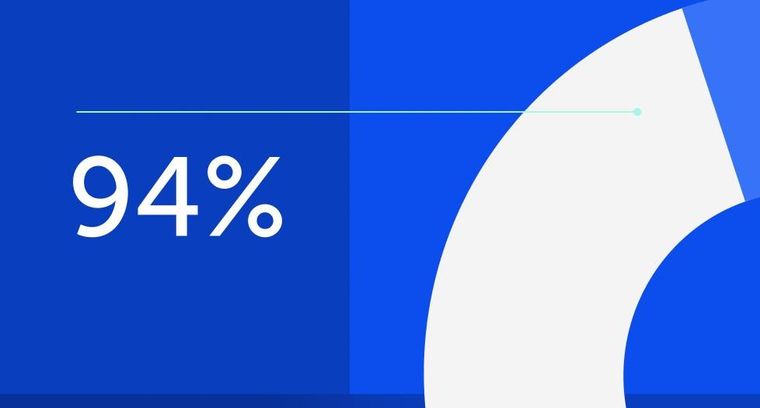
94% of researchers rate our articles as excellent or good
Learn more about the work of our research integrity team to safeguard the quality of each article we publish.
Find out more
REVIEW article
Front. Microbiol., 01 July 2022
Sec. Microbial Physiology and Metabolism
Volume 13 - 2022 | https://doi.org/10.3389/fmicb.2022.933160
This article is part of the Research TopicAlgal PhotosynthesisView all 13 articles
Light reaction of photosynthesis is efficiently driven by protein complexes arranged in an orderly in the thylakoid membrane. As the 5th complex, NAD(P)H dehydrogenase complex (NDH-1) is involved in cyclic electron flow around photosystem I to protect plants against environmental stresses for efficient photosynthesis. In addition, two kinds of NDH-1 complexes participate in CO2 uptake for CO2 concentration in cyanobacteria. In recent years, great progress has been made in the understanding of the assembly and the structure of NDH-1. However, the regulatory mechanism of NDH-1 in photosynthesis remains largely unknown. Therefore, understanding the regulatory mechanism of NDH-1 is of great significance to reveal the mechanism of efficient photosynthesis. In this mini-review, the author introduces current progress in the research of cyanobacterial NDH-1. Finally, the author summarizes the possible regulatory mechanism of cyanobacterial NDH-1 in photosynthesis and discusses the research prospect.
Cyanobacteria belong to prokaryotic photosynthetic organisms. Like other green plants, the light reaction of photosynthesis is carried out with the participation of two photosystems (photosystem I and II, PSI and PSII). Light energy absorbed by antenna pigments is transferred to the photosystem reaction centers and is converted to assimilative power (ATP and NADPH) via a series of electron transporters. Electron transport between PSI and PSII is mediated by the cytochrome b6f complex (Cyt b6f), coupling the translocation of protons across the thylakoid membranes. The resulting proton concentration difference (ΔpH) and membrane potential difference (Δψ) between inside and outside the thylakoid membrane drive ATP synthase (ATPase) to synthesize ATP. The electron transfer in this process is known as linear electron transport (LET). In addition, the donation of electrons from the PSI reduction side to the electron carriers between two photosystems to form circle electron transport is known as cyclic electron transport around PSI (CET-PSI). CET-PSI is also coupled with the formation of ATP, but there is no accumulation of NADPH or other reducing substances. NADPH and ATP produced by the light reaction are used for CO2 fixation (carbon assimilation). Compared with carbon assimilation, the light energy utilization efficiency in the light reaction is higher. The light reaction is driven by protein complexes arranged orderly on the thylakoid membrane. The protein complexes involved in LET include PSI, PSII, cytochrome b6/f complex, and ATPase, while the complexes involved in CET-PSI include thylakoid membrane-bound NAD(P)H dehydrogenase-like complex (chloroplast NDH or cyanobacterial NDH-1). Studies have demonstrated that NDH/NDH-1 mediated CET-PSI (Mi et al., 1992, 1994, 1995) can protect plants from photoinhibition caused by various stress environmental conditions (Endo et al., 1999; Mi et al., 2001; Wang et al., 2006) for efficient photosynthesis (Munekaga et al., 2004).
Cyanobacteria equip a series of environmental adaptations to efficiently fixate inorganic carbon (Ci) under CO2-limited conditions. All these adaptations, including the active uptake of Ci, the subsequent localized elevation of CO2 around the primary CO2-fixing enzyme, ribulose 1,5-bisphosphate carboxylase/oxygenase (Rubisco), and the partitioning of Rubisco into the carboxysome, are collectively considered as the carbon concentrating mechanism (CCM) (Badger et al., 2002; Badger and Price, 2003; Raven, 2003; Giordano et al., 2005). CCM requires the coordination of two systems, an inorganic carbon transporter system and the carboxysome containing Rubisco. To date, five inorganic carbon transporters have been found, including two Na+-dependent transporters (BicA and SbtA), one ATPase-dependent transporter (BCT1), and two CO2-uptake NDH-1 (type 1 NDH) complexes in Synechocystis sp. PCC 6803 (hereafter Syne6803) and other cyanobacterial strains (Ogawa and Kaplan, 2003; Ogawa and Mi, 2007; Price, 2011). Therefore, cyanobacterial NDH-1 complexes function not only in light reactions but also in carbon assimilation as an important regulator. This mini-review summarizes the recent research progress of characterization and functions of cyanobacterial NDH-1 in photosynthesis and prospects for the future research direction.
