- 1College of Food Science, South China Agricultural University, Guangzhou, China
- 2Guangdong Provincial Key Laboratory of Microbial Safety and Health, State Key Laboratory of Applied Microbiology Southern China, Institute of Microbiology, Guangdong Academy of Sciences, Guangzhou, China
- 3Key Laboratory of Agricultural Microbiomics and Precision Application, Ministry of Agriculture and Rural Affairs, Guangdong Academy of Sciences, Guangzhou, China
Accurate serotyping is essential for effective infection control. Pseudomonas aeruginosa serogroup G is one of the most common serogroups found in water. Conventional serotyping methods are not standardized and have several shortcomings. Therefore, a robust method for rapidly identifying P. aeruginosa serotypes is required. This study established a real-time PCR method for identifying P. aeruginosa serogroup G strains using novel target gene primers based on comparative genomic analysis. A total of 343 genome sequences, including 16 P. aeruginosa serogroups and 67 other species, were analyzed. Target genes identified were amplified using real-time PCR for detecting P. aeruginosa serogroup G strains. Eight serogroup G genes, PA59_01276, PA59_01887, PA59_01888, PA59_01891, PA59_01894, PA59_04268, PA59_01892, and PA59_01896, were analyzed to determine specific targets. A real-time fluorescence quantitative PCR method, based on the novel target PA59_01276, was established to detect and identify serogroup G strains. The specificity of this method was confirmed using P. aeruginosa serogroups and non-P. aeruginosa species. The sensitivity of this real-time PCR method was 4 × 102 CFU/mL, and it could differentiate and detect P. aeruginosa serogroup G in the range of 4.0 × 103–4.0 × 108 CFU/mL in artificially contaminated drinking water samples without enrichment. The sensitivity of these detection limits was higher by 1–3 folds compared to that of the previously reported PCR methods. In addition, the G serum group was accurately detected using this real-time PCR method without interference by high concentrations of artificially contaminated serum groups F and D. These results indicate that this method has high sensitivity and accuracy and is promising for identifying and rapidly detecting P. aeruginosa serogroup G in water samples. Moreover, this research will contribute to the development of effective vaccines and therapies for infections caused by multidrug-resistant P. aeruginosa.
Introduction
Pseudomonas aeruginosa (P. aeruginosa) is a widespread pathogen found in water. It is a versatile opportunistic pathogen that thrives in moist environments, such as soil and water, causing water-borne diseases and nosocomial infections (Falkinham et al., 2015). In recent decades, the number of P. aeruginosa-related water-borne illnesses has increased dramatically (Catho et al., 2021; Petitjean et al., 2021). P. aeruginosa infection may cause a variety of diseases, including those of the respiratory tract (predominantly cystic fibrosis), circulatory system (bacteremia and sepsis), central nervous system, heart (endocarditis), ears (including otitis external), eyes, bones, gastrointestinal tract, urinary tract, and skin (Carmeli et al., 2016; Lee et al., 2017; Morand and Morand, 2017). The epidemiology, virulence, and drug resistance of P. aeruginosa are closely related to its serotype (Ayi, 2015; Catho et al., 2021).
Serotyping is a phenotypic typing technique used for epidemiological sorting, clinical drug resistance analysis, disease transmission monitoring, and P. aeruginosa infection source tracing (Thrane et al., 2016; Del Barrio-Tofino et al., 2019). Based on the O-specific antigen (OSA, B-band), P. aeruginosa is classified into 14 serogroups (serogroups A-N), which correspond to the early international typing of O antigen serum (O1–O20) (Homma, 1982; Knirel et al., 2010; Lam et al., 2011; Zhao et al., 2018). There is no difference between the serogroups G and O6 in the reaction principle, classification, and scope of inclusion, except for the name (Riaz and Hashmi, 2019; Nasrin et al., 2022). Serogroup G, often present in food and water, is the most widespread serotype, and one of the five most frequently studied serotypes (Faure et al., 2003; Mena and Gerba, 2009; Koch et al., 2014; Ayi, 2015; Balabanova et al., 2020; Howlader et al., 2021). Serogroup G is considered the primary cause of burn wound infections, and it is one of the predominant P. aeruginosa serotypes among clinical isolates (Pirnay et al., 2002). A link exists between the clinical prevalence and O antigen serotype, such as among 1445 P. aeruginosa isolates analyzed from humans, 17.8% were identified as serogroup G (Qi et al., 2014; Del Barrio-Tofino et al., 2019). The P. aeruginosa serogroup G strain ST3449 shows resistance to multiple antibiotics and often leads to lung diseases such as cystic fibrosis and multiple drug resistant in infection in patients (Diaz-Rios et al., 2021). P. aeruginosa serogroup G is exceptionally resistant to antibiotics (over 90%), leading to a high mortality rate in burn victims (Nasrin et al., 2022). Rapid identification of the serogroup G strain is essential for early diagnosis.
Routine detection of P. aeruginosa serotypes is based on biochemical and slide agglutination tests. Determining the serotype of P. aeruginosa requires 5–8 days. In addition, slide agglutination has several drawbacks: it is time-consuming, labor-intensive, requires high-quality antiserum, and requires standardization of the antiserum (Koch et al., 2014; Allison and Castric, 2016; Thrane et al., 2016; Li et al., 2018). There are similarities between the different P. aeruginosa serotyping systems; however, the reason for the choice which serotyping method is not apparent. The conventional serotyping methods are not standard, and there are at least eight serotyping methods for P. aeruginosa. In addition, existing serotyping plans do not cover all serotypes of P. aeruginosa (Kaluzny et al., 2007; Kintz et al., 2008; Koch et al., 2014). A serotype kit for P. aeruginosa strains evaluated in which 37.5% of the strains were non-typeable (Le Berre et al., 2011). Serotyping is the most common approach for identifying P. aeruginosa strain; however, molecular typing and identification methods must be incorporated into epidemiological research for determining the relationship between the disease and the source of P. aeruginosa contamination.
Polymerase chain reaction is favored for pathogen detection because of its specificity, sensitivity, rapidity, and simplicity. Real-time PCR often used to ascertain product safety, quality, and authenticity. Several methods related to serum detection have also been reported, such as Real-time PCR method, which permits continuous monitoring of reaction progress, quantification of target DNA (Koch et al., 2014; Li et al., 2018). Nevertheless, these studies are restricted by incomplete strain information or the unavailability of a figure from an available public genomics library, resulting in a lack of detection targets. Therefore, identifying other OSA-related genes in the P. aeruginosa genome could be beneficial (Thrane et al., 2016).
The evolution of bioinformatics and whole-genome sequencing technology has enabled obtaining the genome sequence of P. aeruginosa with serogroup information; the sequences can be obtained from the National Biotechnology Information Center (NCBI1). Considering the research on vaccine efficacy and drug development and the shortcomings of traditional serotyping methods, a robust molecular typing method for the specific identification of P. aeruginosa serogroup G is essential. Therefore, we aimed to use comparative genomic analysis to identify new molecular targets for different P. aeruginosa serotypes and determine new serogroup G-specific molecular targets to establish a sensitive real-time PCR method for the rapid quantitative detection of P. aeruginosa serogroup G. Furthermore, the established methods were applied to the detection of actual water samples to provide a faster and efficient ways for the risk investigation of water pollution and provide a scientific basis for reducing pollution. The flowchart of the experimental method involved in this study is shown in Figure 1.
