenesis/glycolysis, nitrogen metabolism, and sulfur metabolism was also discovered. Most of the genes in the aforementioned pathways, such as pyruvate carboxylase (PYC), isocitrate dehydrogenase 2 (IDH2), dihydrolipoamide succinyltransferase (DLAT), enolase (ENO), 3-phosphoglycerate kinase (PGK), 2,3-bisphosphoglycerate-independent phosphoglycerate mutase I (gpmI), ATP synthase 4 (ATP4), NADH dehydrogenase (ubiquinone) 8 (NDUFB8), NADH:ubiquinone oxidoreductase 2 (NDUFV2), cysteine synthase 1 (cysK), cystathionine gamma-synthase (metB), and glutamate-ammonia ligase (glnA), were downregulated (Supplementary Table S6).
Previous studies have demonstrated that (E)-2-hexenal could visibly transform the mitochondrial morphology, restrain the TCA cycle, and reduce 15.93% ATP content of Penicillium digitatum at MFC for 120 min (Zheng et al., 2015). Under extreme environmental conditions, the downregulated energy-requiring processes are a very crucial method to get rid of energy crisis and keep the cells alive (Stasolla, 2010).
The cell membranes maintain functions and cell shapes and are one of the primary targets of essential oils (EOs) to describe their antifungal potential (Siroli et al., 2015). These structural changes in several targets including cell wall, mitochondrion, cell membrane, and inherited matter, have been put forward to illuminate the antifungal activity of essential oils or their volatile ingredients (Shao et al., 2013; Fabra et al., 2016). Salem et al. (2016) reported that these genes were sharply induced when S. cerevisiae cells were exposed to α-terpinene and stimulated compensatory mechanism of cytoderm to conquer the toxicity of terpinene. Terpenoid has the lipophilic property, so it can preferentially enter into the lipid membrane, which leads to increasing fluidity of the bilayer lipid membrane and ultimately increasing membrane permeability (Xu et al., 2017). (E)-2-hexenal could constrain fungal pathogens by disrupting the integrity and permeability of the cell membrane (Ma et al., 2019).
These data provide extensive gene expression information and will certainly accelerate the study of ergosterol biosynthesis and mitochondrial dysfunction in B. cinerea at the level of transcriptional expression when exposed to (E)-2-hexenal.
Data availability statement
The datasets presented in this study can be found in online repositories. The names of the repository/repositories and accession number(s) can be found in the article/Supplementary material.
Author contributions
GS and SD conducted the experiments. QL analyzed the data. HW and MY contributed to the manuscript writing and review. JZ designed the experiments and drafted the manuscript. All authors read and approved the manuscript.
Funding
This research was funded by the Natural Science Foundation of Hunan Province (No. 2019JJ40282), general project of the Education Department of Hunan Province (19C0757), and Talent Acquisition Fund of the Hunan University of Science and Technology (E52062). The authors acknowledge these organizations for financial support.
Conflict of interest
The authors declare that the research was conducted in the absence of any commercial or financial relationships that could be construed as a potential conflict of interest.
Publisher’s note
All claims expressed in this article are solely those of the authors and do not necessarily represent those of their affiliated organizations, or those of the publisher, the editors and the reviewers. Any product that may be evaluated in this article, or claim that may be made by its manufacturer, is not guaranteed or endorsed by the publisher.