Through the genome comparison of chloroplast and cyanobacteria, 15 highly homologous ndh genes, namely NdhA-O, were found in cyanobacteria (Kaneko et al., 1996) and the corresponding homologous proteins (NdhA-O) were identified (Friedrich and Scheide, 2000; Prommeenate et al., 2004; Rumeau et al., 2005). An additional 4 proteins NdhP, NdhQ, NdhS, and NdhV were successively identified (Battchikova et al., 2011a; Nowaczyk et al., 2011; Chen et al., 2016). In contrast to the crystal structure of Complex I (Baradaran et al., 2013), cyanobacterial NDH-1 is speculated to possess an oxygenic photosynthesis-specific (OPS) domain (Birungi et al., 2010) comprised of NdhL-NdhQ, -NdhS, -NdhV. NdhL is necessary for the docking of hydrophilic subcomplex to hydrophobic subcomplex in both cyanobacteria (Battchikova et al., 2005) and plants (Shimizu et al., 2008). Deletion of NdhL lowered CET-PSI activity in cyanobacteria (Mi et al., 1992). By studying NDH-1 mutants and protein-protein interaction of multiple subunits, our group found that NdhM locates in the core of the NDH-1 hydrophilic arm of cyanobacteria and plays a key role in the assembly and activity of the NDH-1 hydrophilic arm (He et al., 2016). NdhN also affects the assembly and activity of NDH-1 (He and Mi, 2016). In contrast, although the little contribution of NdhO to CET-PSI activity, it plays an important role in respiratory metabolism under the condition of limited inorganic carbon (He and Mi, 2016). NdhV is a subunit which functions in regulation of NDH-1 activity. Mutation of NdhV resulted in the instability and decrease of NDH activity in Arabidopsis (Fan et al., 2015), loss of the upregulation of NDH-1 level and activity induced at high light (Chen et al., 2016), and causing heat sensitivity (Gao et al., 2016) in cyanobacteria. E. coli NDH-1 complex is the mode of complex I with the smallest components of protein subunits, composed of 14 subunits from NuoA to NuoN. This smallest module carries out the most basic energy conversion reaction (Friedrich and Scheide, 2000). Comparing the NDH composition of E. coli, cyanobacteria and chloroplasts indicate that NdhA-K is a conserved component of NDH-1 in prokaryotes and eukaryotes. However, the homologous proteins of three subunits NuoE, NuoF, and NuoG involved in NADH oxidation in E. coli were not found in chloroplasts and cyanobacteria. Since these three subunits contain NADH-binding sites, cofactor FMN, and iron-sulfur clusters, whether thylakoid membrane NDH-1 can directly oxidize NADH, or NADPH remains to be confirmed. Experiment using thylakoid membranes has demonstrated that ferredoxin (Fd) can donate an electron to PQ via cyanobacterial NDH-1 (Mi et al., 1995). At present, it is believed that the electron donor of chloroplast NDH is Fd rather than NAD (P) H (Shikanai, 2014), which is based on their laboratory study that the C-terminal of CRR31 (NdhS) subunit from Arabidopsis (Yamamoto et al., 2011; Yamamoto and Shikanai, 2013) and cyanobacterium Syne 6803 (Battchikova et al., 2011a), contains SH3 (Src homology 3) domain-like fold, which serves as Fd docking site. In addition, in vitro experiments have proved that the reduction of PQ is required for binding Fd and catalytic activity (Yamamoto et al., 2011). The C-terminal of NdhS of cyanobacteria is conserved, indicating that the electron donor element of thylakoid membrane NDH is conserved (Battchikova et al., 2011b). It was also demonstrated that thermophilic cyanobacterial Fd can interact with NDH-1 probably via the interaction of NdhS with NdhH or NdhI by using surface plasmon resonance (SPR) (He et al., 2015). Recently, cyanobacterial NDH-1 structures provided strong evidence that Fd binds with cyanobacterial NDH-1 via extensive contact with NdhI and NdhH (Pan et al., 2020; Zhang et al., 2020).
Proteomic analysis of cyanobacterial NDH-1 complexes has revealed the presence of three complexes NDH-1L/-1L' (large size), NDH-1M (medium size), and NDH-1S (small size) in Synechosystis PCC 6803 (Herranen et al., 2004). NDH-1L/-1L' is composed of NDH-1M, NdhD1/D2, NdhF1, NdhP, and NdhQ, which are involved in respiration and CET-PSI, while NDH-MS is composed of NDH-1M and NDH-1S (including NdhD3, NdhF3, CupA, and CupS) and NDH-MS' composed of NDH-1M and NDH-1S' (including NdhD3, NdhF3, CupB, and CupS') participate in CO2 uptake (Ogawa and Mi, 2007). Three types of cyanobacteria NDH-1 complexes contain NDH-1M as a skeleton. The expression of NDH-1L is stable under different growth conditions; however, the NDH-1L' has not been detected on the protein level (Zhang et al., 2004). Similar to bacterial complex I, NDH-1L forms an L-shaped architecture by analysis of the complex isolated from Thermosynechococcus elongatus strain earlier with electron microscopy in low resolution (Arteni et al., 2006) and recently with cryo-EM in high resolution: the previous two structures contained no NdhV (Laughlin et al., 2019; Schuller et al., 2019) and the latter two structures contained NdhV and Fd (Pan et al., 2020; Zhang et al., 2020). NDH-1MS is inducible at limiting inorganic carbon conditions and has a high uptake affinity for CO2, which is easily dissociated into NDH-1M and NDH-1S (Herranen et al., 2004). The NDH-1MS complex has been isolated from a Thermosynechococcus elongatus strain in which the C terminus of NdhL has been tagged with 6-His. This complex is easily dissociated into NDH-1M and NDH-1S complexes (Zhang et al., 2005). Single-particle electron microscopy analysis of purified thylakoid membrane components indicated that NDH-1MS had a U-shaped structure (Arteni et al., 2006). CupA is responsible for the formation of a U-shape by binding at the tip of the membrane-bound arm of NDH-1MS in both T. elongatus and Syne 6803 (Folea et al., 2008). The structure of NDH-1MS has also been resolved by cryo-EM (Schuller et al., 2020). NDH-1MS' is involved in the complex of constitutive CO2 uptake, and its key gene CupB is homologous with CupA (Madsen et al., 2002; Shibata et al., 2002). CupB protein is dependent on NdhD4 to locate on the thylakoid membrane, and a 450 kDa NDH-1MS' complex was identified by isolation and purification (Xu et al., 2008).
