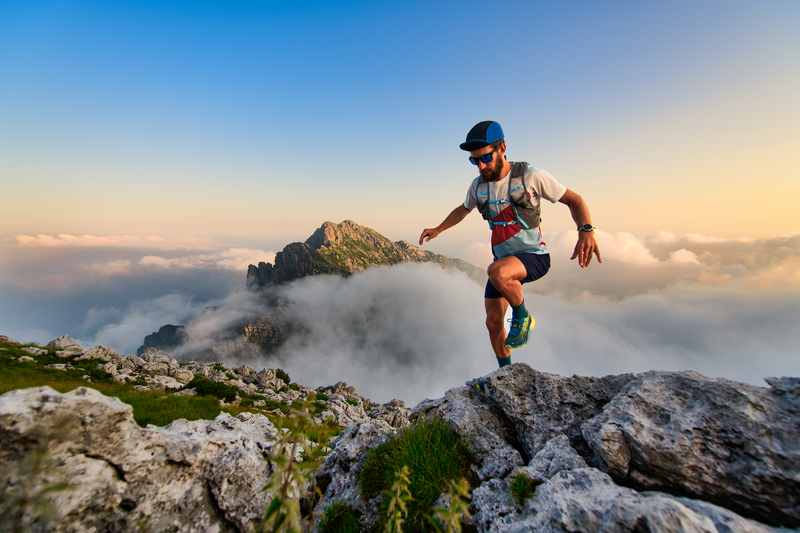
95% of researchers rate our articles as excellent or good
Learn more about the work of our research integrity team to safeguard the quality of each article we publish.
Find out more
REVIEW article
Front. Microbiol. , 09 June 2022
Sec. Antimicrobials, Resistance and Chemotherapy
Volume 13 - 2022 | https://doi.org/10.3389/fmicb.2022.930629
This article is part of the Research Topic Antimicrobial Peptides and mRNA Therapy: Clinical, Veterinary and Plant Pathology Perspectives with Special Attention to Combatting MDR pathogens View all 5 articles
Antibiotics play a vital role in saving millions of lives from fatal infections; however, the inappropriate use of antibiotics has led to the emergence and propagation of drug resistance worldwide. Multidrug-resistant bacteria represent a significant challenge to treating infections due to the limitation of available antibiotics, necessitating the investigation of alternative treatments for combating these superbugs. Under such circumstances, antimicrobial peptides (AMPs), including human-derived AMPs and bacteria-derived AMPs (so-called bacteriocins), are considered potential therapeutic drugs owing to their high efficacy against infectious bacteria and the poor ability of these microorganisms to develop resistance to them. Several staphylococcal species including Staphylococcus aureus, Staphylococcus epidermidis, Staphylococcus haemolyticus, and Staphylococcus saprophyticus are commensal bacteria and known to cause many opportunistic infectious diseases. Methicillin-resistant Staphylococci, especially methicillin-resistant S. aureus (MRSA), are of particular concern among the critical multidrug-resistant infectious Gram-positive pathogens. Within the past decade, studies have reported promising AMPs that are effective against MRSA and other methicillin-resistant Staphylococci. This review discusses the sources and mechanisms of AMPs against staphylococcal species, as well as their potential to become chemotherapies for clinical infections caused by multidrug-resistant staphylococci.
Staphylococci are Gram-positive, facultative anaerobe, and some staphylococcal species are commensal bacteria in humans, mainly on the skin. Staphylococci are clinically classified into two groups, coagulase-positive Staphylococcus aureus and coagulase-negative staphylococci (CoNS). Staphylococcus aureus shows higher virulence than CoNS because S. aureus produces various virulence factors such as exotoxins, immune evasion factors, adhesins, and exoenzymes (Lowy, 1998; Foster, 2004). Staphylococcus aureus is associated with both human commensal and clinical infections (Lowy, 1998; Wertheim et al., 2005). Staphylococcus aureus can cause skin and soft tissue disease, pleuropulmonary disease, medical device-related bloodstream infections, food poisoning, and even infective endocarditis or osteomyelitis (Lowy, 1998; Lindsay and Holden, 2004; Tong et al., 2015; Kavanagh et al., 2018; Lakhundi and Zhang, 2018; Oliveira et al., 2018; Turner et al., 2019; Horino and Hori, 2020; Pimentel de Araujo et al., 2021). Although less virulent than S. aureus, CoNS including Staphylococcus epidermidis, Staphylococcus haemolyticus, Staphylococcus saprophyticus, Staphylococcus capitis, Staphylococcus lugdunensis, Staphylococcus hominis, Staphylococcus schleiferi, and Staphylococcus warneri are also important staphylococcal pathogen and are usually associated with hospital infections such as skin and soft tissue disease, sepsis, meningitis, endocarditis, and catheter- or implanted device-mediated infections (Vuong and Otto, 2002; Otto, 2009; Becker et al., 2014; Natsis and Cohen, 2018; Azimi et al., 2020; Parthasarathy et al., 2020).
Since the introduction of methicillin in clinical practice, methicillin-resistant S. aureus (MRSA) has evolved by acquisition of mecA coding PBP2’ and spread to worldwide (Chambers and DeLeo, 2009). The first report of MRSA was published in 1961 (Barber, 1961). In the 1980s and 1990s, hospital-acquired MRSA strains with multidrug resistance spread across the world (Crossley et al., 1979; Lowy, 1998; Chambers and DeLeo, 2009). Later, community-acquired MRSA strains, which typically cause skin and soft tissue infections in healthy patients, have been firstly reported in the 1980s (Levine et al., 1982; Saravolatz et al., 1982; Lakhundi and Zhang, 2018). In 2004, a livestock-associated MRSA strain was identified from the family of a pig farmer and their pig (Voss et al., 2005; Crespo-Piazuelo and Lawlor, 2021). The increased usage of vancomycin as an alternative to methicillin to treat MRSA infections has led to the emergence of vancomycin-intermediate S. aureus (VISA; Hiramatsu et al., 1997) and vancomycin-resistant S. aureus (VRSA) strains (Weigel et al., 2003). As with MRSA, methicillin-resistant S. epidermidis (MRSE) has also been a serious threat considering its high prevalence in some areas in the world during the 2000s (Carbon, 2000) and its recent global spread (Lee et al., 2018). In addition, the mecA gene was also identified in other CoNS including S. haemolyticus, S. hominis, S. capitis, and S. warneri (Humphries et al., 2020). Furthermore, the reduced susceptibility to glycopeptide has also been reported in S. epidermidis and S. haemolyticus (Biavasco et al., 2000).
The ever-increasing burden of global widespread multidrug-resistant bacteria has significantly challenged with the ability of available antibiotics to treat infections and prompted the discovery of novel antimicrobial compounds to overcome the shortage of therapeutic options. Antimicrobial peptides (AMPs) produced by living organisms such as humans and bacteria are candidates for promising strategies to control these superbugs. AMPs generally have a broad spectrum of activity against bacteria, viruses, fungi, and parasites, a specific mode of action, low risk of resistance development, high stability in wide ranges of pH and temperature, low toxicity to eukaryotic cells, and immunomodulatory effects (Zhang and Gallo, 2016). They exhibit antimicrobial activity via interaction with bacterial membrane, causing membrane dysfunction and disruption, disturbance of cell wall, DNA/RNA, and protein synthesis (Nguyen et al., 2011; Mahlapuu et al., 2016; Figure 1). Therefore, AMPs represent a novel alternative therapeutic for the control of critical pathogens in the future. However, clinical applications of AMPs raise several concerns such as toxicity, immunogenicity, and hemolytic activity (Moravej et al., 2018; Lei et al., 2019). As mentioned above, S. aureus and CoNS have developed resistance to many antimicrobial agents, especially methicillin, and many staphylococcal strains may be resistant to glycopeptides in the future. Therefore, the development of AMPs as new alternative antimicrobial agents against Staphylococci is of great importance. In this review, we provide an overview of human and bacterial AMPs that are effective against staphylococcal pathogens, their structures and mode of action, the current stage of investigation, and their potential as therapeutic agents in clinical treatment against staphylococcal infections.
Figure 1. The membrane-disruptive and non-membrane-disruptive antibacterial mechanisms of antimicrobial peptides (AMPs). In the membrane-disruptive mechanisms, three types of interaction can occur between the membrane and the AMPs, including: (i) barrel-stave model: the peptide monomers form a hydrophilic transmembrane channel by arranging parallelly to the phospholipids of the membrane; (ii) carpet model: the peptides solubilize the membrane into micellar structures; and (iii) toroidal model: the lipid moieties fold inward due to the cascade aggregation of peptide monomers, forming a peptide-and-lipid-lined channel.
There are various ways to classify AMPs, for example, based on their sequences, structures, or mode of action. Based on structure, AMPs can be classified into subclasses, including α-helical, β-sheet, and extended/random-coil peptides (Mahlapuu et al., 2016; Falanga and Galdiero, 2017). The first subclass, α-helical AMPs, are unstructured in aqueous environments but become amphipathic α-helical structures upon contact with biological membranes (Takahashi et al., 2010). Their main activity involves the disruption of bacterial membranes, with a broad antimicrobial spectrum including Gram-positive and Gram-negative bacteria, fungi, and parasites (Lee et al., 2013a; Kim et al., 2014; Raja et al., 2017). In contrast to α-helical peptides, β-sheet AMPs are structured in aqueous solution and do not undergo conformational changes when they interact with membranes (Lee et al., 2016). They also contain cysteine residues that form disulfide bridges, which reinforce their structure and diminish protease degradation (Lee et al., 2016). These AMPs disrupt membranes in a wide range of organisms and have become potential therapeutics as antibacterial, antiviral, antifungal, and anti-inflammatory agents (Panteleev et al., 2015). The third subclass of AMPs, extended/random-coil peptides, lack secondary structures and contain specific amino acids such as histidine (salivary histatins), proline (insect-derived pyrrhocoricin, drosocin, and apidaecin), tryptophan, and arginine (bovine lactoferrin and human lysozyme; Nguyen et al., 2011; Mahlapuu et al., 2016). These AMPs exert their antimicrobial activity, including bactericidal, fungicidal, or antiparasitic effects, through inducing membrane leakage or disturbing nucleic acid synthesis, protein production, or cell-wall synthesis by interacting with intracellular targets (Nguyen et al., 2011; Lombardi et al., 2019). The amino acid sequences of some representatives of each subclass are displayed in Figure 2.
Figure 2. Amino acid sequences of human antimicrobial peptides (AMPs). The disulfide bonds in α- and β-defensins are indicated by solid lines. The histamine residues in Histatin-1 and -3 are indicated by bold letters.
LL37, first identified as human CAP18, is a human AMP that has a linear cationic α-helical structure (Larrick et al., 1995; Vandamme et al., 2012). It has antibacterial, antifungal, and antiviral activities, promotes angiogenesis and wound healing, and mediates immunomodulatory and inflammatory responses (Bandurska et al., 2015). LL-37 was identified at various sites, including leukocytes (monocytes, neutrophils, T cells, NK cells, and B cells), epithelial cells of the testis, gastrointestinal tract, respiratory tract, and skin (Dürr et al., 2006). LL-37 can act on both Gram-positive and Gram-negative bacteria. Previous studies have reported that LL-37 has antimicrobial activity against various bacterial species: Escherichia coli (Smeianov et al., 2000; Aghazadeh et al., 2019), Klebsiella pneumoniae (Smeianov et al., 2000), Pseudomonas aeruginosa (Smeianov et al., 2000; Travis et al., 2000), Neisseria gonorrhoeae (Bergman et al., 2005), and S. aureus (Travis et al., 2000; Midorikawa et al., 2003). LL-37 exerts antibacterial activity through disruption of cell membranes and inhibition of cell wall, nucleic acid, or protein synthesis (Zanetti, 2004; Brogden, 2005). In addition, LL-37 was shown to have greater biofilm eradication capacity against S. aureus than conventional antibiotics such as gentamicin, vancomycin, rifampin, doxycycline, and cefazolin (Noore et al., 2013; Kang et al., 2019). LL-37 was able to kill S. aureus at nanomolar concentrations, while doxycycline and cefazoline acted at millimolar concentrations (Noore et al., 2013). LL-37 also inhibits the initial attachment and biofilm formation of S. epidermidis at low concentrations (Hell et al., 2010).
