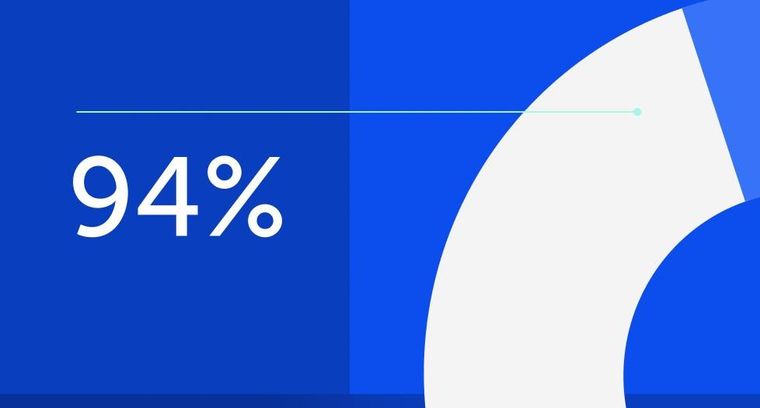
94% of researchers rate our articles as excellent or good
Learn more about the work of our research integrity team to safeguard the quality of each article we publish.
Find out more
REVIEW article
Front. Microbiol., 09 June 2022
Sec. Microbiotechnology
Volume 13 - 2022 | https://doi.org/10.3389/fmicb.2022.929730
This article is part of the Research TopicBiotechnological Advances in Bioremediation of Heavy Metal Polluted SoilsView all 9 articles
A number of anthropogenic and weathering activities accumulate heavy metals in soils, causing adverse effects on soil characteristics, microbial activity (diversity), agricultural practices, and underground aquifers. Controlling soil heavy metal pollution is difficult due to its persistence in soils, resulting in the deposition and transmission into the food web via agricultural food products, ultimately affecting human health. This review critically explores the potential for remediation of metal-contaminated soils using a biochar-based responsible approach. Plant-based biochar is an auspicious bio-based residue substance that can be used for metal-polluted soil remediation and soil improvement as a sustainable approach. Plants with rapid growth and increased biomass can meet the requirements for phytoremediation in large quantities. Recent research indicates significant progress in understanding the mechanisms of metal accumulation and contaminant movement in plants used for phytoremediation of metal-contaminated soil. Excessive contamination reduces plant biomass and growth, which has substantial hyperaccumulating possibilities and is detrimental to the phytoremediation process. Biochar derived from various plant sources can promote the growth and phytoremediation competence of native or wild plants grown in metal-polluted soil. Carbon-enriched biochar encourages native microbial growth by neutralizing pH and providing nutritional support. Thus, this review critically discusses the influence of plant and agricultural waste-based biochar on plant phytoremediation potential in metal-contaminated soils.
Land degradation and soil contamination are a persistent threat to humans’ and the environment’s wellbeing (Azam, 2016). Heavy metal and metalloid intensification in soil have increased rapidly in terms of natural phenomena and anthropogenic activities, including mining, agricultural activities, and industrial and municipal discharge, which all pose severe threats to environmental protection and public health (Sharma et al., 2022). Because they are non-biodegradable, they might remain in the soil, enter into the food chain via agricultural crops, and even accumulate in humans via biomagnification/bioaccumulation (Gogoi et al., 2021). Heavy metals are a class of elements distinguished by their high atomic weight and mass, with a specific density of greater than 5 g/cm3 (Buha et al., 2014). There are 21 non-metals, 16 light metals, and 53 heavy metals among the 90 naturally occurring elements (Gholizadeh and Hu, 2021). Such elements are divided into two categories: those that could be needed in trace amounts (Cu, Zn, Ni, Fe, V, Mn, Co, and Mo) by certain organisms and others such as Pb, As, Cd, and Hg, which are entirely considered as dangerous (Naja and Volesky, 2017). Heavy metals in their natural state are not available for root uptake or are not accessible to living beings. Anthropogenic sources, such as battery manufacturing, mineral extraction (mining), explosives, pesticides, herbicides, chemical fertilizers, and effluent irrigation, cause an excessive increase in such elements, contributing to their deposition and distribution (Dutta and Sharma, 2019). When these activities exceed acceptable levels, they endanger all living beings and have disastrous effects on their concentration. These elements are tolerant in various ways depending on the life form with which they are confronted (Villa-Achupallas et al., 2018). Metal pollution has posed a significant risk to human health as well as the environment due to its toxic nature. Hence, remediation of metal pollution from soils is critical (Rathour et al., 2022). Numerous remediation strategies depending on their mobilization or immobilization mechanisms have indeed been established to address these issues (Akcil et al., 2015; Wang et al., 2021). However, they are typically very expensive, and planned remediation is often delayed due to the absence of sufficient funds (Yrjälä and Lopez-Echartea, 2021). Unique nature-based substances are emerging that ought to be cost-effective in remedial work but necessitate further development because they require useful insights into the structure–function relationships (Byrne et al., 2018). Biochar, a carbon-rich component, is thought to play an important role in the bioavailability of heavy metal-polluted soil, resulting in biotransformation and bioremediation (Yu et al., 2019). However, biochar is frequently produced from various feedstocks using different pyrolysis processes; hence, the surface characteristics may vary significantly (Yu et al., 2019). Plants and biochar blending can be used to enhance the in situ or on-site bioremediation. Nevertheless, this is critical to address a few essential lines of study to ensure the safe and long-term use of biochar. Biochar is being developed for use in the environmental cleanup of both inorganic and organic contaminants, and their integration with phytoremediation is an excellent option (Rodriguez-Franco and Page-Dumroese, 2021). Since then, biochar-blended phytoremediation has grown in popularity as a groundbreaking technology for enhancing the phytoremediation potency in metal-polluted soils (Muthusaravanan et al., 2020). Various biochar properties demonstrate their influence on heavy metal transport, mobilization, and precipitation, improving soil structure, the release of nutrients, and microbial diversity, thus supporting plant growth (Yuan et al., 2019). This review presents the environmental influence and applications of biochar-blended phytoremediation of heavy metal-polluted soils and their interaction with plants during remediation.
The primary ingredients for biochar production are agricultural, forestry, household, and livestock waste (Figure 1), which are all abundant across the world. Agricultural waste has previously been used in a limited number of applications, including as a renewable source and animal feed (Spiertz and Ewert, 2009). Another report stated that the nationwide possibilities for producing biochar from agricultural biomass have been calculated and predicted to be around 3.1 million tons of biochar from around 10.7 million tons of biomass (Awasthi et al., 2021). The highest biomass is derived primarily from rice husk, which has a yield of 6.8 million t y–1 and can produce biochar up to 1.77 million t y–1, accounting for approximately 56.48% of the total nationwide biochar fabrication potential (Susilawati et al., 2020).