The plastid coding subunits of NDH in terrestrial plant chloroplast are highly homolog to NDH-1L in cyanobacteria. In addition, plant chloroplast NDH contains at least 18 nucleus-encoded subunits formed from different subcomplexes (Ifuku et al., 2011; Ueda et al., 2012). Chloroplast NDH can form a supercomplex with a PSI core via two minor light-harvesting complexes I (LHCI) subunits, Lhca5 and Lhca5, for the association of PSI-LHCI (Kouril et al., 2014; Otani et al., 2018). Recently, two research works have reported the structure of chloroplast PSI-NDH supercomplex that the PSI-NDH is composed of two copies of the PSI-LHCI subcomplex, one NDH complex, and two monomeric LHCI proteins, Lhca5 and Lhca6, mediate the binding of two PSI complexes to NDH (Shen et al., 2022; Su et al., 2022).
As the fifth complex, chloroplast NDH only participates in CET-PSI and cyanobacterial NDH-1 is also involved in CCM, playing important role in regulating photosynthesis. Figure 1 summarizes the possible mechanism of NDH-1 based on a series of research works.
Figure 1. A schematic representation of the possible mechanism of cyanobacterial NDH-1 in photosynthesis. As NDH-1L is involved in CET-PSI and respiratory pathways, NDH-1MS' and NDH-MS participate in CO2 uptake. These pathways couple the generation of trans-thylakoid membrane proton gradient to synthesize ATP for photoprotection, photosynthetic machinery regulation, and energy supply for carbon assimilation, especially under changing environments.
Cyanobacteria could survive in a low CO2 environment in water due to their CCMs, which can increase the CO2 concentration around the active site of Rubisco, the key enzyme of photosynthetic carbon assimilation, and overcome their low affinity for CO2, thereby effectively assimilating CO2 (Price et al., 1998; Kaplan and Reinhold, 1999). The CCMs and inorganic carbon transport of cyanobacteria are ATP-consuming processes. The Syne6803 mutant defective in NdhB (Ogawa, 1991a), NdhH, NdhJ, NdhN, or NdhM (He and Mi, 2016; He et al., 2016) completely lost CET-PSI activity mediated by HDH-1 and could not survive in the concentration of air CO2. The mutants lost partial NDH-1 activity and grew slower in air CO2 (Ogawa, 1991b; He and Mi, 2016). Klughammer et al. (Klughammer et al., 1999) used NdhD3-, NdhF3-mutants of Synechococcus PCC7002, to study the CO2 uptake efficiency. They observed that these mutants could neither induce efficient CO2 uptake nor efficient carbonate transport. They proposed that NDH-1 special subunits encoded by NdhD3 and NdhF participate in high-affinity CO2 uptake. Ogawa's group proposed the involvement of NDH-1 in energy conduction and induction of a high-affinity inorganic carbon transport by studying mutants involved in CCM (Ohkawa et al., 2000). Further research indicated that deletion of NdhDs suppressed the building up of the trans-thylakoidal ΔpH resulting in the loss of the CO2 uptake function (Han et al., 2017). Therefore, cyanobacterial NDH-1 would provide ATP for CCM.
As the assimilation force, ATP and NADPH produced in photoreaction are used for CO2 assimilation. According to theoretical calculations, the ratio of ATP/NADPH required to assimilate each molecule of CO2 is 1.5. However, in C3 plants, due to the existence of photorespiration, the required theoretical value of ATP/NADPH increases to 1.66. When plants are in various developmental stages with different energy requirements or facing environmental changes, the fixed proportion of ATP and NADPH produced by LET often could not meet the needs of CO2 assimilation. Since CET-PSI only produces ATP, additional ATP can be provided (Shikanai, 2007) to meet the needs of ATP for photosynthetic carbon assimilation. In Syne6803, deletion of NdhV caused a decrease in a trans-thylakoid membrane ΔpH and loss of the upregulation of CET-PSI activity at high light (Chen et al., 2016). Also, the mutant that deleted either NdhD3 or NdhD4 significantly lowered the trans-thylakoid membrane's proton gradient (Han et al., 2017) and could not survive under CO2 conditions. Therefore, the ΔpH generated by NDH-1 drives the ATPase to synthesize ATP for active CO2 uptake and regulation of pH in the cytosol.
Hibino et al. reported that the expression of NdhK and NdhI genes was increased under high salt concentration, and CET-PSI mediated by NDH-1 was accordingly promoted (Hibino et al., 1996). Under the shock of a high concentration of salt, the ndhB deletion mutant could not restore its photosynthetic ability (Tanaka et al., 1997). Therefore, it is considered that CET-PSI mediated by NDH-1 is essential for cyanobacteria to adapt to salt-stressed condition. Based on plants, adapting to stressed conditions is an energy-consuming process; it is suggested that CET-PSI mediated by NDH-1 might provide extra ATP for cells to adapt to these energy-consuming reactions. The demand and proportion of ATP and NADPH in cyanobacteria and higher plants vary with the change in the photosynthetic environment or developmental stages. When the CO2 concentration becomes low, it is necessary to concentrate CO2 by consuming energy, to increase the demand for ATP. The proportion of ATP and NADPH produced by LET is certain and could not be adjusted. However, through CET-PSI, it is possible to adjust the proportion of ATP and NADPH.
