- College of Plant Sciences, Jilin University, Changchun, China
The necrotrophic fungus Sclerotinia sclerotiorum is a devastating pathogen. S. sclerotiorum can cause Sclerotinia stem rot in more than 600 species of plants, which results in serious economic losses every year. Chitin is one of the most important polysaccharides in fungal cell walls. Chitin and β-Glucan form a scaffold that wraps around the cell and determines the vegetative growth and pathogenicity of pathogens. UDP-GlcNAc is a direct precursor of chitin synthesis. During the synthesis of UDP-GlcNAc, the conversion of GlcNAc-6P to GlcNAc-1P that is catalyzed by AGM1 (N-acetylglucosamine-phosphate mutase) is a key step. However, the significance and role of AGM1 in phytopathogenic fungus are unclear. We identified a cytoplasm-localized SsAGM1 in S. sclerotiorum, which is homologous to AGM1 of Saccharomyces cerevisiae. We utilized RNA interference (RNAi) and overexpression to characterize the function of SsAGM1 in S. sclerotiorum. After reducing the expression of SsAGM1, the contents of chitin and UDP-GlcNAc decreased significantly. Concomitantly, the gene-silenced transformants of SsAGM1 slowed vegetative growth and, importantly, lost the ability to produce sclerotia and infection cushion; it also lost virulence, even on wounded leaves. In addition, SsAGM1 was also involved in the response to osmotic stress and inhibitors of cell wall synthesis. Our results revealed the function of SsAGM1 in the growth, development, stress response, and pathogenicity in S. sclerotiorum.
Introduction
The ascomycete Sclerotinia sclerotiorum (Lib.) de Bary is an important necrotrophic, soilborne, phytopathogenic fungus, which is distributed throughout the world (Boland and Hall, 1994). S. sclerotiorum is remarkable for its extremely broad host range, and diseases caused by this pathogen occur in more than 600 plant species, which include almost all dicotyledons and some monocotyledons (Liang and Rollins, 2018; Xia et al., 2019). It can infect many crops of economic importance, such as oil crops including soybeans, Brassica napus, and sunflowers (Boland and Hall, 1994); vegetable crops including lettuce and tomatoes, and ornamental crops including tulips and chrysanthemums (Bolton et al., 2006).
Importantly, the damage caused by S. sclerotiorum is enormous globally every year (Xu T. et al., 2018). The vegetative hyphae of S. sclerotiorum interweave and gather together to formed hard, black sclerotia (Erental et al., 2008). Sclerotia is the resting and propagation structure of S. sclerotiorum, which can survive in the soil for many years where it resists adverse environmental conditions (Zhang et al., 2021; Yu and Rollins, 2022). In addition, S. sclerotiorum has a massive and complex pathogenetic arsenal, which includes an array of cell wall–degrading enzymes, phytotoxins, and other secondary metabolites that kill host cells and facilitate infection (Kabbage et al., 2013; Seifbarghi et al., 2017; Xu L. et al., 2018; Allan et al., 2019). S. sclerotiorum can also secrete oxalic acid to inhibit host immune responses in the early stage of infection, and it induces cell necrosis in the later stage of infection (Kim et al., 2008; Williams et al., 2011). Recent investigations showed that there is a very complex mechanism of interaction between S. sclerotiorum and its hosts (Mbengue et al., 2016; Liang and Rollins, 2018). It is extremely difficult to control the disease caused by S. sclerotiorum due to its extensive host range, complex pathogenesis, and the strong survival ability of sclerotia. At present, chemical control is used mainly to control Sclerotinia diseases because there is a lack of resistant cultivars (Seifbarghi et al., 2017; Xia et al., 2019). Therefore, identifying and characterizing the growth, development, and pathogenesis are helpful to explore potential drug targets and to provide a new strategy for controlling diseases caused by S. sclerotiorum.
The fungal cell wall is a rigid but dynamic structure, which is essential for the growth, development, and pathogenicity of plant pathogenic fungi (Gow et al., 2017; Patel and Free, 2019; Plaza et al., 2020). Furthermore, a series of elegant studies has shown recently that cell walls have extraordinary effects on many aspects of fungal physiology, which include cell survival, morphogenesis, virulence, perception of environmental changes, and host-pathogen interactions (Geoghegan et al., 2017; Latge et al., 2017; Alcazar-Fuoli et al., 2020). In Saccharomyces cerevisiae, one-fifth of the yeast genome, or about 1,200 genes, is devoted to the biosynthesis of the cell wall, which includes the assembly of basic components, the supply of substrates, signal transduction, and regulation (de Groot et al., 2001; Klis et al., 2006). This fact also demonstrates the importance of fungal cell walls. The cell wall of fungi is composed mainly of chitin, mannan, and glucan, and polysaccharides account for about 90% of the cell wall (Klis et al., 2006; Oliveira-Garcia and Deising, 2013). Chitin accounts for 10–20% of the total cell wall contents of filamentous fungi (Klis, 1994; Arroyo et al., 2016). Chitin plays an essential role in maintaining cell wall integrity and pathogenicity of phytopathogenic fungi (Arroyo et al., 2016; Patel and Free, 2019). Therefore, many enzymes and regulatory factors in the process of chitin biosynthesis can be used as effective targets of fungicides.
The biosynthesis of chitin in fungi initiates from glucose, mannose, and fructose, and which are transformed into Fructose 6-phosphate (Fru-6P) through a series of biochemical reactions (Hofmann et al., 1994). Fru-6P is converted to glucosamine 6-phosphate by aminoamide: fructose-6-phosphate aminotransferase (GFA1). Glucosamine 6-phosphate is further N-acetylated by GlcN-6P acetyltransferase (GNA1) to form GlcNAc-6P (Fang et al., 2013). GlcNAc-6P is converted to GlcNAc-1P by N- acetylglucosamine-phosphate mutase (AGM1), which catalyzes intramolecular phosphoryl transfer on arrange of phosphosugar substrates (Milewski et al., 2006; Fang et al., 2013). GlcNAc-1P is transformed into UDP-GlcNAc (uridine diphosphate-N-acetylglucosamine) under the action of UDP-GlcNAc pyrophosphorylase (UAP1) (Milewski et al., 2006). UDP-GlcNAc is the direct precursor of chitin (Free, 2013). Finally, chitin is synthesized by plasma membrane-associated chitin synthases (Free, 2013; Gow et al., 2017). In this pathway, the conversion of GlcNAc-6P to GlcNAc-1P that is catalyzed by AGM1 is a vital step in the synthesis of UDP-GlcNAc.
AGM1 has been isolated and identified from S. cerevisiae, Aspergillus fumigatus, Candida albicans, and Homo sapiens (Hofmann et al., 1994; Mio et al., 2000; Fang et al., 2013). In yeast, AGM1 is an essential gene for cell survival. The deletion of AGM1 gene resulted in abnormal division and a decrease in survival rate (Hofmann et al., 1994). Mice that lacked the AGM1 homologous gene (pgm3) died before implantation, and heterozygotes had congenital hematopoietic and reproductive defects (Greig et al., 2007). In the human pathogenic fungus A. fumigatus, reducing the expression of AfAGM1 resulted in defects in hyphae growth, and melanin synthesis (Fang et al., 2013). However, the function of AGM1 in phytopathogenic fungi is poorly understood.
In this study, we identified SsAGM1 (i.e., the homolog of AGM1 in S. cerevisiae) in S. sclerotiorum. We employed RNA interference (RNAi) and overexpression to characterize the function of SsAGM1 in S. sclerotiorum. We showed that UDP-GlcNAc synthesis that was mediated by SsAGM1 affected many aspects of S. sclerotiorum, which included the content of chitin in the cell wall, cell wall integrity, mycelial growth, formation of infection cushions, sclerotia formation, stress response, and pathogenicity. We demonstrated that SsAGM1 was essential for the growth, development, and pathogenicity of S. sclerotiorum.
Materials and Methods
Fungal Strains and Culture Conditions
In this study, S. sclerotiorum strain UF-1, which was isolated from infected petunias in Florida, United States, was used as a wild-type strain (WT) (Li et al., 2018). WT and all transgenic strains in this study were initially grown on potato glucose agar (PDA) plate (200 g potato, 20 g glucose, 15 g agar, constant volume to 1 L) and cultured at 25°C in the dark. Arabidopsis thaliana (Arabidopsis ecotype Col-0), Glycine max (soybean Williams 82), and Solanum lycopersicum (tomato Zhongshu 4) were grown in the glasshouse under natural sunlight with temperature in the 22–25°C range.
Identification and Sequence Analysis of SsAMG1
The gene and protein sequences of SsAMG1 were obtained from the NCBI database,1 and the conserved domain of SsAMG1 was predicted by BLAST and the conserved domain database. The homologous protein of SsAMG1 in other species was obtained from NCBI and related literature. Multiple sequence alignments were constructed with ClustalW with full-length protein sequences (Thompson et al., 1994). A phylogenetic tree was constructed using MEGA 5 with the neighbor-joining algorithm under default settings and 1,000 bootstrap replications (Tamura et al., 2007).
Plasmid Constructs and Transformation
The pSilent-Dual1 and pYF11 (Fan et al., 2017; Jiao et al., 2022) vectors, which were used for gene silencing and overexpression vectors in this study, respectively, contained a geneticin (G418) resistance marker. To silence SsAGM1 of S. sclerotiorum, four fragments of the coding region of SsAGM1 were amplified with specific primers that used S. sclerotiorum cDNA as a template. The PCR products were ligated into the XbaI restriction site of the pSilent-Dual1 vector to generate pSD-SsAGM1-T1, pSD-SsAGM1-T2, pSD-SsAGM1-T3, and pSD-SsAGM1-T4 constructs (Supplementary Figure 1). To generate S. sclerotiorum overexpression strains of SsAGM1, the full-length coding region was amplified with specific primers that used S. sclerotiorum cDNA as a template. The amplified product was connected to the pYF11 vector that was linearized with XhoI restriction enzyme by seamless cloning to produce the pYF11: SsAGM1:eGFP vector. The above vectors were verified by sequencing and then used for genetic transformation of S. sclerotiorum. The pSilent-Dual1 was used as an empty vector control. All primers used in this assay are listed with brief descriptions in Supplementary Table 1.
The preparation of S. sclerotiorum protoplasts was carried out as described previously (Qu et al., 2014). These constructs were transferred into S. sclerotiorum protoplasts by the PEG-mediated transformation protocol (Rollins, 2003). Transformants were screened three times with a PDA plate that contained G418 (100 μg/mL).
Reverse-Transcription, Quantitative Real-Time PCR Analysis
All strains were inoculated on a PDA that was covered with cellophane at 25°C in the dark. The hyphae were collected and frozen immediately in liquid nitrogen. Total RNA was extracted and purified by a TransZol Up Plus RNA Kit (TransGen Biotech). Total RNA was reverse transcribed using EasyScript All-in-One First-Strand cDNA Synthesis SuperMix (TransGen Biotech) to synthesize cDNA that was used for subsequent experiments. cDNA was diluted 10 times as a template. Reverse-transcription, quantitative real-time PCR (RT-qPCR) was performed with the TransStart Green qPCR SuperMix (TransGen Biotech). SsActin of S. sclerotiorum was used as an internal reference. The relative expression levels were calculated with three technical replications using the 2–ΔΔCt method. All primers used in this assay are listed with brief descriptions in Supplementary Table 1. All experiments were repeated three times independently.
