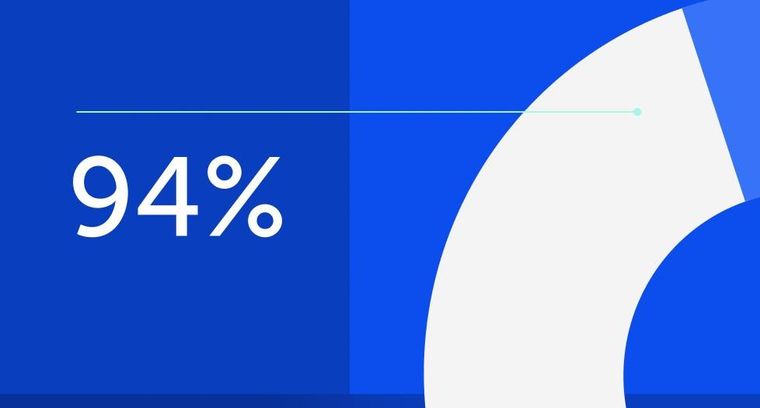
94% of researchers rate our articles as excellent or good
Learn more about the work of our research integrity team to safeguard the quality of each article we publish.
Find out more
REVIEW article
Front. Microbiol., 14 July 2022
Sec. Microorganisms in Vertebrate Digestive Systems
Volume 13 - 2022 | https://doi.org/10.3389/fmicb.2022.929346
This article is part of the Research TopicImpact of Gut Ecosystem in Health and Diseases: Microbiome, Mucosal Barrier and Cytokine MilieuView all 5 articles
The intestinal barrier is a structure that prevents harmful substances, such as bacteria and endotoxins, from penetrating the intestinal wall and entering human tissues, organs, and microcirculation. It can separate colonizing microbes from systemic tissues and prevent the invasion of pathogenic bacteria. Pathological conditions such as shock, trauma, stress, and inflammation damage the intestinal barrier to varying degrees, aggravating the primary disease. Intestinal probiotics are a type of active microorganisms beneficial to the health of the host and an essential element of human health. Reportedly, intestinal probiotics can affect the renewal of intestinal epithelial cells, and also make cell connections closer, increase the production of tight junction proteins and mucins, promote the development of the immune system, regulate the release of intestinal antimicrobial peptides, compete with pathogenic bacteria for nutrients and living space, and interact with the host and intestinal commensal flora to restore the intestinal barrier. In this review, we provide a comprehensive overview of how intestinal probiotics restore the intestinal barrier to provide new ideas for treating intestinal injury-related diseases.
The intestinal barrier is an important line of defense to maintain the homeostasis of the intestinal microenvironment and is divided into mechanical, chemical, immune, and microbial barriers, which interact to maintain intestinal homeostasis (Camilleri et al., 2012). Intestinal microbes are an essential part of the intestinal barrier and help maintain normal intestinal barrier function. These microbes are abundant in the host intestine, which they colonize in a symbiotic manner. Some intestinal microbes act as probiotics, that is, they are beneficial to the host’s health. The Food and Agriculture Organization of the United Nations and the WHO define probiotics as “live microorganisms, which when administered in adequate amounts confer a health benefit on the host” (Hill et al., 2014; Araya et al., 2015). Binda et al. (2020) proposed the minimum standards required to correctly use the term “probiotics.” Probiotics must have “strain characteristics, intended use safety, clinical trials, and the ability to survive at effective doses within the product’s shelf life.” They have numerous functions, including participating in food digestion, promoting intestinal motility, synthesizing vitamins, decomposing harmful substances, and enhancing the intestinal barrier (Vitetta et al., 2018; Rose et al., 2021; Tang et al., 2021). With the development of molecular biology, genetic engineering, fermentation culture, microfluidics, and experimental models in recent years, it has been possible to use single or compound probiotics to restore the intestinal barrier, providing a new clinical treatment option for intestinal barrier damage-related diseases.
The intestinal mechanical barrier comprises various intestinal epithelial cells and intercellular junction complexes differentiated from intestinal stem cells (ISCs) localized at the bottom of crypts (Capaldo et al., 2017; Ge et al., 2020). Probiotics can increase the expression of genes and proteins involved in tight junction (TJ) signaling, regulate the apoptosis of intestinal epithelial cells (IECs), and induce the proliferation of IECs to restore the intestinal mechanical barrier (Ohland and Macnaughton, 2010; Sharma et al., 2010; Ashida et al., 2011; La Fata et al., 2018).
The TJs between IECs can selectively transport substances, prevent pathogenic bacteria and harmful substances from entering the intestinal lumen, and maintain normal intestinal barrier function (Paradis et al., 2021). The TJ-related complex proteins of IECs are intracellular and membrane proteins. Some of the intracellular proteins are zonula occludens (ZO)1, ZO2, ZO3, and cingulin (Balda and Matter, 2000; Suzuki, 2020). Membrane proteins include a variety of transmembrane proteins in TJs, such as the occlusive protein (occludin), synaptic connexin (claudin), and junction adhesion molecules (JAMS; Colegio et al., 2003; Campbell et al., 2017). Probiotics affect the intestinal barrier function by regulating the expression of genes and proteins involved in TJ signaling in IECs (Table 1).
Table 1. List of probiotics that affect the tight junction to restore the intestinal mechanical barrier.
Ukena et al. (2007) found that when Escherichia coli Nissle1917 (EcN 1917) colonized the intestinal tract of germ-free mice, the gene and protein expression of the TJ molecule ZO1 increased, thereby increasing the TJ structure between IECs and decreasing intestinal permeability, which could improve intestinal epithelial barrier function. ZO1 expression also increased after transplanting EcN 1917 into colitis mice. However, this does not suggest that EcN 1917 has the same effect in the human intestinal environment, and the establishment and maintenance of the intestinal defense system may be a requirement for bacterial colonization and adhesion.
Lactobacillus reuteri maintains the integrity of the intestinal barrier by increasing the expression of TJ proteins in IECs. Wang et al. (2016) revealed that L. reuteri LR1 could mitigate enterotoxigenic E. coli (ETEC)-induced membrane barrier damage by maintaining the correct localization of ZO1 and inhibiting the destruction of ZO1 protein. However, Yi et al. (2018) discovered that L. reuteri LR1 may increase the gene and protein expression of the TJ molecules ZO1 and occludin through the myosin light chain kinase signaling pathway, thereby alleviating the damage of intestinal epithelial barrier integrity caused by ETEC K88 infection. Ahl et al. (2016) found that L. reuteri can also increase the expression of the TJ proteins, occludin and ZO1, strengthen the intestinal barrier, and ameliorate dextran sulfate sodium (DSS)-induced colitis. Tulyeu et al. (2019) revealed that L. reuteri could prevent the decrease of TJ proteins expression in IECs caused by ovalbumin sensitization and substantially increase the protein expression of ZO-1; occludin; and claudin1, 3, 5, 7, 8, 9, and 15 in rat IECs, thereby effectively ameliorating the intestinal mucosal barrier function.
Lactobacillus rhamnosus affects TJ protein expression to restore the intestinal mechanical barrier. Johnson-Henry et al. (2008) found that E. coli O157:H7 caused abnormal distribution of ZO1 and claudin1 in polarized epithelial cells, as well as a decrease in ZO1 expression, resulting in increased permeability and decreased barrier function in an in vitro cell model. They cultured L. rhamnosus GG with polarized epithelial cells to induce the redistribution of ZO1 and claudin1 and increased expression of ZO1, improving barrier function. The postbiotic HM0539 from L. rhamnosus GG can enhance the resistance of mice to E. coli O157:H7 infection by attenuating the destruction of TJ proteins (Zhang et al., 2020).
Lactobacillus plantarum MB452 increases the gene and protein expression of ZO1, ZO2, occludin, and cingulin and regulates the expression of TJ protein-degrading genes (such as itchy E3 ubiquitin protein ligase and snail family transcriptional regressor 1), which stabilizes TJs and improves intestinal barrier function (Anderson et al., 2010). Furthermore, the reduction in proteasome gene expression induced by L. plantarum MB452 may be an additional mechanism to enhance TJ integrity. Karczewski et al. (2010) found that L. plantarum WCFS1 increases the expression of occluding- and ZO1-coding genes. They injected L. plantarum WCFS1 strain into the duodenum of healthy subjects and found that TLR2 pathway activation affected the expression and distribution of TJ proteins. Another study revealed that L. plantarum regulates protein levels and distribution of claudin1, occludin, JAM1, and ZO1 in an in vitro model, protecting Caco-2 cells from enteroinvasive E. coli and improving intestinal barrier function (Qin et al., 2009).