In studying the kinetics of NADPH fluorescence (blue-green fluorescence), we observed that when the inhibitor of Calvin cycle iodoacetic acid (IAA) was added, the amplitude of rapid NADP reduction is approximately doubled in wild-type but not in the ndhB gene deletion mutant M55 cells. Apparently, in wild-type cells, the rate of the Calvin cycle is sufficiently high to prevent full NADP reduction even upon the onset of saturating illumination. This is possible only if there is a correspondingly high ATP supply. On the other hand, in M55 cells, full NADP reduction is obtained. This suggests that in M55 cells, ATP limits the activity of the Calvin cycle due to the lack of CET-PSI phosphorylation (Mi, 2000).
The redistribution of excitation energy between the two light systems (state transitions) is mainly to adjust the excitation imbalance of the two photosystems. It is a mechanism for plants to resist the light damage caused by excessive excitation energy. In cyanobacteria, it has been reported that NDH-1 participates in state transitions of excitation energy between PS I and II by CET-PSI. NDH-1 inactivated mutants, M55, lost the function of state transitions and were locked in state 1 (Schreiber et al., 1995). The mobility of the major accessory's light-harvesting complex phycobilisome (PBS) is related to state transitions, CET-PSI, and respiration. The immobilization of PBS at PSII inhibited the increase in CET-PSI and decrease in respiration that occurred during the movement of PBS from PSII to PSI. In contrast, the immobilization of PBS at PSI inhibited the increase in respiration and decrease in CET-PSI that occurred when PBS moved from PSI to PSII (Ma et al., 2007). State transitions in cyanobacteria, dependent on “spillover” and membrane fluidity, are induced by redox changes in the PQ pool. The light-induced state transitions induced by PBS movement caused redox poise of the PQ pool. A protein kinase known as Stt7/Stn7 has been implicated in state transitions by acting as the LHC II kinase, it seems necessary to assume that a bifurcated redox signaling pathway carries information from the PQ pool (Allen et al., 2011). NDH-I-mediated CET-PSI might participate in the redistribution of excitation energy by affecting the redox state of plastoquinone (Ma et al., 2007, 2008). However, how the redox state is sensed in cyanobacterial state transition is not clear. Plants balance their redox system by regulating CET-PSI or respiratory electron transport so that it will not be over oxidized or over-reduced. Under normal physiological conditions, when the Calvin cycle is fully active, the reduction is mainly caused by LET, and the proportion of CET-PSI is small enough to be ignored. However, when CO2 assimilation is decreased, NADPH and reduced ferredoxin will accumulate too much, and CET-PSI would be promoted under stressed conditions. We observed that when dark-adapted Syne6803 cells were moved to light, NDH-1 expression was significantly induced, and NDH-1 mediated CET-PSI and respiratory electron transport were upregulated (Mi et al., 2001). Therefore, it is suggested that NDH-1 mediated CET-PSI might co-operate with respiratory alternative oxidase transfer electrons to O2, thereby avoiding the over-reduction of the PQ pool under various stresses.
Our group found that the CO2 uptake systems NDH-1MS and NDH-1MS' are associated with a carbonic anhydrase EcaB, which catalyzes the conversion of CO2 into in response to high pH values, high light, or low CO2 concentrations, thereby helping to balance the redox state of inter photosystem electron carriers and maintain efficient photosynthesis (Sun et al., 2019).
Photosynthesis needs light energy, but the excess excitation energy would cause photoinhibition and even cause photodamage of PSII. The CET-PSI is involved in eliminating excess light energy through the mechanism of heat dissipation to protect plants from light damage caused by strong light. CET-PSI can also reduce photoinhibition by downstream regulation of PSII by generating transmembrane proton gradient (Heber, 1992). We found that the deletion of ndhc-j-k in tobacco leads to the accumulation of reactive oxygen species under low or high-temperature stresses (Wang et al., 2006). In addition, a low concentration of NaHSO3 promotes CET-PSI in Syne6803 (Wang et al., 2003) and tobacco under the condition of dark-light transition, alleviating photooxidative damage and improving photosynthesis by promoting photosynthetic phosphorylation mediated by NDH-1 (Wu et al., 2011, 2012).
The NDH-1 plays an important role in the regulation of efficient operation of photosynthetic apparatus under changed environmental conditions. Structure bases have been made in NDH-1L and NDH-1MS. It is not clear whether there is a cross-talk between NDH-1L mediated CET-PS I and NDH-1MS/NDH-1MS' involvement in CO2 uptake. How does NDH-1 function in coordination with light reaction and carbon assimilation? In the future, we may use a high-resolution cryo-EM to observe and analyze active forms and states of NDH-1, and deeply study the dynamic regulation mechanism of NDH-1. With the development of molecular biology, proteomics, metabolomics, computational biology, and structural biology, it is possible to reveal the complicated regulatory network of NDH-1 in photosynthesis.
MH wrote this manuscript.
This work was supported by funds from Synthesis Biology [2019YFA0904602] and the Strategic Priority Research Program of CAS [XDB27020106].
The author declares that the research was conducted in the absence of any commercial or financial relationships that could be construed as a potential conflict of interest.
All claims expressed in this article are solely those of the authors and do not necessarily represent those of their affiliated organizations, or those of the publisher, the editors and the reviewers. Any product that may be evaluated in this article, or claim that may be made by its manufacturer, is not guaranteed or endorsed by the publisher.