Measurement of the Content of Chitin and Uridine Diphosphate-N-Acetylglucosamine in Hyphae
The chitin content was determined according to the method described previously with modifications (Bulik et al., 2003). First, the mycelial samples were dried and ground sufficiently. For each sample, 5 mg of dried mycelium was resuspended in 1 mL 6% KOH and heated at 80°C for 90 min. After cooling to room temperature, the sample was centrifuged (1,600 × g, 10 min) and resuspended with phosphate buffered saline, and this was repeated three times. Finally, the sample was resuspended in 500 μL McIlvaine’s buffer (pH 6.0), mixed gently with 100 μL Streptomyces duplicatus chitinase (Sigma), and incubated at 37°C for 18 h. We extracted 100 μL from each sample, and these were mixed fully with 100 μL 0.27 M NaB4O7 (pH 9.0) were fully mixed in 2 mL centrifuge tubes and heated at 98°C for 10 min. Then, 1 mL Ehrlich’s reagent (10 g β-dimethylaminobenzaldehyde in 1.25 mL of concentrated HCl and 98.75 mL glacial acetic acid) was added to each sample and incubated at 37°C for 20 min. One milliliter of the sample was transferred to a cuvette and the absorbance at 585 nm was recorded. A standard curve was prepared with GlcNAc (BBI, China). All experiments were repeated three times independently.
To determine the content of UDP-GlcNAc in WT, control strain (CK), and in gene-silenced and overexpression transformants, the mycelia of all strains were inoculated in liquid PD medium (200 g potato, 20 g glucose per liter) for 3 days at 25°C in the dark. The method for determining the UDP-GlcNAc content was performed as described in the manual (Qingdao Kechuang, China).
Mycelia Growth, Sclerotia, and Formation of Infection Cushions
To observe mycelia growth and sclerotia formation, the WT, CK, gene-silenced transformants, and overexpression transformants were inoculated on PDA plates for 2–15 days at 25°C in the dark; colony diameters were measured every 12 h. Infection cushions of strains were observed on glass slides. The formation of infection cushions was observed under a microscope at 12 h after inoculation. All experiments were repeated three times independently.
Determinations of Cell Wall Sensitivity and Tolerance of Osmotic Stress
We determined the inhibition rate of hyphal growth when cultured with inhibitors of cell wall synthesis and external osmotic substances. Agar plugs with actively growing hyphae that were obtained from WT, CK, gene-silenced transformants, and overexpression transformants were inoculated on PDA plates that contained Congo red (CR, 500 μg/mL), CFW (50 μg/mL, SIGMA, 910090), 0.005% SDS, 1 M sorbitol, 0.7 M KCl, and 0.7 M NaCl. The colony diameters were measured, and the inhibition rate of hyphae growth was calculated at 48 h after inoculation. Inhibition rate = (diameter of untreated strain—diameter of treated strain)/(diameter of untreated strain × 100%). All experiments were conducted three times independently.
Subcellular Localization of SsAGM1
To observe the subcellular localization of SsAGM1, overexpression transformants OE-SsAGM1-2 and OE-SsAGM1-11 were inoculated on PDA medium and covered with cellophane for 12 h at 25°C in the dark. The hyphae were viewed under a fluorescence microscope with a 480-nm light.
Pathogenicity Assays
The pathogenicity of S. sclerotiorum was determined by infecting leaves of soybean, Arabidopsis, and tomato. First, the WT, CK, gene-silenced transformants, and overexpression transformants were cultured on PDA plates. Agar plugs (0.5 cm diameter) were obtained from the edge of PDA-cultured colonies and inoculated on intact soybean, Arabidopsis, intact tomato leaves, and wounded tomato leaves at room temperature. The inoculated leaves were kept in a sealed container with high humidity for 4 days. The lesion area was measured and photographed at 36 and 48 h after inoculation, and the lesion area was calculated using ImageJ. Pathogenicity tests were repeated three times independently.
Statistical Analysis
Data were tested for homogeneity of variances first. If variances were equal, statistical analysis was performed with one-way ANOVA using SPSS. Pairwise comparisons were performed with Tukey’s HSD (Honestly Significant Difference) using SPSS. In this study, all data were expressed as the mean ± SD, and the p-value < 0.05 was considered statistically significant. Statistical charts were constructed using GraphPad Prism 8.0.2.
Results
Identification of SsAGM1 in Sclerotinia sclerotiorum
The SS1G_01582 of S. sclerotiorum is the ortholog of S. cerevisiae AGM1(YEL058W). SS1G_01582 has a 1,667-bp open reading frame, which encodes a predicted protein with a length of 538 amino acids. The ORF of the SS1G-01582 gene was interrupted by a 50-bp intron. The protein encoded by the SS1G_01582 was annotated in GenBank, which contained the conserved domain of AGM1 (N-acetylglucosamine-phosphate mutase). Protein BLAST searches performed with AGM1 proteins of S. cerevisiae and A. fumigatus suggested that AGM1 of S. sclerotiorum shared 66.61 and 45.86% sequence identity with S. cerevisiae AGM1 and A. fumigatus AfAGM1, respectively. Therefore, we designated this gene as SsAGM1. The phylogenetic tree analysis demonstrated that SsAGM1 sequences from S. sclerotiorum and Botrytis cinerea Bcpcm1 (Accession number: XP_024551673.1) formed a single clade, with a bootstrap of 100% (Figure 1A). The sequence motif Ser/Thr-X-Ser-His-Asn-Pro is highly conserved, and serine phosphorylation at the third position is necessary to give full play to its activity (Raimi et al., 2018). Through the alignment of the amino acid sequence of the conserved domain, we found that the conserved sequence motif Ser/Thr-X-Ser-His-Asn-Pro was contained in SsAGM1 (Figure 1B).
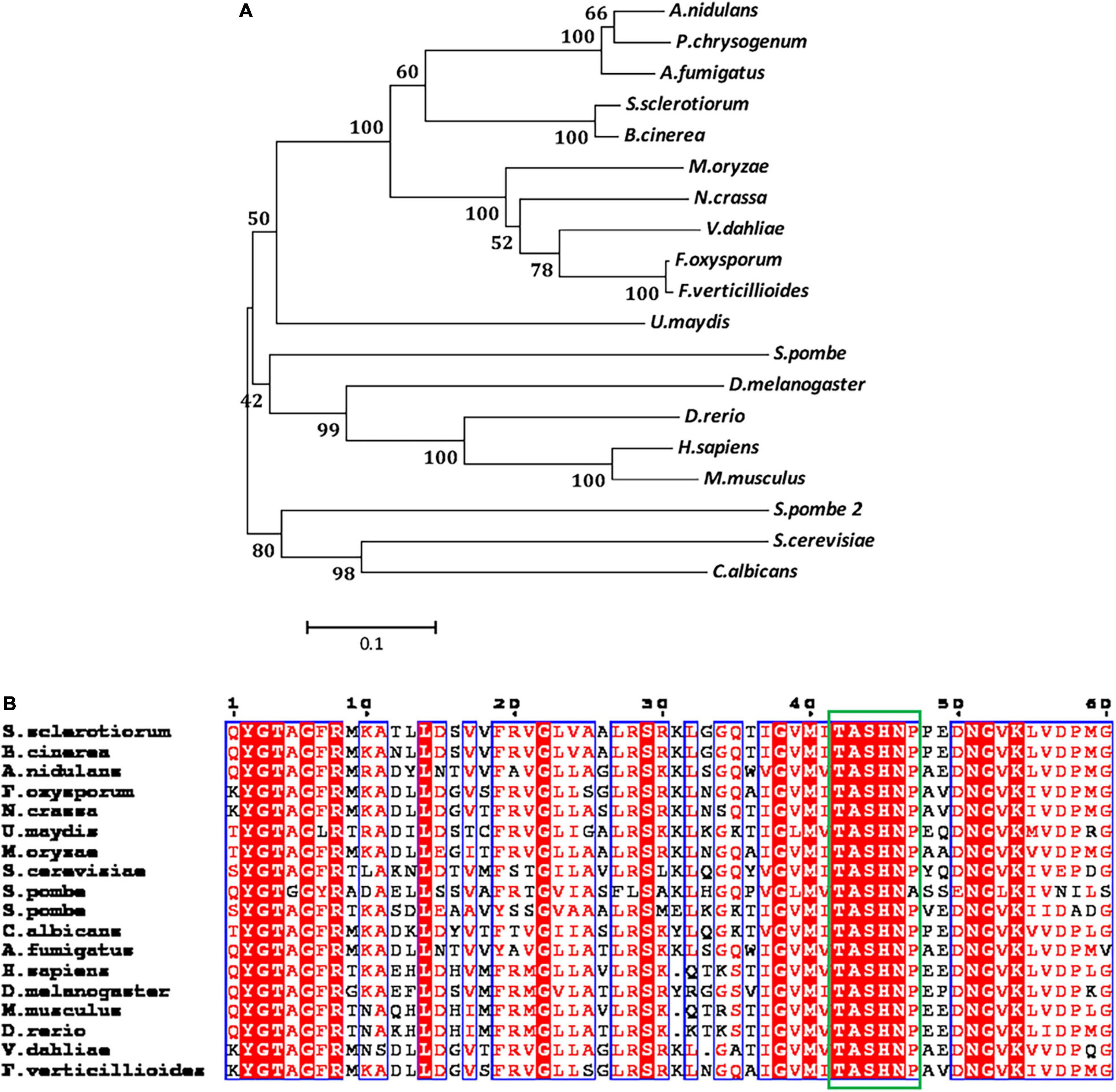
Figure 1. SsAGM1 encodes an N-acetylglucosamine-phosphate mutase in Sclerotinia sclerotiorum. (A) Phylogenetic analysis of SsAGM1 and its homologs. The amino acid sequences of SsAGM1 and its homologs were aligned using ClustalW, and the phylogenetic tree was constructed using MEGA 5 with the neighbor-joining algorithm. GenBank accession numbers of S. sclerotiorum and other species are in Supplementary Table 2. (B) Alignments of the conserved domains in SsAGM1 and its homologs. Alignments of the conserved domains by ClustalW and shown by ESPript 3.0. The conserved sequence motif Ser/Thr-X-Ser-His-Asn-Pro is shown in the green box. Residues with a score above defined threshold are written in red and boxed in blue.
To elucidate the function of SsAGM1 in S. sclerotiorum, we replaced SsAGM1 with hyg by homologous recombination to obtain a knockout mutant. PCR screens performed with many transformants always led to amplification of an 800 bp fragment of the SsAGM1, which indicated that SsAGM1 was not replaced completely. In S. cerevisiae and A. fumigatus, the AGM1 gene was lethal (Hofmann et al., 1994; Fang et al., 2013). We speculated that SsAGM1 was also a lethal gene in S. sclerotiorum. Therefore, we employed RNA interference and obtained the gene-silenced transformants of SsAGM1. The transformants obtained from the pSilent-Dual1 empty vector was used as the control strain (CK) in this study. The expression of SsAGM1 in gene-silenced transformants SsAGM1-T3-4, SsAGM1-T3-5, SsAGM1-T4-4, and SsAGM1-T4-10 decreased by about 60–70% compared with WT and CK (SsAGM1-T3-4: decreased by 60%, SsAGM1-T3-5: decreased by 55%, SsAGM1-T4-4: decreased by 72%, SsAGM1-T4-10 decreased by 70%) (Figure 2). There was no obvious difference in the expression of SsAGM1 between WT and CK.