Bifidobacterium infantis and Lactobacillus acidophilus normalize the expression of the TJ proteins, occludin and claudin1, in an in vitro Caco-2 intestinal epithelial cell model, preventing barrier damage due to IL-1 stimulation (Guo et al., 2017). Furthermore, In a neonatal mouse necrotizing enterocolitis (NEC) model, B. infantis modulates the proper localization of claudin 4 and occludin in TJs, attenuates intestinal permeability, protects intestinal barrier function, and reduces the incidence of NEC (Bergmann et al., 2013). Peng et al. (2019) revealed that Bacillus subtilis CW14 could mitigate the damage of intestinal epithelial cell microvilli and the TJ proteins, ZO1 and claudin1, caused by ochratoxin A, and maintain genome stability. L. acidophilus LA1 considerably enhances the intestinal TJ barrier mediated by a TLR2 heterodimeric complex. LA1 increases the expression of the TJ protein occludin, but also prevents DSS-induced downregulation of occludin expression in mouse intestinal tissues and enhances TJ structure (Al-Sadi et al., 2021).
In addition to a single strain that can restore the intestinal barrier and improve the intestinal barrier function, a mixture of probiotics can also restore the intestinal barrier. Zhao et al. (2021) confirmed that the probiotic mixture (B. infantis, L. acidophilus, Enterococcus, and Bacillus cereus) could enhance the expression of claudin-1 and occludin, by regulating the pregnane X receptor-c-Jun N-terminal kinase signaling pathway, and ameliorate intestinal barrier damage in neonatal necrotizing enterocolitis. Furthermore, co-culture of Saccharomyces boulardii CNCM I-745 with T84 cells in vitro revealed that S. boulardii altered the distribution of ZO1 and maintained the barrier function (Pontier-Bres et al., 2015).
Intestinal probiotics can regulate the apoptosis of IECs, promote the proliferation of IECs, mitigate intestinal damage, and restore the intestinal mechanical barrier (Odenwald and Turner, 2017; Alam and Neish, 2018). L. rhamnosus GG promotes the proliferation of IECs by secreting the protein p40. p40 upregulates disintegrin and metalloproteinase domain-containing protein 17 catalytic activity to phosphorylate epidermal growth factor receptor and activate the phosphatidylinositol 3-kinase (PI3K)/protein kinase B signaling pathway, reduces apoptosis, and preserves barrier function (Yan et al., 2013; Shen et al., 2018; Capurso, 2019). Hou et al. (2018) demonstrated that L. reuteri D8 stimulates lamina propria lymphocytes to secrete IL-22 via aryl hydrocarbon receptor, and then activates the phosphorylation of signal transducer and activator of transcription 3 (STAT3) to promote the proliferation of IECs and increase the growth of intestinal organs, thus recovering the intestinal mucosal barrier, which restores the structural damage to the intestinal epithelium caused by TNF treatment. In a mouse model of inflammatory bowel disease (IBD), continuous administration of B. subtilis during the remission period can maintain the integrity of the intestinal barrier by regulating the proliferation of IECs and alleviate IBD (Liu et al., 2021). Lactobacillus casei and Clostridium butyricum can also promote the proliferation of IECs and restore the intestinal barrier (Ichikawa et al., 1999).
The intestinal chemical barrier comprises mucin (MUC), antimicrobial peptides (AMPs), digestive fluids, lysozymes, mucopolysaccharides, other chemicals, and antimicrobial substances (Ren et al., 2019; Ge et al., 2020). Mucins are divided into transmembrane mucins and gel-forming mucins (Johansson and Hansson, 2016). The predominant membrane mucins in the intestine of humans and mice are MUC13 and MUC17 (Layunta et al., 2021), while MUC2 is the major secretory mucin in the gastrointestinal tract and is a major component of intestinal mucus (Tailford et al., 2015; Johansson and Hansson, 2016). MUC2 is the main component of the intestinal chemical barrier, covering IECs to form an intestinal mucus layer, which improves food absorption, provides attachment sites for intestinal symbiotic bacteria, and limits the combination of pathogens and IECs. AMPs are a type of polypeptide secreted by Paneth cells (Schoenborn et al., 2019) and have bactericidal, anti-inflammatory, immunity-improving, and tissue restoration-promoting effects (Bakshani et al., 2018). Several studies have shown that probiotics can regulate the expression of mucin, affect the formation of the mucus layer, and maintain the intestinal barrier function.
The increase in MUC2 expression by L. acidophilus A4 and its cell extracts significantly inhibited the attachment of E. coli O157:H7 to HT-29 IECs (Kim et al., 2008). Similar results were obtained when treating colorectal adenocarcinoma (Caco-2) cells with L. acidophilus LA1 (Bernet et al., 1994). In an in vitro model, L. casei GG increased the expression of MUC2 gene and protein to inhibit the translocation of specific pathogenic bacteria (Mattar et al., 2002). Caballero-Franco et al. (2007) revealed that the probiotic mixture VSL#3 (Lactobacillus, Bifidobacterium, and Streptococcus) increased the gene expression level of MUC1, MUC2, and MUC3.
Cazorla et al. (2018) found that the oral administration of L. casei CRL 43 and L. paracasei collection Nationale de cultures de microorganisms (CNCM) I-1518 to mice could increase the number of Paneth cells in the small intestine, and the AMPs released by Paneth cells could kill microorganisms, reduce the translocation of pathogens in the mucosa, and induce a barrier against intestinal infection. Ogawa et al. (2001) found that lactic acid bacteria and L. casei can also secrete acetic and lactic acids to reduce intestinal pH, inhibit the growth of pathogens, promote the balance of intestinal flora, and maintain the intestinal barrier function.
The intestinal immune barrier comprises gut-associated lymphoid tissue (GALT) and immune cells in the intestine. In the intestinal epithelial mucosa, propria contains Peyer’s node and immune cells from the innate and adaptive immune system, such as thymus (T) and bone marrow-or bursa-derived (B) cells, dendritic cells, and macrophages, together with the antibacterial peptides secreted by Paneth cells and secretory immunoglobulin A (IgA) from plasma cells. These participate in the immune defense mechanism of the intestinal barrier (Wells et al., 2017).
Probiotics can directly or indirectly regulate immune and anti-inflammatory functions. In vivo experiments in mice demonstrated that Lactobacillus can upregulate the expression of major histocompatibility complex II and the costimulatory cluster of differentiation (CD) 86, CD80, and CD40 and promote the maturation of dendritic cells (Drakes et al., 2004). B. infantis promotes the maturation of dendritic cells and accumulation of tolerogenic CD103 dendritic cells in GALT, which further regulates T-cell differentiation, induces anti-inflammatory factor expression, and improves intestinal mucosal immune response (Konieczna et al., 2013; Fu et al., 2017). EcN 1917 regulates T cell activation by affecting the T cell cycle and apoptosis to maintain intestinal immune homeostasis (Sturm et al., 2005). Jiang et al. (2019) found that L. reuteri induced macrophage activation in mice and enhanced macrophage phagocytosis to improve the immune function of the intestinal mucosa. L. plantarum 8,826 from the National Collection of Industrial Food and Marine Bacteria enhances the activity of T cell subsets and natural killer cells and increases the activity of the cytokine IL-10 in vitro (Dong et al., 2012). Kaushal and Kansal (2014) demonstrated that L. acidophilus and B. bifidum increased the phagocytic potential of aged mouse macrophages to improve immunity. A recent probiotic study showed that L. rhamnosus can reduce intestinal ischemia–reperfusion injury in mice by activating IL-10 release from macrophages through the TLR2 receptor signaling pathway (Hu et al., 2022).
Probiotics stimulate plasma cells to secrete IgA, but probiotic-induced IgA secretion may be strain-specific. Wang et al. (2019) reported that C. butyricum and Enterococcus faecalis increase the level of IgA in pig serum and improve immunity. Another study on probiotics found that after gavage of mice with E. faecalis CECT7121 increased intestinal mucosal IgA levels and enhanced local mucosal immune responses (Castro et al., 2016). El Hadad et al. (2019) showed that different concentrations of B. bifidum significantly stimulated the production of immune IgA in the intestinal mucosa of mice. Long-term consumption of fermented milk containing L. casei DN-114001 increased the amount of IgA in the large intestine and proved to be beneficial to the immune system of the intestinal mucosa (de Moreno de LeBlanc et al., 2008). S. boulardii was able to increase intestinal secretory IgA levels and modulate intestinal immunity in mice (Martins et al., 2009).