Materials and Methods
Mining of P. aeruginosa Serogroup G-Specific Targets
A total of 343 genome sequences, including those of 16 P. aeruginosa serotypes and 67 other species, were downloaded from the NCBI Genome database (see text footnote 1). Bacterial whole-genome sequences contain informative features of their evolutionary pathways, and accurately discriminate among populations, strains or closely related species. In this study, in order to ensure that the obtained target genes have excellent specificity, the sequences of selected genes need higher coverage and highly homologous with other genes. Of the 67 species used for analysis, 54 different species belong to the same pseudomonas genus, the remaining species are gram – negative and gram – positive representative species. For P. aeruginosa, 12,439 genomes were uploaded to NCBI, of which 142 genomes (contigs ≤ 200) carried serum-related information. Information regarding the Pseudomonas genomes is presented in Supplementary Table 1. Genome annotation was performed on all analyzed isolates using Prokka v1.11 (Seemann, 2014). The Prokka output was used to construct the pan-genome using Roary v3.11.2 (Page et al., 2015). A core genome was determined for each isolate using a 99% cutoff with a BLASTP identity cutoff of 85% (Pang et al., 2019). Genes that matched all P. aeruginosa serogroup G genomic sequences were considered highly conserved. They were used in the subsequent alignment of genomic sequences from other P. aeruginosa serotypes and other Pseudomonas species. Using Harvest v1.1.2 to generate the core genome alignment of P. aeruginosa with the ATCC33350 genome as a reference (Treangen et al., 2014). After remove the putative recombined regions, Genealogies Unbiased by recomBinations In Nucleotide Sequences (Gubbins) was used for recombination analysis (Li et al., 2022). Single nucleotide polymorphisms (SNPs) were extracted from the recombination-free core-genome alignment using the script available at https://github.com/sanger-pathogens/snp-sites. Based on the SNP alignment, FastTree v.2.1.10 with the general time-reversible (GTR) and gamma model of nucleotide substitution were used to construct a maximum-likelihood (ML) phylogenetic tree (Cheng et al., 2021). Using iTOL to visualize and annotate the ML phylogeny, and the results showed in Supplementary Figure 1 (Letunic and Bork, 2019). Specific genes were screened according to the following criteria: 100% presence in P. aeruginosa serogroup G strains and lack of presence in non-target P. aeruginosa strains. These candidate targets were then screened against the nucleotide collection (nr/nt) databases using the online BLAST program2 to ensure specificity.
Bacterial Strains and Genomic DNA Extraction
In total, 254 strains were isolated from water (using the Chinese National Standard method with some modifications, GB 8537-2016) and food samples (using the standard SN/T 5228.9-2019 with some modifications), including 222 P. aeruginosa strains belonging to 13 serotypes and 24 non-P. aeruginosa strains were used (Table 1). All bacterial strains were cultured in Luria-Bertani (LB) broth at 37°C. Bacterial cultures were collected via centrifugation at 12,000 × g for 5 min. Genomic DNA from these cells was extracted and purified using ENZ and a Bacterial Genome Kit (Omega Bio-Tek Inc., Norcross, GA, United States), as per the manufacturer’s instructions. The concentration and purity of DNA were estimated using agarose gel electrophoresis and a NanoDrop 2000c UV- is spectrophotometer (Thermo Fisher Scientific, Waltham, MA, United States). The genomic DNA was stored at −20°C until use.
Evaluation of the Specificity and Sensitivity of Candidate Target Genes
Primer Premier software (version 6.0) was used to design primers for species-specific targets of P. aeruginosa serogroup G. Primer sequences are listed in Table 2. The specificity of each primer was assessed against the bacterial sequences listed in Table 1. Each 20 μL PCR mixture consisted of 10 μL of 2 × Taq Master mix (Novoprotein Scientific, Shanghai, China), 0.5 μL of forward and reverse primers (10 μM), 2 μL of target DNA template, and sterile distilled water (to a final volume of 20 μL). The PCR conditions used were 98°C for 3 min, followed by 35 cycles of denaturation at 95°C for 30 s, annealing at 60°C for 30 s, elongation at 72°C for 1 min, and final elongation at 72°C for 10 min. The products were analyzed using 2% agarose gel electrophoresis and visualized using GoldView® staining (0.01%, v/v) under ultraviolet light.
Ten-fold serial dilutions of P. aeruginosa serogroup G strain PA206052 (108 to 101 CFU/mL) were subjected to DNA extraction as described above. For each dilution, 2 μL was used as a template for PCR amplification. The target gene with the highest detection limit was selected for further experiments.
Real-Time Polymerase Chain Reaction Conditions for the Detection of P. aeruginosa Serogroup G
The total reaction volume was 20 μL, including 10 μL of TB Green™ Premix Ex Taq™ II (TaKaRa, Biotech, Dalian, China), 1 μL each of forward and reverse primers (10 μM), 6 μL of sterile water, and 2 μL of purified bacterial genomic DNA as the template. A Light Cycler 96 System (Roche, Switzerland) was used for thermal cycling as follows: denaturation at 95°C for 60 s, followed by 40 cycles of denaturation at 95°C for 10 s, and annealing at 60°C for 30 s. Real-time PCR was performed in triplicate, with parallel analysis in 96-well plates. The DNA template was substituted with sterile water in the negative controls to ensure the absence of contaminants.
Evaluation of Real-Time Polymerase Chain Reaction Specificity and Co-infection-Related Interference
Genomic DNA from 13 P. aeruginosa strains and 15 other bacterial strains was used as a template for real-time PCR analysis to evaluate the specificity of the assay (Supplementary Table 4). To further assess the potential interference caused by co-infection, P. aeruginosa serogroups D and F (interfering bacteria) were mixed with the sample. The target serogroup G was cultured for 12 h, and the initial concentration of each bacterial suspension was determined using the plate count method. The concentration of the target bacterium, serogroup G, was adjusted to 104 CFU/mL, and the interfering bacteria were diluted to 108–101 CFU/ml. The cultures of P. aeruginosa serogroup G cultures were mixed with those of the other serotypes at ratios (D or F to G) of 104:1, 103:1, 102:1, 10:1, 1:1, 1:10, 1:102, and 1:103. Purified DNA was extracted from the mixtures, as described in section “Mining of P. aeruginosa Serogroup G-Specific Targets,” and used as a template for real-time PCR.
Detection of P. aeruginosa Serogroup G in Artificially Inoculated Bottled Drinking Water
Bottled drinking water samples, purchased from a local supermarket, autoclaved at 121°C/0.1 MPa for 15 min, were negative for P. aeruginosa, as assessed using the traditional culture method (Marei, 2020). Briefly, 1 mL of each bottled drinking water sample was added to 9 mL of saline solution to obtain the matrix. Different concentrations of the PA206052 culture were inoculated into the matrix, yielding final bacterial concentrations ranging from 4.0 × 108 CFU/mL to 4.0 × 101 CFU/ml. Subsequently, 1 ml of the suspension containing the strain PA206052 strain collected from each sample was subjected to DNA extraction. Genomic DNA (50 ng) was subsequently used for PCR and real-time PCR analysis. The amplification systems and procedures are described in Sections “Evaluation of the Specificity and Sensitivity of Candidate Target Genes” and “Real-time Polymerase Chain Reaction Conditions for the Detection of P. aeruginosa Serogroup G.”
Testing of Natural Water Samples
Thirty-seven aquatic samples (from the surrounding living environment and random from tributaries of the Pearl River Basin) were collected to validate the efficacy of the real-time PCR method. The water samples were tested for the presence of P. aeruginosa serogroup G using the traditional culture method and the slide agglutination method using P. aeruginosa antisera (Denka Seiken, Tokyo, Japan). P. aeruginosa contamination in water is usually low; therefore, an enrichment procedure was employed before PCR analysis. Briefly, a water sample (250 mL) was filtered through a 0.45 μm membrane (Millipore Co., Billerica, MA, United States) in a stainless-steel multi-line filter system (Huankai Co., Guangzhou, China). The membrane was placed in LB broth at 37°C for 12 h. Whole-cell DNA was extracted using a bacterial genomic DNA purification kit (Omega Bio-Tek Inc., Norcross, GA, United States), according to the manufacturer’s instructions. For each sample, 1 mL of the LB enrichment culture was collected. Genomic DNA was extracted from the LB enrichment cultures for PCR and real-time PCR assessment.
Results
Mining for P. aeruginosa Serogroup G-Specific Target Sequences
A total of 343 P. aeruginosa strain genome assemblies were downloaded from the NCBI Genome bank (last accessed on January 31, 2022). The complete genome (GCA_009497675.1_ASM949767v1) of P. aeruginosa serogroup G, PA59 strain (CP024630.1), retrieved from the GenBank database was used as a reference sequence. Candidate serogroup G-specific target sequences were obtained via pan-genome analysis the P. aeruginosa strain sequences. All candidate target sequences were further searched against the NCBI databases using the online BLAST program based on sequence similarity with phylogenetically connected or aloof species (Supplementary Figure 1). Fourteen candidate serogroup G-specific targets existed only in serogroup G strains (Supplementary Table 2); the nucleic acid sequences of the targets are shown in Supplementary Table 2. One of gene, PA_01889, has been reported in the literature (Li et al., 2018). When whole genome sequence was used to mine G-specific target genes, the number of interspecific gene sequences with low homology of P. aeruginosa may have little effect on the number of serum-specific targets obtained (Supplementary Table 5).
