- 1Faculty of Biotechnology, Department of Molecular Microbiology, University of Wrocław, Wrocław, Poland
- 2Department of Microbiology and Molecular Medicine, University of Geneva, Geneva, Switzerland
- 3Faculty of Biology, Ludwig-Maximilians-Universität München, Planegg-Martinsried, Germany
- 4Department of Biochemistry, University of Oxford, Oxford,United Kingdom
- 5Institute of General Microbiology, Kiel University, Kiel, Germany
Most bacteria use the ParABS system to segregate their newly replicated chromosomes. The two protein components of this system from various bacterial species share their biochemical properties: ParB is a CTPase that binds specific centromere-like parS sequences to assemble a nucleoprotein complex, while the ParA ATPase forms a dimer that binds DNA non-specifically and interacts with ParB complexes. The ParA-ParB interaction incites the movement of ParB complexes toward the opposite cell poles. However, apart from their function in chromosome segregation, both ParAB may engage in genus-specific interactions with other protein partners. One such example is the polar-growth controlling protein DivIVA in Actinomycetota, which binds ParA in Mycobacteria while interacts with ParB in Corynebacteria. Here, we used heterologous hosts to investigate whether the interactions between DivIVA and ParA or ParB are maintained across phylogenic classes. Specifically, we examined interactions of proteins from four bacterial species, two belonging to the Gram positive Actinomycetota phylum and two belonging to the Gram-negative Pseudomonadota. We show that while the interactions between ParA and ParB are preserved for closely related orthologs, the interactions with polarly localised protein partners are not conferred by orthologous ParABs. Moreover, we demonstrate that heterologous ParA cannot substitute for endogenous ParA, despite their high sequence similarity. Therefore, we conclude that ParA orthologs are fine-tuned to interact with their partners, especially their interactions with polarly localised proteins are adjusted to particular bacterial species demands.
Introduction
Although most bacteria use the same machinery to replicate and segregate their chromosomes, they employ different strategies to coordinate their cell cycle. Because of adaptations to various environmental niches, bacterial species differ significantly with respect to cell shape, generation time, mode of growth, and metabolic diversity. The demands of diverse cell cycles or modes of growth require cell cycle checkpoints that depend on specific protein–protein interactions. In the majority of bacterial species, cell division by binary fission directly follows chromosome duplication and segregation (Thanbichler, 2010; Reyes-Lamothe et al., 2012). Chromosome replication is initiated at the origin of replication (oriC) region. Shortly after duplication, daughter oriCs are actively separated and moved towards the future daughter cell(s), while the rest of the chromosome still undergoes replication (Webb et al., 1997; Lemon and Grossman, 2000; Viollier et al., 2004; Bates and Kleckner, 2005; Reyes-Lamothe et al., 2008; Trojanowski et al., 2018). At the same time, the bacterial cells grow, which in the case of rod-shaped bacteria results from the extension of either the lateral cell wall or cell poles. The position of oriCs in new-born cells is precisely controlled, but it may differ even among closely related bacteria, such as Corynebacteria and Mycobacteria (Donovan et al., 2010; Trojanowski et al., 2015; Böhm et al., 2017). In most bacteria (with the exception of gammaproteobacteria), the segregation and subsequent positioning of oriC depends on chromosome segregation proteins, namely, ParA and ParB. Interestingly, these proteins are also involved in interactions that allow the coordination of cell cycle processes (Pióro and Jakimowicz, 2020).
Segregation proteins share their biochemical properties in all studied bacterial species, with ParA being a P–loop ATPase and ParB a CTPase which, thanks to the HTH domain, binds to parS DNA sequences. In almost 70% of the studied bacterial chromosomes, parS sequences are located in the vicinity of oriC, however, their number and exact positioning differ among bacteria (Jakimowicz et al., 2007; Livny et al., 2007; Toro et al., 2008; Dubarry et al., 2019; Böhm et al., 2020). ParB interactions with parS are followed by CTP-dependent ParB spreading at neighbouring DNA. These interactions lead to the formation of higher-order nucleoprotein complexes (segrosomes; Osorio-Valeriano et al., 2019; Soh et al., 2019; Jalal et al., 2020; Babl et al., 2022), which are actively moved by ParA. Upon binding ATP, ParA forms a dimer that non-specifically interacts with DNA. Nucleoprotein complexes formed by ParB stimulate the ATPase activity of ParA and trigger its release from DNA, resulting in a concentration gradient of nucleoid-bound ParA. The ParB complexes are moved towards higher concentrations of DNA-bound ParA, leading to separation of replicated oriC regions (Leonard et al., 2004, 2005; Livny et al., 2007; Lim et al., 2014; Hu et al., 2017).
Interestingly, the positioning of oriC regions and segrosomes, as well as the pattern of their segregation, differs among the studied bacterial species. In a free living curved alphaproteobacterium Caulobacter crescentus, cell division generates two cell types, flagellated and stalked with oriCs, located at the old cell pole. Upon initiation of replication (which takes place in stalked cell), one of the oriCs and segrosomes is moved towards the opposite cell pole (Jensen et al., 2001; Toro et al., 2008). Closely related Rhodobacter sphaeroides possesses two chromosomes, divides symmetrically and positions both oriC in distinct manner, with oriCI and ParB1 located similarly to C. crescentus (Dubarry et al., 2019). However, polarly extending cells of two related Actinomycetota species, i.e., Mycobacterium smegmatis and Corynebacterium glutamicum, differ in chromosome arrangement. In fast-growing club-shaped C. glutamicum, overlapping rounds of chromosome replication result in multiple copies of chromosomes in the cells, and in newborn cells, oriCs are localised at both cell poles (Böhm et al., 2017). Contrary, in slow-growing M. smegmatis oriC of a single chromosome is positioned at some distance from the pole, and upon initiation of replication, both oriCs are moved in a partially asymmetrical manner (Ginda et al., 2017). This indicates the existence of different cell cycle checkpoints even in closely related bacterial species.