The metabolites of probiotics, such as short-chain fatty acids (SCFAs), can act on immune cells, such as mononuclear phagocytes and lymphocytes, affect the release of inflammatory factors and immune chemotaxis, and inhibit the proliferation of immune effector cells. Moreover, they can participate in immune regulation in the intestine (Kabat et al., 2014).
SCFAs can reduce the activity of the nuclear transcription factor NF-κB by inhibiting histone deacetylase; inhibit neutrophils and macrophages from releasing IL-8, tumor necrosis factor-α, and other inflammatory factors; and prevent chemotaxis of neutrophils to inflammation sites, thereby reducing intestinal inflammation (Tan et al., 2014). Kim et al. (2013) found that SCFAs activate mitogen kinase signaling through G protein-coupled receptors on intestinal epithelial cells, promote the production of immune factors, improve intestinal immune function in mice, and enhance intestinal barrier function.
The intestinal microbial barrier is a bacterial membrane barrier formed by the commensal gut microbiota tightly adhering to the surface of the intestinal epithelial mucosa (Kayama et al., 2020). Intestinal probiotics are an important part of the intestinal microbial barrier, which helps regulate the balance of the number and structure of intestinal microflora. Probiotics can compete with pathogens for nutrients, and through space barriers, they competitively inhibit the attachment sites of targeted cells or the spread of microcolonies to resist the invasion of pathogens (Corr et al., 2009; Hynönen and Palva, 2013; Ge et al., 2020).
L. rhamnosus and L. acidophilus can competitively attach to the adhesion sites of HEp-2 cells and T84 cells, reducing the adhesion sites on the cell surface and preventing the invasion of pathogenic microorganisms, such as EPEC (Sherman et al., 2005). EcN can secrete a non-bacteriocin component to act on pathogenic microorganisms or host cells to weaken the adhesion of pathogenic microorganisms (Smajs et al., 2012). Bacillus mesentericus, C. butyricum and E. faecalis increase the diversity and abundance of intestinal microbes and maintain the balance of intestinal flora (Chen et al., 2010).
L. plantarum increased the abundance of Bifidobacterium and Lactobacillus in the cecum of mice treated with cyclophosphamide and decreased the abundance of E. coli and Enterococcus (Meng et al., 2019). Additionally, the mixture of Lactobacillus fermentum GOS57 and L. plantarum GOS42 was able to reduce the number of Enterobacteriaceae, increase the abundance of Lactobacillus, adjust the balance of intestinal flora, and enhance the intestinal barrier function (Linninge et al., 2019). Lactobacillus rhamnosus and L. bulgaricus can increase the abundance of beneficial bacteria in the intestines, inhibit the growth of harmful bacteria, and help restore the imbalance of intestinal flora caused by antibiotics (Li et al., 2020). However, Li et al. (2019) revealed that the intestinal flora of children with repeated respiratory infections is imbalanced, where the number of Bifidobacterium and Lactobacillus significantly decreases, and the number of E. coli increases. The administration of Bifidobacterium quadruple live bacterial tablets can effectively increase the number of Bifidobacterium and Lactobacillus in infected children, thereby maintaining the balance of intestinal flora and reducing the incidence of infection.
Research on probiotics regarding the restoration of intestinal barrier function should be verified in human trials, which would lead to the development of treatments to improve the intestinal barrier, alleviate symptoms, and allow patients to recover quickly from disease. The safety of probiotics in humans needs to be prioritized, and although experiments in other animals and in vitro tests can further our understanding of the mechanisms of action, the human gut is rich in microbial species, the crosstalk between host and microbes is complex, and the physiology of non-human animals differs from that of humans. Therefore, animal and in vitro experiments are not sufficient for predicting the function of probiotic microorganisms in humans, and inferences from animal models and in vitro experiments cannot be extrapolated to humans (Hill et al., 2014; Abid and Koh, 2019; Suez et al., 2019). Common experimental models for probiotic studies and their advantages and limitations are listed in Table 2.
Although these experimental models cannot be extrapolated to humans, they can be used to study the mechanism of action of probiotic strains. The results of such experiments do have therapeutic value for disease models related to intestinal damage. Probiotics from such experiments are safe to use without side effects before clinical trials if they meet the expected therapeutic goals and are administered under clinic conditions. Recent studies have reported probiotics that positively affect intestinal barrier function (Bron et al., 2017; Rose et al., 2021), but the specific mechanisms still need to be elucidated. In the future, these models can be used to study the specific mechanisms of action of probiotics and the effect of single or mixed strains on certain intestinal immune cells, identify more strains that can improve intestinal barrier function, and reveal the biological properties of the strains.
After entering the intestinal tract, the effect of probiotics on intestinal barrier function is intricate, and the specific mechanism of action may vary depending on the strain. Furthermore, the beneficial effect may be a result of a combination of actions, which may be related to the enzymes or metabolites produced by specific strains. Previous research on the mechanism of action of probiotics has been relatively superficial, with much of the research limited to in vitro or animal experiments. Regarding intestinal barrier function, increasing evidence indicates that probiotics improve intestinal barrier function through TLR-like receptors (especially TLR2) and that probiotics can enhance host intestinal immunity through TLR-like receptor-related cytokines and signaling pathways (Castillo et al., 2011; Ryu et al., 2016; Paveljšek et al., 2021). In addition, myeloid differentiation factor (MyD88); nuclear factor-kappa-B (NF-κB); mitogen-activated protein kinase (MAPK); protein kinase C(PKC); protein PI3K; and the STAT, p38, and ERK1/2 signaling pathways may be related to intestinal barrier function (Patel and Lin, 2010; Thomas and Versalovic, 2010; Yousefi et al., 2019), and the effect of probiotics may result from the coordination of multiple signaling pathways.
For example, L. rhamnosus can stimulate GALT to induce an immune response, produce immune factors, and resist invasion by pathogenic microorganisms. Furthermore, L. rhamnosus and its effective components (surface-layer protein and exopolysaccharides) pass through TOLL-like receptors to mediate the regulation of NF-κB, MAPK, and extracellular signal-regulated kinase (ERK) signaling pathways to regulate intestinal cytokines (Good et al., 2014; Gao et al., 2017; Geng et al., 2021). Yang et al. (2021) found that L. plantarum may inhibit the activation of the p38 and ERK1/2 MAPK signaling pathways mediated by TLR-4 to combat the excessive activation of the innate immune response caused by ETEC K88. Lactobacillus delbrueckii CIDCA 133 improves 5-fluorouracil chemotherapy-induced mucositis by regulating inflammatory pathways through the TLR2/4/Myd88/NF-κB signaling pathway (Barroso et al., 2022). Bacillus enhances intestinal immune function and is associated with the TLR2/4/Myd88/NF-κB signaling pathway (Du et al., 2018). However, the specific immune mechanism requires further research.
Peroxisome proliferator activated receptor-gamma (PPAR-γ), a nuclear hormone receptor that can regulate intestinal inflammation, is mainly expressed in the colon and may be another target of probiotics to regulate the intestinal barrier (Dubuquoy et al., 2006; Marion-Letellier et al., 2009). Eun et al. (2007) reported that the inhibition of intestinal inflammatory mediator expression by L. casei may be associated with PPAR-γ activation. Another study found that the protective effect of probiotics on epithelial barrier function is dependent on PPAR-γ activation (Ewaschuk et al., 2007). However, there is still a paucity of studies on the relationship between probiotics and PPAR-γ.
Probiotics improve intestinal barrier function by not only inhibiting host intestinal inflammatory response but also altering the thickness, nature, and secretion of intestinal mucus. The composition of intestinal microorganisms affects the nature of intestinal mucus, and a possible mechanism is the expression of glycosyltransferases, which varies according to the type and number of strains. The entry of probiotics into the organism changes the composition of intestinal microorganisms, which can change the nature and increase the secretion of mucus (Paone and Cani, 2020). Reportedly, L. reuteri improves the intestinal barrier by increasing mucus thickness in a mouse model of colitis (Ahl et al., 2016). In addition, probiotics increase the expression and localization of TJ proteins and mucin-related genes.
Host intestinal epithelial cells form a structural interface that separates the lamina propria from the intestinal lumen. Therefore, the intestinal microorganisms in the lumen are in close contact with epithelial cells. The intestinal epithelium can distinguish between commensal and pathogenic microbiota through pattern recognition receptors that activate inflammation-related signaling pathways to resist pathogen invasion (O'Callaghan and Corr, 2019). The interaction between the microbiota in the intestinal lumen and intestinal epithelial cells and immune cells affects intestinal immunity. Microbiota interferes with bacterial adhesion, colonization, and invasion by affecting the expression of mucin genes in host goblet cells and stimulate the expression of antimicrobial peptides secreted by Paneth cells to affect intestinal immunity (Malago, 2015). Host and microbial interactions in the intestinal lumen maintain the homeostasis of the intestinal microenvironment.