However, MRSA tends to have elevated resistance to LL-37 compared to MSSA (Ouhara et al., 2008). Aureolysin, a metalloproteinase produced by some S. aureus strains, was shown to cleave and inactivate LL-37—the more aureolysin produced by an S. aureus strain, the less susceptibility of this strain to the antimicrobial fragment LL-17-37 (Sieprawska-Lupa et al., 2004). LL-37 derivatives have been investigated to improve the quality of LL-37 in terms of their stability, hemolysis and cytotoxicity, cell selectivity, and biofilm eradication (Chen et al., 2021; Ridyard and Overhage, 2021). D-LL-37, an LL-37 derivative in which all amino acids are changed to the D-form, displayed protease-resistance properties while possessing biofilm-inhibition capacity equal to the L-peptide isomer LL-37 (inhibiting ~40% biofilm formation at a concentration of 10 μg/ml) and immunostimulatory activity and wound-healing properties on the host higher than the L-peptide (Dean et al., 2011). SK-24, which corresponds to residues 9–32 of LL-37, demonstrated killing activity against S. aureus and Staphylococcal biofilm reduction, which was superior to LL-37 and several other derivatives (Zhang et al., 2021). KE-18, a derivative corresponding to residues 15–32 of LL-37, showed significant biofilm prevention against S. aureus (Luo et al., 2017). KR-12-a5 is another LL-37-derived peptide, a KR-12 analogue corresponding to residues 18–29, which exhibits higher antimicrobial activities against MRSA than LL-37 (Kim et al., 2017).
Defensins are AMPs that belong to the β-sheet subclass and carry six disulfide-linked cysteine residues and 4–10 arginine residues per molecule (Ganz et al., 1985). Defensins have been widely discovered from plants, insects, and mammals, with broad antimicrobial activity against Gram-positive and Gram-negative bacteria, enveloped- and non-enveloped viruses, and yeast and filamentous-phase fungi (Ganz et al., 1990; Gao et al., 2021). Various functions of defensins have been investigated, including pore formation, neutralization or inactivation of secreted toxins, modulation of the immune system and enhancement of antibacterial effects, and induction of cytokine and chemokine expression to fight against bacteria (Gao et al., 2021).
Two main defensin subfamilies, α- and β-defensins, have been reported in humans and some other mammals (Ganz, 2003), while another subfamily, θ-defensins, was later identified in non-human primates such as rhesus macaque monkeys or baboons (Tang et al., 1999; Garcia et al., 2008). Alpha-defensins differ from beta-defensins by the length of peptide chains between the six cysteine residues and the connecting patterns of the cysteine pairs to form disulfide bonds (Ganz, 2003). Human α-defensins have been identified from human neutrophils, gastrointestinal tract, and epithelial tissues, and β-defensins have been identified from neutrophils, leukocytes, epithelial cells, blood plasma, and urine (Schneider et al., 2005; Gao et al., 2021). To date, six human α-defensins have been reported, including human neutrophil peptides 1–4 (HNPs1–4) and human enteric defensins 5–6 (HD5 and HD6; Gao et al., 2021). Among HNPs1–4, HNP2 demonstrated powerful antibacterial activity against S. aureus, surpassing the other three HNPs (Ericksen et al., 2005). HD5 and HNP2 exhibited comparable activity against S. aureus, while HD6 did not exhibit antibacterial activity (Ericksen et al., 2005).
More than 30 human β-defensin genes have been discovered; however, only a few have been intensively investigated (Schutte et al., 2002; Vankeerberghen et al., 2005). They were identified in various organs and solutions inside the human body, for example, in the hemodialysis solution from patients with renal failure, gastrointestinal tract, urogenital tract, respiratory tract, oral cavity, oral epithelium, damaged psoriasis skin, skin, tonsil, testicles, and antrum (Gao et al., 2021). Among the four primarily studied human β-defensins (hBD-1–4), hBD-3 exhibited antibacterial activity against S. pyogenes and S. aureus, including multidrug-resistant S. aureus, through the cell wall perforation effect (Harder et al., 2001; Schneider et al., 2005). Additionally, hBD-3 has been reported to effectively eliminate staphylococci biofilms, even with MRSA and MRSE, and was significantly more effective than clindamycin (Huang et al., 2012; Lee et al., 2013b). At low concentrations (4–8 μg/ml), hBD-3 effectively restricted bacterial adhesion after 6 h and biofilm formation after 12 h against MRSA and MRSE (Zhu et al., 2013). However, MRSA was reported to have higher resistance to hBD-3 than MSSA, with 55% of the tested MRSA strains exhibiting greater than 20% survival under treatment with 1 μg/ml hBD-3 compared to 13% of the tested MSSA strains (Midorikawa et al., 2003). Nevertheless, combinations of defensins with methicillin or β-defensins and CAP18 can have a synergistic effect on S. aureus, including MRSA (Midorikawa et al., 2003). H4, a chimeric human defensin that combines the sequences of hBD-3 and hBD-4, showed superior antibacterial activity against S. aureus compared with that of hBD-3 and hBD-4 and conferred high salt tolerance (Yu et al., 2021). hBTD-1 and [D]hBTD-1, chimeric analogues of human β-defensin 1 and θ-defensin, respectively, exhibited considerable activity against S. aureus biofilms as well as planktonic forms (Mathew et al., 2017).
Histatins are histidine-rich proteins that are secreted by human parotid and submandibular glands into the salivary glands (Oppenheim et al., 1988). They were shown to have a broad spectrum of antibacterial and antifungal activities (Tsai and Bobek, 1998; Rothstein et al., 2001; Sajjan et al., 2001). Histatin 5 (Hst5) was shown to kill 60–70% of S. aureus in 10–100 mM sodium phosphate buffer (NaPB) but had limited activity against S. aureus biofilms (Du et al., 2017). Hst 5 may attack S. aureus through multiple targets and energy-independent mechanisms (Du et al., 2017).
P-113, a histatin derivative in which residues 4–15 are the same as those of histatin 5, showed a high bactericidal effect on MRSA (Sajjan et al., 2001; Giacometti et al., 2005). Compared to histatin 5, several synthetic histatin analogues, e.g., dhvar 1 and dhvar2, demonstrated increased antibacterial activity against MRSA (Helmerhorst et al., 1997). At a concentration of 2 mg/ml, dhvar1, dhvar4, and dhvar5 exhibited an antibacterial effect against S. epidermidis (Elving et al., 2000).
Bacteriocins are ribosomally synthesized AMPs produced by bacteria and have been classified based on the producer organism, inhibitory spectrum, molecular size, chemical structure, mode of action, or plasmid nature (Jack et al., 1995; Ennahar et al., 2000; Oscáriz and Pisabarro, 2001; Kumariya et al., 2019). Various classification schemes for bacteriocins have been proposed over the years. In this review, we adopt an updated classification by Soltani et al. (2021). The classification of bacteriocins is presented in Table 1. The bacteriocins from Gram-positive and Gram-negative bacteria were classified into two classes, namely class I and class II. Class I bacteriocins are ribosomally synthesized and posttranslationally modified peptides (RiPPs; Arnison et al., 2013) with molecular masses <5 kDa. Class I bacteriocins are further subdivided based on their modifications. They can be divided into lantibiotics, sactibiotics, linaridins, thiopeptides, glycocins, circular peptides, and bottromycins from Gram-positive bacteria; nucleotide peptides and siderophore peptides from Gram-negative bacteria; linear azol(in)e-containing peptides (LAPs) and lasso peptides from both Gram-positive and Gram-negative bacteria; and cyanobactins produced by cyanobacteria. Among these, lantibiotics have been widely investigated for therapeutic applications (Soltani et al., 2021; Fernandes and Jobby, 2022). Lantibiotics usually contain 19–38 amino acids that carry unusual amino acid residues, namely lanthionine, β-methyllanthionine, and dehydrated amino acids (Willey and van der Donk, 2007; Arnison et al., 2013). Lanthionine and β-methyllanthionine are conducted from dehydration of serine and threonine, yielding di-dehydroalanine and di-dehydrobutyrine residues, respectively, followed by forming thioether linkages for stabilization. Other class I-bacteriocins have their characteristic modification, such as sactibiotics with sulfur to α-carbon linkage, glycocins with glucosylated cysteine, thiopeptides with a 6-membered nitrogen-containing ring, bottromycins with methylated amino acids, and C-terminal decarboxylated thiazole (Table 1; Figure 3). Class II bacteriocins consist of unmodified peptides, which are further divided into three subclasses: pediocin-like single peptides, unmodified single peptides, and two-peptide bacteriocins.
Nisin is a 34-amino acid lantibiotic produced by Gram-positive bacteria, including Lactococcus, Staphylococcus, and Streptococcus species (Gross and Morell, 1971; O’Connor et al., 2015; O’Sullivan et al., 2020; Figure 3). To date, several variants of nisin have been reported, for example, nisin A (Gross and Morell, 1971), nisin Z (Mulders et al., 1991), nisin Q (Zendo et al., 2003), nisin U (Wirawan et al., 2006), nisin F (de Kwaadsteniet et al., 2008), nisin H (O’Connor et al., 2015), nisin O (Hatziioanou et al., 2017), nisin J (O’Sullivan et al., 2020), and nisin P (Garcia-Gutierrez et al., 2020). Nisin has an antibacterial effect against a wide spectrum of Gram-positive bacteria, including staphylococci, streptococci, enterococci, bacilli, and listeria. Nisin binds to lipid II, which is a membrane component required for peptidoglycan biosynthesis, and then permeabilizes the cell membrane and inhibits cell wall synthesis (Breukink and de Kruijff, 1999; Lubelski et al., 2008). Additionally, nisin was shown to cause cell shrinkage and chromosomal DNA condensation in a MRSA model, suggesting that nisin interferes with DNA replication or segregation in the bacteria (Jensen et al., 2020).
Nisin A exhibited high antibacterial activity against both planktonic and biofilm S. aureus cells (Okuda et al., 2013). The combination of nisin A and vancomycin was reported to effectively inhibit S. aureus biofilm formation and reduce the thickness of preformed biofilms produced by multidrug-resistant S. aureus (Angelopoulou et al., 2020). Nisin A and nisin Z differ by a single substitution at the 27th amino acid residue, with a histidine in nisin A and an asparagine in nisin Z. The structural alteration gives nisin Z a higher solubility and diffusion ability while maintaining its antimicrobial activity compared to nisin A, providing an advantage for nisin Z in the food preservation industry (de Vos et al., 1993; Laridi et al., 2003). The combination of nisin Z (1 μg/ml) and methicillin (32 μg/ml) significantly reduced the growth of MRSA (3.1 log reduction after 3 h of treatment; Ellis et al., 2019). Nisin U, a variant produced by Streptococcus uberis, exhibited an inhibitory effect against some staphylococci, such as Staphylococcus simulans and Staphylococcus cohnii, but not against S. aureus (Wirawan et al., 2006). Nisin F is a variant produced by L. lactis subsp. lactis and showed antimicrobial activity against Staphylococcus carnosus and S. aureus (de Kwaadsteniet et al., 2008). Nisin J, a nisin variant produced by S. capitis, demonstrated high antimicrobial activity against Staphylococcus species, including MRSA (O’Sullivan et al., 2020). Nisin H, a bacteriocin produced by Streptococcus hyointestinalis and conferring an intermediate structure between lactococcal nisin A and streptococcal nisin U, also exhibited a bactericidal effect against S. aureus (O’Connor et al., 2015). Nisin P was produced by Streptococcus agalactiae and showed antibacterial activity against staphylococci, but the effect was not as high as nisin A and H (Garcia-Gutierrez et al., 2020). Ripcin B–G, a synthetic peptide generated by the fusion of ripcin and the C-terminal end of nisin (1–20), exhibited stronger and selective bactericidal activity against S. aureus, including MRSA (Zhao and Kuipers, 2021). Nisin encapsulated in nanofibers made of polyvinyl alcohol, wheat gluten, and zirconia exhibited well-controlled release and high inhibition activity against S. aureus (H. Wang et al., 2015). Nisin-biogel, a delivery system for nisin based on guar gum gel, has been developed and displayed antimicrobial activity against S. aureus (Jesus et al., 2021). At subinhibitory levels, it suppressed some virulence factors, such as the factors related to biofilm formation, coagulase, and protein A; however, the expression of some other virulence genes, such as spA (staphylococcal protein A), coa (coagulase), icaA (intracellular adhesin A), and icaD (intracellular adhesin D), was elevated, requiring a thorough consideration of the optimal dosage when applying nisin in clinical practice (Jesus et al., 2021).