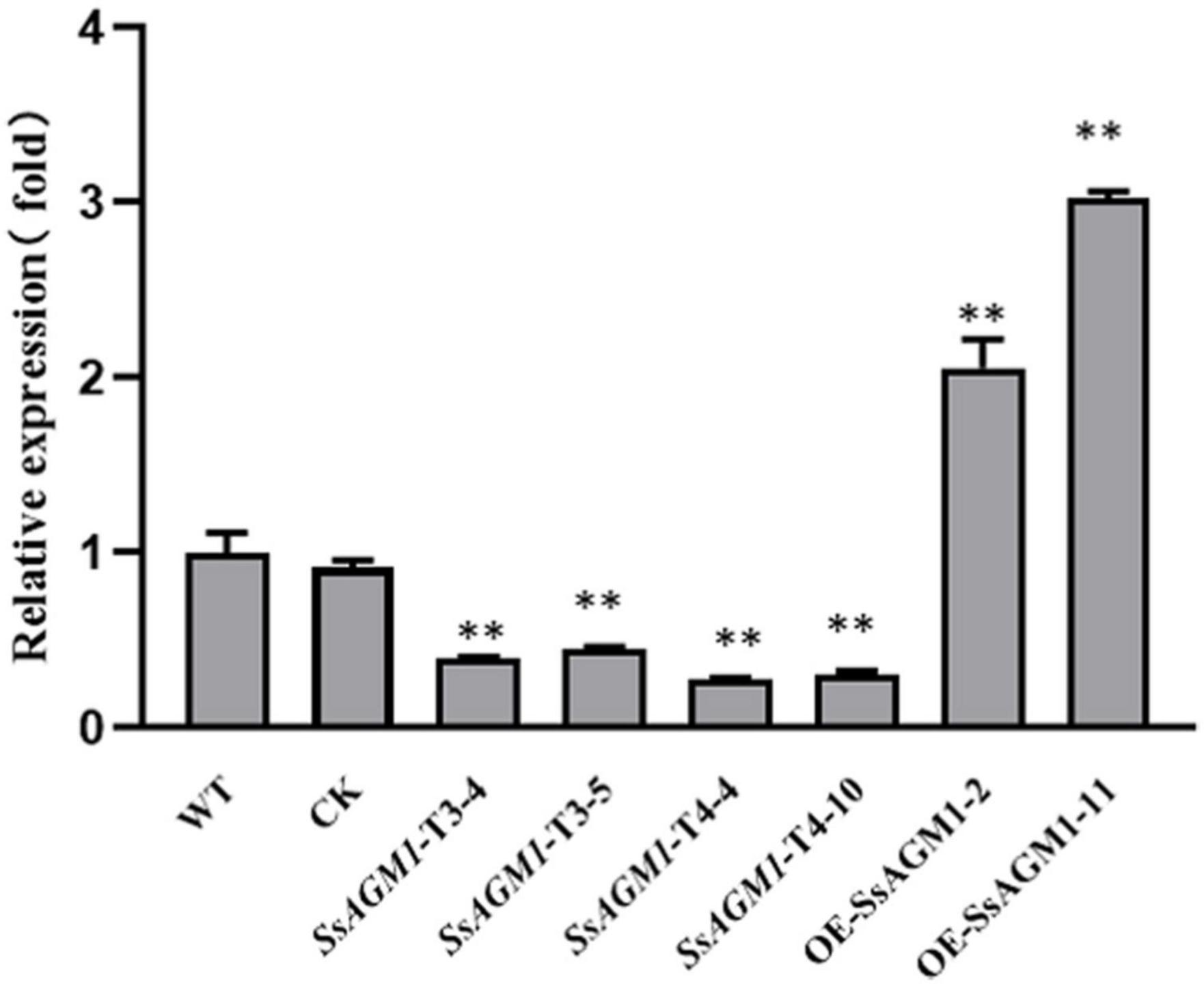
Figure 2. The relative expression level of SsAGM1 in wild-type strain, control strain, gene-silenced transformants, and overexpression transformants was measured by RT-qPCR. The SsActin in each sample was used as an internal control. The relative expression of SsAGM1 in WT was set as “1.” Data represent means ± SD. One-way ANOVA, n = 3; ** represents significant difference from WT at p < 0.05. WT, Wild-type strain; CK, the control strain.
In addition, we constructed the gene-overexpression vector pYF11:SsAGM1:eGFP, and the overexpression transformants of SsAGM1 were obtained. The expression of SsAGM1 in OE- SsAGM1-2 and OE-SsAGM1-11 was up-regulated by 2–3 times compared with WT (Figure 2). Furthermore, fluorescence microscopy showed that SsAGM1- GFP fusion proteins were distributed in the cytoplasm of S. sclerotiorum (Supplementary Figure 2). These data indicated that we identified a conserved SsAGM1 that was essential for the survival of S. sclerotiorum, and it was located in the cytoplasm.
SsAGM1 Was Involved in Uridine Diphosphate-N-Acetylglucosamine and Chitin Synthesis in Sclerotinia sclerotiorum
Calcoflour white (CFW) is a common fluorochrome used to detect defective mutants in the cell wall (Ram and Klis, 2006). To clarify the function of SsAGM1 in chitin synthesis, we stained the hyphae of the WT, CK, gene-silenced transformants, and overexpression transformants with CFW. In WT, CK, and overexpressed transformants, fluorescence gathered mainly at the septa and tips of hyphae, which are the most active places of chitin synthesis. However, the fluorescence was not concentrated at the hyphal tips in gene-silenced transformants, but it was distributed randomly and exhibited an irregular dot aggregation in the hyphae of gene-silenced transformants (Figure 3A). CFW staining showed that chitin synthesis and distribution were abnormal. AGM1 is required for UDP-GlcNAc synthesis (Milewski et al., 2006). We analyzed the accumulation of UDP-GlcNAc in hyphae quantitatively. The content of UDP-GlcNAc decreased by about 15% in SsAGM1 gene-silenced transformants compared with WT and CK, whereas the content of UDP-GlcNAc increased by 10% in overexpressed transformants compared with WT (Figure 3C). In addition, we also analyzed the content of chitin in the cell wall of strains quantitatively. The content of chitin decreased by about 20–30% in SsAGM1 gene-silenced transformants compared with WT and CK, whereas the content of chitin increased by about 15% in overexpressed transformants compared with WT (Figure 3B). There was no obvious difference in the content of UDP-GlcNAc and chitin between WT and CK.
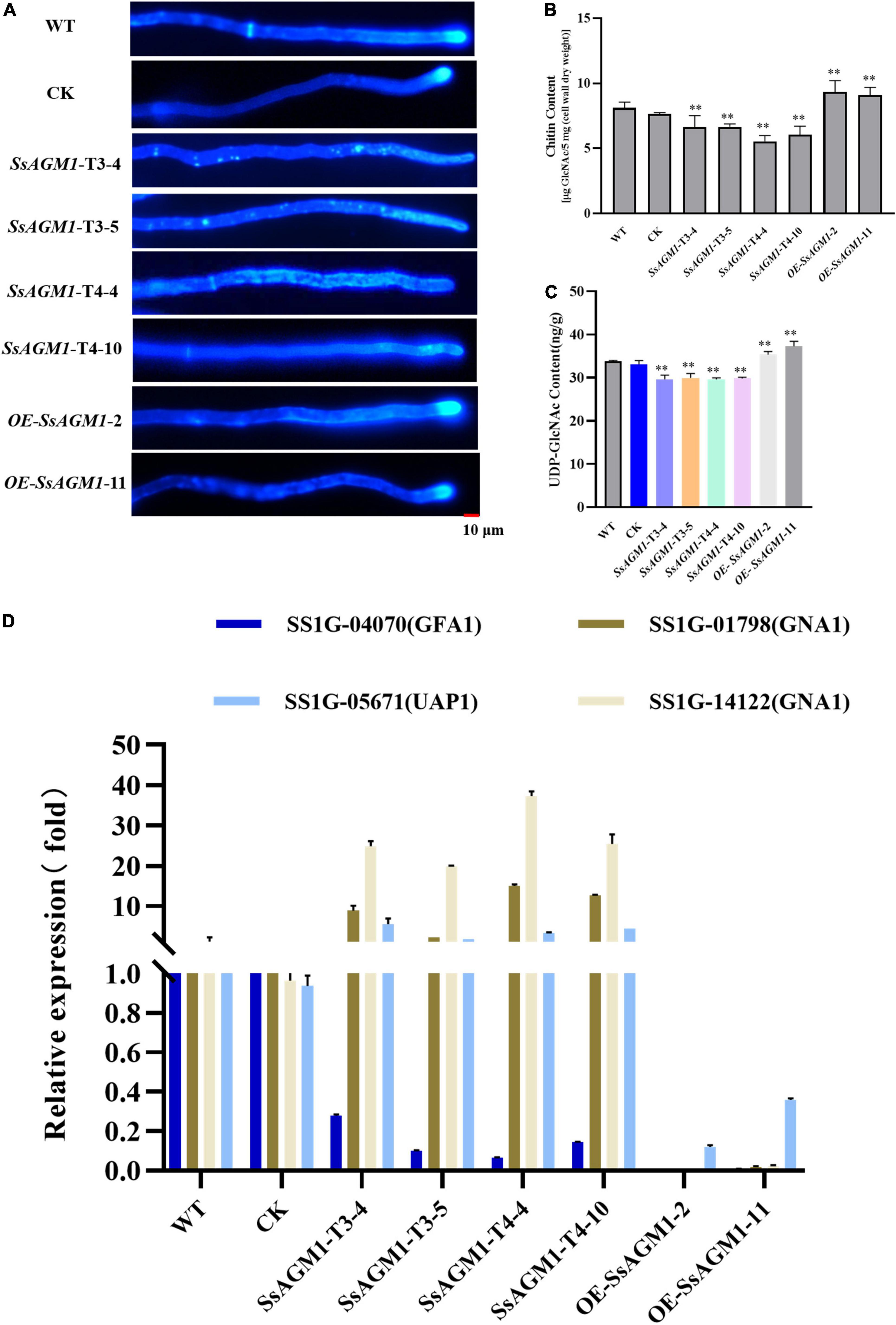
Figure 3. SsAGM1 is involved in UDP-GlcNAc and chitin synthesis in Sclerotinia sclerotiorum. (A) Wild-type strain, control strain, gene-silenced transformants, and overexpression transformants were treated with Calcofluor white (CFW) and observed using a fluorescence microscope. Strains were inoculated on PD for 12 h, and hyphae were stained by CFW (10 μg/mL) for 3 min in the dark. Scale bars, 10 μm. WT, Wild-type strain; CK, the control strain. (B) The determination of chitin content used the fluorimetric Morgan-Elson method. (C) Quantitation of UDP- GlcNAc content. Data represent means ± SD, n = 3. One-way ANOVA; **represents significant difference from WT at p < 0.05. (D) The analysis of the expression of key genes in the UDP-GlcNAc synthesis pathway by RT-qPCR. GFA1, glutamine: Fru-6P amidotransferase; GNA1, GlcN-6P acetyltransferase; UAP1, UDP-GlcNAc pyrophosphorylase. Error bars: the standard deviation of three replicates.
UDP-GlcNAc is synthesized from Fru-6P catalyzed successively by GFA1, GNA1, AGM1, and UAP1 (Milewski et al., 2006). We explored the effect on the expression of other genes in the UDP-GlcNAc synthesis pathway further after reducing the expression of SsAGM1. RT-qPCR was used to detect the expression of SS1G_04070 (GFA1), SS1G_01798 (GNA1), SS1G_14122 (GNA1), and SS1G_05671 (UAP1) of S. sclerotiorum. Compared with WT and CK, the expression of SS1G_05671, SS1G_01798, and SS1G_14122 increased significantly in SsAGM1 gene-silenced transformants, and the expression of SS1G_04070 decreased significantly. The expression of four genes in the overexpressed transformants decreased significantly compared with WT (Figure 3D). There was no significant difference in the expression of four genes between CK and WT. The results demonstrated that SsAGM1 was involved in UDP-GlcNAc and chitin synthesis in S. sclerotiorum.