Supplementation of probiotics may increase the number of certain microbes in the gut or metabolites of some specific strains that may improve intestinal barrier function by modulating intestinal immunity, TJ fraction, mucins, and changes in the intestinal microenvironment. These effects may be mediated by a crosstalk mechanism between probiotic and commensal bacteria. For example, Sugahara et al. (2015) supplemented human gut microbes in model mice with B. longum and found increased production of fecal pimelate, biotin, and butyrate, which may be caused by crosstalk between B. longum and the intestinal commensal microbiota.
Probiotics can compete with potentially pathogenic bacteria for nutrients and adhesion sites and inhibit their growth. As microbes establish a balanced microecosystem in the intestine, pathogenic bacteria must compete for binding sites and nutrients to survive. This competition between probiotics and pathogenic bacteria may reduce the possibility of colonization by pathogenic bacteria, reducing the chance of developing infectious diseases in the intestine (Bermudez-Brito et al., 2012; Stavropoulou and Bezirtzoglou, 2020). Furthermore, the metabolites of probiotics, such as SCFAs, can regulate intestinal pH, increase mucin gene expression (Burger-van Paassen et al., 2009), increase mucus production, and change the nature of mucus to prevent the adhesion of pathogenic bacteria. Fermentation products can also regulate intestinal immunity (Halloran and Underwood, 2019; Ratajczak et al., 2019), the specific mechanism of which may result from the combined action of the strains and their metabolites with intestinal immune cells or immune signaling pathways.
In summary, the intestinal barrier can separate substances from the intestinal cavity, prevent the invasion of pathogens, maintain the stability of the internal environment of the body, and protect the life and health of the human host. Probiotics can restore the intestinal mechanical, chemical, immune, and microbial barriers through various ways and maintain normal intestinal barrier function (Figure 1).
Figure 1. Probiotics regulate the intestinal barrier. Probiotics can increase the expression of tight junction-related genes and proteins, affect the apoptosis and proliferation of intestinal epithelial cells to restore the intestinal mechanical barrier, and restore the intestinal chemical barrier by increasing the expression of mucin and regulating intestinal pH. Probiotics also promote the maturation of immune cells, enhance the activity of immune cells, affect signal transduction pathways, and their metabolites promote the release of immune factors to restore the intestinal immune barrier. Probiotics restore the intestinal microbial barrier by regulating the balance of intestinal flora.
Presently, a myriad of studies has shown that probiotics can restore the intestinal barrier and treat intestinal injury-related diseases by enhancing TJs, increasing the expression of mucin, regulating the immune system, and inhibiting the adhesion of pathogenic bacteria. However, the mechanisms underlying the restoration of the intestinal barrier by probiotics have not been fully studied, and thus, more in-depth research is needed. Moreover, owing to the diversity of probiotics, limitations of the technology for extracting single probiotics, and complexity of the mechanism of compound probiotics, applying probiotics to the treatment of intestinal-related diseases by restoring the intestinal barrier faces ongoing challenges. With the development of biomarkers, genetic engineering, fermentation, separation, extraction technologies, and experimental design, researchers can further clarify the mechanism of probiotics at the genetic and molecular levels, make further breakthroughs in the screening, processing, and clinical application of probiotics, and develop more probiotics for the treatment of intestinal injury-related diseases.
All authors made significant contributions to the conception and design of the study, participated in the drafting of the article and in the critical revision of the intellectual content, and approved the final manuscript. All authors contributed to the article and approved the submitted version.
This study was supported by grants from the National Natural Science Foundation of China (31960236), Natural Science Foundation of Gansu Province (21JR7RA369), and the Talent Innovation and Entrepreneurship Project of Lanzhou City (2019-RC-34).
The authors declare that the research was conducted in the absence of any commercial or financial relationships that could be construed as a potential conflict of interest.
All claims expressed in this article are solely those of the authors and do not necessarily represent those of their affiliated organizations, or those of the publisher, the editors and the reviewers. Any product that may be evaluated in this article, or claim that may be made by its manufacturer, is not guaranteed or endorsed by the publisher.
We would like to thank Editage (www.editage.cn) for English language editing and Xiao-Jing Song for critically revising the manuscript.
Abid, M. B., and Koh, C. J. (2019). Probiotics in health and disease: fooling mother nature? Infection 47, 911–917. doi: 10.1007/s15010-019-01351-0
Ahl, D., Liu, H., Schreiber, O., Roos, S., Phillipson, M., and Holm, L. (2016). Lactobacillus reuteri increases mucus thickness and ameliorates dextran sulphate sodium-induced colitis in mice. Acta Physiol. 217, 300–310. doi: 10.1111/apha.12695
Alam, A., and Neish, A. (2018). Role of gut microbiota in intestinal wound healing and barrier function. Tissue Barriers 6:1539595. doi: 10.1080/21688370.2018.1539595
Al-Asmakh, M., and Zadjali, F. (2015). Use of germ-free animal models in microbiota-related research. J. Microbiol. Biotechnol. 25, 1583–1588. doi: 10.4014/jmb.1501.01039
Al-Sadi, R., Nighot, P., Nighot, M., Haque, M., Rawat, M., and Ma, T. Y. (2021). Lactobacillus acidophilus induces a strain-specific and toll-Like receptor 2-dependent enhancement of intestinal epithelial tight junction barrier and protection Against intestinal inflammation. Am. J. Pathol. 191, 872–884. doi: 10.1016/j.ajpath.2021.02.003
Anderson, R. C., Cookson, A. L., McNabb, W. C., Park, Z., McCann, M. J., Kelly, W. J., et al. (2010). Lactobacillus plantarum MB452 enhances the function of the intestinal barrier by increasing the expression levels of genes involved in tight junction formation. BMC Microbiol. 10:316. doi: 10.1186/1471-2180-10-316
Araya, M., Morelli, L., Reid, G., Sanders, M. E., and Embarek, P. B. (2015). Guidelines for the Evaluation of Probiotics in Food. London, ON, Canada: MDC Publishers Sdn Bhd.