Epidermin is a 21-amino acid lantibiotic produced by S. epidermidis that exhibits an antimicrobial effect against staphylococci and streptococci (Schnell et al., 1988; Figure 4). Epidermin kills bacteria by inhibiting cell wall synthesis by interacting with the cell wall precursor lipid II and sometimes by causing pore formation (Bonelli et al., 2006).
Epidermin significantly reduced the S. epidermidis cells attached to silicone catheters in an in vitro catheter colonization model (Fontana et al., 2006). Epidermin exhibited antibacterial activity against >85% of tested S. aureus (165 strains) involved in bovine mastitis (Varella Coelho et al., 2007). In another study, epidermin showed antibacterial activity against 81.3% of tested S. aureus involved in human infections, including MRSA endemic clones in Brazil (Nascimento et al., 2006). Epidermin also exhibited antibacterial effects against S. haemolyticus, S. capitis, S. simulans, S. saprophyticus, S. hominis, and S. epidermidis, although no activity was observed against some tested S. aureus (Nakazono et al., 2022).
Nukacin is a type A(II) lantibiotic that was first identified in 1998 from S. warneri ISK-1 (Kimura et al., 1998). This peptide consists of 27 amino acid residues and arrests cell wall biosynthesis by binding to lipid II (Islam et al., 2012; Figure 4). Nukacin ISK-1 showed a bacteriostatic effect against Bacillus subtilis by stopping cell growth without pore formation (Asaduzzaman et al., 2009) while exhibiting bactericidal activity against Micrococcus luteus and S. simulans via pore formation and cell lysis (Roy et al., 2014). Several variants of nukacin have been reported, including nukacin KQU-131 produced by S. hominis (Wilaipun et al., 2008), nukacin 3299 produced by S. simulans (Ceotto et al., 2010), and nukacin IVK45 and nukacin KSE650 produced by S. epidermidis (Janek et al., 2016; Nakazono et al., 2022). Nukacin ISK-1 exerted a bacteriostatic effect against MRSA planktonic cells; however, activity against biofilm cells was not observed (Okuda et al., 2013). Nukacin 3299 exhibited antibacterial activity against 66.7% (18/27) of S. aureus strains involved in bovine mastitis (Ceotto et al., 2010). Nukacin KSE650 showed antibacterial activity against S. haemolyticus, S. capitis, S. simulans, S. saprophyticus, S. hominis, and S. epidermidis, although no activity was observed against some tested S. aureus, while Nukacin ISK-1 showed an antibacterial effect against S. aureus (Nakazono et al., 2022). The difference of four amino acid residues between Nukacin ISK1 and Nukacin KSE650 mature peptide caused different susceptibility against S. aureus strains.
Mutacins are bacteriocins produced by Streptococcus mutans, which have been classified into two types: lantibiotics containing unusual amino acid residues and non-lantibiotics consisting of unmodified peptides (Merritt and Qi, 2012). The lantibiotic mutacins include mutacin I, II, III/1140, B-Ny266 (Figure 4), Smb, and K8 and usually confer a wider spectrum of activity. In contrast, non-lantibiotics, such as mutacin IV, V, VI, and N, play an important role at the S. mutans intraspecies level and in closely related species (Merritt and Qi, 2012). Lantibiotic mutacins were reported to have bactericidal activity through pore formation and inhibiting cell wall synthesis (Hasper et al., 2006). Against S. aureus, mutacin III exhibited the highest antibacterial activity; mutacin I, IIIb (mutacin B-Ny266), and IVb showed intermediate activity, while mutacin II, IV, K8, and Smb had almost no effect (Watanabe et al., 2021).
Garvicin KS is a leaderless multipeptide bacteriocin produced by Lactococcus garvieae (Thapa et al., 2020). Garvicin KS showed bactericidal activity against 50/53 tested S. aureus strains (Chi and Holo, 2018). Against the least sensitive strain, the combination of nisin and garvicin KS showed a synergetic effect by completely killing the bacteria after 12 h. The combination of farnesol and garvicin KS was not effective; however, combinations of farnesol and nisin or the three compounds rapidly eradicated S. aureus (Chi and Holo, 2018). Garvicin KS and micrococcin P1 displayed a synergetic effect against S. aureus biofilms, including MRSA (Kranjec et al., 2020). Pep5 is a 34-amino acid residue lantibiotic produced by S. epidermidis (Kaletta et al., 1989). Pep5 exhibited inhibitory activity against 77.2 and 87.5% of the tested coagulase-negative staphylococci and S. aureus, respectively (Nascimento et al., 2006).
To evaluate the effect of AMPs including human AMPs and bacteriocins on S. aureus and the host, in vivo experiments using S. aureus-infected animal models were conducted. In a skin infection murine model, garvicin KS and micrococcin P1 displayed synergistic effects against MRSA (Ovchinnikov et al., 2020). In a rat S. aureus-induced uterine endometritis model, nisin (25 mg/kg) administration significantly restored the inflammation of the endometrium and improved the expression of several serum cytokines, which showed high expression in the endometritis (Jia et al., 2019). Nisin-eluting nanofibers were also shown to be effective against skin infection by MRSA in mice (Heunis et al., 2013). Mersacidin, a lantibiotic produced by Bacillus sp., was shown to eradicate the colonization of human-derived MRSA in a mouse rhinitis model (Kruszewska et al., 2004). Sublancin, a glycosylated AMP produced by B. subtilis, exhibited antibacterial and immunomodulatory effects against a MRSA-infected mouse model that induced intraperitoneal (Wang et al., 2017; Li et al., 2021) or intestinal injury (Wang et al., 2014). K2A and R13A, two analogues of mutacin 1,140, have been reported to improve pharmacokinetics in vivo and efficiently rescue mice infected with MRSA at 10 mg/kg (100% protection) or 2.5 mg/kg (50% protection; Geng et al., 2018). In an intraperitoneal infection mouse model, mutacin B-Ny266 effectively protected the mice from mortality induced by S. aureus. At a dose <1 mg/kg, mutacin B-Ny266 showed a comparable ED50 (effective dose protecting 50% of the animals) to that of vancomycin (Mota-Meira et al., 2005). Epidermicin NI01, a synthetic AMP, effectively protected Galleria mellonella larvae from MRSA infection without signs of toxicity or stimulating the host immune system (Gibreel and Upton, 2013).
Regarding human AMPs, many experiments to show the effect of AMPs, especially LL-37 and beta-defensin, on various S. aureus infection animal model have been reported. The application of LL-37 promoted tissue regeneration including re-epithelialization and angiogenesis in MRSA-infected surgical wounds (Simonetti et al., 2021). Hybrid peptide CaD23 consisted of LL-37 and human beta-defensin-2 showed a good efficiency with the reduction of S. aureus cells by 94% in a murine keratitis model (Ting et al., 2021). The LL 37 derivatives, 17tF-W, eliminated MRSA USA300 cells in catheter and its surrounding tissues of a murine infection model (Narayana et al., 2019). Both LL-37 and IDR-1 (an innate defense regulator peptide) exhibited immunomodulation effects and restored pulmonary function in mice with MRSA pneumonia (Sun et al., 2013). Histatin 5, Dh5 (residues 11–24 of histatin), P-113, Dhvar4 (an increased amphipathicity variant from Dh5), and Dhvar5 (a reduced amphipathicity variant from Dh5) showed bactericidal effects against S. aureus, including MRSA, in vivo (Welling et al., 2007). In an in vivo MRSA osteomyelitis prevention model, 24 mg Dhvar-5 beads showed a significant reduction in bacterial load inoculated in rabbit femora compared to the control; however, complete sterilization of the femora could not be observed (Faber et al., 2004). 99mTc-HBD-3, a human β-defensin 3 radiolabeled with 99mTc, demonstrated favorable uptake of AMPs at the infected site in an S. aureus-infected rat model (Follacchio et al., 2019). HDMP, a human defensin-6 mimic peptide, significantly rescued mice with MRSA bacteremia at a survival rate as high as 100%, which was higher than that of vancomycin (83.3%) at the same dosage (5 mg/kg; Fan et al., 2020).
The promising results of AMP activity observed in preclinical studies have led to investigations in human clinical trials to evaluate their safety and effectiveness. Clinical trials targeting S. aureus infections have been carried out (Table 2). Compared to placebo, topical LL-37 treatment significantly improved the healing rate of hard-to-heal venous leg ulcers, without any local or systemic safety concerns (Grönberg et al., 2014). Intranasal treatment with LTX-109, a chemically synthesized, peptide-mimetic drug, was reported to effectively eradicate the persistent colonization of MRSA and MSSA in the nasal cavity without the signs of adverse effects (Nilsson et al., 2015). PLG0206, an engineered AMP, efficaciously reduced the bacterial count, including S. epidermidis and S. aureus, in patients with chronic periprosthetic joint infections (Huang et al., 2021). GSK132232, a synthetic AMP, rapidly decreased lesion size and pain in acute bacterial skin and skin structure infections, although several mild-to-moderate adverse effects were observed, including nausea, vomiting, diarrhea, and headache (Corey et al., 2014). Numerous AMPs have undergone clinical trials; however, only a few AMPs are currently approved for clinical application, including nisin, gramicidin, polymyxins, daptomycin, and melittin (Dijksteel et al., 2021).
Table 2. Some antimicrobial peptides (AMPs) under investigation and clinical phase of development for treatment of Staphylococcal infections.
Although AMPs represent potential alternative clinical antimicrobials, continuous exposure to AMPs could lead to the development of resistance in formerly susceptible cells. Bacteria can escape bacteriocins through acquired resistance and innate resistance involved in cell wall synthesis, cytoplasmic membrane synthesis, cell envelope alterations, membrane permeability and specific receptor expression, or energy metabolism and transport (de Freire Bastos et al., 2015; Andersson et al., 2016; Soltani et al., 2021). In S. aureus, several factors were reported to be involved in resistance to AMPs (Figures 5, 6). The disruption of either Dlt or MprF results in elevated sensitivity to defensins (Peschel et al., 1999, 2001; Kawada-Matsuo et al., 2013). Dlt is associated with the addition of alanine to teichoic acids on the cell wall, while MprF is associated with the addition of lysine to phosphatidylglycerol in the cell membrane (Figure 5). Amino acid incorporation causes a shift to a weak negative charge on cell surfaces (Peschel et al., 1999, 2001). A transporter of VraDE regulated by one two-component system, BraRS, is associated with resistance to nisin A, Nukacin ISK-1, and bacitracin (Kawada-Matsuo et al., 2013). In BraRS (NsaRS)/BraDE system, sensing of nisin A by BraDE results in the autophosphorylation of BraS, followed by the phosphorylation of BraR (Hiron et al., 2011; Randall et al., 2018). The phosphorylated BraR can bind to the upstream region of vraDE, giving rise to the increased expression of vraDE (Figure 5). The transporter PmtA-D regulated by PmtR is associated with resistance to beta-defensin 3 and nisin A (Cheung et al., 2018). Another factor, staphylokinase, was also involved in alpha-defensins resistance because staphylokinase directly binds to alpha-defensin, causing the neutralization of its activity (Bokarewa et al., 2006). These factors are generally conserved among S. aureus strains. Therefore, S. aureus has a natural resistant system against several bacteriocins, although S. aureus retained sensitivity when treated with high concentrations of AMPs.