SsAGM1 Contributed to Stress Tolerance in Sclerotinia sclerotiorum
Because chitin is an important component of cell walls (Patel and Free, 2019), we examined the role of SsAGM1 in the response to inhibitors of cell wall synthesis in S. sclerotiorum. CFW, congo red (CR), and sodium dodecyl sulfonate (SDS) are synthesis inhibitors of cell walls, and they are also common chemicals for detecting cell wall-defective mutants (Ram and Klis, 2006). Therefore, WT, CK, gene-silenced transformants, and overexpression transformants were inoculated on a PDA that contained CR, CFW, and SDS. We found that the tolerance of gene-silenced transformants to inhibitors of cell wall synthesis decreased significantly compared with WT and CK, although the tolerance to inhibitors of cell wall synthesis of overexpression transformants increased significantly compared with WT (Figures 4A,B). There was no significant difference in the tolerance to inhibitors of cell wall synthesis between CK and WT. The results suggested that SsAGM1 regulated the tolerance to inhibitors of cell wall synthesis positively, and it may be involved in maintaining cell wall integrity in S. sclerotiorum.
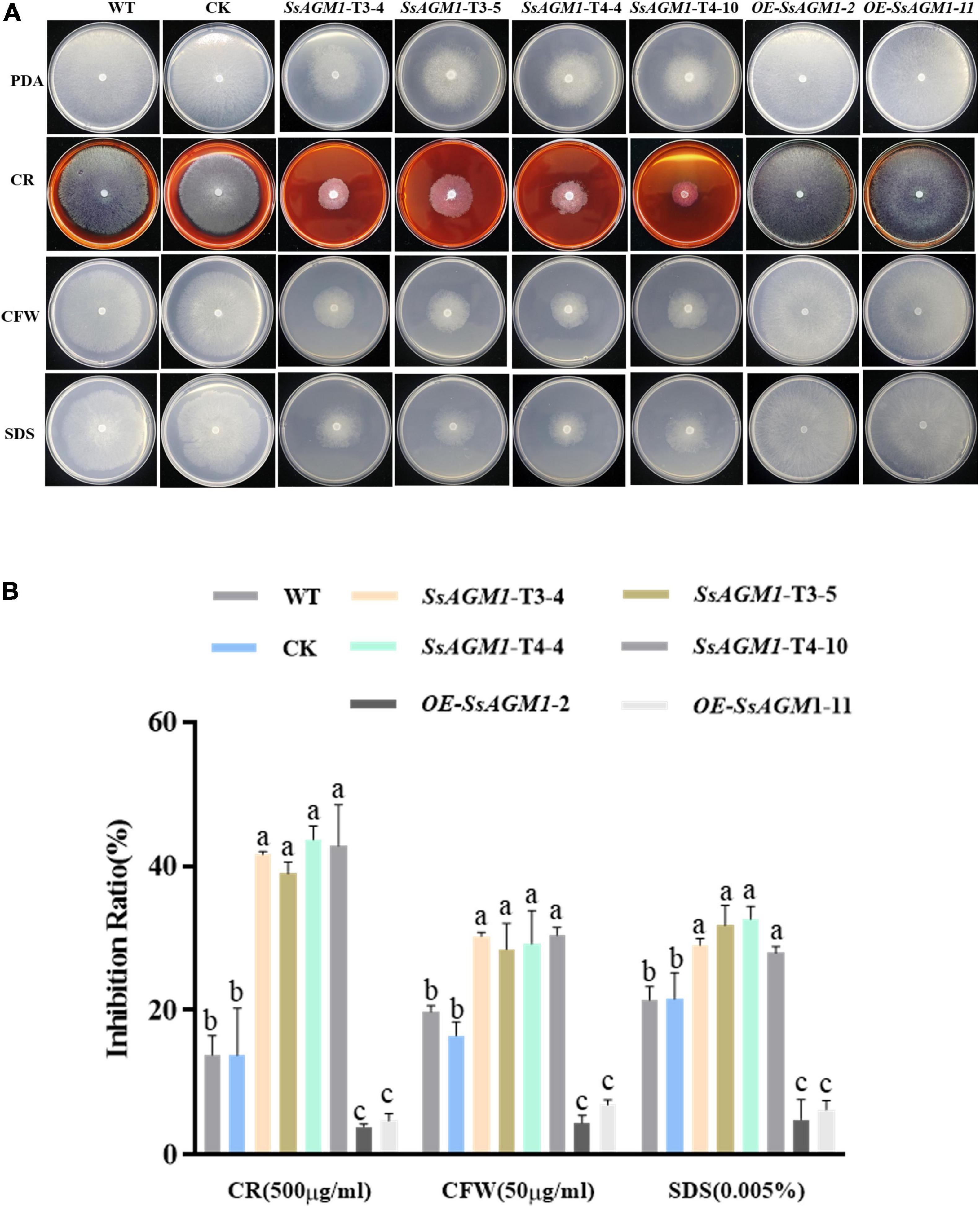
Figure 4. SsAGM1 contributes to the tolerance to inhibitors of cell wall synthesis in Sclerotinia sclerotiorum. (A) The growth phenotypes of wild-type strain, control strain, gene-silenced transformants, and overexpression transformants on PDA supplemented with CR, SDS, and CFW for 48 h after inoculation. WT, Wild-type strain; CK, the control strain; CFW, Calcofluor white; CR, Congo red; SDS, sodium dodecyl sulfate. (B) The inhibition rate of hyphae growth on PDA supplemented with different inhibitors of cell wall synthesis. Inhibition rate = (diameter of untreated strain - diameter of treated strain)/(diameter of untreated strain × 100%). Data represent means ± SD. One-way ANOVA; n = 3; different letters indicate statistically significant differences (p < 0.05).
Furthermore, we investigated whether SsAGM1 was involved in other stresses, such as osmotic stress. The WT, CK, gene-silenced transformants, and overexpression transformants were inoculated on a medium that contained NaCl, KCl, and D-sorbitol. The growth inhibition rate of gene-silenced transformants was significantly higher than in WT or CK (Figures 5A,B). However, the growth inhibition rate of overexpression transformants was significantly lower than in WT (Figures 5A,B). There was no significant difference in the growth inhibition rate between CK and WT. This indicated that SsAGM1 also positively regulated tolerance to osmotic stress of S. sclerotiorum. Thus, these results showed that SsAGM1 contributed to a variety of stress tolerance in S. sclerotiorum.
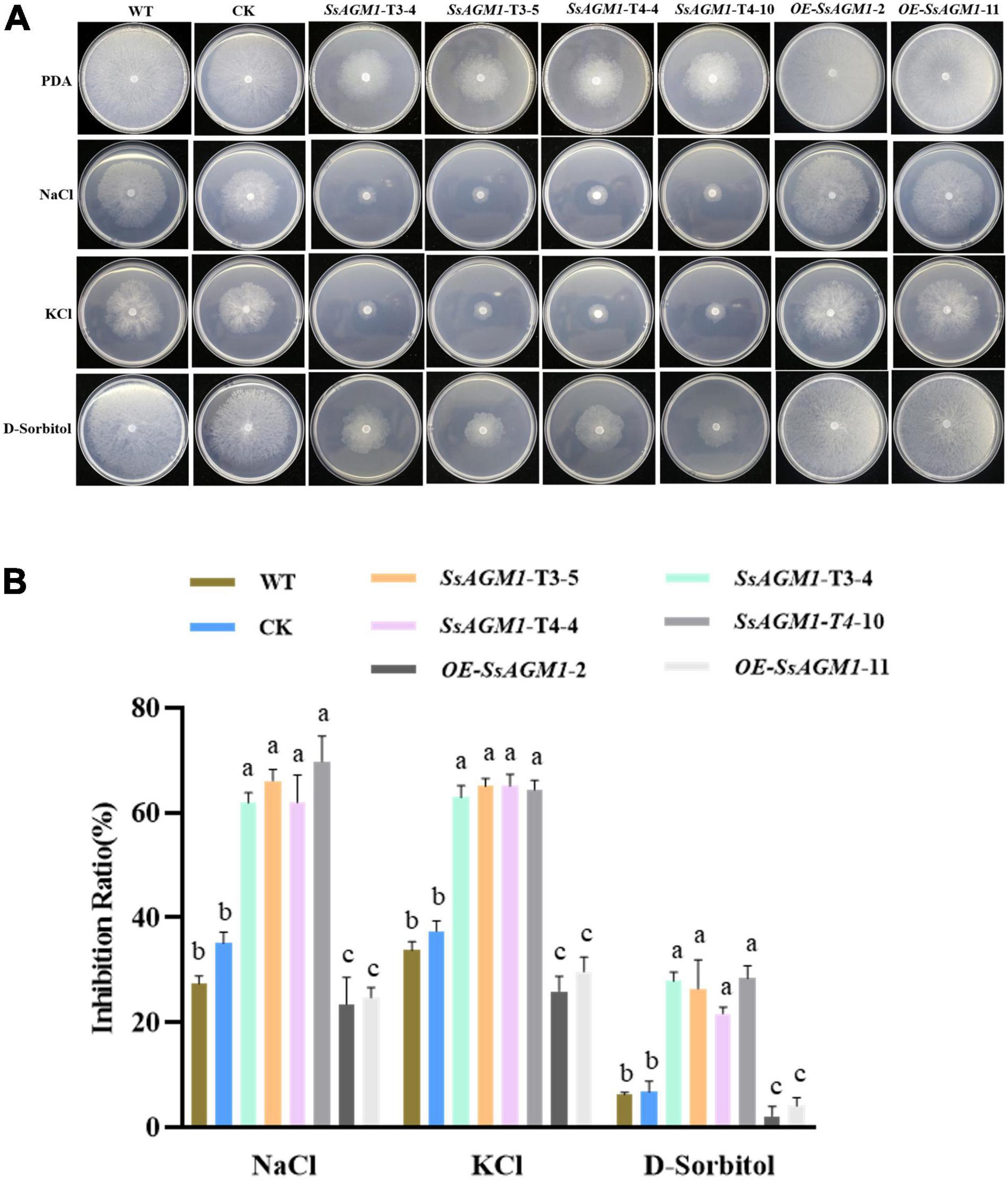
Figure 5. SsAGM1 contributes to the response to osmotic stress in Sclerotinia sclerotiorum. (A) The growth phenotypes of wild-type strain, control strain, gene-silenced transformants, and overexpression transformants on PDA supplemented with NaCl, KCl, and D-sorbitol for 48 h after inoculation. (B) The inhibition rate of hyphae growth on PDA supplemented with different external osmotic substances. Inhibition rate = (diameter of untreated strain - diameter of treated strain)/(diameter of untreated strain × 100%). One-way ANOVA; n = 3; different letters indicate statistically significant differences (p < 0.05). Data represent means ± SD. WT, Wild-type strain; CK, the control strain.