Ashida, H., Ogawa, M., Kim, M., Mimuro, H., and Sasakawa, C. (2011). Bacteria and host interactions in the gut epithelial barrier. Nat. Chem. Biol. 8, 36–45. doi: 10.1038/nchembio.741
Bakshani, C. R., Morales-Garcia, A. L., Althaus, M., Wilcox, M. D., Pearson, J. P., Bythell, J. C., et al. (2018). Evolutionary conservation of the antimicrobial function of mucus: a first defence against infection. NPJ Biofilms Microbiom. 4:14. doi: 10.1038/s41522-018-0057-2
Balda, M. S., and Matter, K. (2000). Transmembrane proteins of tight junctions. Semin. Cell Dev. Biol. 11, 281–289. doi: 10.1006/scdb.2000.0177
Barroso, F. A. L., de Jesus, L. C. L., da Silva, T. F., Batista, V. L., Laguna, J., Coelho-Rocha, N. D., et al. (2022). Lactobacillus delbrueckii CIDCA 133 ameliorates chemotherapy-induced Mucositis by modulating epithelial barrier and TLR2/4/Myd88/NF-κB signaling pathway. Front. Microbiol. 13:858036. doi: 10.3389/fmicb.2022.858036
Bergmann, K. R., Liu, S. X., Tian, R., Kushnir, A., Turner, J. R., Li, H. L., et al. (2013). Bifidobacteria stabilize claudins at tight junctions and prevent intestinal barrier dysfunction in mouse necrotizing enterocolitis. Am. J. Pathol. 182, 1595–1606. doi: 10.1016/j.ajpath.2013.01.013
Bermudez-Brito, M., Plaza-Díaz, J., Muñoz-Quezada, S., Gómez-Llorente, C., and Gil, A. (2012). Probiotic mechanisms of action. Ann. Nutr. Metab. 61, 160–174. doi: 10.1159/000342079
Bernet, M. F., Brassart, D., Neeser, J. R., and Servin, A. L. (1994). Lactobacillus acidophilus LA 1 binds to cultured human intestinal cell lines and inhibits cell attachment and cell invasion by enterovirulent bacteria. Gut 35, 483–489. doi: 10.1136/gut.35.4.483
Binda, S., Hill, C., Johansen, E., Obis, D., Pot, B., Sanders, M. E., et al. (2020). Criteria to qualify microorganisms as "probiotic" in foods and dietary supplements. Front. Microbiol. 11:1662. doi: 10.3389/fmicb.2020.01662
Bron, P. A., Kleerebezem, M., Brummer, R. J., Cani, P. D., Mercenier, A., MacDonald, T. T., et al. (2017). Can probiotics modulate human disease by impacting intestinal barrier function? Br. J. Nutr. 117, 93–107. doi: 10.1017/s0007114516004037
Brosnahan, A. J., and Brown, D. R. (2012). Porcine IPEC-J2 intestinal epithelial cells in microbiological investigations. Vet. Microbiol. 156, 229–237. doi: 10.1016/j.vetmic.2011.10.017
Burger-van Paassen, N., Vincent, A., Puiman, P. J., van der Sluis, M., Bouma, J., Boehm, G., et al. (2009). The regulation of intestinal mucin MUC2 expression by short-chain fatty acids: implications for epithelial protection. Biochem. J. 420, 211–219. doi: 10.1042/bj20082222
Caballero-Franco, C., Keller, K., De Simone, C., and Chadee, K. (2007). The VSL#3 probiotic formula induces mucin gene expression and secretion in colonic epithelial cells. Am. J. Physiol. Gastrointest. Liver Physiol. 292, G315–G322. doi: 10.1152/ajpgi.00265.2006
Camilleri, M., Madsen, K., Spiller, R., Greenwood-Van Meerveld, B., and Verne, G. N. (2012). Intestinal barrier function in health and gastrointestinal disease. Neurogastroenterol. Motil. 24, 503–512. doi: 10.1111/j.1365-2982.2012.01921.x
Campbell, H. K., Maiers, J. L., and DeMali, K. A. (2017). Interplay between tight junctions and adherens junctions. Exp. Cell Res. 358, 39–44. doi: 10.1016/j.yexcr.2017.03.061
Capaldo, C. T., Powell, D. N., and Kalman, D. (2017). Layered defense: how mucus and tight junctions seal the intestinal barrier. J. Mol. Med. 95, 927–934. doi: 10.1007/s00109-017-1557-x
Capurso, L. (2019). Thirty years of Lactobacillus rhamnosus GG: a review. J. Clin. Gastroenterol. 53, S1–S41. doi: 10.1097/mcg.0000000000001170
Cario, E., Gerken, G., and Podolsky, D. K. (2004). Toll-like receptor 2 enhances ZO-1-associated intestinal epithelial barrier integrity via protein kinase C. Gastroenterology 127, 224–238. doi: 10.1053/j.gastro.2004.04.015
Castillo, N. A., Perdigón, G., and de Moreno de Leblanc, A. (2011). Oral administration of a probiotic Lactobacillus modulates cytokine production and TLR expression improving the immune response against Salmonella enterica serovar Typhimurium infection in mice. BMC Microbiol. 11:177. doi: 10.1186/1471-2180-11-177
Castro, M. S., Molina, M. A., Azpiroz, M. B., Díaz, A. M., Ponzio, R., Sparo, M. D., et al. (2016). Probiotic activity of Enterococcus faecalis CECT7121: effects on mucosal immunity and intestinal epithelial cells. J. Appl. Microbiol. 121, 1117–1129. doi: 10.1111/jam.13226
Cazorla, S. I., Maldonado-Galdeano, C., Weill, R., De Paula, J., and Perdigón, G. D. V. (2018). oral administration of probiotics increases Paneth cells and intestinal antimicrobial activity. Front. Microbiol. 9:736. doi: 10.3389/fmicb.2018.00736
Chen, C. C., Kong, M. S., Lai, M. W., Chao, H. C., Chang, K. W., Chen, S. Y., et al. (2010). Probiotics have clinical, microbiologic, and immunologic efficacy in acute infectious diarrhea. Pediatr. Infect. Dis. J. 29, 135–138. doi: 10.1097/inf.0b013e3181b530bf
Colegio, O. R., Van Itallie, C., Rahner, C., and Anderson, J. M. (2003). Claudin extracellular domains determine paracellular charge selectivity and resistance but not tight junction fibril architecture. Am. J. Physiol. Cell Physiol. 284, C1346–C1354. doi: 10.1152/ajpcell.00547.2002
Corr, S. C., Hill, C., and Gahan, C. G. (2009). Understanding the mechanisms by which probiotics inhibit gastrointestinal pathogens. Adv. Food Nutr. Res. 56, 1–15. doi: 10.1016/s1043-4526(08)00601-3
de Moreno de LeBlanc, A., Chaves, S., Carmuega, E., Weill, R., Antóine, J., and Perdigón, G. (2008). Effect of long-term continuous consumption of fermented milk containing probiotic bacteria on mucosal immunity and the activity of peritoneal macrophages. Immunobiology 213, 97–108. doi: 10.1016/j.imbio.2007.07.002
Dong, H., Rowland, I., and Yaqoob, P. (2012). Comparative effects of six probiotic strains on immune function in vitro. Br. J. Nutr. 108, 459–470. doi: 10.1017/s0007114511005824
Drakes, M., Blanchard, T., and Czinn, S. (2004). Bacterial probiotic modulation of dendritic cells. Infect. Immun. 72, 3299–3309. doi: 10.1128/iai.72.6.3299-3309.2004
Du, W., Xu, H., Mei, X., Cao, X., Gong, L., Wu, Y., et al. (2018). Probiotic Bacillus enhance the intestinal epithelial cell barrier and immune function of piglets. Benef. Microbes 9, 743–754. doi: 10.3920/bm2017.0142
Dubuquoy, L., Rousseaux, C., Thuru, X., Peyrin-Biroulet, L., Romano, O., Chavatte, P., et al. (2006). PPARgamma as a new therapeutic target in inflammatory bowel diseases. Gut 55, 1341–1349. doi: 10.1136/gut.2006.093484
El Hadad, S., Zakareya, A., Al-Hejin, A., Aldahlawi, A., and Alharbi, M. (2019). Sustaining exposure to high concentrations of bifidobacteria inhibits gene expression of Mouse's mucosal immunity. Heliyon 5:e02866. doi: 10.1016/j.heliyon.2019.e02866
Eun, C. S., Han, D. S., Lee, S. H., Jeon, Y. C., Sohn, J. H., Kim, Y. S., et al. (2007). Probiotics may reduce inflammation by enhancing peroxisome proliferator activated receptor gamma activation in HT-29 cells. Korean J. Gastroenterol. 49, 139–146.