Allen, J. F., Santabarbara, S., Allen, C. A., and Puthiyaveetil, S. (2011). Discrete redox signaling pathways regulate photosynthetic light-harvesting and chloroplast gene transcription. PLoS ONE 6, e026372. doi: 10.1371/journal.pone.0026372
Arteni, A. A., Zhang, P. P., Battchikova, N., Ogawa, T., Aro, E. M., and Boekema, E. J. (2006). Structural characterization of NDH-1 complexes of Thermosynechococcus elongatus by single particle electron microscopy. Biochimica Et Biophysica Acta-Bioenergetics 1757, 1469–1475. doi: 10.1016/j.bbabio.2006.05.042
Badger, M. R., Hanson, D., and Price, G. D. (2002). Evolution and diversity of CO2 concentrating mechanisms in cyanobacteria. Funct. Plant Biol. 29, 161–173. doi: 10.1071/PP01213
Badger, M. R., and Price, G. D. (2003). CO2 concentrating mechanisms in cyanobacteria: molecular components, their diversity and evolution. J. Exp. Bot. 54, 609–622. doi: 10.1093/jxb/erg076
Baradaran, R., Berrisford, J. M., Minhas, G. S., and Sazanov, L. A. (2013). Crystal structure of the entire respiratory complex I. Nature 494, 443–448. doi: 10.1038/nature11871
Battchikova, N., Eisenhut, M., and Aro, E.-M. (2011b). Cyanobacterial NDH-1 complexes: Novel insights and remaining puzzles. Biochim. Biophys. Acta-Bioenerg. 1807, 935–944. doi: 10.1016/j.bbabio.2010.10.017
Battchikova, N., Wei, L., Du, L., Bersanini, L., Aro, E.-M., and Ma, W. (2011a). Identification of novel Ssl0352 protein (NdhS), essential for efficient operation of cyclic electron transport around photosystem I, in NADPH:plastoquinone oxidoreductase (NDH-1) complexes of synechocystis sp. PCC 6803. J. Biol. Chem. 286, 36992–37001. doi: 10.1074/jbc.M111.263780
Battchikova, N., Zhang, P. P., Rudd, S., Ogawa, T., and Aro, E. M. (2005). Identification of NdhL and Ssl1690 (NdhO) in NDH-1L, and NDH-1M complexes of Synechocystis sp PCC 6803. J. Biol. Chem. 280, 2587–2595. doi: 10.1074/jbc.M410914200
Birungi, M., Folea, M., Battchikova, N., Xu, M., Mi, H. L., Ogawa, T., et al. (2010). Possibilities of subunit localization with fluorescent protein tags and electron microscopy examplified by a cyanobacterial NDH-1 study. Biochim. Biophys. Acta-Bioenergetics 1797, 1681–1686. doi: 10.1016/j.bbabio.2010.06.004
Chen, X., He, Z., Xu, M., Peng, L., and Mi, H. (2016). NdhV subunit regulates the activity of type-1 NAD(P)H dehydrogenase under high light conditions in cyanobacterium Synechocystis sp PCC 6803. Sci. Rep. 6:2016. doi: 10.1038/srep28361
Endo, T., Shikanai, T., Takabayashi, A., Asada, K., and Sato, F. (1999). The role of chloroplastic NAD(P)H dehydrogenase in photoprotection. FEBS Lett. 457, 5–8. doi: 10.1016/S0014-5793(99)00989-8
Fan, X., Zhang, J., Li, W., and Peng, L. (2015). The NdhV subunit is required to stabilize the chloroplast NADH dehydrogenase-like complex in Arabidopsis. Plant J. 82, 221–231. doi: 10.1111/tpj.12807
Folea, I. M., Zhang, P., Nowaczyk, M. M., Ogawa, T., Aro, E.-M., and Boekema, E. J. (2008). Single particle analysis of thylakoid proteins from Thermosynechococcus elongatus and Synechocystis 6803: Localization of the CupA subunit of NDH-1. FEBS Lett. 582, 246–251. doi: 10.1016/j.febslet.2007.12.012
Friedrich, T., and Scheide, D. (2000). The respiratory complex I of bacteria, archaea and eukarya and its module common with membrane-bound multisubunit hydrogenases. FEBS Lett. 479, 1–5. doi: 10.1016/S0014-5793(00)01867-6
Gao, F., Zhao, J., Wang, X., Qin, S., Wei, L., and Ma, W. (2016). NdhV is a subunit of NADPH dehydrogenase essential for cyclic electron transport in synechocystis sp strain PCC 6803. Plant Physiol. 170, 752–760. doi: 10.1104/pp.15.01430
Giordano, M., Beardall, J., and Raven, J. A. (2005). CO2 concentrating mechanisms in algae: mechanisms, environmental modulation, and evolution. Annu. Rev. Plant Biol. 56, 99–131. doi: 10.1146/annurev.arplant.56.032604.144052
Han, X., Sun, N., Xu, M., and Mi, H. (2017). Co-ordination of NDH and Cup proteins in CO2 uptake in cyanobacterium Synechocystis sp PCC 6803. J. Exp. Bot. 68, 3869–3877. doi: 10.1093/jxb/erx129
He, Z., and Mi, H. (2016). Functional characterization of the subunits N, H, J, and O of the NAD(P)H dehydrogenase complexes in synechocystis sp strain PCC 6803. Plant Physiol. 171, 1320–1332. doi: 10.1104/pp.16.00458
He, Z., Xu, M., Wu, Y., Lv, J., Fu, P., and Mi, H. (2016). NdhM is required for the stability and the function of NAD(P)H dehydrogenase complexes involved in CO2 uptake in Synechocystis sp. strain PCC 6803. J. Biol. Chem. 2016, 5902–5912. doi: 10.1074/jbc.M115.698084