Supplementary material
The Supplementary Material for this article can be found online at: https://www.frontiersin.org/articles/10.3389/fmicb.2022.951751/full#supplementary-material
References
AbuQamar, S., Moustafa, K., and Tran, L. (2016). ‘Omics’ and plant responses to Botrytis cinerea. Front. Plant Sci. 7:1658. doi: 10.3389/fpls.2016.01658
Alcazar-Fuoli, L., and Mellado, E. (2013). Ergosterol biosynthesis in Aspergillus fumigatus: its relevance as an antifungal target and role in antifungal drug resistance. Front. Microbiol. 3:439. doi: 10.3389/fmicb.2012.00439
Blanco-Ulate, B., Amrine, K., Collins, T., Rivero, R., Vicente, A., Morales-Cruz, A., et al. (2015). Developmental and metabolic plasticity of white-skinned grape berries in response to Botrytis cinerea during Noble Rot. Plant Physiol. 169, 2422–2443. doi: 10.1104/pp.15.00852
Fabra, M., Flores-López, M., Cerqueira, M., de Rodriguez, D., Lagaron, J., and Vicente, A. (2016). Layer-by-layer technique to developing functional nanolaminate films with antifungal activity. Food Bioproc. Tech. 9, 471–480. doi: 10.1007/s11947-015-1646-1
Gasecka, M., Magdziak, Z., Siwulski, M., and Mleczek, M. (2018). Profile of phenolic and organic acids, antioxidant properties and ergosterol content in cultivated and wild growing species of Agaricus. Eur. Food Res. Technol. 244, 259–268. doi: 10.1007/s00217-017-2952-9
Gautam, P., Shankar, J., Madan, T., Sirdeshmukh, R., Sundaram, C., Gade, W., et al. (2008). Proteomic and transcriptomic analysis of Aspergillus fumigatus on exposure to amphotericin B. Antimicrob. Agents Chemother. 52, 4220–4227.
Helal, G., Sarhan, M., Abu, S., and Abou, E. (2007). Effects of Cymbopogon citratus L. Essential oil on the growth, morphogenesis and aflatoxin production of Aspergillus flavus ML2-strain. J. Basic Microbiol. 47, 5–15. doi: 10.1002/jobm.200510084
Hokken, M., Zoll, J., Coolen, J., Zwaan, B., Verweij, P., and Melchers, W. (2019). Phenotypic plasticity and the evolution of azole resistance in Aspergillus fumigatus; an expression profile of clinical isolates upon exposure to itraconazole. BMC Genom. 20:28. doi: 10.1186/s12864-018-5255-z
Huysmans, M., Lema, S., Coll, N., and Nowack, M. (2017). Dying two deaths - programmed cell death regulation in development and disease. Curr. Opin. Plant Biol. 35, 37–44. doi: 10.1016/j.pbi.2016.11.005
Korn, V., Pennerman, K., Padhi, S., and Bennett, J. (2021). Trans-2-hexenal downregulates several Pathogenicity genes of Pseudogymnoascus destructans, the causative agent of white-nose syndrome in bats. J. Ind. Microbiol. Biot. 48, 1–8. doi: 10.1093/jimb/kuab060
Kumar, D., and Kirti, P. (2015). Pathogen-induced SGT1 of Arachis diogoi induces cell death and enhanced disease resistance in tobacco and peanut. Plant Biotechnol. J. 13, 73–84. doi: 10.1111/pbi.12237
Kunishima, M., Yamauchi, Y., Mizutani, M., Kuse, M., Takikawa, H., and Sugimoto, Y. (2016). Identification of (Z)-3:(E)-2-hexenal isomerases essential to the production of the leaf aldehyde in plants. J. Biol. Chem. 291, 14023–14033. doi: 10.1074/jbc.M116.726687
Leeuwen, M., Krijgsheld, R., Wyatt, K., Golovina, E., Menke, H., Dekker, A., et al. (2013). The effect of natamycin on the transcriptome of conidia of Aspergillus niger. Stud. Mycol. 74, 71–85. doi: 10.3114/sim0013
Legay, G., Marouf, E., Berger, D., Neuhaus, J., Mauch-Mani, B., and Slaughter, A. (2011). Identification of genes expressed during the compatible interaction of grapevine with Plasmopara viticola through suppression subtractive hybridization (SSH). Eur. J. Plant Pathol. 129, 281–301. doi: 10.1007/s10658-010-9676-z