Ewaschuk, J., Endersby, R., Thiel, D., Diaz, H., Backer, J., Ma, M., et al. (2007). Probiotic bacteria prevent hepatic damage and maintain colonic barrier function in a mouse model of sepsis. Hepatology 46, 841–850. doi: 10.1002/hep.21750
Fu, L., Song, J., Wang, C., Fu, S., and Wang, Y. (2017). Bifidobacterium infantis potentially alleviates shrimp Tropomyosin-induced allergy by Tolerogenic dendritic cell-dependent induction of regulatory T cells and alterations in gut microbiota. Front. Immunol. 8:1536. doi: 10.3389/fimmu.2017.01536
Gao, K., Wang, C., Liu, L., Dou, X., Liu, J., Yuan, L., et al. (2017). Immunomodulation and signaling mechanism of Lactobacillus rhamnosus GG and its components on porcine intestinal epithelial cells stimulated by lipopolysaccharide. J. Microbiol. Immunol. Infect. 50, 700–713. doi: 10.1016/j.jmii.2015.05.002
Ge, P., Luo, Y., Okoye, C. S., Chen, H., Liu, J., Zhang, G., et al. (2020). Intestinal barrier damage, systemic inflammatory response syndrome, and acute lung injury: A troublesome trio for acute pancreatitis. Biomed. Pharmacother. 132:110770. doi: 10.1016/j.biopha.2020.110770
Geng, T., He, F., Su, S., Sun, K., Zhao, L., Zhao, Y., et al. (2021). Probiotics Lactobacillus rhamnosus GG ATCC53103 and Lactobacillus plantarum JL01 induce cytokine alterations by the production of TCDA, DHA, and succinic and palmitic acids, and enhance immunity of weaned piglets. Res. Vet. Sci. 137, 56–67. doi: 10.1016/j.rvsc.2021.04.011
Good, M., Sodhi, C. P., Ozolek, J. A., Buck, R. H., Goehring, K. C., Thomas, D. L., et al. (2014). Lactobacillus rhamnosus HN001 decreases the severity of necrotizing enterocolitis in neonatal mice and preterm piglets: evidence in mice for a role of TLR9. Am. J. Physiol. Gastrointest. Liver Physiol. 306, G1021–G1032. doi: 10.1152/ajpgi.00452.2013
Guo, S., Gillingham, T., Guo, Y., Meng, D., Zhu, W., Walker, W. A., et al. (2017). Secretions of Bifidobacterium infantis and Lactobacillus acidophilus protect intestinal epithelial barrier function. J. Pediatr. Gastroenterol. Nutr. 64, 404–412. doi: 10.1097/mpg.0000000000001310
Halloran, K., and Underwood, M. A. (2019). Probiotic mechanisms of action. Early Hum. Dev. 135, 58–65. doi: 10.1016/j.earlhumdev.2019.05.010
Hasannejad-Bibalan, M., Mojtahedi, A., Eshaghi, M., Rohani, M., Pourshafie, M. R., and Talebi, M. (2020). The effect of selected Lactobacillus strains on dextran sulfate sodium-induced mouse colitis model. Acta Microbiol. Immunol. Hung. 67, 138–142. doi: 10.1556/030.2020.00834
Hill, C., Guarner, F., Reid, G., Gibson, G. R., Merenstein, D. J., Pot, B., et al. (2014). Expert consensus document. The international scientific Association for Probiotics and Prebiotics consensus statement on the scope and appropriate use of the term probiotic. Nat. Rev. Gastroenterol. Hepatol. 11, 506–514. doi: 10.1038/nrgastro.2014.66
Hou, Q., Ye, L., Liu, H., Huang, L., Yang, Q., Turner, J. R., et al. (2018). Lactobacillus accelerates ISCs regeneration to protect the integrity of intestinal mucosa through activation of STAT3 signaling pathway induced by LPLs secretion of IL-22. Cell Death Differ. 25, 1657–1670. doi: 10.1038/s41418-018-0070-2
Hu, J., Deng, F., Zhao, B., Lin, Z., Sun, Q., Yang, X., et al. (2022). Lactobacillus murinus alleviate intestinal ischemia/reperfusion injury through promoting the release of interleukin-10 from M2 macrophages via toll-like receptor 2 signaling. Microbiome 10:38. doi: 10.1186/s40168-022-01227-w
Huang, L., Cui, K., Mao, W., Du, Y., Yao, N., Li, Z., et al. (2020). Weissella cibaria attenuated LPS-induced dysfunction of intestinal epithelial barrier in a Caco-2 cell monolayer model. Front. Microbiol. 11:2039. doi: 10.3389/fmicb.2020.02039
Hynönen, U., and Palva, A. (2013). Lactobacillus surface layer proteins: structure, function and applications. Appl. Microbiol. Biotechnol. 97, 5225–5243. doi: 10.1007/s00253-013-4962-2
Ichikawa, H., Kuroiwa, T., Inagaki, A., Shineha, R., Nishihira, T., Satomi, S., et al. (1999). Probiotic bacteria stimulate gut epithelial cell proliferation in rat. Dig. Dis. Sci. 44, 2119–2123. doi: 10.1023/a:1026647024077
Jiang, P., Yang, W., Jin, Y., Huang, H., Shi, C., Jiang, Y., et al. (2019). Lactobacillus reuteri protects mice against Salmonella typhimurium challenge by activating macrophages to produce nitric oxide. Microb. Pathog. 137:103754. doi: 10.1016/j.micpath.2019.103754
Johansson, M. E., and Hansson, G. C. (2016). Immunological aspects of intestinal mucus and mucins. Nat. Rev. Immunol. 16, 639–649. doi: 10.1038/nri.2016.88
Johnson-Henry, K. C., Donato, K. A., Shen-Tu, G., Gordanpour, M., and Sherman, P. M. (2008). Lactobacillus rhamnosus strain GG prevents enterohemorrhagic Escherichia coli O157:H7-induced changes in epithelial barrier function. Infect. Immun. 76, 1340–1348. doi: 10.1128/iai.00778-07
Kabat, A. M., Srinivasan, N., and Maloy, K. J. (2014). Modulation of immune development and function by intestinal microbiota. Trends Immunol. 35, 507–517. doi: 10.1016/j.it.2014.07.010
Kahlert, S., Junnikkala, S., Renner, L., Hynönen, U., Hartig, R., Nossol, C., et al. (2016). Physiological concentration of exogenous lactate reduces Antimycin A triggered oxidative stress in intestinal epithelial cell line IPEC-1 and IPEC-J2 In vitro. PLoS One 11:e0153135. doi: 10.1371/journal.pone.0153135
Karczewski, J., Troost, F. J., Konings, I., Dekker, J., Kleerebezem, M., Brummer, R. J., et al. (2010). Regulation of human epithelial tight junction proteins by Lactobacillus plantarum in vivo and protective effects on the epithelial barrier. Am. J. Physiol. Gastrointest. Liver Physiol. 298, G851–G859. doi: 10.1152/ajpgi.00327.2009
Kaushal, D., and Kansal, V. K. (2014). Dahi containing Lactobacillus acidophilus and Bifidobacterium bifidum improves phagocytic potential of macrophages in aged mice. J. Food Sci. Technol. 51, 1147–1153. doi: 10.1007/s13197-012-0637-8
Kayama, H., Okumura, R., and Takeda, K. (2020). Interaction Between the microbiota, epithelia, and immune cells in the intestine. Annu. Rev. Immunol. 38, 23–48. doi: 10.1146/annurev-immunol-070119-115104
Kiesler, P., Fuss, I. J., and Strober, W. (2015). Experimental models of inflammatory bowel diseases. Cell. Mol. Gastroenterol. Hepatol. 1, 154–170. doi: 10.1016/j.jcmgh.2015.01.006
Kim, M. H., Kang, S. G., Park, J. H., Yanagisawa, M., and Kim, C. H. (2013). Short-chain fatty acids activate GPR41 and GPR43 on intestinal epithelial cells to promote inflammatory responses in mice. Gastroenterology 145, 396–406.e391-310. doi: 10.1053/j.gastro.2013.04.056
Kim, Y., Kim, S. H., Whang, K. Y., Kim, Y. J., and Oh, S. (2008). Inhibition of Escherichia coli O157:H7 attachment by interactions between lactic acid bacteria and intestinal epithelial cells. J. Microbiol. Biotechnol. 18, 1278–1285.