Biochar is being used as a soil conditioner and is acquired at low temperature pyrolysis—ranging from 400 to 700°C—of numerous biomasses including manure (cow dung), agriculture waste (wastes of maize, sugarcane, weeds, and so on), and biosolids in the absence of oxygen. It is thus differentiated from charcoal (Khiari et al., 2019). The physical and chemical properties of biochar obtained from wood, agricultural residues, poultry manure, or sludge at various pyrolysis temperatures are summarized in Table 1. Although the physicochemical characteristics of biochar diversified substantially due to the fabrication from a wide range of feedstocks using varying pyrolysis processes, biochar is usually basic in nature with such a large specific surface area, huge porosity, changeable charges, and different functional groups, as mentioned in Table 1. Such properties can also have an impact on pH, conductivity (CEC), and surface adsorption capacities. Biochar particle size is determined by the standard particle size of the feedstock; nevertheless, it is usually much smaller due to shrinking and attrition during the pyrolysis process. Due to the improved tensile strength of raw materials at higher pyrolysis temperatures, it may yield smaller-sized biochar particles (Albalasmeh et al., 2020). The functional groups on the surface of biochar, porous structure, and ionic charges can aid in the physical adsorption (Zhang et al., 2020), co-precipitation (Chen et al., 2022), complexation, mobilization/immobilization (Hu et al., 2020), and detoxification (Alrashidi et al., 2020) of metal pollutants and support the hyperaccumulator’s phytoremediation potential.
Table 1. Physical and chemical properties of biochar obtained from various plant residues and manure.
Biochar consists of a variety of nutrients, including K, Mg, K, Ca, and P, which are derived from the pyrolysis raw material. During pyrolysis, the dissolved organic material is also formed (de Figueiredo et al., 2021). Hence, the biochar amalgamation could provide plants and microorganisms with bioavailable nutrients. The quantity and the type of the bioavailable nutrient content in biochar, on the other hand, are highly dependent on the raw material (feedstock) and pyrolysis conditions (Yang et al., 2019).
The elements such as C and N in biochar differed significantly while obtained from pine trees, poultry manure, and peanut husk at 400 and 500°C, respectively (El-Bassi et al., 2021). Furthermore, transferable phosphate, potassium, calcium, and magnesium were significantly higher in biochar produced at 500°C than in biochar produced at 400°C (Ferjani et al., 2019). The deviation was primarily associated with the high pyrolysis temperature, which enhanced raw material mineralization besides reduced CEC. From this standpoint, obtaining nutrient-enriched biochar from a nutrient-enriched raw material under appropriate pyrolysis conditions is critical (Ma et al., 2015). In fact, plant-derived biochar appear to have a reduced nutrient composition than biochar derived from manure (Embrandiri et al., 2012).
When biochar is applied to the soil, it appears as a separate particulate matter that differs from many other kinds of solid organic materials, which are either encapsulated in soil pore spaces or adsorbed on the mineral surfaces and obscured in aggregate particles (Kumar et al., 2018). Biochar with much more aromatic black carbons on the exterior seems to be more consistent in soil than other forms of organic carbons, thus improving carbon storage potential in soil properties (Lian and Xing, 2017). A previous study reported that the biochar mineralization rates are very low, with carbon half-lives up to 100 years (Williams et al., 2019). According to another investigation, perfect biochar particles were found in soils in wet tropical climates including the Amazon for millennia (Agegnehu et al., 2017).
Biogeochemical interactions in the ecosystem have a significant impact on the destiny, transfer, and conversion or modifications of metals and metalloids (Breda et al., 2018). Because ionic metals and metalloids can occur in both anionic and cationic aspects, their behavior will be influenced by interactions with anionic and cationic charges of the biochar surface (Fijałkowska et al., 2021). When combined with topsoil, biochar with negative charges can strongly adsorb positive components (e.g., Cd2+ and Pb2+), whereas biochar with cationic charges can maintain anionic metal(loid)s (e.g., arsenite and arsenate) (Gupta et al., 2021). Adsorption mechanism, surface (co)precipitation, and surface complexation with active functional moieties are the major mechanisms for the immobilization of cationic metals (including Pb2+) and metalloids through biochar (Gupta et al., 2021). Thus, the biochar-stimulated improvements in soils, including increased soil pH, can reduce the bioavailability of cationic metals and metalloids even further. Since the physical and chemical attributes of biochar depend on the raw material type and pyrolysis circumstances (e.g., temperature and frequency of temperature rise), it is essential to recognize appropriate raw materials for biochar fabrication that have the efficiency to remediate various metals and metalloids in specific soils (Akhil et al., 2021). Anionic metalloids, including Cr, Se, and As, are frequently found as dominant species in soils with alkaline pH compared to cationic metalloids that are poorly adsorbed by negatively charged soil (Gupta et al., 2021).
The redox potential of metals and metalloids can influence their mobility in soils. For instance, the reduced redox potential of As (As3+ and As5+) has much higher permeability in soils than the increased redox potential of Cr (Cr6+ and Cr3+) (Wang et al., 2020). Furthermore, the oxidation state of soils can influence the redox potential of metals and metalloids. For instance, it has been revealed that biochar converts Cr6+ to the less mobile Cr3+ through consistently transferring electrons, which may have been connected with oxygen-containing active functional groups on that biochar surface (Dong et al., 2017). Furthermore, microbial metabolism utilizing biochar-derived organic carbon material can reduce Cr6+. The poor Cr solubility led to the reduction process, and Cr immobilization in soil has been enhanced. The adsorption and desorption mechanisms of metalloids and metals in soils are also significantly influenced by pH and organic matter because the adsorption of positively charged metals in biochar is high in acidic soil. In acidic soils (pH 3.5–6.0), Cr occurs predominantly in the positively charged forms Cr3(OH)45+ and Cr(OH)2+ (Wang et al., 2020). The biochar amendment to soil could perhaps alter the dissolved organic content (DOC) and pH, thus resulting in the mobility of metals and metalloids.
According to some research findings, biochar-amended soil could improve the mobility of metals and metalloids such as Sb, As, and Cu (Beesley et al., 2013; Sun et al., 2018). For example, increased pH in biochar-amended soils led to increased As mobility (Beesley et al., 2013). Electrostatic interaction between anionic As and Sb elements and negatively charged biochar substrates may enhance effective desorption of As and Sb by increasing mobilization. In the case of Cu, mobility is strongly correlated with the DOC content in biochar. Cu can be immobilized by the adsorption process in the biochar (prepared at 600°C) surface with an elevated DOC content (Sun et al., 2018).