SsAGM1 Was Involved in Vegetative Growth and Sclerotia Formation in Sclerotinia sclerotiorum
Cell wall integrity was involved in the formation of sclerotia (Cong et al., 2022), and SsAGM1 was involved in the maintenance of cell wall integrity and chitin synthesis. This suggested that SsAGM1 may be involved in vegetative growth, especially the formation of sclerotia. To identify the function of SsAGM1 in the vegetative growth and formation of sclerotia in S. sclerotiorum, WT, CK, gene-silenced transformants, and overexpression transformants were inoculated on PDA plates and cultured at 25°C in the dark. The growth rate of hyphae in gene-silenced transformants was significantly lower than in WT, CK, or overexpression transformants (Figure 6A). WT, CK, and overexpression transformants formed black and mature sclerotia at 15 days after inoculation, but gene-silenced transformants never formed sclerotia even if the culture time was prolonged (Figure 6B). There was no obvious difference in vegetative growth and formation of sclerotia between WT, CK, and overexpression transformants. The results suggested that SsAGM1 played a critical role in the vegetative growth and formation of sclerotia in S. sclerotiorum.
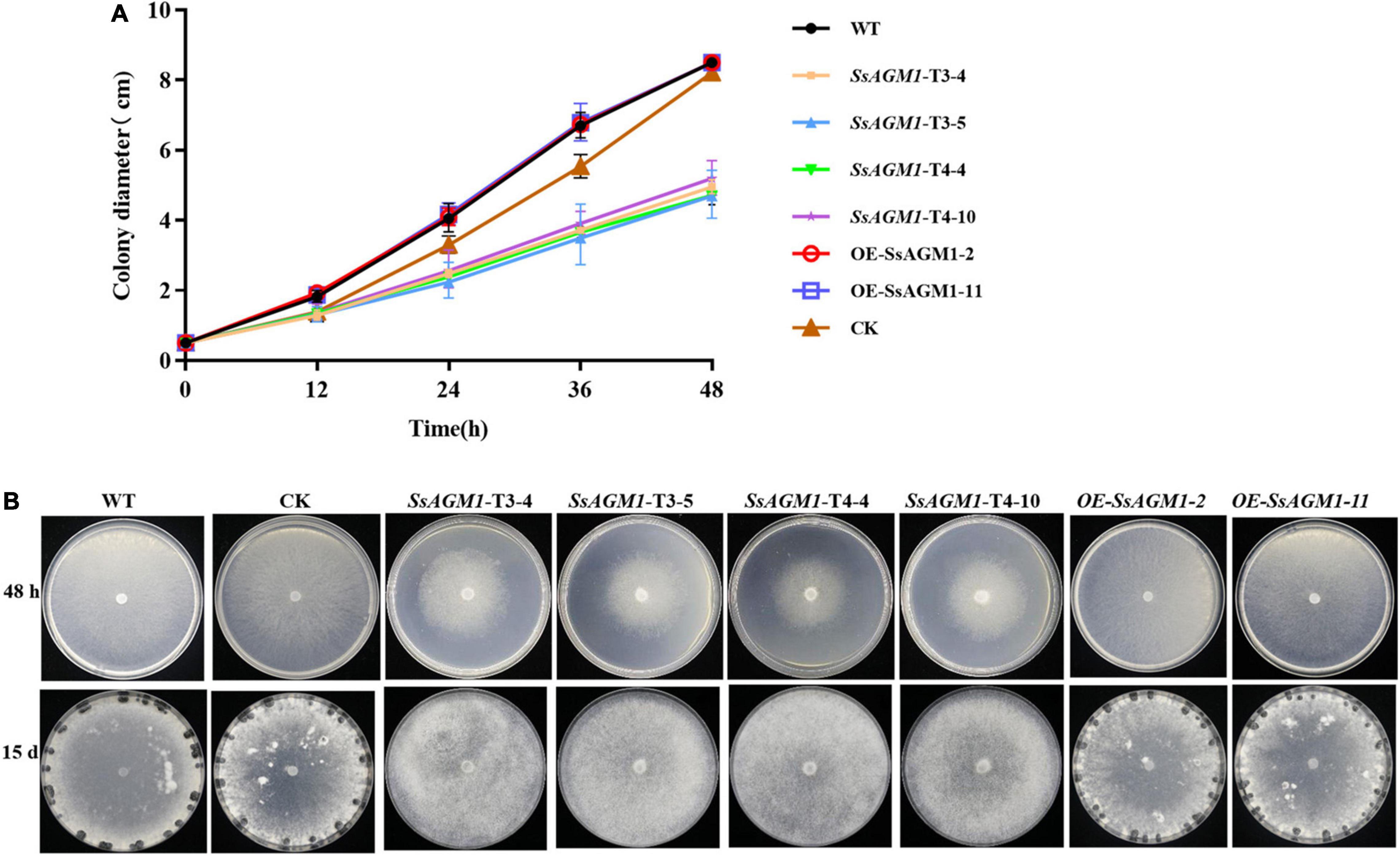
Figure 6. SsAGM1 is involved in vegetative growth and sclerotia formation in Sclerotinia sclerotiorum. (A) The hyphae growth rate in wild-type strain, control strain, gene-silenced transformants, and overexpression transformants. Error bars indicate the standard deviation of three replicates. (B) Sclerotial formation and colony morphology among wild-type strain, control strain, gene-silenced transformants, and overexpression transformants at 24 h or 15 days after inoculation. WT, Wild-type strain; CK, the control strain.
SsAGM1 Was Essential for Formation of Infection Cushions in Sclerotinia sclerotiorum
The fungal cell wall can sense various stimuli in the external environment, such as hydrophobicity, hardness, plant lipids, plant hormones, or secretory enzymes, and then it can trigger the formation of infection structures during the infection process, such as appressoria or infection cushions (Kou and Naqvi, 2016). Therefore, the disruption of the function and integrity of cell walls usually affects the formation of infected structures. For example, the deletion of MoTip41 resulted in disruption of CWI, and it resulted in abnormal appressoria in Magnaporthe oryzae (Qian et al., 2021). In Colletotrichum higginsianum, ChMK1 played an essential role in cell wall integrity, and the deletion of ChMK1 resulted in the absence of infection structures (Wei et al., 2016). Formation of infection cushions in WT, CK, gene-silenced transformants, and overexpression transformants was determined on the surface of a hydrophobic interface (glass slide) at 48 h after inoculation. WT and overexpression transformants formed many infection cushions, but the hyphae gathered together and did not form infection structures in gene-silenced transformants (Figures 7A,B). There was no obvious difference in formation of infection cushions between WT, CK, and overexpression transformants. These results suggested that SsAGM1 was indispensable for formation of infection cushions in S. sclerotiorum.
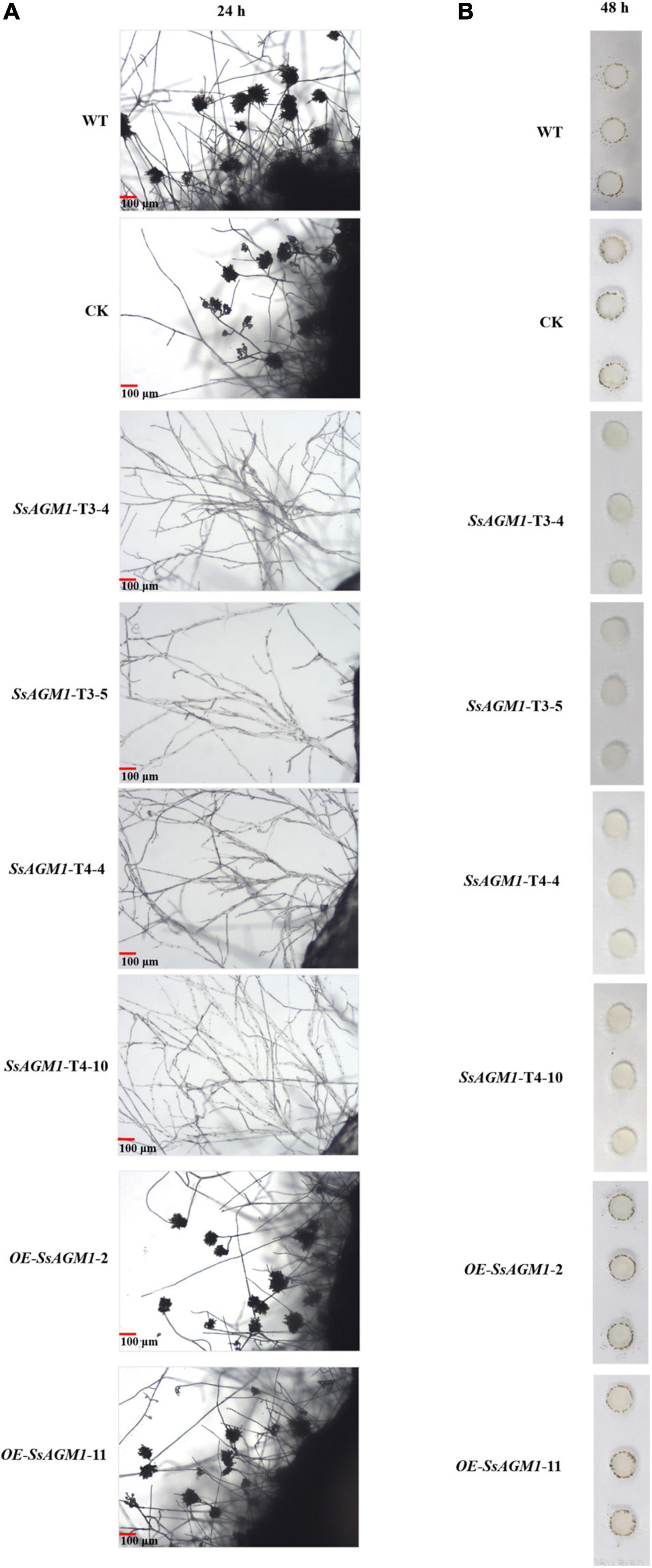
Figure 7. SsAGM1 is indispensable for the formation of infection cushions in Sclerotinia sclerotiorum. (A) Micro observation of mycelial morphology on a glass slide at 24 h after inoculation. (B) Infection cushions were observed on glass slides that surrounded mycelia-colonized agar plugs at 48 h after inoculation.
SsAGM1 Was Critical for Pathogenicity of Sclerotinia sclerotiorum
We investigated the role of SsAGM1 in S. sclerotiorum further for pathogenicity in WT, CK, gene-silenced transformants, and overexpression transformants that were inoculated on intact Arabidopsis, tomato, and soybean leaves, and virulence was evaluated 36 or 48 h after inoculation. The WT, CK, and overexpression transformants caused disease symptoms on Arabidopsis, tomato, and soybean leaves (Figures 8A–C). By contrast, the gene-silenced transformants were unable to invade intact leaves. Such disease symptoms did not emerge when the incubation time was extended to 4 days (Figures 8A,C), which excluded the possibility that the loss of pathogenicity was attributable to the reduced growth rates of the gene-silenced transformants. The infection experiment was also carried out using wounded tomato leaves, and similar results were obtained (Figure 8C). Inoculated areas at wound sites with gene-silenced transformants did not develop disease symptoms, but this may have caused minor necroses at the margins of the wounds at 4 days after inoculation (Figure 8C). This excluded the possibility of the loss of pathogenicity due to the inability to form infectious structures. In addition, quantitative analysis of the lesion area indicated that the pathogenicity of CK and gene-overexpression transformants were not significantly different from WT (Figure 8D). On the basis of these results, we concluded that SsAGM1 played an essential role in pathogenicity of S. sclerotiorum. However, we need to confirm further the specific mechanism of SsAGM1 that affected the pathogenicity in S. sclerotiorum.