Figure 5. Examples of bacteriocin resistance mechanisms in Staphylococcus aureus. I. In the ApsRS system, the sensing of cationic antimicrobial peptides (AMPs) results in the autophosphorylation of ApsS, followed by the phosphorylation of ApsR. The phosphorylated ApsR can bind to the upstream regions of mprF and dltABCD, increasing the expression of these factors. MprF is associated with the addition of lysine to phosphatidylglycerol in the cell membrane, and DltABCD is associated with the addition of alanine to teichoic acids on the cell wall. Amino acid incorporation causes a shift to a weak negative charge on the cell surfaces and makes the cell less sensitive to cationic AMPs. II. In the BraRS (NsaRS)/BraDE system, sensing of nisin A by BraDE results in the autophosphorylation of BraS, followed by the phosphorylation of BraR. The phosphorylated BraR can bind to the upstream region of vraDE, giving rise to the increased expression of an ABC transporter VraDE which expels the AMPs from the cell.
Figure 6. Schematic diagram of the nisin A highly resistant mechanism. I. A point mutation in the promoter region results in the higher expression of braXRS. This leads to the increased induction of vraDE expression by nisin A. II. A point mutation in braS (encoding a sensor protein) causes nisin A-independent phosphorylation of BraS, resulting in increased phosphorylated BraR, which induces a constant expression of vraDE. III. A point mutation in braR (encoding a response regulator) results in nisin A-independent activation of vraDE expression. IV. A point mutation in pmtR encoding a negative regulator PmtR for pmtABCD expression. Mutated PmtR, which lacks the DNA binding ability, results in a constant pmtABCD expression.
It was questionable whether highly resistance could evolve if exposed to a high concentration of nisin A. After several experimental trials, finally, some research groups were able to obtain highly nisin A-resistant S. aureus strains with mutations in BraRS, or PmtR when exposing S. aureus to sub-MICs of nisin A (Blake et al., 2011; Arii et al., 2019; Kawada-Matsuo et al., 2020, 2021). Arii et al. (2019) isolated several nisin A highly resistant S. aureus strains by exposing sub-MIC of nisin A three times and obtained two types of the mutants, with mutations in braRS or pmtR (Figure 6). The mutants with braRS mutation showed higher expression of VraDE, which is an effector for nisin A resistance, than that of the wildtype, while the mutants with pmtR mutation did not show high expression of VraDE. Three mutants with braRS mutation had different point mutation sites, including the upstream region of braXRS, braR, or braS. The point mutation upstream of the braXRS region was associated with the increased promoter activity, causing high expression of braRS. By the point mutation of braR, the mutated BraR without phosphorylation was able to bind to the upstream region of braRS. The braS mutation was found in the histidine kinase region, suggesting that the mutated BraS is autophosphorylated without the stimulation of nisin A. Blake et al. also reported the point mutation of NsaS (BraS) in nisin-resistant S. aureus strains. Another nisin A highly resistant S. aureus mutant was isolated by the mutation of PmtR with the increased expression of PmtA-D, an ABC transporter, involved in the susceptibility to nisin A and beta-defensin (Kawada-Matsuo et al., 2020, 2021). Since PmtR was a negative regulator for PmtA-D expression, the mutated PmtR could not bind to the upstream region of pmtA-D. In addition, this mutant also exhibited increased virulence in a mouse bacteremia model. Dobson et al. (2014) reported that AMP-resistant S. aureus strains selected by pexiganan, iseganan, or melittin showed higher survival in Tenebrio molitor, an insect model. Therefore, considerations should be taken into account to overcome the emergence of bacteriocin-resistant bacteria. The combination of bacteriocins with different modes of action or the combination of conventional antibiotics and bacteriocins may allow a reduction in dosage and avoid the development of bacteriocin resistance (de Freire Bastos et al., 2015).
Staphylococci including S. aureus and CoNS are important human pathogens associated with potentially life-threatening infections. The emergence of drug-resistant Staphylococci has significantly challenged the available treatment options, necessitating the discovery of novel therapeutics. AMPs exhibit excellent promise as alternatives to conventional antibiotics due to their broad-spectrum activity; rapid mode of action; low risk of resistance development and anti-inflammatory and immunomodulatory effects; synergistic effects with conventional antibiotics; and clinical efficacy against some multidrug-resistant bacteria. This review discusses potential AMPs, focusing on human AMPs and bacteriocins, which display antibacterial activity against Staphylococci, including methicillin-resistant staphylococci, in vitro and in some infection models, and presents the current clinical investigation phase of some AMPs. The production costs, cytotoxic effects, reduced efficacy in the body (low stability, high susceptibility to proteolysis, reduced activity in physiological conditions), and resistance development are the major obstacles that challenge the clinical usage of AMPs (Pachón-Ibáñez et al., 2017; Dijksteel et al., 2021). The incorporation of AMPs into artificial materials, the development of innovative formulation or delivery systems, and the combination with conventional antibiotics may provide effective strategies to overcome the disadvantages of AMPs and promote their market authorization as novel AMP-based drugs.
ML, MK-M, and HK conceptualized and revised the manuscript. ML drafted the manuscript and tables and produced the figures under the guidance of HK. MK-M acquired the fund for the project. All authors contributed to the article and approved the submitted version.
This research was funded by Grant-in-Aid for Scientific Research (C) (grant no: 21K09858) from the Ministry of Education, Culture, Sports, Sciences, and Technology of Japan.
The authors declare that the research was conducted in the absence of any commercial or financial relationships that could be construed as a potential conflict of interest.
All claims expressed in this article are solely those of the authors and do not necessarily represent those of their affiliated organizations, or those of the publisher, the editors and the reviewers. Any product that may be evaluated in this article, or claim that may be made by its manufacturer, is not guaranteed or endorsed by the publisher.
Aghazadeh, H., Memariani, H., Ranjbar, R., and Pooshang Bagheri, K. (2019). The activity and action mechanism of novel short selective LL-37-derived anticancer peptides against clinical isolates of Escherichia coli. Chem. Biol. Drug Des. 93, 75–83. doi: 10.1111/CBDD.13381
Andersson, D. I., Hughes, D., and Kubicek-Sutherland, J. Z. (2016). Mechanisms and consequences of bacterial resistance to antimicrobial peptides. Drug Resist. Updat. 26, 43–57. doi: 10.1016/J.DRUP.2016.04.002
Angelopoulou, A., Field, D., Pérez-Ibarreche, M., Warda, A. K., Hill, C., and Paul Ross, R. (2020). Vancomycin and nisin A are effective against biofilms of multi-drug resistant Staphylococcus aureus isolates from human milk. PLoS One 15:e0233284. doi: 10.1371/JOURNAL.PONE.0233284
Arii, K., Kawada-Matsuo, M., Oogai, Y., Noguchi, K., and Komatsuzawa, H. (2019). Single mutations in BraRS confer high resistance against nisin A in Staphylococcus aureus. Microbiologyopen 8:e791. doi: 10.1002/MBO3.791
Arnison, P. G., Bibb, M. J., Bierbaum, G., Bowers, A. A., Bugni, T. S., Bulaj, G., et al. (2013). Ribosomally synthesized and post-translationally modified peptide natural products: overview and recommendations for a universal nomenclature. Nat. Prod. Rep. 30, 108–160. doi: 10.1039/c2np20085f
Asaduzzaman, S. M., Nagao, J. I., Iida, H., Zendo, T., Nakayama, J., and Sonomoto, K. (2009). Nukacin ISK-1, a bacteriostatic lantibiotic. Antimicrob. Agents Chemother. 53, 3595–3598. doi: 10.1128/AAC.01623-08
Azimi, T., Mirzadeh, M., Sabour, S., Nasser, A., Fallah, F., and Pourmand, M. R. (2020). Coagulase-negative staphylococci (CoNS) meningitis: a narrative review of the literature from 2000 to 2020. New Microbes New Infect. 37:100755. doi: 10.1016/J.NMNI.2020.100755
Bandurska, K., Berdowska, A., Barczyńska-Felusiak, R., and Krupa, P. (2015). Unique features of human cathelicidin LL-37. Biofactors 41, 289–300. doi: 10.1002/BIOF.1225
Barber, M. (1961). Methicillin-resistant staphylococci. J. Clin. Pathol. 14, 385–393. doi: 10.1136/JCP.14.4.385
Becker, K., Heilmann, C., and Peters, G. (2014). Coagulase-negative staphylococci. Clin. Microbiol. Rev. 27, 870–926. doi: 10.1128/CMR.00109-13
Bergman, P., Johansson, L., Asp, V., Plant, L., Gudmundsson, G. H., Jonsson, A. B., et al. (2005). Neisseria gonorrhoeae downregulates expression of the human antimicrobial peptide LL-37. Cell. Microbiol. 7, 1009–1017. doi: 10.1111/J.1462-5822.2005.00530.X
Biavasco, F., Vignaroli, C., and Varaldo, P. E. (2000). Glycopeptide resistance in coagulase-negative staphylococci. Eur. J. Clin. Microbiol. Infect. Dis. 19, 403–417. doi: 10.1007/S100960000299
Blake, K. L., Randall, C. P., and O’Neill, A. J. (2011). In vitro studies indicate a high resistance potential for the lantibiotic nisin in Staphylococcus aureus and define a genetic basis for nisin resistance. Antimicrob. Agents Chemother. 55, 2362–2368. doi: 10.1128/AAC.01077-10