Biochar aids in the bioremediation of organic and inorganic pollutants. The primary mechanism is an upsurge of microbial diversity that degrades hydrocarbons (petroleum) in biochar-amended polluted soils (Karppinen et al., 2017). Heavy metals and metalloids cannot be deteriorated or completely eradicated from the ecosystem, but they can be transformed from one form to another, from higher concentration to lower concentration. Heavy metals and metalloids can also accumulate in organisms (Verma et al., 2021). Hence, most frequently, two strategies are used for the heavy metal and metalloid bioremediation process (Li et al., 2019). Absorption and accumulation of metals and metalloids in timber plants and crops with bioenergy potential in metal-polluted farmlands, and their deduction by harvesting the biomass containing/accumulated with metals and metalloids, and the transformation of toxic metals and metalloids into lesser toxic products (complex form), which can be adsorbed by native microorganisms and further reduce their toxicity and migration (Sun et al., 2018).
Cd2+ denotes cationic metal ion (A) physiological adsorption of cationic metals and metalloids of water from soil pores; (B) biochar co-precipitation with chloride, carbonates, silicate, and phosphate with metals; (C) complex formation with biochar surface functional groups; and (D) gradual nutritional discharge of DOC, N, Ca, P, and K for growth of plants and microbes in the root region (Figure 2). The mechanisms (A), (B), and (C) can minimize the bioavailable metal content in pore water, lowering phytotoxicity even further.
The negatively charged outer layer of biochar and its alkaline character can adsorb and sustain toxic metals through various mechanisms. Biochar, via gradually discharging nutrients and maintaining healthy soil structures and properties, also generates much more favorable soil conditions for the growth of beneficial microbes and plants (Das et al., 2021).
The existence of biochar significantly increased the lowering precipitation of Cr6+ to Cr3+ in the contaminated soils due to remarkably improved microbial activities encouraged by releasing carbon and other nutrients from biochar (Choppala et al., 2012). Furthermore, a decrease in the concentration may aid in the immobilization of metals and metalloids, including Cr6+ and U6+, but no evidence to date has demonstrated the role of biochar in bioremediation/phytoremediation. In addition to the effectively improved bioremediation, the existence of biochar does provide an indirect mechanism for metal and metalloid bioremediation (Sun et al., 2018; Gong et al., 2019).
Calcite precipitation caused by microbes can firmly adsorb and co-precipitate metals and metalloids on the surfaces. The metal ions along with an ionic radius similar to that of Ca2+, including Cu2+, Cd2+, and Pb2+, may be integrated into calcite crystal particles through alternative reactions during calcite precipitation (Achal et al., 2011). Biochar aided this strategy by making microbe-friendly soil conditions and potentially increasing bioremediation efficiency (Arif et al., 2020). Bambusa vulgaris biochar with an O2-releasing bead has been recently demonstrated as a promising O2-releasing substance used in soils and groundwater bioremediation (Wu et al., 2015). This kind of biochar does have the potential to enhance the oxidation level (from As3+ to a less mobile form) of metals and metalloids.
A few research studies have investigated on biochar-augmented phytostabilization of metals and metalloids (e.g., Zn, As, Ni, Cd, Sb, Cu, and Cr) in contaminated soils (Uchimiya et al., 2012). Figure 3 represents the possible influence of biochar on bioremediation/phytoremediation of metal-contaminated soil. Arsenic (As) is well-recognized to react differently from some other metals and metalloids since the mobility of As can be diminished in acidic soils, owing to the enhanced sorption process on ferric oxide under an acidic environment. Hartley et al. (2009) demonstrated that biochar can also be applied for phytostabilization with Miscanthus species. Moreover, the analysis revealed that adding biochar derived from hardwood to soil samples did not improve As transport to Miscanthus plants, whereas alkaline biochar can mobilize As in metal-contaminated soils (Hartley et al., 2009). Cu and Pb were relatively straightforward to be stabilized in biochar-administered soils, whereas Cd and Ni differed widely depending on the nature of biochar used (Uchimiya et al., 2012; Sun et al., 2018). The stabilization mechanism is often probably due to increases in soil pH. The detailed research has shown that soil alterations (addition of lime) can be merged with phytoremediators to considerably reduce the bioavailability of metals and metalloids. Furthermore, biochar is also more effective at governing the accessibility of toxic compounds, as well as enhancing plant biomass fabrication and restoration performance (Břendová et al., 2015; Maddalwar et al., 2021).
Figure 3. Phytoremediation potential of hyperaccumulator on metal-contaminated soil with and without the amendment of biochar.
Plant yield increases with biochar supplementation (Ambika et al., 2022) are connected to water and nutrient retainment, enhanced biological activities, and neutralized soil pH. Hence, biochar has the efficiency to be used as an amendment to reduce metal bioaccumulation in plants. Moreover, alterations in soil pH in the rhizosphere can feasibly influence the metal and metalloid mobilization efficiency of biochar in soils, while rhizosphere acidification should be avoided (Houben and Sonnet, 2015). Biochar is thought to interact with soils and balance their properties for an extended period of time. Thus, the redox mechanisms may cause biochar to change, a process called aging (Gul et al., 2015). The immobilization of heavy metals and metalloids in biochar has been associated with the lability of metals (e.g., Pb2+ is more mobile than Cd2+). A wide range of functional groups, including hydroxyl, carboxylic, and phenolic groups could be established during the aging process, and biochar aging had no effect on the immobilization of positively charged metals and metalloids in soils containing aged biochar (Heitkötter and Marschner, 2015; Fan et al., 2018).
Phytoremediation is a multidisciplinary field with the goal of mobilizing and/or immobilizing pollutants from different environmental conditions (Shah and Daverey, 2020). Phytoremediation encompasses phytostabilization, rhizoremediation, phytoextraction, phytodegradation, and phytovolatilization in general (Shah and Daverey, 2020). In comparison to certain other remediation practices for heavy metals and metalloids, including chemical immobilization, digging, and dumping, phytoremediation is gaining popularity due to its efficiency and lower cost (Wu et al., 2015). Other advantages, including erosion control and pollutant leaching prevention, are critical for future soil management and development. Table 2 summarizes some biochar-assisted phytoremediation plants for metal- and metalloid-polluted soils (Sun et al., 2018).