He, Z., Zheng, F., Wu, Y., Li, Q., Lv, J., Fu, P., et al. (2015). NDH-1L interacts with ferredoxin via the subunit NdhS in Thermosynechococcus elongatus. Photosyn. Res. 126, 341–349. doi: 10.1007/s11120-015-0090-4
Heber, U. (1992). Concerning a dual function of coupled cyclic electro-transport in leaves. Plant Physiol. Plant Physiology 100, 1261–1264. doi: 10.1104/pp.100.4.1621
Herranen, M., Battchikova, N., Zhang, P. P., Graf, A., Sirpio, S., Paakkarinen, V., et al. (2004). Towards functional proteomics of membrane protein complexes in Synechocystis sp PCC 6803. Plant Physiol. 134, 470–481. doi: 10.1104/pp.103.032326
Hibino, T. L. B., Rai, A. K., Ishikawa, H., Kojima, H., Tawada, M., Shimoyama, H., et al. (1996). Salt enhances photosystem I content and cyclic electron flow via NAD(P)H dehydrogenase in halotolerant cyanobacterium Aphanothece halophytica. Aust J Plant Physilol 23, 321–330. doi: 10.1071/PP9960321
Ifuku, K., Endo, T., Shikanai, T., and Aro, E.-M. (2011). Structure of the chloroplast NADH dehydrogenase-like complex: nomenclature for nuclear-encoded subunits. Plant Cell Physiol. 52, 1560–1568. doi: 10.1093/pcp/pcr098
Kaneko, T., Sato, S., Kotani, H., Tanaka, A., Asamizu, E., Nakamura, Y., et al. (1996). Sequence analysis of the genome of the unicellular cyanobacterium Synechocystis sp. strain PCC6803. II. Sequence determination of the entire genome and assignment of potential protein-coding regions (supplement). DNA Res. 3, 185–209. doi: 10.1093/dnares/3.3.185
Kaplan, A., and Reinhold, L. (1999). CO2 concentrating mechanisms in photosynthetic microorganisms. Annu. Rev. Plant Physiol. Plant Mol. Biol. 50:539. doi: 10.1146/annurev.arplant.50.1.539
Klughammer, B., Sultemeyer, D., Badger, M. R., and Price, G. D. (1999). The involvement of NAD(P)H dehydrogenase subunits, NdhD3 and NdhF3, in high-affinity CO2 uptake in Synechococcus sp PCC7002 gives evidence for multiple NDH-1 complexes with specific roles in cyanobacteria. Mol. Microbiol. 32, 1305–1315. doi: 10.1046/j.1365-2958.1999.01457.x
Kouril, R., Strouhal, O., Nosek, L., Lenobel, R., Chamrad, I., Boekema, E. J., et al. (2014). Structural characterization of a plant photosystem I and NAD(P)H dehydrogenase supercomplex. Plant J. 77, 568–576. doi: 10.1111/tpj.12402
Laughlin, T. G., Bayne, A. N., Trempe, J.-F., Savage, D. F., and Davies, K. M. (2019). Structure of the complex I-like molecule NDH of oxygenic photosynthesis. Nature 566, 411–414. doi: 10.1038/s41586-019-0921-0
Ma, W., Ogawa, T., Shen, Y., and Mi, H. (2007). Changes in cyclic and respiratory electron transport by the movement of phycobilisomes in the cyanobacterium Synechocystis sp strain PCC 6803. Biochimica Et Biophysica Acta-Bioenergetics 1767, 742–749. doi: 10.1016/j.bbabio.2007.01.017
Ma, W. M., Deng, Y., and Mi, H. L. (2008). Redox of plastoquinone pool regulates the expression and activity of NADPH dehydrogenase supercomplex in Synechocystis sp strain PCC 6803. Curr. Microbiol. 56, 189–193. doi: 10.1007/s00284-007-9056-x
Madsen, T. V., Olesen, B., and Bagger, J. (2002). Carbon acquisition and carbon dynamics by aquatic isoetids. Aquat. Bot. 73, 351–371. doi: 10.1016/S0304-3770(02)00030-X
Mi, H., Endo, T., Schreiber, U., Ogawa, T., and Asada, K. (1994). Nad(P)H dehydrogenase-dependent cyclic electron flow around photosystem-i in the cyanobacterium synechocystis PCC-6803 - a study of dark-starved cells and spheroplastS. Plant Cell Physiol. 35, 163–173.
Mi, H. K. C. (2000). Schreiber U Light-induced dynamic changes of NADPH fluorescence in Synechocystis PCC 6803 and its ndhB-defective mutant M55. Plant Cell Physiol. 41, 1129–1135. doi: 10.1093/pcp/pcd038
Mi, H. L., Deng, Y., Tanaka, Y., Hibino, T., and Takabe, T. (2001). Photo-induction of an NADPH dehydrogenase which functions as a mediator of electron transport to the intersystem chain in the cyanobacterium Synechocystis PCC6803. Photosyn. Res. 70, 167–173. doi: 10.1023/A:1017946524199
Mi, H. L., Endo, T., Ogawa, T., and Asada, K. (1995). Thylakoid membrane-bound, nadph-specific pyridine-nucleotide dehydrogenase complex mediates cyclic electron-transport in the cyanobacterium synechocystis SP PCC-68038. Plant Cell Physiol. 36, 661–668.
Mi, H. L., Endo, T., Schreiber, U., Ogawa, T., and Asada, K. (1992). Electron donation from cyclic and respiratory flows to the photosynthetic intersystem chain is mediated by pyridine-nucleotide dehydrogenase in the cyanobacterium synechocystis PCC-6803. Plant Cell Physiol. 33, 1233–1237.