Screening Specific Gene Targets for P. aeruginosa Serogroup G
The specificity of the target sequences that were obtained through comparative genomic analysis was tested via PCR. Primers with specific target genes determined by experiments are shown in Table 2. The specific primers are G-PCR-1, G-PCR-4, G-PCR-5, G-PCR-7, G-PCR-9, G-PCR-10, G-PCR-13, and G-PCR-14. The novel specificity genes targets, PA59_01276, PA59_01887, PA59_01888, PA59_01891, PA59_01894, PA59_04268, PA59_01892, and PA59_01896, for all 18 P. aeruginosa serogroup G strains were detected at 100 and 100% exclusivity for the 204 other serogroups strains of P. aeruginosa and 24 other species (Table 1).
Four of these genes encode known proteins: one gene PA59_01887 (wzzB) codes Chain length determinant protein, one gene PA59_01888 (wbpA) regulates UDP-N-acetyl-D-glucosamine 6-dehydrogenase, one gene PA59_01884 (epsF_4) related to the Putative glycosyltransferase EpsF operation, and the gene PA59_01889 (gun) N-acetyl-alpha-D-glucosaminyl-diphospho-ditrans, octacis-undecaprenol 4-epimerase. The remaining four genes: PA59_01891 (group_234447), PA59_01891 (group_40682), PA59_01891 (group_71614), PA59_04268 (group_234710), encode unknown protein (Supplementary Table 2).
Target Gene Sensitivity Evaluation
The sensitivity of the specific genes was further verified by PCR amplification using P. aeruginosa serogroup G strain PA 206052. The lower limit of PCR detection ranged between 103 and 105 CFU/mL for pure culture (Supplementary Table 3). However, when the same concentration of serogroup G genomic DNA was used as a template for PCR amplification, the bands that were amplified using with the PA59_01276 primers were brighter than those that were amplified using with the other primers. Based on these results, combine with the LOD and product size results, we chose targeting gene PA59_01276 set for our real-time PCR assay for P. aeruginosa serogroup G detection. Thus, the PA59_01276 primer set was chosen for further experiments. We established a real-time PCR approach; the linear regression equation was y = −2.8718x + 45.515 (R2 = 0.9914), and the detection limit for pure P. aeruginosa serogroup G was 102 CFU/mL (Figure 2).
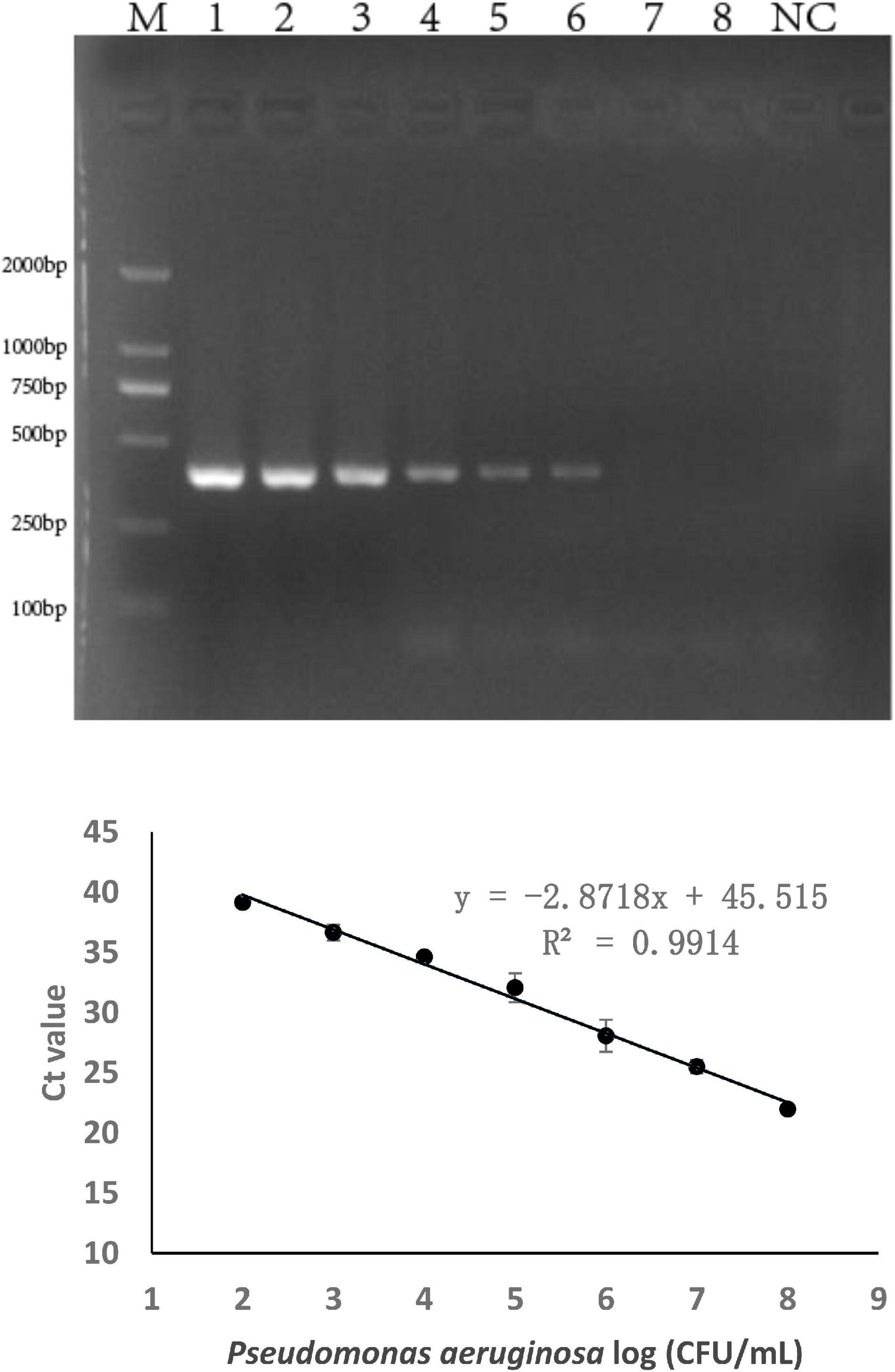
Figure 2. PCR detection sensitivity using primers for P. aeruginosa serogroup G-specific genes. 2.1: Amplification using PA59_01276 primers. Lane M: DL DNA 2000 marker, lane NC: negative control, lanes 1–8: Ten-fold sample dilutions (4.0 × 108 CFU/mL to 4.0 × 101 CFU/mL). 2.2: Establishment of a standard curve for the detection of P. aeruginosa serogroup G. Plots of Ct values against the log numbers of P. aeruginosa (4.0 × 102 CFU/mL–4.0 × 108 CFU/mL) in pure culture.
Specificity and Anti-interference Detection Using the Real-Time Polymerase Chain Reaction Assay
The specificity of the real-time PCR method based on PA59_01276 primers was checked in 13 P. aeruginosa and 16 other bacterial species (Supplementary Table 4). DNA amplification showed exclusivity for P. aeruginosa serogroup G. To further assess the precision of its susceptibility and interference, P. aeruginosa serogroup G strain PA206052 was mixed with other serotypes at various proportions. All amplifications showed near cycle threshold (Ct) values (Figure 3), irrespective of the target to interfering strain proportion, indicating that the presence of serotypes F and D did not interfere with serogroup G detection.