Leyva Salas, M., Mounier, J., Valence, F., Coton, M., Thierry, A., and Coton, E. (2017). Antifungal microbial agents for food biopreservation-A review. Microorganisms 5, 1–35. doi: 10.3390/microorganisms5030037
Li, J., Liu, J., Zhang, H., and Xie, C. (2011). Identification and transcriptional profiling of differentially expressed genes associated with resistance to Pseudoperonospora cubensis in cucumber. Plant Cell Rep. 30, 345–357. doi: 10.1007/s00299-010-0959-9
Livak, K., and Schmittgen, T. (2001). Analysis of relative gene expression data using real-Time quantitative PCR and the 2−ΔΔCT method. Methods 25, 402–408. doi: 10.1006/meth.2001.1262
Long, N., Xu, X., Zeng, Q., Sang, H., and Lu, L. (2017). Erg4A and Erg4B Are required for conidiation and azole resistance via regulation of ergosterol biosynthesis in Aspergillus fumigatus. Appl. Environ. Microb. 83, 1–14. doi: 10.1128/AEM.02924-16
Lun, A., Bach, K., and Marioni, J. (2016). Pooling across cells to normalize single-cell RNA sequencing data with many zero counts. Genome Biol. 17, 1–14. doi: 10.1186/s13059-016-0947-7
Ma, S., Zhang, J., Chen, S., and Zeng, L. (2019). The effects of (E)-2-hexenal on morphological, physiological, and biochemical indices of postharvest disease Penicillium cyclopium spores. J. Food Safety 39:e12700. doi: 10.1111/jfs.12700
Mang, H., Laluk, K., Parsons, E., Kosma, D., Cooper, B., Park, H., et al. (2009). The Arabidopsis RESURRECTION1 gene regulates a novel antagonistic interaction in plant defense to biotrophs and necrotrophs. Plant Physiol. 151, 290–305. doi: 10.1104/pp.109.142158
Mao, X., Cai, T., Olyarchuk, J., and Wei, L. (2005). Automated genome annotation and pathway identification using the KEGG Orthology (KO) as a controlled vocabulary. Bioinformatics 21, 3787–3793. doi: 10.1093/bioinformatics/bti430
Menniti, A., Gregori, R., and Neri, F. (2010). Activity of natural compounds on Fusarium verticillioides and fumonisin production in stored maize kernels. Int. J. Food Microbiol. 136, 304–309. doi: 10.1016/j.ijfoodmicro.2009.10.008
Ni, Y., Guo, N., Zhao, Q., and Guo, Y. (2016). Identification of candidate genes involved in wax deposition in Poa pratensis by RNA-seq. BMC Genom. 17:314. doi: 10.1186/s12864-016-2641-2
Oliveira, D., Furtado, F., Gomes, A., Belut, B., Nascimento, E., Morais, S., et al. (2020). Chemical constituents and antileishmanial and antibacterial activities of essential oils from Scheelea phalerata. ACS Omega 5, 1363–1370. doi: 10.1021/acsomega.9b01962
Paul, S., Dubey, R., Maheswari, D., and Kang, S. (2011). Trachyspermum ammi (L.) fruit essential oil influencing on membrane permeability and surface characteristics in inhibiting food-borne pathogens. Food Control 22, 725–731. doi: 10.1016/j.foodcont.2010.11.003
Patrignani, F., Siroli, L., Serrazanetti, D., Gardini, F., and Lanciotti, R. (2015). Innovative strategies based on the use of essential oils and their components to improve safety, shelf-life and quality of minimally processed fruits and vegetables. Trends Food Sci. Technol. 46, 311–319. doi: 10.1016/j.tifs.2015.03.009
Prost, I., Dhondt, S., Rothe, G., Vicente, J., Rodriguez, M. J., Kift, N., et al. (2005). Evaluation of the antimicrobial activities of plant oxylipins supports their involvement in defense against pathogens. Plant Physiol. 139, 1902–1913. doi: 10.1104/pp.105.066274