Munekaga, Y., Hashimoto, M., Miyaka, C., Tomizawa, K. I., Endo, T., Tasaka, M., et al. (2004). Cyclic electron flow around photosystem I is essential for photosynthesis. Nature 429, 579–582. doi: 10.1038/nature02598
Nowaczyk, M. M., Wulfhorst, H., Ryan, C. M., Souda, P., Zhang, H., Cramer, W. A., et al. (2011). NdhP and NdhQ: two novel small subunits of the cyanobacterial NDH-1 complex. Biochemistry 50, 1121–1124. doi: 10.1021/bi102044b
Ogawa, T. (1991a). A gene homologous to the subunit-2 gene of nadh dehydrogenase is essential to inorganic carbon transport of synechocystis PCC6803. Proc. Natl. Acad. Sci. U.S.A. 88, 4275–4279. doi: 10.1073/pnas.88.10.4275
Ogawa, T. (1991b). Cloning and inactivation of a gene essential to inorganic carbon transport of synechocystis PCC6803. Plant Physiol. 96, 280–284. doi: 10.1104/pp.96.1.280
Ogawa, T., and Kaplan, A. (2003). Inorganic carbon acquisition systems in cyanobacteria. Photosyn. Res. 77, 105–115. doi: 10.1023/A:1025865500026
Ogawa, T., and Mi, H. (2007). Cyanobacterial NADPH dehydrogenase complexes. Photosyn. Res. 93, 69–77. doi: 10.1007/s11120-006-9128-y
Ohkawa, H., Price, G. D., Badger, M. R., and Ogawa, T. (2000). Mutation of ndh genes leads to inhibition of CO2 uptake rather than HCO3- uptake in Synechocystis sp strain PCC 6803. J. Bacteriol. 182, 2591–2596. doi: 10.1128/JB.182.9.2591-2596.2000
Otani, T., Kato, Y., and Shikanai, T. (2018). Specific substitutions of light-harvesting complex I proteins associated with photosystem I are required for supercomplex formation with chloroplast NADH dehydrogenase-like complex. Plant J. 94, 122–130. doi: 10.1111/tpj.13846
Pan, X., Cao, D., Xie, F., Xu, F., Su, X., Mi, H., et al. (2020). Structural basis for electron transport mechanism of complex I-like photosynthetic NAD(P)H dehydrogenase. Nat. Commun. 11:14456. doi: 10.1038/s41467-020-14456-0
Price, G. D. (2011). Inorganic carbon transporters of the cyanobacterial CO2 concentrating mechanism. Photosyn. Res. 109, 47–57. doi: 10.1007/s11120-010-9608-y
Price, G. D., Sultemeyer, D., Klughammer, B., Ludwig, M., and Badger, M. R. (1998). The functioning of the CO2 concentrating mechanism in several cyanobacterial strains: a review of general physiological characteristics, genes, proteins, recent advances. Canad. J. Botany-Revue Canadienne De Botanique 76, 973–1002. doi: 10.1139/cjb-76-6-973
Prommeenate, P., Lennon, A. M., Markert, C., Hippler, M., and Nixon, P. J. (2004). Subunit composition of NDH-1 complexes of Synechocystis sp PCC 6803 - Identification of two new ndh gene products with nuclear-encoded homologues in the chloroplast Ndh complex. J. Biol. Chem. 279, 28165–28173. doi: 10.1074/jbc.M401107200
Raven, J. A. (2003). Inorganic carbon concentrating mechanisms in relation to the biology of algae. Photosyn. Res. 77, 155–171. doi: 10.1023/A:1025877902752
Rumeau, D., Becuwe-Linka, N., Beyly, A., Louwagie, M., Garin, J., and Peltier, G. (2005). New subunits NDH-M, -N, and -O, encoded by nuclear genes, are essential for plastid Ndh complex functioning in higher plants. Plant Cell 17, 219–232. doi: 10.1105/tpc.104.028282
Schreiber, U. E. T., Mi, H. L., and Asada, K. (1995). Quenching analysis of chlorophyll fluorescence by the saturation pulse method: prticular aspects relating to the study of eukayotic algae and cyanobacteria. Plant Cell Physiol. 36, 873–882. doi: 10.1093/oxfordjournals.pcp.a078833
Schuller, J. M., Birrell, J. A., Tanaka, H., Konuma, T., Wulflhorst, H., Cox, N., et al. (2019). Structural adaptations of photosynthetic complex I enable ferredoxin-dependent electron transfer. Science 363:257. doi: 10.1126/science.aau3613
Schuller, J. M., Saura, P., Thiemann, J., Schuller, S. K., Gamiz-Hernandez, A. P., Kurisu, G., et al. (2020). Redox-coupled proton pumping drives carbon concentration in the photosynthetic complex I. Nat. Commun. 11:494. doi: 10.1038/s41467-020-14347-4
Shen, L., Tang, K., Wang, W., Wang, C., Wu, H., Mao, Z., et al. (2022). Architecture of the chloroplast PSI–NDH supercomplex in Hordeum vulgare. Nature 601, 649–654. doi: 10.1038/s41586-021-04277-6
Shibata, M., Ohkawa, H., Katoh, H., Shimoyama, M., and Ogawa, T. (2002). Two CO2 uptake systems in cyanobacteria: four systems for inorganic carbon acquisition in Synechocystis sp strain PCC6803. Funct. Plant Biol. 29, 123–129. doi: 10.1071/PP01188
Shikanai, T. (2007). Regulation of photosynthesis via PSI cyclic electron transport. Photosyn. Res. 91:PS141. doi: 10.1007/978-1-4020-6709-9_214
Shikanai, T. (2014). Central role of cyclic electron transport around photosystem I in the regulation of photosynthesis. Curr. Opin. Biotechnol. 26, 25–30. doi: 10.1016/j.copbio.2013.08.012
Shimizu, H., Peng, L., Myouga, F., Motohashi, R., Shinozaki, K., and Shikanai, T. (2008). CRR23/NdhL is a subunit of the chloroplast NAD(P)H dehydrogenase complex in Arabidopsis. Plant Cell Physiol. 49, 835–842. doi: 10.1093/pcp/pcn058