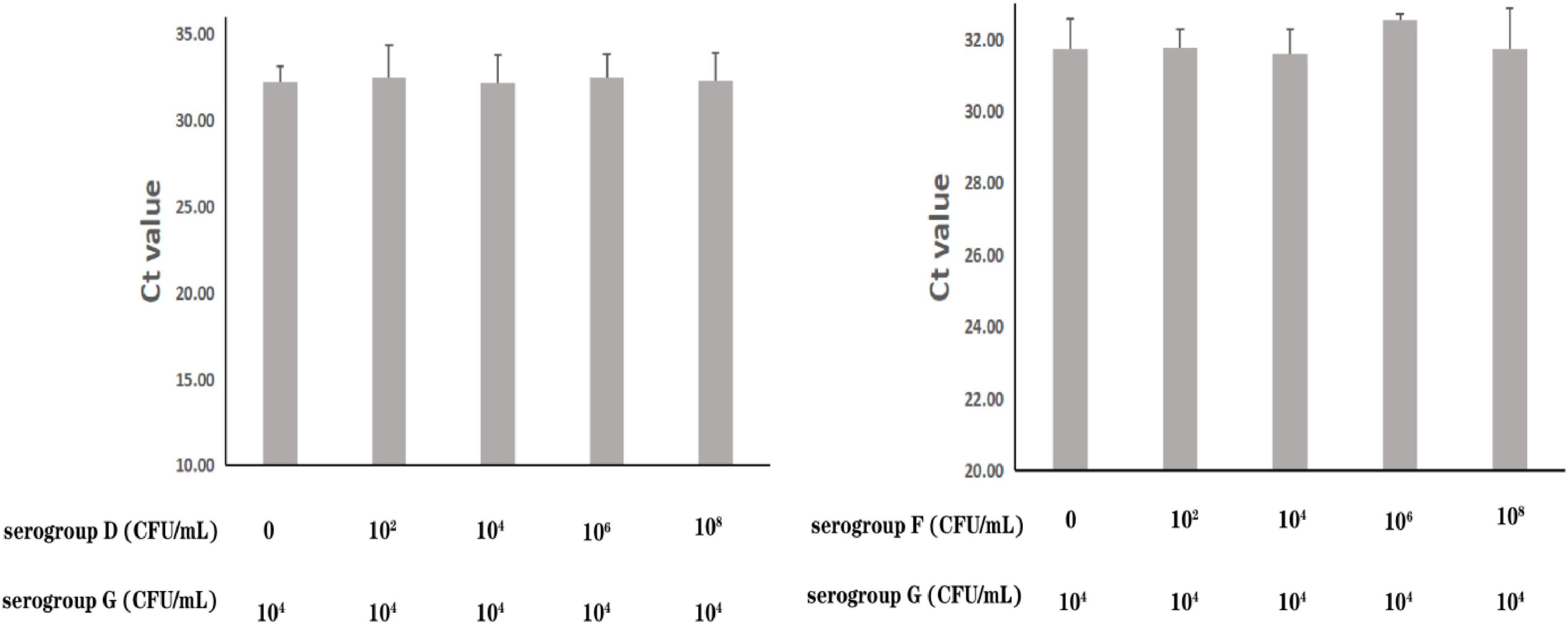
Figure 3. Assessment of interference with the real-time PCR-based detection of P. aeruginosa serogroup G by co-infection with other serogroups. Detection of P. aeruginosa serogroup G (4.0 × 104 CFU/mL) in the presence of P. aeruginosa serogroup D (0, 4.7 × 102 CFU/mL, 4.7 × 104 CFU/mL, 4.7 × 106 CFU/mL, 4.7 × 108 CFU/mL, respectively) (3.1) and serogroup F (0, 2.4 × 102 CFU/mL, 2.4 × 104 CFU/mL, 2.4 × 106 CFU/mL, 2.4 × 108 CFU/mL, respectively) (3.2).
Detection of P. aeruginosa Serogroup G in Artificially Contaminated Bottled Drinking Water via Polymerase Chain Reaction and Real-Time Polymerase Chain Reaction
The methods were applied to the detection of P. aeruginosa serogroup G in artificially contaminated bottled drinking water samples. P. aeruginosa (4.0 × 108 to 4.0 × 101 CFU/mL) was added to the sample, and real-time PCR and end-point PCR were used to detect serogroup G in the spiked samples. As shown in Figure 4, the detection limit in the artificially bottled drinking water samples was 4.0 × 104 CFU/mL, detected using end-point PCR. The real-time PCR detection conditions were further optimized to establish a standard curve of detection quantity of the P. aeruginosa G serum group. The linear detection range of this method was 4.0 × 108 CFU/mL to 4.0 × 103 CFU/mL (Figure 4), and the linear regression equation was y = −2.9846x + 49.195 (R2 = 0.9852). The limit of detection (LOD) of the novel target-based real-time PCR assay was calculated as 4.0 × 103 CFU/mL for the P. aeruginosa serogroup G. In comparison with the end-point PCR approach, and the real-time PCR approach was more sensitive by order of magnitude.
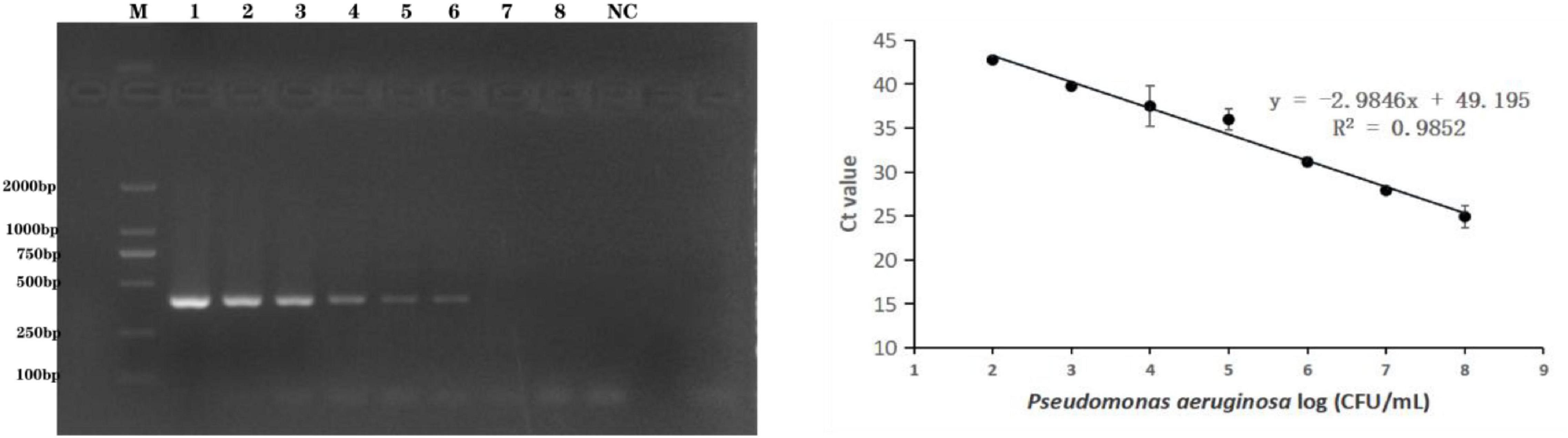
Figure 4. Practical application for the detection of P. aeruginosa serogroup G in spiked drinking water samples. 4.1: Amplification of PCR assay using PA59_01276 primers. Lanes 1–8: Samples spiked with 10-fold dilutions of serogroup G (4.0 × 108 CFU/mL–4.0 × 101 CFU/mL), lane M: DL DNA 2000 marker; lane NC: negative control. 4.2: Amplification of real-time PCR assay using PA59_01276 primers. Plots of Ct values against the log numbers of P. aeruginosa (4.0 × 102 CFU/mL–4.0 × 108 CFU/mL) in artificially contaminated drinking water.
Detection of P. aeruginosa Serogroup G in Water Samples
As determined via the traditional culture approach and slide agglutination using P. aeruginosa antisera, three of the 37 samples (sample numbers 25, 30, and 37) collected from the surrounding living surroundings and markets were contaminated with P. aeruginosa serogroup G. These results were consistent with those obtained using real-time PCR and PCR. After three parallel tests were carried out using the samples, the mean average Ct value for P. aeruginosa serogroup G detection was 20.87, 28.26, and 25.68, respectively (Table 3 and Supplementary Figure 2).