Konieczna, P., Ferstl, R., Ziegler, M., Frei, R., Nehrbass, D., Lauener, R. P., et al. (2013). Immunomodulation by Bifidobacterium infantis 35624 in the murine lamina propria requires retinoic acid-dependent and independent mechanisms. PLoS One 8:e62617. doi: 10.1371/journal.pone.0062617
Krishnan, M., Penrose, H. M., Shah, N. N., Marchelletta, R. R., and McCole, D. F. (2016). VSL#3 probiotic stimulates T-cell protein tyrosine phosphatase-mediated recovery of IFN-γ-induced intestinal epithelial barrier defects. Inflamm. Bowel Dis. 22, 2811–2823. doi: 10.1097/mib.0000000000000954
La Fata, G., Weber, P., and Mohajeri, M. H. (2018). Probiotics and the gut immune system: indirect regulation. Probiotics Antimicrob. Proteins 10, 11–21. doi: 10.1007/s12602-017-9322-6
Layunta, E., Jäverfelt, S., Dolan, B., Arike, L., and Pelaseyed, T. (2021). IL-22 promotes the formation of a MUC17 glycocalyx barrier in the postnatal small intestine during weaning. Cell Rep. 34:108757. doi: 10.1016/j.celrep.2021.108757
Li, C., Niu, Z., Zou, M., Liu, S., Wang, M., Gu, X., et al. (2020). Probiotics, prebiotics, and synbiotics regulate the intestinal microbiota differentially and restore the relative abundance of specific gut microorganisms. J. Dairy Sci. 103, 5816–5829. doi: 10.3168/jds.2019-18003
Li, K. L., Wang, B. Z., Li, Z. P., Li, Y. L., and Liang, J. J. (2019). Alterations of intestinal flora and the effects of probiotics in children with recurrent respiratory tract infection. World J. Pediatr. 15, 255–261. doi: 10.1007/s12519-019-00248-0
Linninge, C., Xu, J., Bahl, M. I., Ahrné, S., and Molin, G. (2019). Lactobacillus fermentum and Lactobacillus plantarum increased gut microbiota diversity and functionality, and mitigated Enterobacteriaceae, in a mouse model. Benef. Microbes 10, 413–424. doi: 10.3920/bm2018.0074
Liu, Y., Yin, F., Huang, L., Teng, H., Shen, T., and Qin, H. (2021). Long-term and continuous administration of Bacillus subtilis during remission effectively maintains the remission of inflammatory bowel disease by protecting intestinal integrity, regulating epithelial proliferation, and reshaping microbial structure and function. Food Funct. 12, 2201–2210. doi: 10.1039/d0fo02786c
Malago, J. J. (2015). Contribution of microbiota to the intestinal physicochemical barrier. Benef. Microbes 6, 295–311. doi: 10.3920/bm2014.0041
Marion-Letellier, R., Déchelotte, P., Iacucci, M., and Ghosh, S. (2009). Dietary modulation of peroxisome proliferator-activated receptor gamma. Gut 58, 586–593. doi: 10.1136/gut.2008.162859
Martins, F. S., Silva, A. A., Vieira, A. T., Barbosa, F. H., Arantes, R. M., Teixeira, M. M., et al. (2009). Comparative study of Bifidobacterium animalis, Escherichia coli, Lactobacillus casei and Saccharomyces boulardii probiotic properties. Arch. Microbiol. 191, 623–630. doi: 10.1007/s00203-009-0491-x
Mattar, A. F., Teitelbaum, D. H., Drongowski, R. A., Yongyi, F., Harmon, C. M., and Coran, A. G. (2002). Probiotics up-regulate MUC-2 mucin gene expression in a Caco-2 cell-culture model. Pediatr. Surg. Int. 18, 586–590. doi: 10.1007/s00383-002-0855-7
Meng, Y., Wang, J., Wang, Z., Zhang, G., Liu, L., Huo, G., et al. (2019). Lactobacillus plantarum KLDS1.0318 ameliorates impaired intestinal immunity and metabolic disorders in cyclophosphamide-treated mice. Front. Microbiol. 10:731. doi: 10.3389/fmicb.2019.00731
O'Callaghan, A. A., and Corr, S. C. (2019). Establishing boundaries: The relationship That exists between intestinal epithelial cells and gut-dwelling Bacteria. Microorganisms 7, 663–674. doi: 10.3390/microorganisms7120663
Odenwald, M. A., and Turner, J. R. (2017). The intestinal epithelial barrier: a therapeutic target? Nat. Rev. Gastroenterol. Hepatol. 14, 9–21. doi: 10.1038/nrgastro.2016.169
Ogawa, M., Shimizu, K., Nomoto, K., Tanaka, R., Hamabata, T., Yamasaki, S., et al. (2001). Inhibition of in vitro growth of Shiga toxin-producing Escherichia coli O157:H7 by probiotic Lactobacillus strains due to production of lactic acid. Int. J. Food Microbiol. 68, 135–140. doi: 10.1016/s0168-1605(01)00465-2
Oh, S. Y., Cho, K. A., Kang, J. L., Kim, K. H., and Woo, S. Y. (2014). Comparison of experimental mouse models of inflammatory bowel disease. Int. J. Mol. Med. 33, 333–340. doi: 10.3892/ijmm.2013.1569
Ohland, C. L., and Macnaughton, W. K. (2010). Probiotic bacteria and intestinal epithelial barrier function. Am. J. Physiol. Gastrointest. Liver Physiol. 298, G807–G819. doi: 10.1152/ajpgi.00243.2009
Paone, P., and Cani, P. D. (2020). Mucus barrier, mucins and gut microbiota: the expected slimy partners? Gut 69, 2232–2243. doi: 10.1136/gutjnl-2020-322260
Paradis, T., Bègue, H., Basmaciyan, L., Dalle, F., and Bon, F. (2021). Tight junctions as a key for pathogens invasion in intestinal epithelial cells. Int. J. Mol. Sci. 22, 2506–2525. doi: 10.3390/ijms22052506
Parthasarathy, G., and Mansfield, L. S. (2009). Recombinant interleukin-4 enhances Campylobacter jejuni invasion of intestinal pig epithelial cells (IPEC-1). Microb. Pathog. 47, 38–46. doi: 10.1016/j.micpath.2009.04.011
Patel, R. M., and Lin, P. W. (2010). Developmental biology of gut-probiotic interaction. Gut Microbes 1, 186–195. doi: 10.4161/gmic.1.3.12484
Paveljšek, D., Ivičak-Kocjan, K., Treven, P., Benčina, M., Jerala, R., and Rogelj, I. (2021). Distinctive probiotic features share common TLR2-dependent signalling in intestinal epithelial cells. Cell. Microbiol. 23:e13264. doi: 10.1111/cmi.13264
Peng, M., Liu, J., and Liang, Z. (2019). Probiotic Bacillus subtilis CW14 reduces disruption of the epithelial barrier and toxicity of ochratoxin A to Caco-2 cells. Food Chem. Toxicol. 126, 25–33. doi: 10.1016/j.fct.2019.02.009
Pontier-Bres, R., Rampal, P., Peyron, J. F., Munro, P., Lemichez, E., and Czerucka, D. (2015). The Saccharomyces boulardii CNCM I-745 strain shows protective effects against the B. anthracis LT toxin. Toxins 7, 4455–4467. doi: 10.3390/toxins7114455
Qin, H., Zhang, Z., Hang, X., and Jiang, Y. (2009). L. plantarum prevents enteroinvasive Escherichia coli-induced tight junction proteins changes in intestinal epithelial cells. BMC Microbiol. 9:63. doi: 10.1186/1471-2180-9-63
Ratajczak, W., Rył, A., Mizerski, A., Walczakiewicz, K., Sipak, O., and Laszczyńska, M. (2019). Immunomodulatory potential of gut microbiome-derived short-chain fatty acids (SCFAs). Acta Biochim. Pol. 66, 1–12. doi: 10.18388/abp.2018_2648
Ren, Z., Guo, C., Yu, S., Zhu, L., Wang, Y., Hu, H., et al. (2019). Progress in Mycotoxins affecting intestinal mucosal barrier function. Int. J. Mol. Sci. 20, 2777–2790. doi: 10.3390/ijms20112777
Ren, C., Zhang, Q., de Haan, B. J., Faas, M. M., Zhang, H., and de Vos, P. (2020). Protective effects of lactic acid bacteria on gut epithelial barrier dysfunction are toll like receptor 2 and protein kinase C dependent. Food Funct. 11, 1230–1234. doi: 10.1039/c9fo02933h
Rose, E. C., Odle, J., Blikslager, A. T., and Ziegler, A. L. (2021). Probiotics, prebiotics and epithelial tight junctions: A promising approach to modulate intestinal barrier function. Int. J. Mol. Sci. 22, 6729–6746. doi: 10.3390/ijms22136729
Ryu, S. H., Park, J. H., Choi, S. Y., Jeon, H. Y., Park, J. I., Kim, J. Y., et al. (2016). The probiotic Lactobacillus prevents Citrobacter rodentium-induced murine colitis in a TLR2-dependent manner. J. Microbiol. Biotechnol. 26, 1333–1340. doi: 10.4014/jmb.1602.02004
Schoenborn, A. A., von Furstenberg, R. J., Valsaraj, S., Hussain, F. S., Stein, M., Shanahan, M. T., et al. (2019). The enteric microbiota regulates jejunal Paneth cell number and function without impacting intestinal stem cells. Gut Microbes 10, 45–58. doi: 10.1080/19490976.2018.1474321
Sharma, R., Young, C., and Neu, J. (2010). Molecular modulation of intestinal epithelial barrier: contribution of microbiota. J. Biomed. Biotechnol. 2010:305879. doi: 10.1155/2010/305879
Shen, X., Liu, L., Peek, R. M., Acra, S. A., Moore, D. J., Wilson, K. T., et al. (2018). Supplementation of p40, a Lactobacillus rhamnosus GG-derived protein, in early life promotes epidermal growth factor receptor-dependent intestinal development and long-term health outcomes. Mucosal Immunol. 11, 1316–1328. doi: 10.1038/s41385-018-0034-3