Su, X., Cao, D., Pan, X., Shi, L., Liu, Z., Dall'OSto, L., et al. (2022). NADH dehydrogenase-like complex with photosystem I from Arabidopsis thaliana. Mol. Plant 15, 454–467. doi: 10.1016/j.molp.2022.01.020
Sun, N., Han, X., Xu, M., Kaplan, A., Espie, G. S., and Mi, H. (2019). A thylakoid-located carbonic anhydrase regulates CO2 uptake in the cyanobacterium Synechocystis sp. PCC 6803. New Phytol. 222, 206–217. doi: 10.1111/nph.15575
Tanaka, Y. K. S., Ishikawa, H., Ogawa, T., and Takabe, T. (1997). Electron flow from NADPH dehydrogenase to photosystem I is required for adaptation to shalt shock in the cyanobacterium Synechocystis sp. PCC 6803. Plant Cell Physiol. 38, 1311–1318. doi: 10.1093/oxfordjournals.pcp.a029123
Ueda, M., Kuniyoshi, T., Yamamoto, H., Sugimoto, K., Ishizaki, K., Kohchi, T., et al. (2012). Composition and physiological function of the chloroplast NADH dehydrogenase-like complex in Marchantia polymorpha. Plant J. 72, 683–693. doi: 10.1111/j.1365-313X.2012.05115.x
Wang, H. W., Mi, H. L., Ye, J. Y., Deng, Y., and Shen, Y. K. (2003). Low concentrations of NaHSO3 increase cyclic photophosphorylation and photosynthesis in cyanobacterium Synechocystis PCC6803. Photosyn. Res. 75, 151–159. doi: 10.1023/A:1022813402265
Wang, P., Duan, W., Takabayashi, A., Endo, T., Shikanai, T., Ye, J. Y., et al. (2006). Chloroplastic NAD(P)H dehydrogenase in tobacco leaves functions in alleviation of oxidative damage caused by temperature stress. Plant Physiol. 141, 465–474. doi: 10.1104/pp.105.070490
Wu, Y., He, W., Ma, W., Shen, Y., and Mi, H. (2012). Low concentrations of NaHSO3 enhance NAD(P)H dehydrogenase-dependent cyclic photophosphorylation and alleviate the oxidative damage to improve photosynthesis in tobacco. Chin. Sci. Bull. 57, 3872–3877. doi: 10.1007/s11434-012-5309-6
Wu, Y., Zheng, F., Ma, W., Han, Z., Gu, Q., Shen, Y., et al. (2011). Regulation of NAD(P)H dehydrogenase-dependent cyclic electron transport around PSI by NaHSO(3) at low concentrations in tobacco chloroplasts. Plant Cell Physiol. 52, 1734–1743. doi: 10.1093/pcp/pcr109
Xu, M., Ogawa, T., Pakrasi, H. B., and Mi, H. (2008). Identification and localization of the CupB protein involved in constitutive CO(2) uptake in the cyanobacterium, Synechocystis sp strain PCC 6803. Plant Cell Physiol. 49, 994–997. doi: 10.1093/pcp/pcn074
Yamamoto, H., Peng, L., Fukao, Y., and Shikanai, T. (2011). An Src homology 3 domain-like fold protein forms a ferredoxin binding site for the chloroplast NADH dehydrogenase-like complex in arabidopsis. Plant Cell 23, 1480–1493. doi: 10.1105/tpc.110.080291
Yamamoto, H., and Shikanai, T. (2013). In planta mutagenesis of Src homology 3 domain-like fold of NdhS, a ferredoxin-binding subunit of the chloroplast NADH dehydrogenase-like complex in arabidopsis A conserved ARG-193 plays a critical role in ferredoxin binding. J. Biol. Chem. 288, 36328–36337. doi: 10.1074/jbc.M113.511584
Zhang, C., Shuai, J., Ran, Z., Zhao, J., Wu, Z., Liao, R., et al. (2020). Structural insights into NDH-1 mediated cyclic electron transfer. Nat. Commun. 11:14732. doi: 10.1038/s41467-020-14732-z
Zhang, P. P., Battchikova, N., Jansen, T., Appel, J., Ogawa, T., and Aro, E. M. (2004). Expression and functional roles of the two distinct NDH-1 complexes and the carbon acquisition complex NdhD3/NdhF3/CupA/Sll1735 in Synechocystis sp PCC 6803. Plant Cell 16, 3326–3340. doi: 10.1105/tpc.104.026526
Keywords: cyanobacteria (blue-green algae), NAD(P)H dehydrogenase complex, photosynthesis, cyclic electron transport around photosystem I, CO2 uptake and concentrating mechanism
Citation: Hualing M (2022) Cyanobacterial NDH-1 Complexes. Front. Microbiol. 13:933160. doi: 10.3389/fmicb.2022.933160
Received: 30 April 2022; Accepted: 24 May 2022;
Published: 01 July 2022.
Edited by:
Weimin Ma, Shanghai Normal University, ChinaReviewed by:
Lianwei Peng, Shanghai Normal University, ChinaCopyright © 2022 Hualing. This is an open-access article distributed under the terms of the Creative Commons Attribution License (CC BY). The use, distribution or reproduction in other forums is permitted, provided the original author(s) and the copyright owner(s) are credited and that the original publication in this journal is cited, in accordance with accepted academic practice. No use, distribution or reproduction is permitted which does not comply with these terms.
*Correspondence: Mi Hualing, bWlobEBjZW1wcy5hYy5jbg==; orcid.org/0000-0003-1021-8372
Disclaimer: All claims expressed in this article are solely those of the authors and do not necessarily represent those of their affiliated organizations, or those of the publisher, the editors and the reviewers. Any product that may be evaluated in this article or claim that may be made by its manufacturer is not guaranteed or endorsed by the publisher.
Research integrity at Frontiers
Learn more about the work of our research integrity team to safeguard the quality of each article we publish.