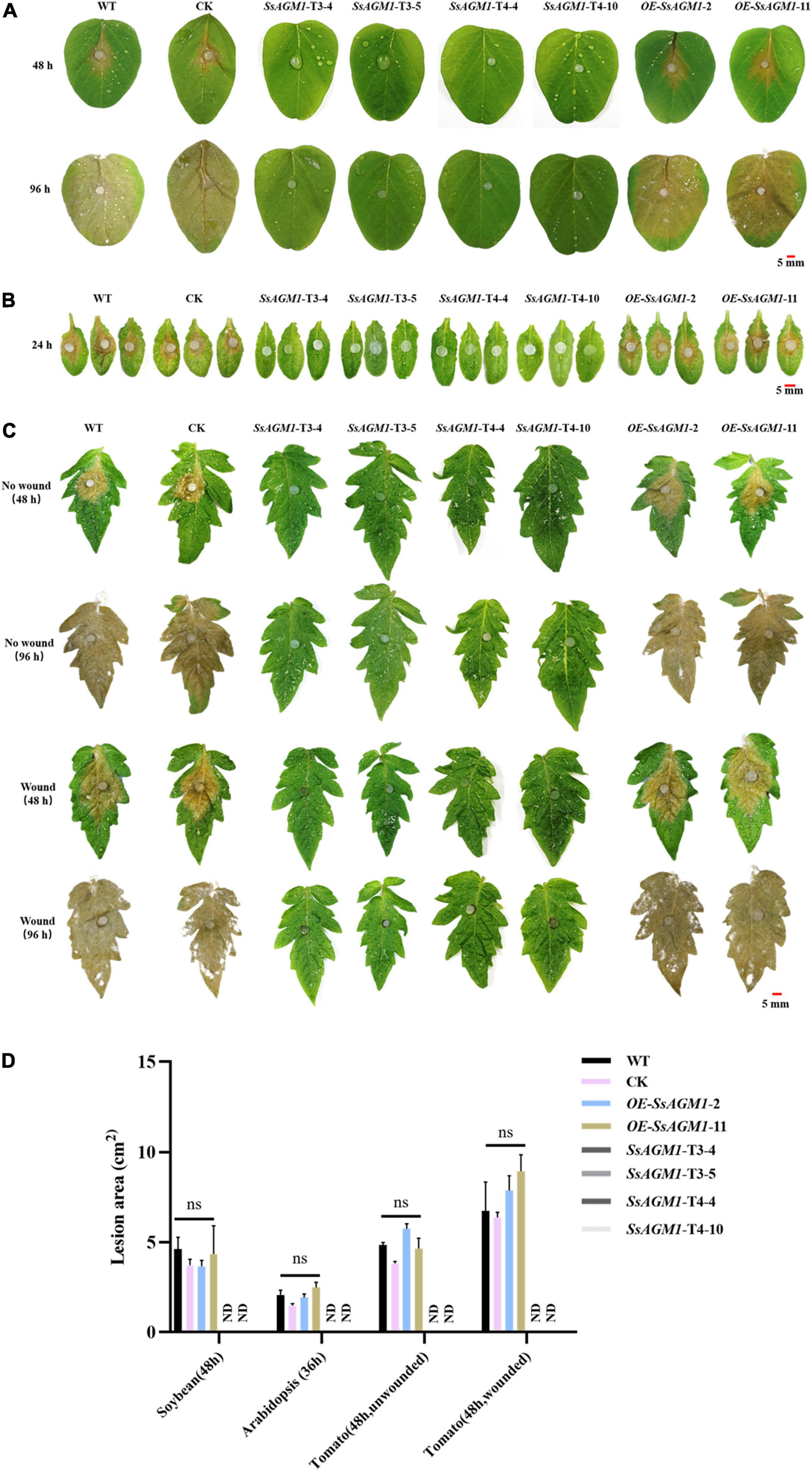
Figure 8. SsAGM1 is essential for pathogenicity in Sclerotinia sclerotiorum. (A,B) Disease symptoms of soybean and Arabidopsis leaves inoculated with wild-type strain, control strain, gene-silenced transformants, and overexpression transformants. (C) Wild-type strain, control strain, gene-silenced transformants, and overexpression transformants were inoculated on intact and wounded tomato leaves. (D) Quantitation of lesion sizes in (A–C). The lesion area was calculated by ImageJ. One-way ANOVA; n = 3; ns, represents no significant difference from WT at p < 0.05. ND, not detected. Data represent means ± SD. WT, Wild-type strain; CK, the control strain.
Discussion
Chitin is one of the most common polysaccharides in nature, which is second only to cellulose in abundance (Brown et al., 2020). As an important component of cell walls, chitin exists in almost all fungal cell walls and plays an important role in the vegetative growth, development, and pathogenicity of pathogenic fungi (Patel and Free, 2019). The biosynthetic pathway of chitin in fungi has been studied in the model organism S. cerevisiae (Hofmann et al., 1994; Klis et al., 2006; Milewski et al., 2006), and many enzymes and regulatory factors in this pathway have also been used as candidate targets for fungicides. Chitin is synthesized by UDP-GlcNAc as a precursor (Free, 2013). In the biosynthesis of UDP-GlcNAc, AGM1 catalyzes intramolecular phosphoryl transfer on the phosphosugar substrate GlcNAc-6P, which was a committed step in the synthesis of UDP-GlcNAc (Fang et al., 2013). To date, AGM1 has been functionally studied in only a few species and has not been systemically studied in phytopathogenic fungus. In this study, the SsAGM1 of S. sclerotiorum was identified, and the results indicated that SsAGM1 plays an important role in the growth, development, cell wall integrity, stress tolerance, and pathogenicity of S. sclerotiorum.
AGM1 was a crucial gene that affected cell survival and the cell cycle in S. cerevisiae. After AGM1 deletion, yeast cannot divide normally, with abnormal morphology and reduced survival rate (Hofmann et al., 1994). In A. fumigatus, it failed to construct the AfAGM1 deletion mutant by knocking out the gene. Finally, inhibiting the expression of AfAGM1 completely resulted in cell death by constructing the conditional inactivation mutant. The silencing of AfAGM1 seriously influenced the growth and development in A. fumigatus (Fang et al., 2013). In mice, embryos lacking the AGM1 ortholog (pgm3) cannot survive (Greig et al., 2007). These findings suggest that homozygous SsAGM1 knockout mutants were not screened in this study, possibly because SsAGM1 is also a lethal gene. We further employed RNAi and overexpression to study the function of SsAGM1. To avoid the potentially biased result caused by RNAi technique, four interference targets were designed, and vectors were constructed. Several gene-silenced transformants were obtained from pSD1-SsAGM1-target 3 and pSD1-SsAGM1-target 4 by resistance screening and RT-qPCR analysis (Figure 2). The transformants obtained from the pSilent-Dual1 empty vector was used as CK in this study. In addition, the gene-overexpression vector pYF11:SsAGM1:eGFP was also constructed and gene-overexpression transformants were obtained (Figure 2). Fluorescence microscopy showed that SsAGM1 was located in the cytoplasm of S. sclerotiorum (Supplementary Figure 2).
In A. fumigatus, the content of UDP-GlcNAc and chitin was significantly reduced in AfAGM1-silenced strains (Fang et al., 2013). The hyphae of WT, CK, gene-silenced transformants, and overexpression transformants were stained with CFW. Chitin did not aggregate at the growth point after SsAGM1 silencing (Figure 3A). The growth point was the most active place for chitin synthesis, and chitin did not gather at the tip of the hyphae, which indicated that chitin synthesis was abnormal. Subsequently, we found by quantitative analysis that the content of UDP-GlcNAc and chitin decreased significantly in SsAGM1 gene-silenced transformants compared with WT and CK, although the content of UDP-GlcNAc and chitin increased significantly in overexpressed transformants compared with WT (Figures 3B,C). The results showed that SsAGM1 was required for the synthesis of UDP-GlcNAc and chitin in S. sclerotiorum. There are many feedback regulation mechanisms in organisms. Thus, the change in the content of chitin and UDP-GlcNAc may affect the expression level of other genes involved in the UDP-GlcNAc biosynthesis pathway. The decrease in chitin and UDP-GlcNAc content induced the high expression of SS1G-01798, SS1G-14122 (GNA1), and SS1G-05671 (UAP1) in SsAGM1 gene-silenced transformants. Moreover, the increase in the expression of SS1G-01798 and SS1G-14122 (GNA1) and the decrease in expression of SsAGM1 resulted in the excessive accumulation of GlcNAc-6P, although the feedback inhibited the expression of SS1G-04070 (GFA1) in SsAGM1 gene-silenced transformants. However, the expression levels of the four genes were inhibited significantly due to the excessive accumulation of chitin and UDP-GlcNAc in the overexpression transformants (Figure 3D). Staining and quantification assays suggested that SsAGM1 is involved in the synthesis of chitin in S. sclerotiorum.
The fungal cell wall acts as the first physical line of defense against external stress and maintains the shape of cell (Lesage and Bussey, 2006; Geoghegan et al., 2017). Chitin is an important component of cell walls, and is thought to be indispensable for fungal cell wall integrity and rigidity (Patel and Free, 2019). The deletion of chitin synthase results in impaired cell wall integrity has been shown in many important pathogenic fungi, such as M. oryzae, Colletotrichum graminicola, and B. cinerea (Amnuaykanjanasin and Epstein, 2003; Soulié et al., 2003; Kong et al., 2012). Thus, abnormal synthesis of chitin may indicated that cell wall integrity was impaired in SsAGM1 gene-silenced transformants. In this study, SsAGM1gene-silenced transformants were more sensitive to cell wall synthesis inhibitors and osmotic stress compared with WT, whereas the tolerance to cell wall synthesis inhibitors and osmotic stress of SsAGM1 overexpression transformants increased significantly compared with WT (Figures 4, 5). These results further demonstrated that SsAGM1 is involved in cell wall integrity and multiple stress tolerance of S. sclerotiorum.
Sclerotia are a long-term survival structure of S. sclerotiorum and the central component of the infection cycle (Bolton et al., 2006). The development and formation mechanism of sclerotia is an important part of the research in S. sclerotiorum. Recent studies have shown that cell wall integrity was related closely to vegetative growth and sclerotia formation of S. sclerotiorum. In S. sclerotiorum, SsFkh1 is involved in the maintenance of cell wall integrity, and SsFkh1 deletion mutants are severely defective in hyphal growth and sclerotia formation (Cong et al., 2022). SsAGM1 was required for chitin synthesis, and it contributed to maintain the integrity of the cell wall. In this study, we found that the silencing of SsAGM1 significantly inhibited the hyphal growth of S. sclerotiorum. In addition, SsAGM1 gene-silenced transformants never formed sclerotia even if the culture time was prolonged (Figure 6). Our results indicated that SsAGM1 was essential for vegetative growth and sclerotia formation in S. sclerotiorum.