The primary method for remediating soil contamination is the phytoextraction process, which is typically associated with the ability of hyperaccumulators and energy plants to bioaccumulate metals and metalloids (Rezania et al., 2016). Numerous plant species were also used to extract various metals (e.g., Cr, Cd, Pb, As, Co, Cu, Zn, and Ni) from soils (Cameselle and Pena, 2016). Plant species preferably being used for phytoextraction should not just accumulate significant concentrations of the target metals and metalloids; nevertheless, they also have an increased biomass yield, tolerate the toxic effects of metals and metalloids, should be adaptable to soil and climatic conditions, are resistant to insects and pathogens, and will also be suitable for cultivation (Ranieri et al., 2020). The effectiveness of phytoextraction is determined by two factors: yield and metal and metalloid concentrations (Cameselle and Pena, 2016). Thus, the uptake of metals and metalloids, which is the outcome of the two factors, can sometimes be positive or negative (Coumar et al., 2016). Based on this, we identify that neither research findings fulfill all of the aforementioned criteria. Nevertheless, one study found that, while biochar-amended metal-polluted soil enhanced the willow plant biomass, the concentrations of Cd and Zn in willow were constant. Nonetheless, phytoextraction is improved (Břendová et al., 2015). In practice, phytoextraction is frequently used in farmland soils to reduce hazardous metal and metalloid concentrations below soil quality standards, thereby improving soil environmental quality and ensuring food security (Sun et al., 2018).
Phytoextraction of heavy metal-polluted soils, including mine sludge, could take centuries. Hence, the pollutant limits of the target agricultural fields should be kept to a minimum for phytoextraction (Mani and Kumar, 2014). However, a hyperaccumulator has the potential to acquire elevated metal and metalloid concentrations, but its slow growth rate frequently limits its application (Ranieri et al., 2020). Energy and economical plants, including sunflower and rapeseed, are often used to retrieve Cd from farmland soils. Recently, biochar-assisted phytoextraction has been emerging rapidly and used in practice. Accordingly, biochar-assisted Brassica napus was used to retrieve Cd metal-polluted agricultural soil (Houben and Sonnet, 2015). Various plant species and biochar are often used in multi-metal-contaminated soils. Nevertheless, limited research has focused on the combined effect of biochar on phytoextraction of heavy metal-contaminated soils (Houben and Sonnet, 2015; Sun et al., 2018). Correspondingly, Amaranthus tricolor was subjected to biochar-assisted phytoextraction to treat Cd-polluted agricultural soils (Lu et al., 2015). So far, many research findings showed that adding biochar to plants considerably reduces heavy metal and metalloid bioavailability. Nevertheless, some plants necessitate elevated doses of bioavailable metals and metalloids to accumulate them. The advantages of biochar include improved contaminated soil physicochemical properties, increased microbial population and activities, and increased ability to enhance agriculture production (Lu et al., 2015; Ye et al., 2016). Hence, using biochar to remediate metal- and metalloid-polluted soils not only immobilizes them but also increases microbial population, lowering the environmental threat of heavy metals and metalloids in soils even further (Frankel et al., 2016).
Phytostabilization is another phytoremediation method that is widely used for the stabilization of metals and metalloids in mine sludges (Barbosa and Fernando, 2018). The revegetation approach reduces dispersion and erosion because plant roots stop leaching, which contributes significantly to the immobilization of metals and metalloids (Sarkar and Sadhukhan, 2022). Precipitation, complexation, metal electron reduction, and root adsorption are the potential phytostabilization mechanisms (Ma et al., 2016). Phytostabilization, as opposed to phytoextraction, is more concerned with metal and metalloid sequestration in the rhizosphere than in other plant tissues (Yan et al., 2020). Metals and metalloids are typically stabilized by applying soil amendments (such as biochar and compost) and microbes in situ, which improve metal immobilization and plant growth (Figure 3; Kumpiene et al., 2019).
Mining (e.g., coal, gold, copper, magnesite, bauxite, and iron mining) activities can degrade soil quality and structure and disturb biological systems and vegetation, thus leading to widespread soil pollution (Gabarrón et al., 2019). Heavy metal toxicity and elevated acidity of soil contaminated by mining activity reduce the revegetation possibilities of metal-polluted soils. Remediation of such metal-polluted soils can be accomplished through phytoremediation, a long-term and cost-effective rehabilitation approach that promotes revegetation to minimize the chances of contaminant transfer and land reclamation. However, these are difficult to accomplish in the absence of appropriate soil amendments (e.g., biochar) (Fellet et al., 2014). The biochar amalgamation with heavy metal-polluted soil may improve pH fertility and water-holding capacity, minimize the mobility of pollutants, and encourage revegetation (Kelly et al., 2014). Phytoremediation of mine sludge soil with biochar obtained from residues of orchard prune and organic manure at four distinct concentration levels (0, 1, 5, and 10%) demonstrated substantial benefits of biochar in revegetating plant species in metal- and metalloid-contaminated soils. Also, the bioavailability of Zn, Cd, and Pb reduced proportionally as the biochar content increased (Fellet et al., 2014).
One of the most important remedial technologies for heavy metal- and metalloid-polluted soils is biochar-blended bioremediation/phytoremediation. Biochar-stimulated phytore- mediation has a significant potential for immobilizing cationic heavy metals and metalloids in mine sludge soils and other metal-contaminated soils, especially those under high acidic conditions. Biochar can significantly decrease the bioavailability and leachability of cationic metals and metalloids in soils; enhance soil structure, physicochemical properties, fertility, and revegetation; and foster soil microbial populations. Nevertheless, since biochar appears to become less efficient in stabilizing highly harmful cationic metals and metalloids, which provide their mobility in soils, the implementation of biochar-aided phytoremediation is competent in attempting to resolve multi-metal-polluted soils. Furthermore, it is essential to select suitable biochar in order to develop a successful strategy for immobilizing anionic metals and metalloids initially through an in vitro approach. Moreover, more extensive research is required to assess the efficacy of biochar-amended bioremediation/phytoremediation of heavy metal-polluted soils. Scientific investigations should concentrate on the following important themes: (A) demonstrating the interrelations between raw materials used in pyrolysis, biochar physicochemical properties, and soil bioremediation/phytoremediation; (B) assessing the biochar consistency and its impacts on the transfer of metals and metalloids in mine sludge and metal-polluted soils in a field-level study; (C) knowing the mechanisms of biochar-influenced bioremediation/phytoremediation, particularly the interactions between biochar, microbial populations, plant roots, and soil particles.
Both authors listed have made a substantial, direct, and intellectual contribution to the work, and approved it for publication.
This work was carried out at the College of Resources and Environment, Southwest University, supported by the Fundamental Research Funds for the Central Universities (No. SWU 020010), Natural Science Foundation of Chongqing (No. cstc2021jcyj-msxmX0827), and Chongqing Returned Overseas Students’ Entrepreneurship and Innovation Support Program (No. cx2021001).
The authors declare that the research was conducted in the absence of any commercial or financial relationships that could be construed as a potential conflict of interest.