Bokarewa, M. I., Jin, T., and Tarkowski, A. (2006). Staphylococcus aureus: Staphylokinase. Int. J. Biochem. Cell Biol. 38, 504–509. doi: 10.1016/J.BIOCEL.2005.07.005
Bonelli, R. R., Schneider, T., Sahl, H. G., and Wiedemann, I. (2006). Insights into in vivo activities of lantibiotics from gallidermin and epidermin mode-of-action studies. Antimicrob. Agents Chemother. 50, 1449–1457. doi: 10.1128/AAC.50.4.1449-1457.2006
Breukink, E., and de Kruijff, B. (1999). The lantibiotic nisin, a special case or not? Biochim. Biophys. Acta 1462, 223–234. doi: 10.1016/S0005-2736(99)00208-4
Brogden, K. A. (2005). Antimicrobial peptides: pore formers or metabolic inhibitors in bacteria? Nat. Rev. Microbiol. 3, 238–250. doi: 10.1038/nrmicro1098
Carbon, C. (2000). MRSA and MRSE: is there an answer? Clin. Microbiol. Infect. 6(SUPPL. 2), 17–22. doi: 10.1046/J.1469-0691.2000.00005.X
Ceotto, H., Holo, H., da Costa, K. F. S., Nascimento, J. S., Salehian, Z., Nes, I. F., et al. (2010). Nukacin 3299, a lantibiotic produced by Staphylococcus simulans 3299 identical to nukacin ISK-1. Vet. Microbiol. 146, 124–131. doi: 10.1016/J.VETMIC.2010.04.032
Chambers, H. F., and DeLeo, F. R. (2009). Waves of resistance: Staphylococcus aureus in the antibiotic era. Nat. Rev. Microbiol. 7, 629–641. doi: 10.1038/NRMICRO2200
Chen, K., Gong, W., Huang, J., Yoshimura, T., and Wang, J. M. (2021). The potentials of short fragments of human anti-microbial peptide LL-37 as a novel therapeutic modality for diseases. Front. Biosci. 26, 1362–1372. doi: 10.52586/5029
Cheung, G. Y. C., Fisher, E. L., McCausland, J. W., Choi, J., Collins, J. W. M., Dickey, S. W., et al. (2018). Antimicrobial peptide resistance mechanism contributes to Staphylococcus aureus infection. J. Infect. Dis. 217, 1153–1159. doi: 10.1093/INFDIS/JIY024
Chi, H., and Holo, H. (2018). Synergistic antimicrobial activity between the broad spectrum bacteriocin garvicin KS and nisin, farnesol and polymyxin B against gram-positive and gram-negative bacteria. Curr. Microbiol. 75, 272–277. doi: 10.1007/S00284-017-1375-Y
Corey, R., Naderer, O. J., O’Riordan, W. D., Dumont, E., Jones, L. S., Kurtinecz, M., et al. (2014). Safety, tolerability, and efficacy of GSK1322322 in the treatment of acute bacterial skin and skin structure infections. Antimicrob. Agents Chemother. 58, 6518–6527. doi: 10.1128/AAC.03360-14/ASSET/A1E9A65E-F743-491D-AAA9-645769B54F21/ASSETS/GRAPHIC/ZAC0111433750004.JPEG
Crespo-Piazuelo, D., and Lawlor, P. G. (2021). Livestock-associated methicillin-resistant Staphylococcus aureus (LA-MRSA) prevalence in humans in close contact with animals and measures to reduce on-farm colonisation. Ir. Vet. J. 74:21. doi: 10.1186/S13620-021-00200-7
Crossley, K., Landesman, B., and Zaske, D. (1979). An outbreak of infections caused by strains of Staphylococcus aureus resistant to methicillin and aminoglycosides. II. Epidemiologic studies. J. Infect. Dis. 139, 280–287. doi: 10.1093/INFDIS/139.3.280
de Freire Bastos, M. D. C., Varella Coelho, M. L., and da Silva Santos, O. C. (2015). Resistance to bacteriocins produced by gram-positive bacteria. Microbiology 161, 683–700. doi: 10.1099/MIC.0.082289-0/CITE/REFWORKS
de Kwaadsteniet, M., ten Doeschate, K., and Dicks, L. M. T. (2008). Characterization of the structural gene encoding nisin F, a new lantibiotic produced by a Lactococcus lactis subsp. lactis isolate from freshwater catfish (Clarias gariepinus). Appl. Environ. Microbiol. 74, 547–549. doi: 10.1128/AEM.01862-07
de Vos, W. M., Mulders, J. W. M., Siezen, R. J., Hugenholtz, J., and Kuipers, O. P. (1993). Properties of nisin Z and distribution of its gene, nisZ, in Lactococcus lactis. Appl. Environ. Microbiol. 59, 213–218. doi: 10.1128/AEM.59.1.213-218.1993
Dean, S. N., Bishop, B. M., and van Hoek, M. L. (2011). Natural and synthetic cathelicidin peptides with anti-microbial and anti-biofilm activity against Staphylococcus aureus. BMC Microbiol. 11:114. doi: 10.1186/1471-2180-11-114
Dijksteel, G. S., Ulrich, M. M. W., Middelkoop, E., and Boekema, B. K. H. L. (2021). Review: lessons learned from clinical trials using antimicrobial peptides (AMPs). Front. Microbiol. 12:616979. doi: 10.3389/fmicb.2021.616979
Dobson, A. J., Purves, J., and Rolff, J. (2014). Increased survival of experimentally evolved antimicrobial peptide-resistant Staphylococcus aureus in an animal host. Evol. Appl. 7, 905–912. doi: 10.1111/EVA.12184
Du, H., Puri, S., McCall, A., Norris, H. L., Russo, T., and Edgerton, M. (2017). Human salivary protein Histatin 5 has potent bactericidal activity against ESKAPE pathogens. Front. Cell. Infect. Microbiol. 7:41. doi: 10.3389/FCIMB.2017.00041
Dürr, U. H. N., Sudheendra, U. S., and Ramamoorthy, A. (2006). LL-37, the only human member of the cathelicidin family of antimicrobial peptides. Biochim. Biophys. Acta 1758, 1408–1425. doi: 10.1016/J.BBAMEM.2006.03.030
Ellis, J. C., Ross, R. P., and Hill, C. (2019). Nisin Z and lacticin 3147 improve efficacy of antibiotics against clinically significant bacteria. Future Microbiol. 14, 1573–1587. doi: 10.2217/FMB-2019-0153
Elving, G. J., van der Mei, H. C., Busscher, H. J., van Nieuw Amerongen, A., Veerman, E. C. I., van Weissenbruch, R., et al. (2000). Antimicrobial activity of synthetic salivary peptides against voice prosthetic microorganisms. Laryngoscope 110(2 Pt 1), 321–324. doi: 10.1097/00005537-200002010-00027
Ennahar, S., Sashihara, T., Sonomoto, K., and Ishizaki, A. (2000). Class IIa bacteriocins: biosynthesis, structure and activity. FEMS Microbiol. Rev. 24, 85–106. doi: 10.1111/J.1574-6976.2000.TB00534.X
Ericksen, B., Wu, Z., Lu, W., and Lehrer, R. I. (2005). Antibacterial activity and specificity of the six human α-defensins. Antimicrob. Agents Chemother. 49, 269–275. doi: 10.1128/AAC.49.1.269-275.2005/ASSET/107106E0-7E17-4495-B3E7-0E50296D74A2/ASSETS/GRAPHIC/ZAC0010546440003.JPEG
Faber, C., Hoogendoorn, R. J. W., Stallmann, H. P., Lyaruu, D. M., van Nieuw Amerongen, A., and Wuisman, P. I. J. M. (2004). In vivo comparison of Dhvar-5 and gentamicin in an MRSA osteomyelitis prevention model. J. Antimicrob. Chemother. 54, 1078–1084. doi: 10.1093/JAC/DKH441
Falanga, A., and Galdiero, S. (2017). Emerging therapeutic agents on the basis of naturally occurring antimicrobial peptides. Amino Acids Peptides Proteins 42, 190–227. doi: 10.1039/9781788010627-00190
Fan, Y., Li, X. D., He, P. P., Hu, X. X., Zhang, K., Fan, J. Q., et al. (2020). A biomimetic peptide recognizes and traps bacteria in vivo as human defensin-6. Sci. Adv. 6:eaaz4767. doi: 10.1126/SCIADV.AAZ4767
Fernandes, A., and Jobby, R. (2022). Bacteriocins from lactic acid bacteria and their potential clinical applications. Appl. Biochem. Biotechnol. doi: 10.1007/S12010-022-03870-3
Follacchio, G. A., Pala, A., Scaccianoce, S., Monteleone, F., Colletti, P. M., Rubello, D., et al. (2019). In vivo microbial targeting of 99mTc-labeled human β-Defensin-3 in a rat model of infection. Clin. Nucl. Med. 44, E602–E606. doi: 10.1097/RLU.0000000000002713
Fontana, M. B. C., Freire De Bastos, M. D. C., and Brandelli, A. (2006). Bacteriocins Pep5 and epidermin inhibit Staphylococcus epidermidis adhesion to catheters. Curr. Microbiol. 52, 350–353. doi: 10.1007/S00284-005-0152-5
Foster, T. J. (2004). The Staphylococcus aureus “superbug”. J. Clin. Invest. 114, 1693–1696. doi: 10.1172/JCI23825
Ganz, T. (2003). Defensins: antimicrobial peptides of innate immunity. Nat. Rev. Immunol. 3, 710–720. doi: 10.1038/nri1180
Ganz, T., Selsted, M. E., and Lehrer, R. I. (1990). Defensins. Eur. J. Haematol. 44, 1–8. doi: 10.1111/J.1600-0609.1990.TB00339.X
Ganz, T., Selsted, M. E., Szklarek, D., Harwig, S. S., Daher, K., Bainton, D. F., et al. (1985). Defensins. Natural peptide antibiotics of human neutrophils. J. Clin. Investig. 76, 1427–1435. doi: 10.1172/JCI112120
Gao, X., Ding, J., Liao, C., Xu, J., Liu, X., and Lu, W. (2021). Defensins: the natural peptide antibiotic. Adv. Drug Deliv. Rev. 179:114008. doi: 10.1016/J.ADDR.2021.114008
Garcia, A. E., Ösapay, G., Tran, P. A., Yuan, J., and Selsted, M. E. (2008). Isolation, synthesis, and antimicrobial activities of naturally occurring theta-defensin isoforms from baboon leukocytes. Infect. Immun. 76, 5883–5891. doi: 10.1128/IAI.01100-08
Garcia-Gutierrez, E., O’Connor, P. M., Saalbach, G., Walsh, C. J., Hegarty, J. W., Guinane, C. M., et al. (2020). First evidence of production of the lantibiotic nisin P. Sci. Rep. 10:3738. doi: 10.1038/S41598-020-60623-0
Geng, M., Ravichandran, A., Escano, J., and Smith, L. (2018). Efficacious analogs of the lantibiotic mutacin 1140 against a systemic methicillin-resistant Staphylococcus aureus infection. Antimicrob. Agents Chemother. 62:e01626–18. doi: 10.1128/AAC.01626-18
Giacometti, A., Cirioni, O., Kamysz, W., D’Amato, G., Silvestri, C., del Prete, M. S., et al. (2005). In vitro activity of the histatin derivative P-113 against multidrug-resistant pathogens responsible for pneumonia in immunocompromised patients. Antimicrob. Agents Chemother. 49, 1249–1252. doi: 10.1128/AAC.49.3.1249-1252.2005
Gibreel, T. M., and Upton, M. (2013). Synthetic epidermicin NI01 can protect galleria mellonella larvae from infection with Staphylococcus aureus. J. Antimicrob. Chemother. 68, 2269–2273. doi: 10.1093/JAC/DKT195
Grönberg, A., Mahlapuu, M., Ståhle, M., Whately-Smith, C., and Rollman, O. (2014). Treatment with LL-37 is safe and effective in enhancing healing of hard-to-heal venous leg ulcers: a randomized, placebo-controlled clinical trial. Wound Repair Regen. 22, 613–621. doi: 10.1111/WRR.12211
Gross, E., and Morell, J. L. (1971). Structure of nisin. J. Am. Chem. Soc. 93, 4634–4635. doi: 10.1021/JA00747A073