Qin, G., Liu, J., Cao, B., Li, B., and Tian, S. (2011). Hydrogen peroxide acts on sensitive mitochondrial proteins to induce death of a fungal pathogen revealed by proteomic analysis. PLoS One 6:e21945. doi: 10.1371/journal.pone.0021945
Ruan, R., Wang, M., Liu, X., Sun, X., Chung, K., and Li, H. (2017). Functional analysis of two sterol regulatory element binding proteins in Penicillium digitatum. PLoS One 12:e0176485. doi: 10.1371/journal.pone.0176485
Reeder, S., Palmer, J., Prokkola, J., Lilley, T., Reeder, D., and Field, K. (2017). Pseudogymnoascus destructans transcriptome changes during white-nose syndrome infections. Virulence 8, 1695–1707. doi: 10.1080/21505594.2017.1342910
Salem, M., Zidan, Y., Mansour, M., Hadidi, N., and AboElgat, W. (2016). Antifungal activities of two essential oils used in the treatment of three commercial woods deteriorated by five common mold fungi. Int. Biodeter. Biodegr. 106, 88–96. doi: 10.1016/j.ibiod.2015.10.010
Scala, A., Allmann, S., Mirabella, R., Haring, M., and Schuurink, R. (2013). Green leaf volatiles: A plant’s multifunctional weapon against herbivores and pathogens. Int. J. Mol. Sci. 14, 17781–17811. doi: 10.3390/ijms140917781
Shao, X., Cao, B., Xu, F., Xie, S., Yu, D., and Wang, H. (2015). Effect of postharvest application of chitosan combined with clove oil against citrus green mold. Postharvest. Biol. Tech. 99, 37–43. doi: 10.1016/j.postharvbio.2014.07.014
Shao, X., Cheng, S., Wang, H., Yu, D., and Mungai, C. (2013). The possible mechanism of antifungal action of tea tree oil on Botrytis cinerea. J. Appl. Microbiol. 114, 1642–1649. doi: 10.1111/jam.12193
Siroli, L., Patrignani, F., Gardini, F., and Lanciotti, R. (2015). Effects of sub-lethal concentrations of thyme and oregano essential oils, carvacrol, thymol, citral and trans-2-hexenal on membrane fatty acid composition and volatile molecule profile of Listeria monocytogenes, Escherichia coli and Salmonella enteritidis. Food Chem. 182, 185–192. doi: 10.1016/j.foodchem.2015.02.136
Sun, H., Zuo, X., Zhang, Q., Gao, J., and Kai, G. (2022). Elicitation of (E)-2-hexenal and 2,3-butanediol on the bioactive compounds in adventitious roots of Astragalus membranaceus var. mongholicus. J. Agric. Food Chem. 70, 470–479. doi: 10.1021/acs.jafc.1c05813
Sun, K., Tuinen, A., van Kan, J., Wolters, A., Jacobsen, E., Visser, R., et al. (2017). Silencing of DND1 in potato and tomato impedes conidial germination, attachment and hyphal growth of Botrytis cinerea. BMC Plant Biol. 17:235. doi: 10.1186/s12870-017-1184-2
Stasolla, C. (2010). Glutathione redox regulation of in vitro embryogenesis. Plant Physiol. Bioch. 48, 319–327. doi: 10.1016/j.plaphy.2009.10.007
Talibi, I., Askarne, L., Boubaker, H., Boudyach, E. H., Msanda, F., Saadi, B., et al. (2012). Antifungal activity of some Moroccan plants against Geotrichum candidum, the causal agent of postharvest citrus sour rot. Crop Prot. 35, 41–46. doi: 10.1016/j.cropro.2011.12.016
Tao, N., OuYang, Q., and Jia, L. (2014). Citral inhibits mycelial growth of Penicillium italicum by a membrane damage mechanism. Food Control 41, 116–121.
Tian, J., Ban, X., Hong, Z., Jingsheng, H., Yuxin, C., and Youwei, W. (2012). The mechanism of antifungal action of essential oil from Dill (Anethum graveolens L.) on Aspergillus avus. PLoS One 7:30147. doi: 10.1371/journal.pone.0030147
Tian, J., Wang, Y., Zeng, H., Li, Z., Zhang, P., Tessema, A., et al. (2015). Efficacy and possible mechanisms of perillaldehyde in control of Aspergillus niger causing grape decay. Int. J. Food Microbiol. 202, 27–34.