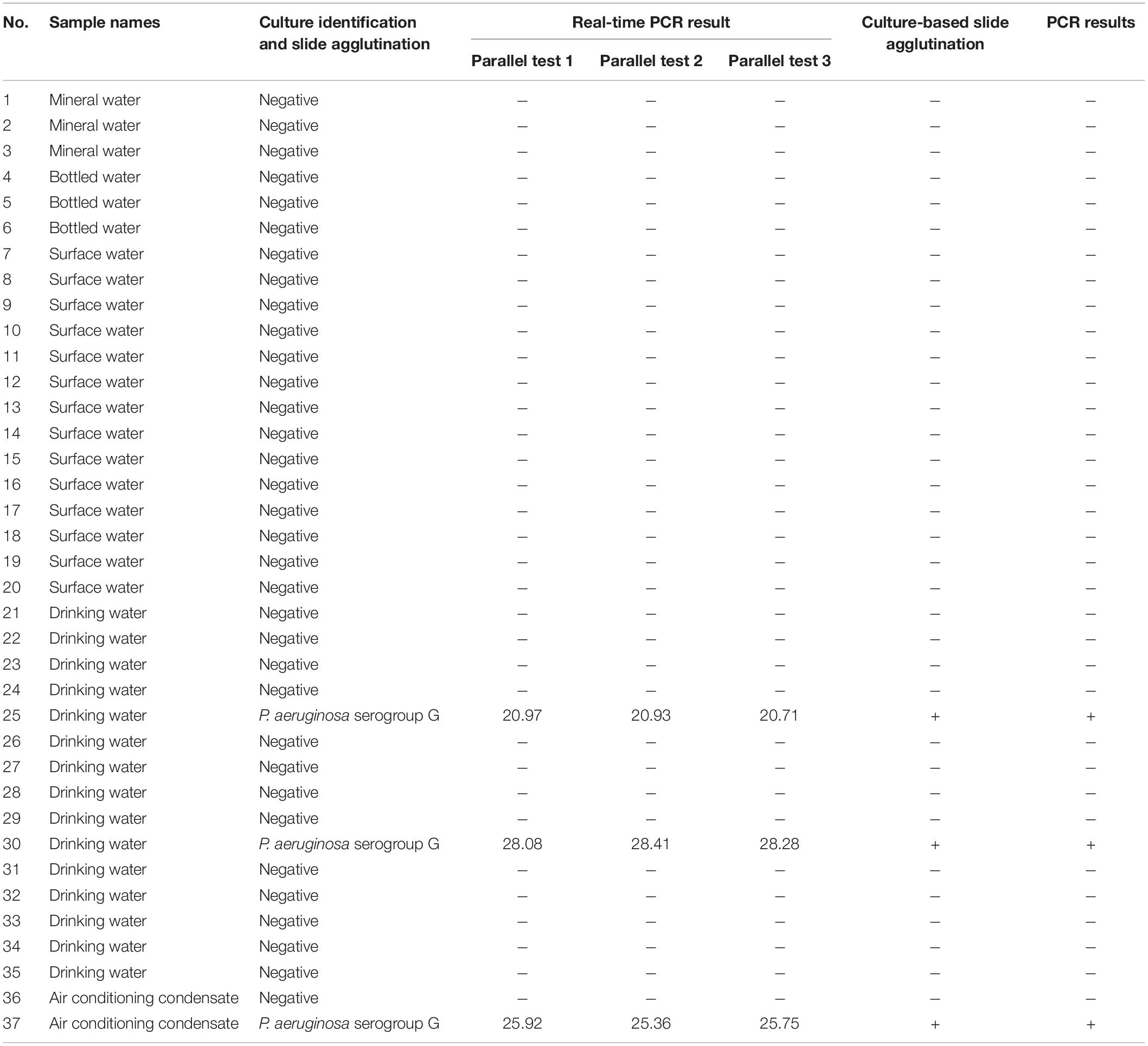
Table 3. Culture-based identification of P. aeruginosa serogroup G, real-time PCR and PCR assay results from 37 water samples.
Discussion
Serological typing is one of the most ordinarily applied phenotypic identification approaches for the classification of P. aeruginosa isolates. In epidemiological research, it is usually needed to quickly trace the transmission of P. aeruginosa by combining molecular typing and classical serotype characteristics. Molecular typing methods commonly include random amplified polymorphic DNA analysis, pulsed-field gel electrophoresis, and multilocus sequence analysis (Toennies et al., 2021). Other approaches for detecting and identifying P. aeruginosa, such as PCR-based open reading frame typing, whole-genome sequencing, and single nucleotide polymorphism typing, are based on genes specific for P. aeruginosa serotypes (Hao et al., 2015; Thrane et al., 2016; Huszczynski et al., 2020). However, thus far, previous studies on detection targets used to identify P. aeruginosa serogroup were performed on a small scale, and the existing approaches for distinguishing serum types are not suitable for separating closely related serum types since their sequences are similar between different serum strains. Consequently, it is required to provide specific molecular targets for serotyping new P. aeruginosa strains and quickly identify different serotypes.
The rapid development of computing and genomics has improved efficiency and personalized serotype-specific target genome mining in recent years. Comparative genomics was chosen because it includes the powerful capabilities of the software, and has the advantage of whole-genome sequences availability, thereby providing more information than a single gene or coding sequence to identify serotype-specific targets. Based on the whole-genome sequencing technology, researchers established a clinical serotyping method, analyzed the assembled input genome through BLASTN, compared the sequence with the OSA cluster database, queried the genome coverage, and classified the OSA cluster with a coverage of more than 95% as serogroup-positive (Thrane et al., 2016). In another serological typing method, the investigator selected a reference genome, divided it into 1000 bp segments in a silica gel, and compared them to all other genome sequences to obtain details of serogroup specificity (Shang et al., 2021). To increase the possibility of identifying specific sequences or gene spacer regions across two genes, researchers used a new comparative genomics method and screened nucleotide sequences for Salmonella serogroup-specific detection (Liu et al., 2011; Yu et al., 2011). However, based on little strain information or the limited number of genomes in the public domain, even if many candidate target sequences are obtained, the verification process is very time-consuming and not suitable for practical applications (Yu et al., 2011). The whole-genome comparisons have been used to discover specific markers in bacteria, such as antibiotic resistance, quorum sensing, biofilm-forming, virulence, and serotype, all of which are ordinarily connected to genes that are acquired from other species through horizontal gene transfer (Thrane et al., 2016; Medina-Rojas et al., 2020; Karash et al., 2021; Mahto et al., 2021; Spinler et al., 2022). In this study, a whole-genome approach was used to identify markers with high reliability and specificity.
To obtain highly feasible and reliable targets, we established a database of 343 strains of P. aeruginosa, which revealed eight serogroup G-specific novel targets. Interestingly, the specific genes were designated related to enzyme and protein coding sequences. In addition to being potential serotype-specific targets, these serotype-specific hypothetical protein-coding regions may also help to analyze the relationship between gene structure and function in the future, to improve the understanding of the unique metabolic behavior of P. aeruginosa serogroup G. Furthermore, recent strategies to expand antibiotic diversity have aimed for exploiting new targets that were identified through genomic approaches (Mills, 2006; Payne et al., 2007). Essential gene codes for antibiotic targets are usually identified via whole genome sequencing, and serum targets established using this method may provide targets for the discovery of new antibiotics. More likely, it will provide a basis for the development of a vaccine based on serogroup G of P. aeruginosa.
Excellent specificity and sensitivity of molecular methods are important for the rapid identification of microorganisms. P. aeruginosa has two antigens, “O” = somatic and “H” = flagellar. Serotyping in most epidemiological studies is done using “O” antigens. Usually, P. aeruginosa produces two distinct forms of O-antigens, namely, a common polysaccharide antigen (CPA, A-band) composed of D-rhamnose homopolymers and an OSA consisting of a heteropolymer with three to five distinct sugars in its repeat units (Lam et al., 2011; Nasrin et al., 2022). The OSA determines the serotype specificity of the bacterium and thus differentiates the P. aeruginosa serotype (Nasrin et al., 2022). Researchers believed that a certain correlation exists between O antigen serotype and toxin secretion. P. aeruginosa may secrete four toxins, including ExoS (exoenzyme S), ExoT (exoenzyme T), ExoU (cytotoxin), and ExoY (Catho et al., 2021). However, some clinical isolates belonging to the serogroup G do not secrete any of the four toxins (Koch et al., 2014; Kuo et al., 2020; Catho et al., 2021). Therefore, the toxin-dependent method is not reliable for serological typing. PCR methods have been used to distinguish the serogroup and serotype of P. aeruginosa (Parsons et al., 2002; Allison and Castric, 2016). The most frequent serogroup G (17.8%) of P. aeruginosa was identified using the OSA cluster database screening method (Del Barrio-Tofino et al., 2019). Based on the genes wbpP and ihfB, PCR methods have been used to distinguish the serogroup G of P. aeruginosa (Koch et al., 2014; Li et al., 2018; Richard et al., 2020). Interestingly, we found that the gene wbgU_1 (PA59_01889) we mined has the same base sequence as target wbpP (PA59_01889) reported in the literature, they are the same target gene. However, the gene ORF_14 was not serogroup G-specific. The coverage of ORF_14 gene was 96.7% in the target serum of serogroup G, non-specific amplification was observed in non-target serum 1.4% (4/281) (Table 4). Essentially, the specificity of molecular targets is very important for serum typing, especially to detect P. aeruginosa serogroup in the context of extremely complex food substrates. After verification, the new molecular detection targets excavated in this study covered 100% of the target serum group G strains but did not exist in the non-target strains. Therefore, the detection targets obtained in this study for P. aeruginosa serogroup G by pan-genome analysis display a better specificity to meet the needs of food safety and water testing.