All claims expressed in this article are solely those of the authors and do not necessarily represent those of their affiliated organizations, or those of the publisher, the editors and the reviewers. Any product that may be evaluated in this article, or claim that may be made by its manufacturer, is not guaranteed or endorsed by the publisher.
Achal, V., Pan, X., and Zhang, D. (2011). Remediation of copper-contaminated soil by Kocuria flava CR1, based on microbially induced calcite precipitation. Ecol. Eng. 37, 1601–1605. doi: 10.1016/j.ecoleng.2011.06.008
Agegnehu, G., Srivastava, A. K., and Bird, M. I. (2017). The role of biochar and biochar-compost in improving soil quality and crop performance: a review. Appl. Soil Ecol. 119, 156–170. doi: 10.1016/j.apsoil.2017.06.008
Akcil, A., Erust, C., Ozdemiroglu, S., Fonti, V., and Beolchini, F. (2015). A review of approaches and techniques used in aquatic contaminated sediments: metal removal and stabilization by chemical and biotechnological processes. J. Clean. Prod. 86, 24–36. doi: 10.1016/j.jclepro.2014.08.009
Akhil, D., Lakshmi, D., Kartik, A., Vo, D.-V. N., Arun, J., and Gopinath, K. P. (2021). Production, characterization, activation and environmental applications of engineered biochar: a review. Environ. Chem. Lett. 19, 2261–2297. doi: 10.1007/s10311-020-01167-7
Albalasmeh, A., Gharaibeh, M. A., Mohawesh, O., Alajlouni, M., Quzaih, M., Masad, M., et al. (2020). Characterization and artificial neural networks modelling of methylene blue adsorption of biochar derived from agricultural residues: effect of biomass type, pyrolysis temperature, particle size. J. Saudi Chem. Soc. 24, 811–823. doi: 10.1016/j.jscs.2020.07.005
Alrashidi, S. H., Sallam, A. S., and Usman, A. R. (2020). Acid-modified and unmodified natural clay deposits for in situ immobilization and reducing phytoavailability of molybdenum in a sandy loam calcareous soil. Sustain 12:8203. doi: 10.3390/su12198203
Ambika, S., Kumar, M., Pisharody, L., Malhotra, M., Kumar, G., Sreedharan, V., et al. (2022). Modified biochar as a green adsorbent for removal of hexavalent chromium from various environmental matrices: mechanisms, methods, and prospects. Chem. Eng. J. 439:135716. doi: 10.1016/j.cej.2022.135716
Arif, I., Batool, M., and Schenk, P. M. (2020). Plant microbiome engineering: expected benefits for improved crop growth and resilience. Trends Biotechnol. 38, 1385–1396. doi: 10.1016/j.tibtech.2020.04.015
Awasthi, M. K., Sarsaiya, S., Wainaina, S., Rajendran, K., Awasthi, S. K., Liu, T., et al. (2021). Techno-economics and life-cycle assessment of biological and thermochemical treatment of bio-waste. Renew. Sustain. Energy Rev. 4:110837. doi: 10.1016/j.rser.2021.110837
Azam, M. (2016). Does environmental degradation shackle economic growth? A panel data investigation on 11 Asian countries. Renew. Sustain. Energy Rev. 65, 175–182. doi: 10.1016/j.rser.2016.06.087
Bandara, T., Herath, I., Kumarathilaka, P., Seneviratne, M., Seneviratne, G., Rajakaruna, N., et al. (2017). Role of woody biochar and fungal-bacterial co-inoculation on enzyme activity and metal immobilization in serpentine soil. J. Soils Sediments 17, 665–673. doi: 10.1007/s11368-015-1243-y
Barbosa, B., and Fernando, A. L. (2018). “Aided phytostabilization of mine waste,” in Bio-Geotechnologies for Mine Site Rehabilitation, eds S. K. Prasad, M. N. V. de Favas, and P. J. C. Maiti (Amsterdam: Elsevier), 147–157. doi: 10.1016/B978-0-12-812986-9.00009-9
Beesley, L., and Marmiroli, M. (2011). The immobilisation and retention of soluble arsenic, cadmium and zinc by biochar. Environ. Pollut. 159, 474–480. doi: 10.1016/j.envpol.2010.10.016
Beesley, L., Marmiroli, M., Pagano, L., Pigoni, V., Fellet, G., Fresno, T., et al. (2013). Biochar addition to an arsenic contaminated soil increases arsenic concentrations in the pore water but reduces uptake to tomato plants (Solanum lycopersicum L.). Sci. Total Environ. 454, 598–603. doi: 10.1016/j.scitotenv.2013.02.047
Breda, S., Chiesa, S., Freitas, R., Figueira, E., Becherini, F., Gobbo, L., et al. (2018). Biogeochemical dynamics and bioaccumulation processes in Manila clam: implications for biodiversity and ecosystem services in the Ria de Aveiro Lagoon. Estuar. Coast. Shelf Sci. 209, 136–148. doi: 10.1016/j.ecss.2018.04.029
Břendová, K., Tlustoš, P., and Száková, J. (2015). Biochar immobilizes cadmium and zinc and improves phytoextraction potential of willow plants on extremely contaminated soil. Plant Soil Environ. 61, 303–308. doi: 10.17221/181/2015-PSE
Buha, J., Mueller, N., Nowack, B., Ulrich, A., Losert, S., and Wang, J. (2014). Physical and chemical characterization of fly ashes from Swiss waste incineration plants and determination of the ash fraction in the nanometer range. Environ. Sci. Technol. 48, 4765–4773. doi: 10.1021/es4047582
Byrne, G., Dimitrov, D., Monostori, L., Teti, R., Van Houten, F., and Wertheim, R. (2018). Biologicalisation: biological transformation in manufacturing. CIRP J. Manufact. Sci. Technol. 21, 1–32. doi: 10.1016/j.cirpj.2018.03.003
Cameselle, C., and Pena, A. (2016). Enhanced electromigration and electro-osmosis for the remediation of an agricultural soil contaminated with multiple heavy metals. Process Saf. Environ. Prot. 104, 209–217. doi: 10.1016/j.psep.2016.09.002
Chen, D., Liu, W., Wang, Y., and Lu, P. (2022). Effect of biochar aging on the adsorption and stabilization of Pb in soil. J. Soils Sediments 22, 56–66. doi: 10.1016/j.ecoenv.2021.113107
Choppala, G., Bolan, N., Megharaj, M., Chen, Z., and Naidu, R. (2012). The influence of biochar and black carbon on reduction and bioavailability of chromate in soils. J. Environ. Qual. 41, 1175–1184. doi: 10.2134/jeq2011.0145