Sherman, P. M., Johnson-Henry, K. C., Yeung, H. P., Ngo, P. S., Goulet, J., and Tompkins, T. A. (2005). Probiotics reduce enterohemorrhagic Escherichia coli O157:H7- and enteropathogenic E. coli O127:H6-induced changes in polarized T84 epithelial cell monolayers by reducing bacterial adhesion and cytoskeletal rearrangements. Infect. Immun. 73, 5183–5188. doi: 10.1128/iai.73.8.5183-5188.2005
Smajs, D., Bureš, J., Smarda, J., Chaloupková, E., Květina, J., Förstl, M., et al. (2012). Experimental administration of the probiotic Escherichia coli strain Nissle 1917 results in decreased diversity of E. coli strains in pigs. Curr. Microbiol. 64, 205–210. doi: 10.1007/s00284-011-0051-x
Stavropoulou, E., and Bezirtzoglou, E. (2020). Probiotics in medicine: a long debate. Front. Immunol. 11:2192. doi: 10.3389/fimmu.2020.02192
Sturm, A., Rilling, K., Baumgart, D. C., Gargas, K., Abou-Ghazalé, T., Raupach, B., et al. (2005). Escherichia coli Nissle 1917 distinctively modulates T-cell cycling and expansion via toll-like receptor 2 signaling. Infect. Immun. 73, 1452–1465. doi: 10.1128/iai.73.3.1452-1465.2005
Suez, J., Zmora, N., Segal, E., and Elinav, E. (2019). The pros, cons, and many unknowns of probiotics. Nat. Med. 25, 716–729. doi: 10.1038/s41591-019-0439-x
Sugahara, H., Odamaki, T., Fukuda, S., Kato, T., Xiao, J. Z., Abe, F., et al. (2015). Probiotic Bifidobacterium longum alters gut luminal metabolism through modification of the gut microbial community. Sci. Rep. 5:13548. doi: 10.1038/srep13548
Sun, H., Chow, E. C., Liu, S., Du, Y., and Pang, K. S. (2008). The Caco-2 cell monolayer: usefulness and limitations. Expert Opin. Drug Metab. Toxicol. 4, 395–411. doi: 10.1517/17425255.4.4.395
Suzuki, T. (2020). Regulation of the intestinal barrier by nutrients: The role of tight junctions. Anim. Sci. J. 91:e13357. doi: 10.1111/asj.13357
Tailford, L. E., Crost, E. H., Kavanaugh, D., and Juge, N. (2015). Mucin glycan foraging in the human gut microbiome. Front. Genet. 6:81. doi: 10.3389/fgene.2015.00081
Tan, J., McKenzie, C., Potamitis, M., Thorburn, A. N., Mackay, C. R., and Macia, L. (2014). The role of short-chain fatty acids in health and disease. Adv. Immunol. 121, 91–119. doi: 10.1016/b978-0-12-800100-4.00003-9
Tang, C., Kong, L., Shan, M., Lu, Z., and Lu, Y. (2021). Protective and ameliorating effects of probiotics against diet-induced obesity: a review. Food Res. Int. 147:110490. doi: 10.1016/j.foodres.2021.110490
Thomas, C. M., and Versalovic, J. (2010). Probiotics-host communication: modulation of signaling pathways in the intestine. Gut Microbes 1, 148–163. doi: 10.4161/gmic.1.3.11712
Truant, S., Bruyneel, E., Gouyer, V., De Wever, O., Pruvot, F. R., Mareel, M., et al. (2003). Requirement of both mucins and proteoglycans in cell-cell dissociation and invasiveness of colon carcinoma HT-29 cells. Int. J. Cancer 104, 683–694. doi: 10.1002/ijc.11011
Tulyeu, J., Kumagai, H., Jimbo, E., Watanabe, S., Yokoyama, K., Cui, L., et al. (2019). Probiotics prevents sensitization to Oral antigen and subsequent increases in intestinal tight junction permeability in juvenile-Young adult rats. Microorganisms 7, 463–482. doi: 10.3390/microorganisms7100463
Ukena, S. N., Singh, A., Dringenberg, U., Engelhardt, R., Seidler, U., Hansen, W., et al. (2007). Probiotic Escherichia coli Nissle 1917 inhibits leaky gut by enhancing mucosal integrity. PLoS One 2:e1308. doi: 10.1371/journal.pone.0001308
Vitetta, L., Vitetta, G., and Hall, S. (2018). Immunological tolerance and function: associations between intestinal Bacteria, probiotics, prebiotics, and phages. Front. Immunol. 9:2240. doi: 10.3389/fimmu.2018.02240
Wang, K., Chen, G., Cao, G., Xu, Y., Wang, Y., and Yang, C. (2019). Effects of clostridium butyricum and Enterococcus faecalis on growth performance, intestinal structure, and inflammation in lipopolysaccharide-challenged weaned piglets. J. Anim. Sci. 97, 4140–4151. doi: 10.1093/jas/skz235
Wang, Z., Wang, L., Chen, Z., Ma, X., Yang, X., Zhang, J., et al. (2016). In vitro evaluation of swine-derived Lactobacillus reuteri: probiotic properties and effects on intestinal porcine epithelial cells challenged with Enterotoxigenic Escherichia coli K88. J. Microbiol. Biotechnol. 26, 1018–1025. doi: 10.4014/jmb.1510.10089
Wells, J. M., Brummer, R. J., Derrien, M., MacDonald, T. T., Troost, F., Cani, P. D., et al. (2017). Homeostasis of the gut barrier and potential biomarkers. Am. J. Physiol. Gastrointest. Liver Physiol. 312, G171–G193. doi: 10.1152/ajpgi.00048.2015
Yan, F., Liu, L., Dempsey, P. J., Tsai, Y. H., Raines, E. W., Wilson, C. L., et al. (2013). A Lactobacillus rhamnosus GG-derived soluble protein, p40, stimulates ligand release from intestinal epithelial cells to transactivate epidermal growth factor receptor. J. Biol. Chem. 288, 30742–30751. doi: 10.1074/jbc.M113.492397
Yang, F., Wang, A., Zeng, X., Hou, C., Liu, H., and Qiao, S. (2015). Lactobacillus reuteri I5007 modulates tight junction protein expression in IPEC-J2 cells with LPS stimulation and in newborn piglets under normal conditions. BMC Microbiol. 15:32. doi: 10.1186/s12866-015-0372-1
Yang, K. M., Zhu, C., Wang, L., Cao, S. T., Yang, X. F., Gao, K. G., et al. (2021). Early supplementation with Lactobacillus plantarum in liquid diet modulates intestinal innate immunity through toll-like receptor 4-mediated mitogen-activated protein kinase signaling pathways in young piglets challenged with Escherichia coli K88. J. Anim. Sci. 99, 1–12. doi: 10.1093/jas/skab128
Yi, P., and Li, L. (2012). The germfree murine animal: an important animal model for research on the relationship between gut microbiota and the host. Vet. Microbiol. 157, 1–7. doi: 10.1016/j.vetmic.2011.10.024
Yi, H., Wang, L., Xiong, Y., Wang, Z., Qiu, Y., Wen, X., et al. (2018). Lactobacillus reuteri LR1 improved expression of genes of tight junction proteins via the MLCK pathway in IPEC-1 cells during infection with Enterotoxigenic Escherichia coli K88. Mediators Inflamm. 2018:6434910. doi: 10.1155/2018/6434910
Yousefi, B., Eslami, M., Ghasemian, A., Kokhaei, P., Salek Farrokhi, A., and Darabi, N. (2019). Probiotics importance and their immunomodulatory properties. J. Cell. Physiol. 234, 8008–8018. doi: 10.1002/jcp.27559
Zhang, H., Gao, J., He, X., Gong, Z., Wan, Y., Hu, T., et al. (2020). The postbiotic HM0539 from Lactobacillus rhamnosus GG prevents intestinal infection by enterohemorrhagic E. coli O157: H7 in mice. Nan Fang Yi Ke Da Xue Xue Bao 40, 211–218. doi: 10.12122/j.issn.1673-4254.2020.02.12
Keywords: intestinal barrier, probiotics, tight junctions, mucins, restore mechanism
Citation: Gou H-Z, Zhang Y-L, Ren L-F, Li Z-J and Zhang L (2022) How do intestinal probiotics restore the intestinal barrier? Front. Microbiol. 13:929346. doi: 10.3389/fmicb.2022.929346
Received: 26 April 2022; Accepted: 27 June 2022;
Published: 14 July 2022.
Edited by:
Akihito Harusato, Kyoto Prefectural University of Medicine, JapanReviewed by:
Thaher Pelaseyed, University of Gothenburg, SwedenCopyright © 2022 Gou, Zhang, Ren, Li and Zhang. This is an open-access article distributed under the terms of the Creative Commons Attribution License (CC BY). The use, distribution or reproduction in other forums is permitted, provided the original author(s) and the copyright owner(s) are credited and that the original publication in this journal is cited, in accordance with accepted academic practice. No use, distribution or reproduction is permitted which does not comply with these terms.
*Correspondence: Lei Zhang, MTM5OTMxODE2NDRAMTM5LmNvbQ==
†These authors have contributed equally to this work
Disclaimer: All claims expressed in this article are solely those of the authors and do not necessarily represent those of their affiliated organizations, or those of the publisher, the editors and the reviewers. Any product that may be evaluated in this article or claim that may be made by its manufacturer is not guaranteed or endorsed by the publisher.
Research integrity at Frontiers
Learn more about the work of our research integrity team to safeguard the quality of each article we publish.