Glycoproteins on the cell wall can sense changes in the external environment and can transmit signals into the cell (Beckerman and Ebbole, 1996; Kou and Naqvi, 2016). In plant pathogenic fungi, hydrophobins on the cell wall provide hydrophobicity to fungal surfaces in contact with air to mediate the attachment of hyphae to the hydrophobic surface, which results in morphogenetic signals (Wosten, 2001). The plant surface is equivalent to a hydrophobic surface, and pathogens recognize the hydrophobic surface, which induced the switching of hyphal morphology (Kou and Naqvi, 2016). In M. oryzae, the hydrophobin MPG1 was necessary for host recognition and generated morphogenetic signals that mediated spore germination and differentiation (Beckerman and Ebbole, 1996). Another cell wall-associated proteins, transmembrane mucin Msb2, is one of the key proteins involved in sensing physical stimuli. Deletion of the MSB2 gene resulted in defects in infection structure and virulence in plant-pathogenic fungi (Lanver et al., 2010; Perez-Nadales and Di Pietro, 2011; Leroch et al., 2015). Thus, the fungal cell wall acts as a signal transduction scaffold for morphogenesis, growth, and virulence, and the disruption of the function and integrity of a cell wall usually affects the formation of infected structures. For example, MoTip41 was involved in CWI in M. oryzae, and the deletion of MoTip41 resulted in abnormal appressoria (Qian et al., 2021). In C. higginsianum, ChMK1 played an essential role in cell wall integrity, and the ChMK1 deletion mutant did not form infection structures (Wei et al., 2016). We simulated the hydrophobic surface by using glass slides and observed the infection structure. On glass slides, the hyphae of gene-silenced transformants gathered into bundles, but they could not produce an infection cushion (Figures 7A,B). This indicated that the silencing of SsAGM1 led to a change in cell wall structure, which affected the receptors on cell walls and the transmission of morphogenetic signals.
Chitin is an important structural compound in both the infection structure and the infection hyphae that are formed in plants. The lack of chitin synthase led to serious pathogenicity defects in several pathogens (Weber et al., 2006; Kim et al., 2009; Larson et al., 2011; Kong et al., 2012; Oliveira-Garcia and Deising, 2013). In plant pathogenic fungi, the delicate regulation of the formation of cell walls was required for structural changes in the cell wall during morphogenesis due to infection (Oliveira-Garcia and Deising, 2013). In general, cell wall integrity is closely related to the virulence of pathogens. In M. oryzae, deletion of the chitin synthase-encoding genes Chs1 and Chs7 resulted in reduced virulence, and deletion of Chs6 resulted in loss of pathogenicity (Kong et al., 2012). In B. cinerea, chitin synthase mutant (Δ Bcchs1) has reduced pathogenicity (Soulié et al., 2003). In C. graminicola, the silencing of the β-1,3-glucan synthase gene GLS1 resulted in severe distortion of necrotrophic hyphae and the loss of pathogenicity (Oliveira-Garcia and Deising, 2013). In this study, we found that the gene-silenced transformants grew on the surface of leaves, but could not invade the inside of the leaf by inoculating the strain on isolated leaves. The gene-silenced transformants could not invade the leaves even at 4 days after inoculation (Figures 8A–C). Myelinolysis was found around the agar block, which may have been caused by the immune response of the host. Furthermore, because the gene-silenced transformants did not form infection cushions, we inoculated strains on the wound of the leaf, but there were still no obvious disease symptoms on the leaf (Figure 8C). This indicated that the silencing of SsAGM1 caused severe pathogenicity defects in S. sclerotiorum, but the pathogenicity defects were not caused mainly by poor growth and the inability to penetrate the host. Therefore, the specific mechanism that allows SsAGM1 to affect the pathogenicity in S. sclerotiorum needs further research.
Collectively, this is the first report on functional characterization of AGM1 in plant pathogenic fungi. Our results showed that SsAGM1 was an essential gene for the growth, development, stress response, and pathogenicity in S. sclerotiorum. Furthermore, the catalytic mechanism of SsAGM1 also needs to be clarified to develop new fungicide targets.
Data Availability Statement
The original contributions presented in the study are included in the article/Supplementary Material, further inquiries can be directed to the corresponding author/s.
Author Contributions
YZ, HP, JZ, and KX planned and designed the research. JZ, HH, and ML performed the experiments. JZ and YZ analyzed the data and wrote the manuscript. HP and YZ provided funding. All authors discussed the data, edited, and approved the manuscript.
Funding
This work was supported financially by the Inter-Governmental International Cooperation Special Project of National Key R&D Program of China (2019YFE0114200), the Jilin Province Science and Technology Development Plan Project (20190201025JC), National Natural Science Foundation of China (31972978), and the Thirteenth National Key R&D Program of China (2019YFD1002000).
Conflict of Interest
The authors declare that the research was conducted in the absence of any commercial or financial relationships that could be construed as a potential conflict of interest.
Publisher’s Note
All claims expressed in this article are solely those of the authors and do not necessarily represent those of their affiliated organizations, or those of the publisher, the editors and the reviewers. Any product that may be evaluated in this article, or claim that may be made by its manufacturer, is not guaranteed or endorsed by the publisher.
Acknowledgments
We would like to thank Thomas A. Gavin, Prof. Emeritus, Cornell University, for help with editing this manuscript.
Supplementary Material
The Supplementary Material for this article can be found online at: https://www.frontiersin.org/articles/10.3389/fmicb.2022.938784/full#supplementary-material
Footnotes
References
Alcazar-Fuoli, L., Bayry, J., and Aimanianda, V. (2020). Editorial: the Role of the Fungal Cell Wall in Host-Fungal Interactions. Front. Cell. Infect. Microbiol. 10:392. doi: 10.3389/fcimb.2020.00392
Allan, J., Regmi, R., Denton-Giles, M., Kamphuis, L. G., and Derbyshire, M. C. (2019). The host generalist phytopathogenic fungus Sclerotinia sclerotiorum differentially expresses multiple metabolic enzymes on two different plant hosts. Sci. Rep. 9:19966. doi: 10.1038/s41598-019-56396-w
Amnuaykanjanasin, A., and Epstein, L. (2003). A class V chitin synthase gene, chsA is essential for conidial and hyphal wall strength in the fungus Colletotrichum graminicola (Glomerella graminicola). Fungal Genet. Biol. 38, 272–285. doi: 10.1016/s1087-1845(02)00563-7
Arroyo, J., Farkas, V., Belen Sanz, A., and Cabib, E. (2016). Strengthening the fungal cell wall through chitin-glucan cross-links: effects on morphogenesis and cell integrity. Cell. Microbiol. 18, 1239–1250. doi: 10.1111/cmi.12615
Beckerman, J. L., and Ebbole, D. J. (1996). MPG1, a gene encoding a fungal hydrophobin of Magnaporthe grisea, is involved in surface recognition. Mol. Plant Microbe Interact. 9, 450–456. doi: 10.1094/mpmi-9-0450
Boland, G. J., and Hall, R. (1994). Index of plant hosts of Sclerotinia sclerotiorum. Can. J. Plant Pathol. 16, 93–108. doi: 10.1080/07060669409500766
Bolton, M. D., Thomma, B. P., and Nelson, B. D. (2006). Sclerotinia sclerotiorum (Lib.) de Bary: biology and molecular traits of a cosmopolitan pathogen. Mol. Plant Pathol. 7, 1–16. doi: 10.1111/j.1364-3703.2005.00316.x
Brown, H. E., Esher, S. K., and Alspaugh, J. A. (2020). Chitin: a “Hidden Figure” in the Fungal Cell Wall. Curr. Top. Microbiol. Immunol. 425, 83–111. doi: 10.1007/82_2019_184
Bulik, D. A., Olczak, M., Lucero, H. A., Osmond, B. C., Robbins, P. W., and Specht, C. A. (2003). Chitin synthesis in Saccharomyces cerevisiae in response to supplementation of growth medium with glucosamine and cell wall stress. Eukaryot. Cell 2, 886–900. doi: 10.1128/ec.2.5.886-900.2003
Cong, J., Xiao, K., Jiao, W., Zhang, C., Zhang, X., Liu, J., et al. (2022). The Coupling Between Cell Wall Integrity Mediated by MAPK Kinases and SsFkh1 Is Involved in Sclerotia Formation and Pathogenicity of Sclerotinia sclerotiorum. Front. Microbiol. 13:816091. doi: 10.3389/fmicb.2022.816091
de Groot, P. W. J., Ruiz, C., de Aldana, C. R. V., Duenas, E., Cid, V. J., Del Rey, F., et al. (2001). A genomic approach for the identification and classification of genes involved in cell wall formation and its regulation in Saccharomyces cerevisiae. Comp. Funct. Genomics 2, 124–142. doi: 10.1002/cfg.85
Erental, A., Dickman, M. B., and Yarden, O. (2008). Sclerotial development in Sclerotinia sclerotiorum: awakening molecular analysis of a “Dormant” structure. Fungal Biol. Rev. 22, 6–16. doi: 10.1016/j.fbr.2007.10.001
Fan, H., Yu, G., Liu, Y., Zhang, X., Liu, J., Zhang, Y., et al. (2017). An atypical forkhead-containing transcription factor SsFKH1 is involved in sclerotial formation and is essential for pathogenicity in Sclerotinia sclerotiorum. Mol. Plant Pathol. 18, 963–975. doi: 10.1111/mpp.12453
Fang, W., Du, T., Raimi, O. G., Hurtado-Guerrero, R., Marino, K., Ibrahim, A. F., et al. (2013). Genetic and structural validation of Aspergillus fumigatus N-acetylphosphoglucosamine mutase as an antifungal target. Biosci. Rep. 33:e00063. doi: 10.1042/BSR20130053
Free, S. J. (2013). Fungal cell wall organization and biosynthesis. Adv. Genet. 81, 33–82. doi: 10.1016/B978-0-12-407677-8.00002-6
Geoghegan, I., Steinberg, G., and Gurr, S. (2017). The Role of the Fungal Cell Wall in the Infection of Plants. Trends Microbiol. 25, 957–967. doi: 10.1016/j.tim.2017.05.015
Gow, N. A. R., Latge, J. P., and Munro, C. A. (2017). The Fungal Cell Wall: structure, Biosynthesis, and Function. Microbiol. Spectr. 5, FUNK–0035–2016. doi: 10.1128/microbiolspec.FUNK-0035-2016
Greig, K. T., Antonchuk, J., Metcalf, D., Morgan, P. O., Krebs, D. L., Zhang, J.-G., et al. (2007). Agm1/Pgm-3-mediated sugar nucleotide synthesis is essential for hematopoiesis and development. Mol. Cell. Biol. 27, 5849–5859. doi: 10.1128/mcb.00802-07