Wang, Y., Sun, Y., Zhang, Y., Zhang, X., and Feng, J. (2016). Antifungal activity and biochemical response of cuminic acid against Phytophthora capsici Leonian. Molecules 21, 756–769. doi: 10.3390/molecules21060756
Wang, Y., Feng, K., Yang, H., Zhang, Z., Yuan, Y., and Yue, T. (2018). Effect of cinnamaldehyde and citral combination on transcriptional profile, growth, oxidative damage and patulin biosynthesis of Pencillium expansum. Front. Microbiol. 9:597. doi: 10.3389/fmicb.2018.00597
Xu, J., Shao, X., Li, Y., Wei, Y., Feng, X., and Wang, H. (2017). Metabolomic analysis and mode of action of metabolites of tea tree oil involved in the suppression of Botrytis cinerea. Front. Microbiol. 8:1017. doi: 10.3389/fmicb.2017.01017
Yahyazadeh, M., Omidbaigi, R., Zare, R., and Taheri, H. (2008). Effect of some essential oils on mycelial growth of Penicillium digitatum Sacc. World J. Microb. Biot. 24, 1445–1450. doi: 10.1007/s11274-007-9636-8
Yang, X., Zhang, L., Xiang, Y., Du, L., Huang, X., and Liu, Y. (2020). Comparative transcriptome analysis of Sclerotinia sclerotiorum revealed its response mechanisms to the biological control agent Bacillus amyloliquefaciens. Sci. Rep. 10, 1–12. doi: 10.1038/s41598-020-69434-9
Young, M., Wakefield, M., Smyth, G., and Oshlack, A. (2010). Gene ontology analysis for RNA-Seq: accounting for selection bias. Genome Biol. 11, 1–12. doi: 10.1186/gb-2010-11-2-r14
Zhang, J., Tian, H., Sun, H., and Wang, X. (2017). Antifungal activity of trans-2-hexenal against Penicillium cyclopium by a membrane damage mechanism. J. Food Biochem. 41:e12289. doi: 10.1111/jfbc.12289
Zhang, J., Zeng, L., Chen, S. Y., Sun, H. L., and Ma, S. (2018). Transcription profile analysis of Lycopersicum esculentum leaves, unravels volatile emissions and gene expression under salinity stress. Plant Physiol. Bioch. 126, 176–189. doi: 10.1016/j.plaphy.2018.02.016
Zheng, S., Jing, G., Wang, X., Ouyang, Q., Jia, L., and Tao, N. (2015). Citral exerts its antifungal activity against Penicillium digitatum by affecting the mitochondrial morphology and function. Food Chem. 158, 76–81. doi: 10.1016/j.foodchem.2015.01.077
Keywords: Botrytis cinerea, (E)-2-hexenal, RNA sequencing, differentially expressed genes, ergosterol content
Citation: Song G, Du S, Sun H, Liang Q, Wang H, Yan M and Zhang J (2022) Antifungal mechanism of (E)-2-hexenal against Botrytis cinerea growth revealed by transcriptome analysis. Front. Microbiol. 13:951751. doi: 10.3389/fmicb.2022.951751
Received: 25 May 2022; Accepted: 18 July 2022;
Published: 22 August 2022.
Edited by:
Boqiang Li, Institute of Botany (CAS), ChinaCopyright © 2022 Song, Du, Sun, Liang, Wang, Yan and Zhang. This is an open-access article distributed under the terms of the Creative Commons Attribution License (CC BY). The use, distribution or reproduction in other forums is permitted, provided the original author(s) and the copyright owner(s) are credited and that the original publication in this journal is cited, in accordance with accepted academic practice. No use, distribution or reproduction is permitted which does not comply with these terms.
*Correspondence: Jihong Zhang, amlob25nemgwMUBobnVzdC5lZHUuY24=