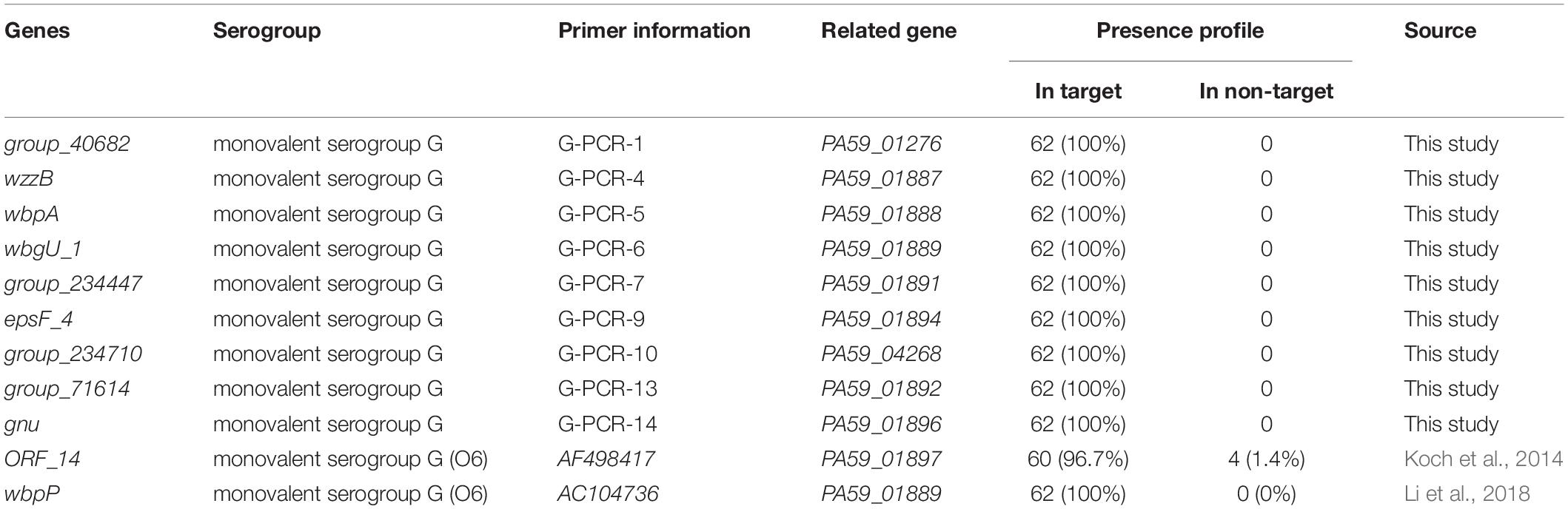
Table 4. Presence profile of novel P. aeruginosa serogroup G-specific targets for target and non-target strains.
The real-time PCR method used in this study targets novel serogroup-specific genes, which helps identify P. aeruginosa serogroup G distinctly from interfering serogroups, such as serogroup D and F, even when the interfering serogroup is more abundant than serogroup G in the sample. The limit of real-time PCR detection for P. aeruginosa serogroup G in a pure culture medium using primers for PA59_01276 was 1.12 × 102 CFU/mL and 4.0 × 103 CFU/mL in food samples contaminated with P. aeruginosa. In a previous study, PCR amplification of wbpP had a limit of ≥103 CFU/mL, and ihfB had a limit of ≥105 CFU/mL for P. aeruginosa serogroup G (Wu et al., 2016). Therefore, our real-time PCR approach is 1–3 orders of magnitude more sensitive than the previously reported method, indicating that we successfully detected P. aeruginosa serogroup G. These values are comparable to those obtained using most PCR methods applied to detect microorganisms in water without prior enrichment (Goepfert et al., 2020). This method has a good consistency for the detection of P. aeruginosa serogroup G without interference from non-target bacteria and actual samples. The results indicated that the chosen target had a strong anti-interference capability and excellent specificity and could quickly, sensitively, and accurately detect P. aeruginosa serogroup G in artificially contaminated samples. The newly mined targets were used to explore the spread of P. aeruginosa serogroup G successfully in actual contaminated water samples.
In addition, the total analysis time, including enrichment culture, sample preparation, genomic DNA extraction, and real-time PCR amplification, took less than 15 h. Compared to the traditional serological typing approach, which requires at least 5 days to complete, the time to obtain results using our method is significantly shorter than that of the conventional serological typing method. At the same time, the cost of real-time PCR is low. Ideally, the cost is only a tenth of traditional typing methods (from $50 for traditional typing methods to $5 for real-time PCR method).
Conclusion
In conclusion, following the analysis of the whole-genome sequences analysis of different P. aeruginosa serogroups from the GenBank, we obtained eight specific detection targets, PA59_01276, PA59_01887, PA59_01888, PA59_01891, PA59_01894, PA59_04268, PA59_01892, and PA59_01896, of monovalent serogroup G by comparative genomics. Based on the novel molecular target gene PA59_01276, we established a real-time fluorescence quantitative PCR approach for the speedy detection and identification of G serotypes. The real-time PCR approach is specific, sensitive, and has a powerful anti-interference ability, with a detection limit of 4.0 × 102 CFU/mL in pure culture and 4.0 × 103 CFU/mL in artificially contaminated drinking water samples. In addition, the 37 actual sample detection for PCR and real-time PCR exhibits satisfactory results. It provides epidemiological investigations with a powerful tool to assess P. aeruginosa contamination for water testing and improving food safety. Moreover, the targets novel serogroup-specific genes that were obtained through this approach can provide a basis for the development of new antibiotics and may promote the development of the P. aeruginosa G serum vaccine.
Data Availability Statement
The original contributions presented in this study are included in the article/Supplementary Material, further inquiries can be directed to the corresponding author/s.
Author Contributions
CW contributed to investigation, methodology, data curation, and writing original draft. QY contributed to the project administration and data curation. YD, QG, RP, and HZ contributed to the data curation. JZ contributed to the supervision and resources. JW and QW contributed to the supervision and writing review and editing. All authors contributed to the article and approved the submitted version.
Funding
This work was supported by the National Key Research and Development Program of China (2017YFC1600403), Guangdong Provincial Key Laboratory (2020B121201009), GDAS’ Special Project of Science and Technology Development (2020GDASYL-20200401002), and GDAS Project of Science and Technology Development (2019GDASYL-0103008).
Conflict of Interest
The authors declare that the research was conducted in the absence of any commercial or financial relationships that could be construed as a potential conflict of interest.
Publisher’s Note
All claims expressed in this article are solely those of the authors and do not necessarily represent those of their affiliated organizations, or those of the publisher, the editors and the reviewers. Any product that may be evaluated in this article, or claim that may be made by its manufacturer, is not guaranteed or endorsed by the publisher.
Acknowledgments
We would like to thank partial P. aeruginosa strains were provided by Zhujiang Hospital, Guangzhou, China.
Supplementary Material
The Supplementary Material for this article can be found online at: https://www.frontiersin.org/articles/10.3389/fmicb.2022.928154/full#supplementary-material
Abbreviations
CFU, colony-forming units; Ct, cycle threshold; LB, Luria–Bertani; NCBI, National Center for Biotechnology Information; PCR, polymerase chain reaction; OSA, O-specific antigen; LOD, limit of detection.