Coumar, M. V., Parihar, R., Dwivedi, A., Saha, J., Rajendiran, S., Dotaniya, M., et al. (2016). Impact of pigeon pea biochar on cadmium mobility in soil and transfer rate to leafy vegetable spinach. Environ. Monit. Assess. 188, 1–10. doi: 10.1007/s10661-015-5028-y
Das, S. K., Ghosh, G. K., Avasthe, R., and Sinha, K. (2021). Compositional heterogeneity of different biochar: effect of pyrolysis temperature and feedstocks. J. Environ. Manag. 278:111501. doi: 10.1016/j.jenvman.2020.111501
de Figueiredo, C. C., Reis, A. D. S. P. J., De Araujo, A. S., Blum, L. E. B., Shah, K., and Paz-Ferreiro, J. (2021). Assessing the potential of sewage sludge-derived biochar as a novel phosphorus fertilizer: influence of extractant solutions and pyrolysis temperatures. Waste Manag. 124, 144–153. doi: 10.1016/j.wasman.2021.01.044
Dong, H., Deng, J., Xie, Y., Zhang, C., Jiang, Z., Cheng, Y., et al. (2017). Stabilization of nanoscale zero-valent iron (nZVI) with modified biochar for Cr (VI) removal from aqueous solution. J. Hazard. Mater. 332, 79–86. doi: 10.1016/j.jhazmat.2017.03.002
Dutta, S., and Sharma, R. (2019). “Sustainable magnetically retrievable nanoadsorbents for selective removal of heavy metal ions from different charged wastewaters,” in Separation Science and Technology ed. S. Ahuja (Amsterdam: Elsevier), 371–416. doi: 10.1016/B978-0-12-815730-5.00015-6
El-Bassi, L., Azzaz, A. A., Jellali, S., Akrout, H., Marks, E. A., Ghimbeu, C. M., et al. (2021). Application of olive mill waste-based biochars in agriculture: impact on soil properties, enzymatic activities and tomato growth. Sci. Total Environ. 755:142531. doi: 10.1016/j.scitotenv.2020.142531
Embrandiri, A., Singh, R. P., Ibrahim, H. M., and Ramli, A. A. (2012). Land application of biomass residue generated from palm oil processing: its potential benefits and threats. Environtalists 32, 111–117. doi: 10.1007/s10669-011-9367-0
Fan, Q., Sun, J., Chu, L., Cui, L., Quan, G., Yan, J., et al. (2018). Effects of chemical oxidation on surface oxygen-containing functional groups and adsorption behavior of biochar. Chemosphere 207, 33–40. doi: 10.1016/j.chemosphere.2018.05.044
Fellet, G., Marmiroli, M., and Marchiol, L. (2014). Elements uptake by metal accumulator species grown on mine tailings amended with three types of biochar. Sci. Total Environ. 468, 598–608. doi: 10.1016/j.scitotenv.2013.08.072
Ferjani, A. I., Jeguirim, M., Jellali, S., Limousy, L., Courson, C., Akrout, H., et al. (2019). The use of exhausted grape marc to produce biofuels and biofertilizers: effect of pyrolysis temperatures on biochars properties. Renew. Sustain. Energy Rev. 107, 425–433. doi: 10.1016/j.rser.2019.03.034
Fijałkowska, G., Wiśniewska, M., Szewczuk-Karpisz, K., Jędruchniewicz, K., and Oleszczuk, P. (2021). Comparison of lead (II) ions accumulation and bioavailability on the montmorillonite and kaolinite surfaces in the presence of polyacrylamide soil flocculant. Chemosphere 276:130088. doi: 10.1016/j.chemosphere.2021.130088
Frankel, M. L., Bhuiyan, T. I., Veksha, A., Demeter, M. A., Layzell, D. B., Helleur, R. J., et al. (2016). Removal and biodegradation of naphthenic acids by biochar and attached environmental biofilms in the presence of co-contaminating metals. Bioresour. Technol. 216, 352–361. doi: 10.1016/j.biortech.2016.05.084
Gabarrón, M., Zornoza, R., Acosta, J. A., Faz, Á, and Martínez-Martínez, S. (2019). “Mining environments,” in Advances in Chemical Pollution, Environmental Management and Protection, ed. P. Pereira (Amsterdam: Elsevier), 157–205. doi: 10.1016/bs.apmp.2019.07.003
Gholizadeh, M., and Hu, X. (2021). Removal of heavy metals from soil with biochar composite: a critical review of the mechanism. J. Environ. Chem. Eng. 9:105830. doi: 10.1016/j.jhazmat.2019.03.080
Gogoi, L., Narzari, R., Chutia, R. S., Borkotoki, B., Gogoi, N., and Kataki, R. (2021). “Chapter Two - Remediation of heavy metal contaminated soil: role of biochar,” in Advances in Chemical Pollution, Environmental Management and Protection, ed. A. K. Sarmah (Amsterdam: Elsevier), 39–63. doi: 10.1016/bs.apmp.2021.08.002
Gong, X., Huang, D., Liu, Y., Zeng, G., Chen, S., Wang, R., et al. (2019). Biochar facilitated the phytoremediation of cadmium contaminated sediments: metal behavior, plant toxicity, and microbial activity. Sci. Total Environ. 666, 1126–1133. doi: 10.1016/j.scitotenv.2019.02.215
Gul, S., Whalen, J. K., Thomas, B. W., Sachdeva, V., and Deng, H. (2015). Physico-chemical properties and microbial responses in biochar-amended soils: mechanisms and future directions. Agric. Ecosyst. Environ. 206, 46–59. doi: 10.1016/j.agee.2015.03.015
Gupta, A., Sharma, V., Sharma, K., Kumar, V., Choudhary, S., Mankotia, P., et al. (2021). A review of adsorbents for heavy metal decontamination: growing approach to wastewater treatment. Materials 14:4702. doi: 10.3390/ma14164702
Hartley, W., Dickinson, N. M., Riby, P., and Lepp, N. W. (2009). Arsenic mobility in brownfield soils amended with green waste compost or biochar and planted with Miscanthus. Environ. Pollut. 157, 2654–2662. doi: 10.1016/j.envpol.2009.05.011
Heitkötter, J., and Marschner, B. (2015). Interactive effects of biochar ageing in soils related to feedstock, pyrolysis temperature, and historic charcoal production. Geoderma 245, 56–64. doi: 10.1016/j.geoderma.2015.01.012
Houben, D., and Sonnet, P. (2015). Impact of biochar and root-induced changes on metal dynamics in the rhizosphere of Agrostis capillaris and Lupinus albus. Chemosphere 139, 644–651. doi: 10.1016/j.chemosphere.2014.12.036
Hu, B., Ai, Y., Jin, J., Hayat, T., Alsaedi, A., Zhuang, L., et al. (2020). Efficient elimination of organic and inorganic pollutants by biochar and biochar-based materials. Biochar 2, 47–64. doi: 10.1007/s42773-020-00044-4