Hofmann, M., Boles, E., and Zimmermann, F. K. (1994). Characterization of the essential yeast gene encoding N-acetylglucosamine-phosphate mutase. Eur. J. Biochem. 221, 741–747. doi: 10.1111/j.1432-1033.1994.tb18787.x
Jiao, W., Yu, H., Cong, J., Xiao, K., Zhang, X., Liu, J., et al. (2022). Transcription factor SsFoxE3 activating SsAtg8 is critical for sclerotia, compound appressoria formation, and pathogenicity in Sclerotinia sclerotiorum. Mol. Plant Pathol. 23, 204–217. doi: 10.1111/mpp.13154
Kabbage, M., Williams, B., and Dickman, M. B. (2013). Cell Death Control: the Interplay of Apoptosis and Autophagy in the Pathogenicity of Sclerotinia sclerotiorum. PLoS Pathog. 9:e1003287. doi: 10.1371/journal.ppat.1003287
Kim, J.-E., Lee, H.-J., Lee, J., Kim, K. W., Yun, S.-H., Shim, W.-B., et al. (2009). Gibberella zeae chitin synthase genes, GzCHS5 and GzCHS7, are required for hyphal growth, perithecia formation, and pathogenicity. Curr. Genet. 55, 449–459. doi: 10.1007/s00294-009-0258-6
Kim, K. S., Min, J.-Y., and Dickman, M. B. (2008). Oxalic acid is an elicitor of plant programmed cell death during Sclerotinia sclerotiorum disease development. Mol. Plant Microbe Interact. 21, 605–612. doi: 10.1094/mpmi-21-5-0605
Klis, F. M. (1994). Review: cell wall assembly in yeast. Yeast 10, 851–869. doi: 10.1002/yea.320100702
Klis, F. M., Boorsma, A., and De Groot, P. W. (2006). Cell wall construction in Saccharomyces cerevisiae. Yeast 23, 185–202. doi: 10.1002/yea.1349
Kong, L.-A., Yang, J., Li, G.-T., Qi, L.-L., Zhang, Y.-J., Wang, C.-F., et al. (2012). Different Chitin Synthase Genes Are Required for Various Developmental and Plant Infection Processes in the Rice Blast Fungus Magnaporthe oryzae. PLoS Pathog. 8:e1002526. doi: 10.1371/journal.ppat.1002526
Kou, Y., and Naqvi, N. I. (2016). Surface sensing and signaling networks in plant pathogenic fungi. Semin. Cell Dev. Biol. 57, 84–92. doi: 10.1016/j.semcdb.2016.04.019
Lanver, D., Mendoza-Mendoza, A., Brachmann, A., and Kahmann, R. (2010). Sho1 and Msb2-Related Proteins Regulate Appressorium Development in the Smut Fungus Ustilago maydis. Plant Cell 22, 2085–2101. doi: 10.1105/tpc.109.073734
Larson, T. M., Kendra, D. F., Busman, M., and Brown, D. W. (2011). Fusarium verticillioides chitin synthases CHS5 and CHS7 are required for normal growth and pathogenicity. Curr. Genet. 57, 177–189. doi: 10.1007/s00294-011-0334-6
Latge, J. P., Beauvais, A., and Chamilos, G. (2017). The Cell Wall of the Human Fungal Pathogen Aspergillus fumigatus: biosynthesis, Organization, Immune Response, and Virulence. Annu. Rev. Microbiol. 71, 99–116. doi: 10.1146/annurev-micro-030117-020406
Leroch, M., Mueller, N., Hinsenkamp, I., and Hahn, M. (2015). The signalling mucin Msb2 regulates surface sensing and host penetration via BMP1 MAP kinase signalling in Botrytis cinerea. Mol. Plant Pathol. 16, 787–798. doi: 10.1111/mpp.12234
Lesage, G., and Bussey, H. (2006). Cell wall assembly in Saccharomyces cerevisiae. Microbiol. Mol. Biol. Rev. 70, 317–343. doi: 10.1128/mmbr.00038-05
Li, J., Zhang, Y., Zhang, Y., Yu, P. L., Pan, H., and Rollins, J. A. (2018). Introduction of Large Sequence Inserts by CRISPR-Cas9 To Create Pathogenicity Mutants in the Multinucleate Filamentous Pathogen Sclerotinia sclerotiorum. Mbio 9, e00567–18. doi: 10.1128/mBio.00567-18
Liang, X., and Rollins, J. A. (2018). Mechanisms of Broad Host Range Necrotrophic Pathogenesis in Sclerotinia sclerotiorum. Phytopathology 108, 1128–1140. doi: 10.1094/PHYTO-06-18-0197-RVW
Mbengue, M., Navaud, O., Peyraud, R., Barascud, M., Badet, T., Vincent, R., et al. (2016). Emerging Trends in Molecular Interactions between Plants and the Broad Host Range Fungal Pathogens Botrytis cinerea and Sclerotinia sclerotiorum. Front. Plant Sci. 7:422. doi: 10.3389/fpls.2016.00422
Milewski, S., Gabriel, I., and Olchowy, J. (2006). Enzymes of UDP-GlcNAc biosynthesis in yeast. Yeast 23, 1–14. doi: 10.1002/yea.1337
Mio, T., Yamada-Okabe, T., Arisawa, M., and Yamada-Okabe, H. (2000). Functional cloning and mutational analysis of the human cDNA for phosphoacetylglucosamine mutase: identification of the amino acid residues essential for the catalysis. Biochim. Biophys. Acta Gene Struct. Exp. 1492, 369–376. doi: 10.1016/s0167-4781(00)00120-2
Oliveira-Garcia, E., and Deising, H. B. (2013). Infection structure-specific expression of beta-1,3-glucan synthase is essential for pathogenicity of Colletotrichum graminicola and evasion of beta-glucan-triggered immunity in maize. Plant Cell 25, 2356–2378. doi: 10.1105/tpc.112.103499
Patel, P. K., and Free, S. J. (2019). The Genetics and Biochemistry of Cell Wall Structure and Synthesis in Neurospora crassa, a Model Filamentous Fungus. Front. Microbiol. 10:2294. doi: 10.3389/fmicb.2019.02294
Perez-Nadales, E., and Di Pietro, A. (2011). The Membrane Mucin Msb2 Regulates Invasive Growth and Plant Infection in Fusarium oxysporum. Plant Cell 23, 1171–1185. doi: 10.1105/tpc.110.075093
Plaza, V., Silva-Moreno, E., and Castillo, L. (2020). Breakpoint: cell Wall and Glycoproteins and their Crucial Role in the Phytopathogenic Fungi Infection. Curr. Protein Pept. Sci. 21, 227–244. doi: 10.2174/1389203720666190906165111
Qian, B., Liu, X., Ye, Z., Zhou, Q., Liu, P., Yin, Z., et al. (2021). Phosphatase-associated protein MoTip41 interacts with the phosphatase MoPpe1 to mediate crosstalk between TOR and cell wall integrity signalling during infection by the rice blast fungus Magnaporthe oryzae. Environ. Microbiol. 23, 791–809. doi: 10.1111/1462-2920.15136
Qu, X., Yu, B., Liu, J., Zhang, X., Li, G., Zhang, D., et al. (2014). MADS-Box Transcription Factor SsMADS Is Involved in Regulating Growth and Virulence in Sclerotinia sclerotiorum. Int. J. Mol. Sci. 15, 8049–8062. doi: 10.3390/ijms15058049
Raimi, O. G., Hurtado-Guerrero, R., and van Aalten, D. M. F. (2018). Evidence for substrate-assisted catalysis in N-acetylphosphoglucosamine mutase. Biochem. J. 475, 2547–2557. doi: 10.1042/BCJ20180172
Ram, A. F., and Klis, F. M. (2006). Identification of fungal cell wall mutants using susceptibility assays based on Calcofluor white and Congo red. Nat. Protoc. 1, 2253–2256. doi: 10.1038/nprot.2006.397
Rollins, J. A. (2003). The Sclerotinia sclerotiorum pac1 gene is required for sclerotial development and virulence. Mol. Plant Microbe Interact. 16, 785–795. doi: 10.1094/mpmi.2003.16.9.785
Seifbarghi, S., Borhan, M. H., Wei, Y., Coutu, C., Robinson, S. J., and Hegedus, D. D. (2017). Changes in the Sclerotinia sclerotiorum transcriptome during infection of Brassica napus. BMC Genomics 18:266. doi: 10.1186/s12864-017-3642-5
Soulié, M. C., Piffeteau, A., Choquer, M., Boccara, M., and Vidal-Cros, A. (2003). Disruption of Botrytis cinerea class I chitin synthase gene Bcchs1 results in cell wall weakening and reduced virulence. Fungal Genet. Biol. 40, 38–46. doi: 10.1016/s1087-1845(03)00065-3
Tamura, K., Dudley, J., Nei, M., and Kumar, S. (2007). MEGA4: molecular evolutionary genetics analysis (MEGA) software version 4.0. Mol. Biol. Evol. 24, 1596–1599. doi: 10.1093/molbev/msm092
Thompson, J. D., Higgins, D. G., and Gibson, T. J. (1994). CLUSTAL W: improving the sensitivity of progressive multiple sequence alignment through sequence weighting, position-specific gap penalties and weight matrix choice. Nucleic Acids Res. 22, 4673–4680. doi: 10.1093/nar/22.22.4673
Weber, I., Assmann, D., Thines, E., and Steinberg, G. (2006). Polar localizing class V myosin chitin synthases are essential during early plant infection in the plant pathogenic fungus Ustilago maydis. Plant Cell 18, 225–242. doi: 10.1105/tpc.105.037341
Wei, W., Xiong, Y., Zhu, W., Wang, N., Yang, G., and Peng, F. (2016). Colletotrichum higginsianum Mitogen-Activated Protein Kinase ChMK1: role in Growth. Front. Microbiol. 7:1212. doi: 10.3389/fmicb.2016.01212
Williams, B., Kabbage, M., Kim, H. J., Britt, R., and Dickman, M. B. (2011). Tipping the balance: sclerotinia sclerotiorum secreted oxalic acid suppresses host defenses by manipulating the host redox environment. PLoS Pathog. 7:e1002107. doi: 10.1371/journal.ppat.1002107
Wosten, H. A. B. (2001). Hydrophobins: multipurpose proteins. Annu. Rev. Microbiol. 55, 625–646. doi: 10.1146/annurev.micro.55.1.625
Xia, S., Xu, Y., Hoy, R., Zhang, J., Qin, L., and Li, X. (2019). The Notorious Soilborne Pathogenic Fungus Sclerotinia sclerotiorum: an Update on Genes Studied with Mutant Analysis. Pathogens 9:27. doi: 10.3390/pathogens9010027
Xu, L., Li, G., Jiang, D., and Chen, W. (2018). Sclerotinia sclerotiorum: an Evaluation of Virulence Theories. Annu. Rev. Phytopathol. 56, 311–338. doi: 10.1146/annurev-phyto-080417-050052
Xu, T., Li, J., Yu, B., Liu, L., Zhang, X., Liu, J., et al. (2018). Transcription Factor SsSte12 Was Involved in Mycelium Growth and Development in Sclerotinia sclerotiorum. Front. Microbiol. 9:2476. doi: 10.3389/fmicb.2018.02476
Yu, P. L., and Rollins, J. A. (2022). The cAMP-dependent protein kinase A pathway perturbs autophagy and plays important roles in development and virulence of Sclerotinia sclerotiorum. Fungal Biol. 126, 20–34. doi: 10.1016/j.funbio.2021.09.004
Keywords: Sclerotinia sclerotiorum, pathogenicity, SsAGM1, infection cushion, sclerotia, chitin, UDP-GlcNAc
Citation: Zhang J, Xiao K, Li M, Hu H, Zhang X, Liu J, Pan H and Zhang Y (2022) SsAGM1-Mediated Uridine Diphosphate-N-Acetylglucosamine Synthesis Is Essential for Development, Stress Response, and Pathogenicity of Sclerotinia sclerotiorum. Front. Microbiol. 13:938784. doi: 10.3389/fmicb.2022.938784
Received: 08 May 2022; Accepted: 06 June 2022;
Published: 23 June 2022.
Edited by:
Md. Motaher Hossain, Bangabandhu Sheikh Mujibur Rahman Agricultural University, BangladeshReviewed by:
Wenxia Fang, Guangxi Academy of Sciences, ChinaJiatao Xie, Huazhong Agricultural University, China
Copyright © 2022 Zhang, Xiao, Li, Hu, Zhang, Liu, Pan and Zhang. This is an open-access article distributed under the terms of the Creative Commons Attribution License (CC BY). The use, distribution or reproduction in other forums is permitted, provided the original author(s) and the copyright owner(s) are credited and that the original publication in this journal is cited, in accordance with accepted academic practice. No use, distribution or reproduction is permitted which does not comply with these terms.
*Correspondence: Hongyu Pan, cGFuaG9uZ3l1QGpsdS5lZHUuY24=; Yanhua Zhang, eWhfemhhbmdAamx1LmVkdS5jbg==