Harder, J., Bartels, J., Christophers, E., and Schröder, J. M. (2001). Isolation and characterization of human beta -defensin-3, a novel human inducible peptide antibiotic. J. Biol. Chem. 276, 5707–5713. doi: 10.1074/JBC.M008557200
Hasper, H. E., Kramer, N. E., Smith, J. L., Hillman, J. D., Zachariah, C., Kuipers, O. P., et al. (2006). An alternative bactericidal mechanism of action for lantibiotic peptides that target lipid II. Science 313, 1636–1637. doi: 10.1126/science.1129818/suppl_file/hasper.som.pdf
Hatziioanou, D., Gherghisan-Filip, C., Saalbach, G., Horn, N., Wegmann, U., Duncan, S. H., et al. (2017). Discovery of a novel lantibiotic nisin O from Blautia obeum A2-162, isolated from the human gastrointestinal tract. Microbiology 163, 1292–1305. doi: 10.1099/MIC.0.000515
Hell, É., Giske, C. G., Nelson, A., Römling, U., and Marchini, G. (2010). Human cathelicidin peptide LL37 inhibits both attachment capability and biofilm formation of Staphylococcus epidermidis. Lett. Appl. Microbiol. 50, 211–215. doi: 10.1111/J.1472-765X.2009.02778.X
Helmerhorst, E. J., van ‘t Hof, W., Veerman, E. C. I., Simoons-Smit, I., and Nieuw Amerongen, A. V. (1997). Synthetic histatin analogues with broad-spectrum antimicrobial activity. Biochem. J. 326, 39–45. doi: 10.1042/BJ3260039
Heunis, T. D. J., Smith, C., and Dicks, L. M. T. (2013). Evaluation of a nisin-eluting nanofiber scaffold to treat Staphylococcus aureus-induced skin infections in mice. Antimicrob. Agents Chemother. 57, 3928–3935. doi: 10.1128/AAC.00622-13/SUPPL_FILE/ZAC999102055SO1.PDF
Hiramatsu, K., Aritaka, N., Hanaki, H., Kawasaki, S., Hosoda, Y., Hori, S., et al. (1997). Dissemination in Japanese hospitals of strains of Staphylococcus aureus heterogeneously resistant to vancomycin. Lancet 350, 1670–1673. doi: 10.1016/S0140-6736(97)07324-8
Hiron, A., Falord, M., Valle, J., Débarbouillé, M., and Msadek, T. (2011). Bacitracin and nisin resistance in Staphylococcus aureus: a novel pathway involving the BraS/BraR two-component system (SA2417/SA2418) and both the BraD/BraE and VraD/VraE ABC transporters. Mol. Microbiol. 81, 602–622. doi: 10.1111/J.1365-2958.2011.07735.X
Horino, T., and Hori, S. (2020). Metastatic infection during Staphylococcus aureus bacteremia. J. Infect. Chemother. 26, 162–169. doi: 10.1016/J.JIAC.2019.10.003
Huang, D., Parker, D. M., Mandell, J. B., Brothers, K. M., Gish, C. G., Koch, J. A., et al. (2021). Prospective activity of PLG0206, an engineered antimicrobial peptide, on chronic periprosthetic joint infection total knee arthroplasty components ex vivo: The knee explant analysis (KnEA) study. Microbiol. Spectr. 9:e0187921. doi: 10.1128/SPECTRUM.01879-21
Huang, Q., Yu, H. J., Liu, G. D., Huang, X. K., Zhang, L. Y., Zhou, Y. G., et al. (2012). Comparison of the effects of human β-defensin 3, vancomycin, and clindamycin on Staphylococcus aureus biofilm formation. Orthopedics 35, e53–e60. doi: 10.3928/01477447-20111122-11
Humphries, R. M., Magnano, P., Burnham, C. A. D., Bard, J. D., Dingle, T. C., Callan, K., et al. (2020). Evaluation of surrogate tests for the presence of mecA-mediated methicillin resistance in Staphylococcus capitis, Staphylococcus haemolyticus, Staphylococcus hominis, and Staphylococcus warneri. J. Clin. Microbiol. 59:e02290–20. doi: 10.1128/JCM.02290-20
Islam, M. R., Nishie, M., Nagao, J. I., Zendo, T., Keller, S., Nakayama, J., et al. (2012). Ring A of nukacin ISK-1: A lipid II-binding motif for type-A(II) lantibiotic. J. Am. Chem. Soc. 134, 3687–3690. doi: 10.1021/JA300007H
Jack, R. W., Tagg, J. R., and Ray, B. (1995). Bacteriocins of gram-positive bacteria. Microbiol. Rev. 59, 171–200. doi: 10.1128/MR.59.2.171-200.1995
Janek, D., Zipperer, A., Kulik, A., Krismer, B., and Peschel, A. (2016). High frequency and diversity of antimicrobial activities produced by nasal Staphylococcus strains against bacterial competitors. PLoS Pathog. 12:e1005812. doi: 10.1371/JOURNAL.PPAT.1005812
Jensen, C., Li, H., Vestergaard, M., Dalsgaard, A., Frees, D., and Leisner, J. J. (2020). Nisin damages the septal membrane and triggers DNA condensation in methicillin-resistant Staphylococcus aureus. Front. Microbiol. 11:1007. doi: 10.3389/FMICB.2020.01007
Jesus, C., Soares, R., Cunha, E., Grilo, M., Tavares, L., and Oliveira, M. (2021). Influence of nisin-biogel at subinhibitory concentrations on virulence expression in Staphylococcus aureus isolates from diabetic foot infections. Antibiotics 10:1501. doi: 10.3390/ANTIBIOTICS10121501
Jia, Z., He, M., Wang, C., Chen, A., Zhang, X., Xu, J., et al. (2019). Nisin reduces uterine inflammation in rats by modulating concentrations of pro- and anti-inflammatory cytokines. Am. J. Reprod. Immunol. 81:e13096. doi: 10.1111/AJI.13096
Kaletta, C., Entian, K. D., Kellner, R., Jung, G., Reis, M., and Sahl, H. G. (1989). Pep5, a new lantibiotic: structural gene isolation and prepeptide sequence. Arch. Microbiol. 152, 16–19. doi: 10.1007/BF00447005
Kang, J., Dietz, M. J., and Li, B. (2019). Antimicrobial peptide LL-37 is bactericidal against Staphylococcus aureus biofilms. PLoS One 14:e0216676. doi: 10.1371/journal.pone.0216676
Kavanagh, N., Ryan, E. J., Widaa, A., Sexton, G., Fennell, J., O’Rourke, S., et al. (2018). Staphylococcal osteomyelitis: disease progression, treatment challenges, and future directions. Clin. Microbiol. Rev. 31:e00084–17. doi: 10.1128/CMR.00084-17
Kawada-Matsuo, M., Le, M. N. T., and Komatsuzawa, H. (2021). Antibacterial peptides resistance in Staphylococcus aureus: various mechanisms and the association with pathogenicity. Genes 12:1527. doi: 10.3390/GENES12101527
Kawada-Matsuo, M., Watanabe, A., Arii, K., Oogai, Y., Noguchi, K., Miyawaki, S., et al. (2020). Staphylococcus aureus virulence affected by an alternative nisin A resistance mechanism. Appl. Environ. Microbiol. 86, 1–14. doi: 10.1128/AEM.02923-19
Kawada-Matsuo, M., Yoshida, Y., Zendo, T., Nagao, J., Oogai, Y., Nakamura, Y., et al. (2013). Three distinct two-component systems are involved in resistance to the class I bacteriocins, nukacin ISK-1 and nisin A, in Staphylococcus aureus. PLoS One 8:e69455. doi: 10.1371/JOURNAL.PONE.0069455
Kim, H., Jang, J. H., Kim, S. C., and Cho, J. H. (2014). De novo generation of short antimicrobial peptides with enhanced stability and cell specificity. J. Antimicrob. Chemother. 69, 121–132. doi: 10.1093/JAC/DKT322
Kim, E. Y., Rajasekaran, G., and Shin, S. Y. (2017). LL-37-derived short antimicrobial peptide KR-12-a5 and its d-amino acid substituted analogs with cell selectivity, anti-biofilm activity, synergistic effect with conventional antibiotics, and anti-inflammatory activity. Eur. J. Med. Chem. 136, 428–441. doi: 10.1016/J.EJMECH.2017.05.028
Kimura, H., Matsusaki, H., Sashihara, T., Sonomoto, K., and Ishizaki, A. (1998). Purification and partial identification of Bacteriocin ISK-1, a new lantibiotic produced by Pediococcus sp. ISK-1. Biosci. Biotechnol. Biochem. 62, 2341–2345. doi: 10.1271/BBB.62.2341
Kranjec, C., Ovchinnikov, K. V., Grønseth, T., Ebineshan, K., Srikantam, A., and Diep, D. B. (2020). A bacteriocin-based antimicrobial formulation to effectively disrupt the cell viability of methicillin-resistant Staphylococcus aureus (MRSA) biofilms. NPJ Biofilms Microbiomes 6:58. doi: 10.1038/S41522-020-00166-4
Kruszewska, D., Sahl, H. G., Bierbaum, G., Pag, U., Hynes, S. O., and Ljungh, Å. (2004). Mersacidin eradicates methicillin-resistant Staphylococcus aureus (MRSA) in a mouse rhinitis model. J. Antimicrob. Chemother. 54, 648–653. doi: 10.1093/JAC/DKH387
Kumariya, R., Garsa, A. K., Rajput, Y. S., Sood, S. K., Akhtar, N., and Patel, S. (2019). Bacteriocins: classification, synthesis, mechanism of action and resistance development in food spoilage causing bacteria. Microb. Pathog. 128, 171–177. doi: 10.1016/J.MICPATH.2019.01.002
Lakhundi, S., and Zhang, K. (2018). Methicillin-resistant Staphylococcus aureus: molecular characterization, evolution, and epidemiology. Clin. Microbiol. Rev. 31:e00020–18. doi: 10.1128/CMR.00020-18
Laridi, R., Kheadr, E. E., Benech, R. O., Vuillemard, J. C., Lacroix, C., and Fliss, I. (2003). Liposome encapsulated nisin Z: optimization, stability and release during milk fermentation. Int. Dairy J. 13, 325–336. doi: 10.1016/S0958-6946(02)00194-2
Larrick, J. W., Hirata, M., Balint, R. F., Lee, J., Zhong, J., and Wright, S. C. (1995). Human CAP18: a novel antimicrobial lipopolysaccharide-binding protein. Infect. Immun. 63, 1291–1297. doi: 10.1128/IAI.63.4.1291-1297.1995
Lee, J. K., Chang, S. W., Perinpanayagam, H., Lim, S. M., Park, Y. J., Han, S. H., et al. (2013b). Antibacterial efficacy of a human β-defensin-3 peptide on multispecies biofilms. J. Endod. 39, 1625–1629. doi: 10.1016/J.JOEN.2013.07.035
Lee, T.-H. N., Hall, K., and Aguilar, M.-I. (2016). Antimicrobial peptide structure and mechanism of action: a focus on the role of membrane structure. Curr. Top. Med. Chem. 16, 25–39. doi: 10.2174/1568026615666150703121700
Lee, E., Jeong, K. W., Lee, J., Shin, A., Kim, J. K., Lee, J., et al. (2013a). Structure-activity relationships of cecropin-like peptides and their interactions with phospholipid membrane. BMB Rep. 46, 282–287. doi: 10.5483/BMBREP.2013.46.5.252
Lee, J. Y. H., Monk, I. R., Gonçalves da Silva, A., Seemann, T., Chua, K. Y. L., Kearns, A., et al. (2018). Global spread of three multidrug-resistant lineages of Staphylococcus epidermidis. Nat. Microbiol. 3, 1175–1185. doi: 10.1038/s41564-018-0230-7
Lei, J., Sun, L. C., Huang, S., Zhu, C., Li, P., He, J., et al. (2019). The antimicrobial peptides and their potential clinical applications. Am. J. Transl. Res. 11, 3919–3931.