Karppinen, E. M., Stewart, K. J., Farrell, R. E., and Siciliano, S. D. (2017). Petroleum hydrocarbon remediation in frozen soil using a meat and bonemeal biochar plus fertilizer. Chemosphere 173, 330–339. doi: 10.1016/j.chemosphere.2017.01.016
Kelly, C. N., Peltz, C. D., Stanton, M., Rutherford, D. W., and Rostad, C. E. (2014). Biochar application to hardrock mine tailings: soil quality, microbial activity, and toxic element sorption. Appl. Geochem. 43, 35–48. doi: 10.1016/j.apgeochem.2014.02.003
Khan, S., Chao, C., Waqas, M., Arp, H. P. H., and Zhu, Y.-G. (2013). Sewage sludge biochar influence upon rice (Oryza sativa L) yield, metal bioaccumulation and greenhouse gas emissions from acidic paddy soil. Environ. Sci. Technol. 47, 8624–8632. doi: 10.1021/es400554x
Khiari, B., Jeguirim, M., Limousy, L., and Bennici, S. (2019). Biomass derived chars for energy applications. Renew. Sustain. Energy Rev. 108, 253–273. doi: 10.1016/j.rser.2019.03.057
Kumar, A., Joseph, S., Tsechansky, L., Privat, K., Schreiter, I. J., Schüth, C., et al. (2018). Biochar aging in contaminated soil promotes Zn immobilization due to changes in biochar surface structural and chemical properties. Sci. Total Environ. 626, 953–961. doi: 10.1016/j.scitotenv.2018.01.157
Kumpiene, J., Antelo, J., Brännvall, E., Carabante, I., Ek, K., Komárek, M., et al. (2019). In situ chemical stabilization of trace element-contaminated soil–Field demonstrations and barriers to transition from laboratory to the field–A review. Appl. Geochem. 100, 335–351. doi: 10.1016/j.apgeochem.2018.12.003
Lehmann, J., Rillig, M. C., Thies, J., Masiello, C. A., Hockaday, W. C., and Crowley, D. (2011). Biochar effects on soil biota–a review. Soil Biol. Biochem. 43, 1812–1836. doi: 10.1016/j.soilbio.2011.04.022
Li, C., Zhou, K., Qin, W., Tian, C., Qi, M., Yan, X., et al. (2019). A review on heavy metals contamination in soil: effects, sources, and remediation techniques. Soil Sediment Contam. Int. J. 28, 380–394. doi: 10.1080/15320383.2019.1592108
Lian, F., and Xing, B. (2017). Black carbon (biochar) in water/soil environments: molecular structure, sorption, stability, and potential risk. Environ. Sci. Technol. 51, 13517–13532. doi: 10.1021/acs.est.7b02528
Lu, H., Li, Z., Fu, S., Méndez, A., Gascó, G., and Paz-Ferreiro, J. (2015). Combining phytoextraction and biochar addition improves soil biochemical properties in a soil contaminated with Cd. Chemosphere 119, 209–216. doi: 10.1016/j.chemosphere.2014.06.024
Ma, Y., Oliveira, R. S., Freitas, H., and Zhang, C. (2016). Biochemical and molecular mechanisms of plant-microbe-metal interactions: relevance for phytoremediation. Front. Plant Sci. 7:918.
Ma, Z., Chen, D., Gu, J., Bao, B., and Zhang, Q. (2015). Determination of pyrolysis characteristics and kinetics of palm kernel shell using TGA–FTIR and model-free integral methods. Energy Convers. Manag. 89, 251–259. doi: 10.3389/fpls.2016.00918
Maddalwar, S., Kumar Nayak, K., Kumar, M., and Singh, L. (2021). Plant microbial fuel cell: opportunities, challenges, and prospects. Bioresour. Technol. 341:125772. doi: 10.1016/j.biortech.2021.125772
Mani, D., and Kumar, C. (2014). Biotechnological advances in bioremediation of heavy metals contaminated ecosystems: an overview with special reference to phytoremediation. Int. J. Environ. Sci. Technol. 11, 843–872. doi: 10.1007/s13762-013-0299-8
Muthusaravanan, S., Sivarajasekar, N., Vivek, J., Vasudha Priyadharshini, S., Paramasivan, T., Dhakal, N., et al. (2020). “Research updates on heavy metal phytoremediation: enhancements, efficient post-harvesting strategies and economic opportunities,” in Green Materials for Wastewater Treatment. Environmental Chemistry for a Sustainable World, Vol. 38, eds M. Naushad and E. Lichtfouse (Cham: Springer), 191–222. doi: 10.1007/978-3-030-17724-9_9
Naja, G. M., and Volesky, B. (2017). “Toxicity and sources of Pb, Cd, Hg, Cr, As, and radionuclides in the environment,” in Handbook of Advanced Industrial and Hazardous Wastes Management, eds L. K. Wang, M.-H. S. Wang, Y.-T. Hung, N. K. Shammas, and J. P. Chen (Boca Raton, FL: CRC Press), 855–903. doi: 10.1201/9781315117423-27
Park, J. H., Choppala, G. K., Bolan, N. S., Chung, J. W., and Chuasavathi, T. (2011). Biochar reduces the bioavailability and phytotoxicity of heavy metals. Plant Soil 348, 439–451. doi: 10.1007/s11104-011-0948-y
Ranieri, E., Moustakas, K., Barbafieri, M., Ranieri, A. C., Herrera-Melián, J. A., Petrella, A., et al. (2020). Phytoextraction technologies for mercury-and chromium-contaminated soil: a review. J. Chem. Technol. Biotechnol. 95, 317–327. doi: 10.1002/jctb.6008
Rathour, R., Kumar, H., Prasad, K., Anerao, P., Kumar, M., Kapley, A., et al. (2022). Multifunctional applications of bamboo crop beyond environmental management: an Indian prospective. Bioengineered 13, 8893–8914. doi: 10.1080/21655979.2022.2056689
Rezania, S., Taib, S. M., Din, M. F. M., Dahalan, F. A., and Kamyab, H. (2016). Comprehensive review on phytotechnology: heavy metals removal by diverse aquatic plants species from wastewater. J. Hazard. Mater. 318, 587–599. doi: 10.1016/j.jhazmat.2016.07.053
Rodriguez-Franco, C., and Page-Dumroese, D. S. (2021). Woody biochar potential for abandoned mine land restoration in the US: a review. Biochar 3, 7–22. doi: 10.1007/s42773-020-00074-y
Sarkar, A. K., and Sadhukhan, S. (2022). Bioremediation of salt-affected soil through plant-based strategies. Adv. Bioremed. Phytoremed. Sustain. Soil Manag. 81–100. doi: 10.1007/978-3-030-89984-4_5