Footnotes
References
Allison, T. M., and Castric, P. (2016). Selective distribution of Pseudomonas aeruginosa O-antigen among strains producing group I pilin. Pathog Dis. 74:ftv102. doi: 10.1093/femspd/ftv102
Ayi, B. (2015). Infections acquired via fresh water: from lakes to hot tubs. Microbiol. Spectr. 3. doi: 10.1128/microbiolspec.IOL5-0019-2015
Balabanova, L., Shkryl, Y., Slepchenko, L., Cheraneva, D., Podvolotskaya, A., Bakunina, I., et al. (2020). Genomic features of a food-derived Pseudomonas aeruginosa strain PAEM and biofilm-associated gene expression under a marine bacterial α-Galactosidase. Int. J. Mol. Sci. 21:7666. doi: 10.3390/ijms21207666
Carmeli, Y., Armstrong, J., Laud, P. J., Newell, P., Stone, G., Wardman, A., et al. (2016). Ceftazidime-avibactam or best available therapy in patients with ceftazidime-resistant Enterobacteriaceae and Pseudomonas aeruginosa complicated urinary tract infections or complicated intra-abdominal infections (REPRISE): a randomised, pathogen-directed, phase 3 study. Lancet Infect. Dis. 16, 661–673.
Catho, G., Martischang, R., Boroli, F., Chraïti, M. N., Martin, Y., Koyluk Tomsuk, Z., et al. (2021). Outbreak of Pseudomonas aeruginosa producing VIM carbapenemase in an intensive care unit and its termination by implementation of waterless patient care. Crit. Care 25:301. doi: 10.1186/s13054-021-03726-y
Cheng, W., Wang, Z., Xu, F., Ahmad, W., Lu, G., Su, Y., et al. (2021). Genome-Wide identification of LRR-RLK family in saccharum and expression analysis in response to biotic and abiotic stress. Curr. Issues Mol. Biol 43, 1632–1651. doi: 10.3390/cimb43030116
Del Barrio-Tofino, E., Sanchez-Diener, I., Zamorano, L., Cortes-Lara, S., Lopez-Causape, C., Cabot, G., et al. (2019). Association between Pseudomonas aeruginosa O-antigen serotypes, resistance profiles and high-risk clones: results from a Spanish nationwide survey. J. Antimicrob Chemoth. 74, 3217–3220. doi: 10.1093/jac/dkz346
Diaz-Rios, C., Hernandez, M., Abad, D., Alvarez-Montes, L., Varsaki, A., Iturbe, D., et al. (2021). New sequence type ST3449 in multidrug-resistant Pseudomonas aeruginosa isolates from a cystic fibrosis patient. Antibiotics-Basel 10:491. doi: 10.3390/antibiotics10050491
Falkinham, J. O., Hilborn, E. D., Arduino, M. J., Pruden, A., and Edwards, M. A. (2015). Epidemiology and ecology of opportunistic premise plumbing pathogens:Legionella pneumophila, Mycobacterium avium, and Pseudomonas aeruginosa. Environ. Health Persp. 123, 749–758. doi: 10.1289/ehp.1408692
Faure, K., Shimabukuro, D., Ajayi, T., Allmond, L. R., Sawa, T., and Wiener-Kronish, J. P. (2003). O-antigen serotypes and type III secretory toxins in clinical isolates of Pseudomonas aeruginosa. J. Clin. Microbiol. 41, 2158–2160. doi: 10.1128/JCM.41.5.2158-2160.2003
Goepfert, L., Kluepfel, J., Heinritz, C., Elsner, M., and Seidel, M. (2020). Macroporous epoxy-based monoliths for rapid quantification of Pseudomonas aeruginosa by adsorption elution method optimized for qPCR. Anal. Bioanal. Chem. 412, 8185–8195. doi: 10.1007/s00216-020-02956-3
Hao, Y., Murphy, K., Lo, R. Y., Khursigara, C. M., and Lam, J. S. (2015). Single-Nucleotide polymorphisms found in the migA and wbpX glycosyltransferase genes account for the intrinsic lipopolysaccharide defects exhibited by Pseudomonas aeruginosa PA14. J. Bacteriol. 197, 2780–2791. doi: 10.1128/JB.00337-15
Homma, J. Y. (1982). Designation of the 13 O-Group antigens of Pseudomonas-Aeruginosa - an amendment for the tentative proposal in 1976. Japanese J. Exp. Med. 52, 317–319.
Howlader, D. R., Das, S., Lu, T., Hu, G., Varisco, D. J., Dietz, Z. K., et al. (2021). Effect of two unique nanoparticle formulations on the efficacy of a broadly protective vaccine against Pseudomonas Aeruginosa. Front. Pharmacol. 12:706157. doi: 10.3389/fphar.2021.706157
Huszczynski, S. M., Lam, J. S., and Khursigara, C. M. (2020). The role of Pseudomonas aeruginosa lipopolysaccharide in bacterial pathogenesis and physiology. Pathogens 9:6.
Kaluzny, K., Abeyrathne, P. D., and Lam, J. S. (2007). Coexistence of two distinct versions of O-antigen polymerase, Wzy-alpha and Wzy-beta, in Pseudomonas aeruginosa serogroup O2 and their contributions to cell surface diversity. J. Bacteriol. 189, 4141–4152. doi: 10.1128/JB.00237-07
Karash, S., Nordell, R., Ozer, E. A., and Yahr, T. L. (2021). Genome sequences of two Pseudomonas aeruginosa isolates with defects in type III secretion system gene expression from a chronic ankle wound infection. Microbiol. Spect. 9:e0034021. doi: 10.1128/Spectrum.00340-21
Kintz, E., Scarff, J. M., DiGiandomenico, A., and Goldberg, J. B. (2008). Lipopolysaccharide O-antigen chain length regulation in Pseudomonas aeruginosa serogroup O11 strain PA103. J. Bacteriol. 190, 2709–2716. doi: 10.1128/JB.01646-07
Knirel, Y. A., Bystrova, O. V., Kocharova, N. A., Zaehringer, U., and Pier, G. B. (2010). Conserved and variable structural features in the lipopolysaccharide of Pseudomonas aeruginosa. J Endotoxin Res. 12, 324–336. doi: 10.1179/096805106X118906
Koch, H., Emrich, T., Jampen, S., Wyss, M., Gafner, V., Lazar, H., et al. (2014). Development of a 4-valent genotyping assay for direct identification of the most frequent Pseudomonas aeruginosa serotypes from respiratory specimens of pneumonia patients. J. Med. Microbiol. 63, 508–517. doi: 10.1099/jmm.0.066043-0
Kuo, C., Hsu, Y., Wang, S., Liou, B., Lim, S. B., Chen, Y., et al. (2020). IGLR-2, a leucine-rich repeat domain containing protein, is required for the host defense in Caenorhabditis elegans. Front. Immunol. 11:561337. doi: 10.3389/fimmu.2020.561337
Lam, J. S., Taylor, V. L., Islam, S. T., Hao, Y., and Kocincova, D. (2011). Genetic and functional diversity of Pseudomonas aeruginosa lipopolysaccharide. Front. Microbiol. 2:118. doi: 10.3389/fmicb.2011.00118
Le Berre, R., Nguyen, S., Nowak, E., Kipnis, E., Pierre, M., Quenee, L., et al. (2011). Relative contribution of three main virulence factors in Pseudomonas aeruginosa pneumonia. Crit. Care Med. 39, 2113–2120. doi: 10.1097/CCM.0b013e31821e899f
Lee, C., Su, T., Ye, J., Hsu, P., Kuo, A., Chia, J., et al. (2017). Risk factors and clinical significance of bacteremia caused by Pseudomonas aeruginosa resistant only to carbapenems. J. Microbiol Immunol. 50, 677–683. doi: 10.1016/j.jmii.2015.06.003
Letunic, I., and Bork, P. (2019). Interactive Tree Of Life (iTOL) v4: recent updates and new developments. Nucleic Acids Res. 47, W256–W259. doi: 10.1093/nar/gkz239
Li, H., Du, Y., Qian, C., Li, L., Jiang, L., Jiang, X., et al. (2018). Establishment of a suspension array for Pseudomonas aeruginosa O-antigen serotyping. J. Microbiol. Meth. 155, 59–64. doi: 10.1016/j.mimet.2018.11.006
Li, K., Wang, S., Liu, W., Kwok, L., Bilige, M., and Zhang, W. (2022). Comparative genomic analysis of 455 Lactiplantibacillus plantarum isolates: habitat-specific genomes shaped by frequent recombination. Food Microbiol. 104:103989. doi: 10.1016/j.fm.2022.103989
Liu, B., Zhang, L., Zhu, X., Shi, C., Chen, J., Liu, W., et al. (2011). PCR identification of Salmonella serogroups based on specific targets obtained by comparative genomics. Int. J. Food Microbiol. 144, 511–518. doi: 10.1016/j.ijfoodmicro.2010.11.010
Mahto, K. U., Kumari, S., and Das, S. (2021). Unraveling the complex regulatory networks in biofilm formation in bacteria and relevance of biofilms in environmental remediation. Crit. Rev. Biochem. Mol. 57, 305–332. doi: 10.1080/10409238.2021.2015747
Marei, E. M. (2020). Isolation and characterization of Pseudomonas aeruginosa and its virulent bacteriophages. Pak. J. Biol. Sci. 23, 491–500. doi: 10.3923/pjbs.2020.491.500
Medina-Rojas, M., Stribling, W., Snesrud, E., Garry, B. I., Li, Y., Mc Gann, P., et al. (2020). Comparison of Pseudomonas aeruginosa strains reveals that exolysin a toxin plays an additive role in virulence. Pathog Dis. 78:ftaa010. doi: 10.1093/femspd/ftaa010
Mena, K. D., and Gerba, C. P. (2009). Risk assessment of Pseudomonas aeruginosa in water. Rev. Environ. Contam Toxicol. 201, 71–115.