Levine, D. P., Cushing, R. D., Jui, J., and Brown, W. J. (1982). Community-acquired methicillin-resistant Staphylococcus aureus endocarditis in the detroit medical center. Ann. Intern. Med. 97, 330–338. doi: 10.7326/0003-4819-97-3-330
Li, J., Chen, J., Yang, G., and Tao, L. (2021). Sublancin protects against methicillin-resistant Staphylococcus aureus infection by the combined modulation of innate immune response and microbiota. Peptides 141:170533. doi: 10.1016/J.PEPTIDES.2021.170533
Lindsay, J. A., and Holden, M. T. G. (2004). Staphylococcus aureus: superbug, super genome? Trends Microbiol. 12, 378–385. doi: 10.1016/J.TIM.2004.06.004
Lombardi, L., Falanga, A., del Genio, V., and Galdiero, S. (2019). A new Hope: self-assembling peptides with antimicrobial activity. Pharmaceutics 11:166. doi: 10.3390/PHARMACEUTICS11040166
Lowy, F. D. (1998). Staphylococcus aureus infections. N. Engl. J. Med. 339, 520–532. doi: 10.1056/NEJM199808203390806
Lubelski, J., Rink, R., Khusainov, R., Moll, G. N., and Kuipers, O. P. (2008). Biosynthesis, immunity, regulation, mode of action and engineering of the model lantibiotic nisin. Cell. Mol. Life Sci. 65, 455–476. doi: 10.1007/S00018-007-7171-2
Luo, Y., McLean, D. T. F., Linden, G. J., McAuley, D. F., McMullan, R., and Lundy, F. T. (2017). The naturally occurring host defense peptide, LL-37, and its truncated Mimetics KE-18 and KR-12 have selected biocidal and antibiofilm activities against Candida albicans, Staphylococcus aureus, and Escherichia coli in vitro. Front. Microbiol. 8:544. doi: 10.3389/FMICB.2017.00544
Mahlapuu, M., Håkansson, J., Ringstad, L., and Björn, C. (2016). Antimicrobial peptides: an emerging category of therapeutic agents. Front. Cell. Infect. Microbiol. 6:194. doi: 10.3389/FCIMB.2016.00194
Mathew, B., Olli, S., Guru, A., and Nagaraj, R. (2017). Chimeric analogs of human β-defensin 1 and θ-defensin disrupt pre-established bacterial biofilms. Bioorg. Med. Chem. Lett. 27, 3264–3266. doi: 10.1016/J.BMCL.2017.06.031
Merritt, J., and Qi, F. (2012). The mutacins of Streptococcus mutans: regulation and ecology. Mol Oral Microbiol 27, 57–69. doi: 10.1111/J.2041-1014.2011.00634.X
Midorikawa, K., Ouhara, K., Komatsuzawa, H., Kawai, T., Yamada, S., Fujiwara, T., et al. (2003). Staphylococcus aureus susceptibility to innate antimicrobial peptides, β-defensins and CAP18, expressed by human keratinocytes. Infect. Immun. 71, 3730–3739. doi: 10.1128/IAI.71.7.3730-3739.2003
Moravej, H., Moravej, Z., Yazdanparast, M., Heiat, M., Mirhosseini, A., Moosazadeh Moghaddam, M., et al. (2018). Antimicrobial peptides: features, action, and their resistance mechanisms in bacteria. Microb. Drug Resist. 24, 747–767. doi: 10.1089/MDR.2017.0392
Mota-Meira, M., Morency, H., and Lavoie, M. C. (2005). In vivo activity of mutacin B-Ny266. J. Antimicrob. Chemother. 56, 869–871. doi: 10.1093/JAC/DKI295
Mulders, J. W. M., Boerrigter, I. J., Rollema, H. S., Siezen, R. J., and de Vos, W. M. (1991). Identification and characterization of the lantibiotic nisin Z, a natural nisin variant. Eur. J. Biochem. 201, 581–584. doi: 10.1111/J.1432-1033.1991.TB16317.X
Nakazono, K., Le, M. N. T., Kawada-Matsuo, M., Kimheang, N., Hisatsune, J., Oogai, Y., et al. (2022). Complete sequences of epidermin and nukacin encoding plasmids from oral-derived Staphylococcus epidermidis and their antibacterial activity. PLoS One 17:e0258283. doi: 10.1371/JOURNAL.PONE.0258283
Narayana, J. L., Mishra, B., Lushnikova, T., Golla, R. M., and Wang, G. (2019). Modulation of antimicrobial potency of human cathelicidin peptides against the ESKAPE pathogens and in vivo efficacy in a murine catheter-associated biofilm model. Biochim. Biophys. Acta Biomembr. 1861, 1592–1602. doi: 10.1016/J.BBAMEM.2019.07.012
Nascimento, J. S., Ceotto, H., Nascimento, S. B., Giambiagi-DeMarval, M., Santos, K. R. N., and Bastos, M. C. F. (2006). Bacteriocins as alternative agents for control of multiresistant staphylococcal strains. Lett. Appl. Microbiol. 42, 215–221. doi: 10.1111/J.1472-765X.2005.01832.X
Natsis, N. E., and Cohen, P. R. (2018). Coagulase-negative Staphylococcus skin and soft tissue infections. Am. J. Clin. Dermatol. 19, 671–677. doi: 10.1007/S40257-018-0362-9
Nguyen, L. T., Haney, E. F., and Vogel, H. J. (2011). The expanding scope of antimicrobial peptide structures and their modes of action. Trends Biotechnol. 29, 464–472. doi: 10.1016/J.TIBTECH.2011.05.001
Nilsson, A. C., Janson, H., Wold, H., Fugelli, A., Andersson, K., Håkangård, C., et al. (2015). LTX-109 is a novel agent for nasal decolonization of methicillin-resistant and -sensitive Staphylococcus aureus. Antimicrob. Agents Chemother. 59, 145–151. doi: 10.1128/AAC.03513-14
Noore, J., Noore, A., and Li, B. (2013). Cationic antimicrobial peptide LL-37 is effective against both extra- and intracellular Staphylococcus aureus. Antimicrob. Agents Chemother. 57, 1283–1290. doi: 10.1128/AAC.01650-12
O’Connor, P. M., O’Shea, E. F., Guinane, C. M., O’Sullivan, O., Cotter, P. D., Ross, R. P., et al. (2015). Nisin H is a new nisin variant produced by the gut-derived strain Streptococcus hyointestinalis DPC6484. Appl. Environ. Microbiol. 81, 3953–3960. doi: 10.1128/AEM.00212-15/FORMAT/EPUB
O’Sullivan, J. N., O’Connor, P. M., Rea, M. C., O’Sullivan, O., Walsh, C. J., Healy, B., et al. (2020). Nisin J, a novel natural nisin variant, is produced by Staphylococcus capitis sourced from the human skin microbiota. J. Bacteriol. 202:e00639–19. doi: 10.1128/JB.00639-19
Okuda, K. I., Zendo, T., Sugimoto, S., Iwase, T., Tajima, A., Yamada, S., et al. (2013). Effects of bacteriocins on methicillin-resistant Staphylococcus aureus biofilm. Antimicrob. Agents Chemother. 57, 5572–5579. doi: 10.1128/AAC.00888-13
Oliveira, W. F., Silva, P. M. S., Silva, R. C. S., Silva, G. M. M., Machado, G., Coelho, L. C. B. B., et al. (2018). Staphylococcus aureus and Staphylococcus epidermidis infections on implants. J. Hosp. Infect. 98, 111–117. doi: 10.1016/J.JHIN.2017.11.008
Oppenheim, F. G., Xu, T., McMillian, F. M., Levitz, S. M., Diamond, R. D., Offner, G. D., et al. (1988). Histatins, a novel family of histidine-rich proteins in human parotid secretion. Isolation, characterization, primary structure, and fungistatic effects on Candida albicans. J. Biol. Chem. 263, 7472–7477. doi: 10.1016/S0021-9258(18)68522-9
Oscáriz, J. C., and Pisabarro, A. G. (2001). Classification and mode of action of membrane-active bacteriocins produced by gram-positive bacteria. Int. Microbiol. 4, 13–19. doi: 10.1007/S101230100003
Otto, M. (2009). Staphylococcus epidermidis – the “accidental” pathogen. Nat. Rev. Microbiol. 7, 555–567. doi: 10.1038/NRMICRO2182
Ouhara, K., Komatsuzawa, H., Kawai, T., Nishi, H., Fujiwara, T., Fujiue, Y., et al. (2008). Increased resistance to cationic antimicrobial peptide LL-37 in methicillin-resistant strains of Staphylococcus aureus. J. Antimicrob. Chemother. 61, 1266–1269. doi: 10.1093/JAC/DKN106
Ovchinnikov, K. V., Kranjec, C., Thorstensen, T., Carlsen, H., and Diep, D. B. (2020). Successful development of bacteriocins into therapeutic formulation for treatment of MRSA skin infection in a murine model. Antimicrob. Agents Chemother. 64:e00829–20. doi: 10.1128/AAC.00829-20
Pachón-Ibáñez, M. E., Smani, Y., Pachón, J., and Sánchez-Céspedes, J. (2017). Perspectives for clinical use of engineered human host defense antimicrobial peptides. FEMS Microbiol. Rev. 41, 323–342. doi: 10.1093/femsre/fux012
Panteleev, P. V., Bolosov, I. A., Balandin, S. V., and Ovchinnikova, T. V. (2015). Structure and biological functions of β-hairpin antimicrobial peptides. Acta Nat. 7, 37–47. doi: 10.32607/20758251-2015-7-1-37-47
Parthasarathy, S., Shah, S., Raja Sager, A., Rangan, A., and Durugu, S. (2020). Staphylococcus lugdunensis: review of epidemiology, complications, and treatment. Cureus 12:e8801. doi: 10.7759/CUREUS.8801
Peschel, A., Jack, R. W., Otto, M., Collins, L. V., Staubitz, P., Nicholson, G., et al. (2001). Staphylococcus aureus resistance to human defensins and evasion of neutrophil killing via the novel virulence factor MprF is based on modification of membrane lipids with l-lysine. J. Exp. Med. 193, 1067–1076. doi: 10.1084/JEM.193.9.1067
Peschel, A., Otto, M., Jack, R. W., Kalbacher, H., Jung, G., and Götz, F. (1999). Inactivation of the dlt operon in Staphylococcus aureus confers sensitivity to defensins, protegrins, and other antimicrobial peptides. J. Biol. Chem. 274, 8405–8410. doi: 10.1074/JBC.274.13.8405
Pimentel de Araujo, F., Monaco, M., del Grosso, M., Pirolo, M., Visca, P., and Pantosti, A. (2021). Staphylococcus aureus clones causing osteomyelitis: a literature review (2000–2020). J. Glob. Antimicrob. Resist. 26, 29–36. doi: 10.1016/J.JGAR.2021.03.030
Raja, Z., AndreA, S., Abbassi, F., Humblot, V., Lequin, O., Bouceba, T., et al. (2017). Insight into the mechanism of action of temporin-SHa, a new broad-spectrum antiparasitic and antibacterial agent. PLoS One 12:e0174024. doi: 10.1371/JOURNAL.PONE.0174024
Randall, C. P., Gupta, A., Utley-Drew, B., Lee, S. Y., Morrison-Williams, G., and O’Neill, A. J. (2018). Acquired nisin resistance in Staphylococcus aureus involves constitutive activation of an intrinsic peptide antibiotic detoxification module. mSphere 3:e00633–18. doi: 10.1128/MSPHEREDIRECT.00633-18
Ridyard, K. E., and Overhage, J. (2021). The potential of human peptide LL-37 as an antimicrobial and anti-biofilm agent. Antibiotics 10:650. doi: 10.3390/ANTIBIOTICS10060650
Rothstein, D. M., Spacciapoli, P., Tran, L. T., Xu, T., Roberts, F. D., Dalla Serra, M., et al. (2001). Anticandida activity is retained in P-113, a 12-amino-acid fragment of histatin 5. Antimicrob. Agents Chemother. 45, 1367–1373. doi: 10.1128/AAC.45.5.1367-1373.2001/ASSET/19B26A2D-F838-4654-B954-789BF10621CA/ASSETS/GRAPHIC/AC0510786003.JPEG
Roy, U., Islam, M. R., Nagao, J. I., Iida, H., Al-Mahin, A., Li, M., et al. (2014). Bactericidal activity of nukacin ISK-1: an alternative mode of action. Biosci. Biotechnol. Biochem. 78, 1270–1273. doi: 10.1080/09168451.2014.918485
Sajjan, U. S., Tran, L. T., Sole, N., Rovaldi, C., Akiyama, A., Friden, P. M., et al. (2001). P-113d, an antimicrobial peptide active against Pseudomonas aeruginosa, retains activity in the presence of sputum from cystic fibrosis patients. Antimicrob. Agents Chemother. 45, 3437–3444. doi: 10.1128/AAC.45.12.3437-3444.2001
Saravolatz, L. D., Markowitz, N., Arking, L., Pohlod, D., and Fisher, E. (1982). Methicillin-resistant Staphylococcus aureus. Epidemiologic observations during a community-acquired outbreak. Ann. Intern. Med. 96, 11–16. doi: 10.7326/0003-4819-96-1-11