Shah, V., and Daverey, A. (2020). Phytoremediation: a multidisciplinary approach to clean up heavy metal contaminated soil. Environ. Technol. Innov. 18:100774. doi: 10.1016/j.chemosphere.2022.134788
Sharma, R., Kumar, R., Hajam, Y. A., and Rani, R. (2022). “Chapter 21 - Role of biotechnology in phytoremediation,” in Phytoremediation, eds R. A. Bhat, F. M. P. Tonelli, G. H. Dar, and K. Hakeem (Cambridge, MA: Academic Press), 437–454. doi: 10.1016/B978-0-323-89874-4.00021-2
Spiertz, J., and Ewert, F. (2009). Crop production and resource use to meet the growing demand for food, feed and fuel: opportunities and constraints. NJAS Wageningen J. Life Sci. 56, 281–300. doi: 10.1016/S1573-5214(09)80001-8
Sun, W., Zhang, S., and Su, C. (2018). Impact of biochar on the bioremediation and phytoremediation of heavy metal (loid) s in soil. Adv. Bioremed. Phytoremed. 149. doi: 10.5772/intechopen.70349
Susilawati, A., Maftuah, E., and Fahmi, A. (2020). The utilization of agricultural waste as biochar for optimizing swampland: a review. IOP Conf. Ser. Mater. Sci. Eng. 980:012065. doi: 10.1088/1757-899X/980/1/012065
Uchimiya, M., Bannon, D. I., Wartelle, L. H., Lima, I. M., and Klasson, K. T. (2012). Lead retention by broiler litter biochars in small arms range soil: impact of pyrolysis temperature. J. Agric. Food Chem. 60, 5035–5044. doi: 10.1021/jf300825n
Verma, S., Bhatt, P., Verma, A., Mudila, H., Prasher, P., and Rene, E. R. (2021). Microbial technologies for heavy metal remediation: effect of process conditions and current practices. Clean Technol. Environ. Policy. doi: 10.1016/j.envint.2008.06.009
Villa-Achupallas, M., Rosado, D., Aguilar, S., and Galindo-Riaño, M. D. (2018). Water quality in the tropical Andes hotspot: the Yacuambi river (southeastern Ecuador). Sci. Total Environ. 633, 50–58. doi: 10.1016/j.scitotenv.2018.03.165
Wang, L., Rinklebe, J., Tack, F. M., and Hou, D. (2021). A review of green remediation strategies for heavy metal contaminated soil. Soil Use Manage. 37, 936–963. doi: 10.1111/sum.12717
Wang, X., Li, L., Yan, X., Meng, X., and Chen, Y. (2020). Processes of Chromium (VI) migration and transformation in chromate production site: a case study from the middle of China. Chemosphere 257:127282. doi: 10.1016/j.chemosphere.2020.127282
Williams, E. K., Jones, D. L., Sanders, H. R., Benitez, G. V., and Plante, A. F. (2019). Effects of 7 years of field weathering on biochar recalcitrance and solubility. Biochar 1, 237–248. doi: 10.1007/s42773-019-00026-1
Wu, C. H., Chang, S. H., and Lin, C. W. (2015). Improvement of oxygen release from calcium peroxide-polyvinyl alcohol beads by adding low-cost bamboo biochar and its application in bioremediation. Clean Soil Air Water 43, 287–295. doi: 10.1002/clen.201400059
Yan, A., Wang, Y., Tan, S. N., Mohd Yusof, M. L., Ghosh, S., and Chen, Z. (2020). Phytoremediation: a promising approach for revegetation of heavy metal-polluted land. Front. Plant Sci. 11:359. doi: 10.3389/fpls.2020.00359
Yang, X., Ng, W., Wong, B. S. E., Baeg, G. H., Wang, C.-H., and Ok, Y. S. (2019). Characterization and ecotoxicological investigation of biochar produced via slow pyrolysis: effect of feedstock composition and pyrolysis conditions. J. Hazard. Mater. 365, 178–185. doi: 10.1016/j.jhazmat.2018.10.047
Ye, J., Zhang, R., Nielsen, S., Joseph, S. D., Huang, D., and Thomas, T. (2016). A combination of biochar–mineral complexes and compost improves soil bacterial processes, soil quality, and plant properties. Front. Microbiol. 7:372. doi: 10.3389/fmicb.2016.00372
Yrjälä, K., and Lopez-Echartea, E. (2021). “Chapter Ten - Structure and function of biochar in remediation and as carrier of microbes,” in Advances in Chemical Pollution, Environmental Management and Protection, ed. A. K. Sarmah (Amsterdam: Elsevier), 263–294. doi: 10.1016/bs.apmp.2021.09.002
Yu, H., Zou, W., Chen, J., Chen, H., Yu, Z., Huang, J., et al. (2019). Biochar amendment improves crop production in problem soils: a review. J. Environ. Manage. 232, 8–21. doi: 10.1016/j.jenvman.2018.10.117
Yuan, P., Wang, J., Pan, Y., Shen, B., and Wu, C. (2019). Review of biochar for the management of contaminated soil: preparation, application and prospect. Sci. Total Environ. 659, 473–490. doi: 10.1016/j.scitotenv.2018.12.400
Keywords: heavy metals, biochar, plants, bioremediation, phytoremediation
Citation: Narayanan M and Ma Y (2022) Influences of Biochar on Bioremediation/Phytoremediation Potential of Metal-Contaminated Soils. Front. Microbiol. 13:929730. doi: 10.3389/fmicb.2022.929730
Received: 27 April 2022; Accepted: 16 May 2022;
Published: 09 June 2022.
Edited by:
Deniz Yildirim, Çukurova University, TurkeyReviewed by:
Lal Singh, National Environmental Engineering Research Institute (CSIR), IndiaCopyright © 2022 Narayanan and Ma. This is an open-access article distributed under the terms of the Creative Commons Attribution License (CC BY). The use, distribution or reproduction in other forums is permitted, provided the original author(s) and the copyright owner(s) are credited and that the original publication in this journal is cited, in accordance with accepted academic practice. No use, distribution or reproduction is permitted which does not comply with these terms.
*Correspondence: Ying Ma, Y2F0aHltYXlpbmdAaG90bWFpbC5jb20=
Disclaimer: All claims expressed in this article are solely those of the authors and do not necessarily represent those of their affiliated organizations, or those of the publisher, the editors and the reviewers. Any product that may be evaluated in this article or claim that may be made by its manufacturer is not guaranteed or endorsed by the publisher.
Research integrity at Frontiers
Learn more about the work of our research integrity team to safeguard the quality of each article we publish.