Mills, S. D. (2006). When will the genomics investment pay off for antibacterial discovery? Biochem. Pharmacol. 71, 1096–1102. doi: 10.1016/j.bcp.2005.11.025
Morand, A., and Morand, J. J. (2017). Pseudomonas aeruginosa en dermatologie. Ann. Dermatologie Vénéréologie 144, 666–675.
Nasrin, S., Hegerle, N., Sen, S., Nkeze, J., Sen, S., Permala-Booth, J., et al. (2022). Distribution of serotypes and antibiotic resistance of invasive Pseudomonas aeruginosa in a multi-country collection. BMC Microbiol. 22:13. doi: 10.1186/s12866-021-02427-4
Page, A. J., Cummins, C. A., Martin, H., Wong, V. K., Sandra, R., Holden, M., et al. (2015). Roary: rapid large-scale prokaryote pan genome analysis. Bioinformatics 31, 3691–3693. doi: 10.1093/bioinformatics/btv421
Pang, R., Xie, T., Wu, Q., Li, Y., Lei, T., Zhang, J., et al. (2019). comparative genomic analysis reveals the potential risk of Vibrio parahaemolyticus isolated from ready-to-eat foods in China. Front. Microbiol. 10:186. doi: 10.3389/fmicb.2019.00186
Parsons, Y. N., Panagea, S., Smart, C., Walshaw, M. J., Hart, C. A., and Winstanley, C. (2002). Use of subtractive hybridization to identify a diagnostic probe for a cystic fibrosis epidemic of Pseudomonas aeruginosa. J. Clin. Microbiol. 40, 4607–4611. doi: 10.1128/JCM.40.12.4607-4611.2002
Payne, D. J., Gwynn, M. N., Holmes, D. J., and Pompliano, D. L. (2007). Drugs for bad bugs: confronting the challenges of antibacterial discovery. Nat. Rev. Drug Discov. 6, 29–40. doi: 10.1038/nrd2201
Petitjean, M., Juarez, P., Meunier, A., Daguindau, E., Puja, H., Bertrand, X., et al. (2021). The rise and the fall of a Pseudomonas aeruginosa endemic lineage in a hospital. Microbial Genomics 7:000629. doi: 10.1099/mgen.0.000629
Pirnay, J. P., De Vos, D., Cochez, C., Bilocq, F., Vanderkelen, A., Zizi, M., et al. (2002). Pseudomonas aeruginosa displays an epidemic population structure. Environ. Microbiol. 4, 898–911. doi: 10.1046/j.1462-2920.2002.00321.x
Qi, J., Li, L., Du, Y., Wang, S., Wang, J., Luo, Y., et al. (2014). The identification, typing, and antimicrobial susceptibility of Pseudomonas aeruginosa isolated from mink with hemorrhagic pneumonia. Vet. Microbiol. 170, 456–461. doi: 10.1016/j.vetmic.2014.02.025
Riaz, M., and Hashmi, M. R. (2019). Linear Diophantine fuzzy set and its applications towards multi-attribute decision-making problems. J. Intell. Fuzzy Syst. 37, 5417–5439.
Richard, G., MacKenzie, C. R., Henry, K. A., Vinogradov, E., Hall, J. C., and Hussack, G. (2020). Antibody binding to the o-specific antigen of Pseudomonas aeruginosa O6 inhibits cell growth. Antimicrob Agents Ch. 64:e02168-19. doi: 10.1128/AAC.02168-19
Seemann, T. (2014). Prokka: rapid prokaryotic genome annotation. Bioinformatics 30, 2068–2069. doi: 10.1093/bioinformatics/btu153
Shang, Y., Ye, Q., Wu, Q., Pang, R., Xiang, X., Wang, C., et al. (2021). PCR identification of Salmonella serovars for the E serogroup based on novel specific targets obtained by pan-genome analysis. Lwt-Food Sci. Technol. 145: 110535.
Spinler, J. K., Raza, S., Thapa, S., Venkatachalam, A., Scott, T., Runge, J. K., et al. (2022). Comparison of whole genome sequencing and repetitive element PCR for multidrug-resistant Pseudomonas aeruginosa strain typing. J. Mol. Diagn. 24, 158–166. doi: 10.1016/j.jmoldx.2021.10.004
Thrane, S. W., Taylor, V. L., Lund, O., Lam, J. S., and Jelsbak, L. (2016). Application of whole-genome sequencing data for O-Specific antigen analysis and in silico serotyping of Pseudomonas aeruginosa isolates. J. Clin. Microbiol. 54, 1782–1788. doi: 10.1128/JCM.00349-16
Toennies, H., Prior, K., Harmsen, D., and Mellmann, A. (2021). Establishment and evaluation of a core genome multilocus sequence typing scheme for whole-genome sequence-based typing of Pseudomonas aeruginosa. J. Clin. Microbiol. 59:e01987-20. doi: 10.1128/JCM.01987-20
Treangen, T. J., Ondov, B. D., Koren, S., and Phillippy, A. M. (2014). The Harvest suite for rapid core-genome alignment and visualization of thousands of intraspecific microbial genomes. Genome Biol. 15:524. doi: 10.1186/s13059-014-0524-x
Wu, Q., Ye, Y., Li, F., Zhang, J., and Guo, W. (2016). Prevalence and genetic characterization of Pseudomonas aeruginosa in drinking water in guangdong province of China. Lwt - Food Sci. Technol. 69, 24–31.
Yu, S., Liu, W., Shi, C., Wang, D., Dan, X., Li, X., et al. (2011). SMM-system: a mining tool to identify specific markers in Salmonella enterica. J. Microbiol. Meth 84, 423–429. doi: 10.1016/j.mimet.2011.01.006
Keywords: serogroup G-specific target, aquatic environment, molecular detection, serotyping, sensitivity
Citation: Wang C, Ye Q, Ding Y, Zhang J, Gu Q, Pang R, Zhao H, Wang J and Wu Q (2022) Detection of Pseudomonas aeruginosa Serogroup G Using Real-Time PCR for Novel Target Genes Identified Through Comparative Genomics. Front. Microbiol. 13:928154. doi: 10.3389/fmicb.2022.928154
Received: 25 April 2022; Accepted: 07 June 2022;
Published: 24 June 2022.
Edited by:
Daniel Yero, Universidad Autónoma de Barcelona, SpainReviewed by:
Feng Zhang, Gansu Agricultural University, ChinaEwa Maria Furmanczyk, Research Institute of Horticulture, Poland
Mehul Jani, University of North Texas, United States
Copyright © 2022 Wang, Ye, Ding, Zhang, Gu, Pang, Zhao, Wang and Wu. This is an open-access article distributed under the terms of the Creative Commons Attribution License (CC BY). The use, distribution or reproduction in other forums is permitted, provided the original author(s) and the copyright owner(s) are credited and that the original publication in this journal is cited, in accordance with accepted academic practice. No use, distribution or reproduction is permitted which does not comply with these terms.
*Correspondence: Juan Wang, d2FuZ2p1YW5Ac2NhdS5lZHUuY24=; Qingping Wu, d3VxcDIwM0AxNjMuY29t
†These authors have contributed equally to this work