Schneider, J. J., Unholzer, A., Schaller, M., Schäfer-Korting, M., and Korting, H. C. (2005). Human defensins. J. Mol. Med. 83, 587–595. doi: 10.1007/S00109-005-0657-1
Schnell, N., Entian, K. D., Schneider, U., Götz, F., Zähner, H., Kellner, R., et al. (1988). Prepeptide sequence of epidermin, a ribosomally synthesized antibiotic with four sulphide-rings. Nature 333, 276–278. doi: 10.1038/333276a0
Schutte, B. C., Mitros, J. P., Bartlett, J. A., Walters, J. D., Jia, H. P., Welsh, M. J., et al. (2002). Discovery of five conserved beta -defensin gene clusters using a computational search strategy. Proc. Natl. Acad. Sci. U. S. A. 99, 2129–2133. doi: 10.1073/PNAS.042692699
Sieprawska-Lupa, M., Mydel, P., Krawczyk, K., Wójcik, K., Puklo, M., Lupa, B., et al. (2004). Degradation of human antimicrobial peptide LL-37 by Staphylococcus aureus-derived proteinases. Antimicrob. Agents Chemother. 48, 4673–4679. doi: 10.1128/AAC.48.12.4673-4679.2004
Simonetti, O., Cirioni, O., Goteri, G., Lucarini, G., Kamysz, E., Kamysz, W., et al. (2021). Efficacy of cathelicidin LL-37 in an MRSA wound infection mouse model. Antibiotics 10:1210. doi: 10.3390/ANTIBIOTICS10101210
Smeianov, V., Scott, K., and Reid, G. (2000). Activity of cecropin P1 and FA-LL-37 against urogenital microflora. Microbes Infect. 2, 773–777. doi: 10.1016/S1286-4579(00)90359-9
Soltani, S., Hammami, R., Cotter, P. D., Rebuffat, S., Ben Said, L., Gaudreau, H., et al. (2021). Bacteriocins as a new generation of antimicrobials: toxicity aspects and regulations. FEMS Microbiol. Rev. 45, 1–24. doi: 10.1093/femsre/fuaa039
Sun, T., Hou, M., Zhang, N., Yang, J., Meng, X., Yang, R., et al. (2013). Antimicrobial peptide LL-37 and IDR-1 ameliorate MRSA pneumonia in vivo. Cell. Physiol. Biochem. 32, 614–623. doi: 10.1159/000354465
Takahashi, D., Shukla, S. K., Prakash, O., and Zhang, G. (2010). Structural determinants of host defense peptides for antimicrobial activity and target cell selectivity. Biochimie 92, 1236–1241. doi: 10.1016/J.BIOCHI.2010.02.023
Tang, Y. Q., Yuan, J., Ösapay, G., Ösapay, K., Tran, D., Miller, C. J., et al. (1999). A cyclic antimicrobial peptide produced in primate leukocytes by the ligation of two truncated alpha-defensins. Science 286, 498–502. doi: 10.1126/SCIENCE.286.5439.498
Thapa, R. K., Winther-Larsen, H. C., Diep, D. B., and Tønnesen, H. H. (2020). Preformulation studies on novel garvicin KS peptides for topical applications. Eur. J. Pharm. Sci. 151:105333. doi: 10.1016/J.EJPS.2020.105333
Ting, D. S. J., Goh, E. T. L., Mayandi, V., Busoy, J. M. F., Aung, T. T., Periayah, M. H., et al. (2021). Hybrid derivative of cathelicidin and human beta defensin-2 against gram-positive bacteria: A novel approach for the treatment of bacterial keratitis. Sci. Rep. 11:18304. doi: 10.1038/S41598-021-97821-3
Tong, S. Y. C., Davis, J. S., Eichenberger, E., Holland, T. L., and Fowler, V. G. (2015). Staphylococcus aureus infections: epidemiology, pathophysiology, clinical manifestations, and management. Clin. Microbiol. Rev. 28, 603–661. doi: 10.1128/CMR.00134-14
Travis, S. M., Anderson, N. N., Forsyth, W. R., Espiritu, C., Conway, B. D., Greenberg, E. P., et al. (2000). Bactericidal activity of mammalian cathelicidin-derived peptides. Infect. Immun. 68, 2748–2755. doi: 10.1128/IAI.68.5.2748-2755.2000
Tsai, H., and Bobek, L. A. (1998). Human salivary histatins: promising anti-fungal therapeutic agents. Crit. Rev. Oral Biol. Med. 9, 480–497. doi: 10.1177/10454411980090040601
Turner, N. A., Sharma-Kuinkel, B. K., Maskarinec, S. A., Eichenberger, E. M., Shah, P. P., Carugati, M., et al. (2019). Methicillin-resistant Staphylococcus aureus: an overview of basic and clinical research. Nat. Rev. Microbiol. 17, 203–218. doi: 10.1038/S41579-018-0147-4
Vandamme, D., Landuyt, B., Luyten, W., and Schoofs, L. (2012). A comprehensive summary of LL-37, the factotum human cathelicidin peptide. Cell. Immunol. 280, 22–35. doi: 10.1016/J.CELLIMM.2012.11.009
Vankeerberghen, A., Scudiero, O., de Boeck, K., Macek, M., Pignatti, P. F., van Hul, N., et al. (2005). Distribution of human beta-defensin polymorphisms in various control and cystic fibrosis populations. Genomics 85, 574–581. doi: 10.1016/J.YGENO.2005.02.003
Varella Coelho, M. L., dos Santos Nascimento, J., Fagundes, P. C., Madureira, D. J., de Oliveira, S. S., de Paiva, V., et al. (2007). Activity of staphylococcal bacteriocins against Staphylococcus aureus and Streptococcus agalactiae involved in bovine mastitis. Res. Microbiol. 158, 625–630. doi: 10.1016/J.RESMIC.2007.07.002
Voss, A., Loeffen, F., Bakker, J., Klaassen, C., and Wulf, M. (2005). Methicillin-resistant Staphylococcus aureus in pig farming. Emerg. Infect. Dis. 11, 1965–1966. doi: 10.3201/EID1112.050428
Vuong, C., and Otto, M. (2002). Staphylococcus epidermidis infections. Microbes Infect. 4, 481–489. doi: 10.1016/S1286-4579(02)01563-0
Wang, H., She, Y., Chu, C., Liu, H., Jiang, S., Sun, M., et al. (2015). Preparation, antimicrobial and release behaviors of nisin-poly (vinyl alcohol)/wheat gluten/ZrO2 nanofibrous membranes. J. Mater. Sci. 50, 5068–5078. doi: 10.1007/S10853-015-9059-0/FIGURES/9
Wang, S., Wang, Q., Zeng, X., Ye, Q., Huang, S., Yu, H., et al. (2017). Use of the antimicrobial peptide sublancin with combined antibacterial and immunomodulatory activities to protect against methicillin-resistant Staphylococcus aureus infection in mice. J. Agric. Food Chem. 65, 8595–8605. doi: 10.1021/ACS.JAFC.7B02592/ASSET/IMAGES/ACS.JAFC.7B02592.SOCIAL.JPEG_V03
Wang, Q., Zeng, X., Wang, S., Hou, C., Yang, F., Ma, X., et al. (2014). The bacteriocin sublancin attenuates intestinal injury in young mice infected with Staphylococcus aureus. Anat. Rec. 297, 1454–1461. doi: 10.1002/AR.22941
Watanabe, A., Kawada-Matsuo, M., Le, M. N. T., Hisatsune, J., Oogai, Y., Nakano, Y., et al. (2021). Comprehensive analysis of bacteriocins in Streptococcus mutans. Sci. Rep. 11:12963. doi: 10.1038/S41598-021-92370-1
Weigel, L. M., Clewell, D. B., Gill, S. R., Clark, N. C., McDougal, L. K., Flannagan, S. E., et al. (2003). Genetic analysis of a high-level vancomycin-resistant isolate of Staphylococcus aureus. Science 302, 1569–1571. doi: 10.1126/SCIENCE.1090956
Welling, M. M., Brouwer, C. P. J. M., van ‘t Hof, W., Veerman, E. C. I., and Nieuw Amerongen, A. V. (2007). Histatin-derived monomeric and dimeric synthetic peptides show strong bactericidal activity towards multidrug-resistant Staphylococcus aureus in vivo. Antimicrob. Agents Chemother. 51, 3416–3419. doi: 10.1128/AAC.00196-07
Wertheim, H. F. L., Melles, D. C., Vos, M. C., van Leeuwen, W., van Belkum, A., Verbrugh, H. A., et al. (2005). The role of nasal carriage in Staphylococcus aureus infections. Lancet Infect. Dis. 5, 751–762. doi: 10.1016/S1473-3099(05)70295-4
Wilaipun, P., Zendo, T., Okuda, K. I., Nakayama, J., and Sonomoto, K. (2008). Identification of the nukacin KQU-131, a new type-A(II) lantibiotic produced by Staphylococcus hominis KQU-131 isolated from Thai fermented fish product (Pla-ra). Biosci. Biotechnol. Biochem. 72, 2232–2235. doi: 10.1271/BBB.80239
Willey, J. M., and van der Donk, W. A. (2007). Lantibiotics: peptides of diverse structure and function. Annu. Rev. Microbiol. 61, 477–501. doi: 10.1146/ANNUREV.MICRO.61.080706.093501
Wirawan, R. E., Klesse, N. A., Jack, R. W., and Tagg, J. R. (2006). Molecular and genetic characterization of a novel nisin variant produced by Streptococcus uberis. Appl. Environ. Microbiol. 72, 1148–1156. doi: 10.1128/AEM.72.2.1148-1156.2006
Yu, W., Ning, N., Xue, Y., Huang, Y., Guo, F., Li, T., et al. (2021). A chimeric cationic peptide composed of human β-defensin 3 and human β-defensin 4 exhibits improved antibacterial activity and salt resistance. Front. Microbiol. 12:663151. doi: 10.3389/FMICB.2021.663151
Zanetti, M. (2004). Cathelicidins, multifunctional peptides of the innate immunity. J. Leukoc. Biol. 75, 39–48. doi: 10.1189/JLB.0403147
Zendo, T., Fukao, M., Ueda, K., Higuchi, T., Nakayama, J., and Sonomoto, K. (2003). Identification of the lantibiotic nisin Q, a new natural nisin variant produced by Lactococcus lactis 61-14 isolated from a river in Japan. Biosci. Biotechnol. Biochem. 67, 1616–1619. doi: 10.1271/BBB.67.1616
Zhang, L. J., and Gallo, R. L. (2016). Antimicrobial peptides. Curr. Biol. 26, R14–R19. doi: 10.1016/J.CUB.2015.11.017
Zhang, Y., Narayana, J. L., Wu, Q., Dang, X., and Wang, G. (2021). Structure and activity of a selective antibiofilm peptide SK-24 derived from the NMR structure of human cathelicidin LL-37. Pharmaceuticals 14:1245. doi: 10.3390/PH14121245
Zhao, X., and Kuipers, O. P. (2021). Nisin- and ripcin-derived hybrid lanthipeptides display selective antimicrobial activity against Staphylococcus aureus. ACS Synth. Biol. 10, 1703–1714. doi: 10.1021/ACSSYNBIO.1C00080/SUPPL_FILE/SB1C00080_SI_001.PDF
Keywords: antimicrobial peptides, staphylococci, MRSA, MRSE, human AMPs, bacteriocins
Citation: Le MN, Kawada-Matsuo M and Komatsuzawa H (2022) Efficiency of Antimicrobial Peptides Against Multidrug-Resistant Staphylococcal Pathogens. Front. Microbiol. 13:930629. doi: 10.3389/fmicb.2022.930629
Received: 28 April 2022; Accepted: 18 May 2022;
Published: 09 June 2022.
Edited by:
András Fodor, University of Szeged, HungaryReviewed by:
Márió Gajdács, University of Szeged, HungaryCopyright © 2022 Le, Kawada-Matsuo and Komatsuzawa. This is an open-access article distributed under the terms of the Creative Commons Attribution License (CC BY). The use, distribution or reproduction in other forums is permitted, provided the original author(s) and the copyright owner(s) are credited and that the original publication in this journal is cited, in accordance with accepted academic practice. No use, distribution or reproduction is permitted which does not comply with these terms.
*Correspondence: Hitoshi Komatsuzawa, a29tYXRzdXpAaGlyb3NoaW1hLXUuYWMuanA=
Disclaimer: All claims expressed in this article are solely those of the authors and do not necessarily represent those of their affiliated organizations, or those of the publisher, the editors and the reviewers. Any product that may be evaluated in this article or claim that may be made by its manufacturer is not guaranteed or endorsed by the publisher.
Research integrity at Frontiers
Learn more about the work of our research integrity team to safeguard the quality of each article we publish.