The specific positioning of oriC is usually dependent on ParA or ParB interactions. Anchoring oriC at the cell poles is mediated in C. crescentus due to the interaction of ParB complexes with cell pole scaffold PopZ protein (Holmes et al., 2016). PopZ interacts at least eight other proteins and also ParA-PopZ interactions contribute to the chromosome segregation (Ptacin et al., 2014). Interestingly while in C. glutamicum, ParB interacts with the polar growth determinant DivIVA (Donovan et al., 2012), in M. smegmatis, ParA instead of ParB was shown to be a DivIVA interaction partner and this interaction facilitates segrosome separation and affects cell elongation (Ginda et al., 2013; Pióro et al., 2019). In C. crescentus, ParA also interacts with TipN, the protein localised at the new cell pole, and this interaction is required for proper chromosome segregation (Schofield et al., 2010). Moreover, the ParB protein controls cell division thanks to the interaction with the regulator of FtsZ polymerisation, MipZ protein in C. crescentus and R. sphaeroides or directly with FtsZ in C. glutamicum (Thanbichler and Shapiro, 2006; Donovan et al., 2010; Dubarry et al., 2019). Finally, by recruiting the structural maintenance of the chromosome (SMC) complex, ParB protein coordinates chromosome segregation with chromosome compaction (Gruber and Errington, 2009; Sullivan et al., 2009; Minnen et al., 2011; Chan et al., 2020).
Due to its numerous interactions, the ParABS system plays a role in the control of the bacterial cell cycle, and its varied functions are manifested by a variety of phenotypes that result from parAB deletion. In some bacteria, including C. crescentus, the parAB genes are essential for cell survival (Mohl and Gober, 1997), but in many other bacterial species, their deletion is possible but causes a range of chromosome defects from mild to more severe (Jakimowicz et al., 2007; Donovan et al., 2010; Ginda et al., 2013). Overproduction of ParA in C. crescentus, M. smegmatis, and C. glutamicum also impacts culture growth, altering cell length and impairing chromosome segregation, but usually to a lesser extent than deletion (Mohl and Gober, 1997; Donovan et al., 2010; Ginda et al., 2013). Thus, ParA and ParB proteins not only execute chromosome segregation but also indirectly influence cell elongation.
Comparative bioinformatics analysis revealed that ParABS systems are very conserved among a broad range of studied bacteria (Livny et al., 2007). On the other hand, recent studies show that components of the ParABS system take part in many different cellular processes, which indicates that they acquired new functions during evolution. The genus-specific interactions raise the question of compatibility of ParAB systems among different bacterial species. Here, we compared the heterologous interactions of ParAB proteins from four different bacterial species, two Gram-positive and two Gram-negative. Next, we examined ParA functionality in heterologous systems using M. smegmatis and C. crescentus as the host for overexpression of closely and distantly related homologues. Finally, we focused on most closely related actinobacterial ParA proteins and we tested their combability in C. glutamicum and M. smegmatis using complementation assays.
Materials and Methods
Cloning and Construct Preparation
DNA manipulations were performed using standard protocols (Sambrook and Russell, 2001). Reagents and enzymes were supplied by New England Biolabs (NEB), Merck, Roth and Thermo-Scientific. Oligonucleotides were synthesized by Merck, Genomed, and Microsynth, and sequencing was performed by Microsynth. The genetic construct preparation and M. smegmatis, C. glutamicum, and C. crescentus modifications are described in detail in the Supplementary Material.
Escherichia coli Growth Conditions
Escherichia coli strains were grown in lysogeny broth (LB) medium at 37°C [DH5α, BL21(DE3)] or 30°C (BTH101). Culture conditions, antibiotic concentrations, and transformation protocols followed standard procedures (Sambrook and Russell, 2001).
BTH Analysis and Fluorescence Microscopy Analysis of Escherichia coli
Bacterial two-hybrid (BTH) interaction studies were performed as previously described (Karimova et al., 1998). To analyse the interaction between the studied proteins in the BTH system, pUT18C and pKT25 derivatives with analysed genes were transformed into E. coli BTH101 and plated on LB containing 0.004% X-gal, 50 μg/ml kanamycin, 100 μg/ml ampicillin, and 0.5 mM isopropyl-β-D-1-thiogalactopyranoside (IPTG). After 2 days of incubation at 30°C, the selected representative colonies of each transformation were plated together on LB containing X-gal, kanamycin, ampicillin, and IPTG.
For assays of ParAs and DivIVA colocalisation in E. coli, BL21 (DE3) cells containing pJP108 (Ptacin et al., 2010), pJP108divIVAMs (Pióro et al., 2019), or pCD74 containing divIVACg-mcherry gene and pACYCDuet-1 vector derivatives containing egfp-parA genes were cultured to log phase in the presence of ampicillin and chloramphenicol. The expression of the divIVACg-mcherry and egfp-parA genes was induced by the addition of 0.1 mM IPTG, while the expression of ics-mcherry and mcherry-divIVAMs in pJP108 and its derivative was induced with 0.05% arabinose for 1 h.
For colocalisation analysis of M. smegmatis ParB with DivIVA in E. coli, BL21(DE3) cells containing pJP108, pJP108divIVAMs or pCD74, and pET28aparBMs-mneon or pACYCparBMs-mneon (when coexpressed with pCD74) were cultured to log phase in the presence of ampicillin and chloramphenicol (in the case of pACYC). The expression of divIVACg-mcherry (from pCD74) and parBMs-mneon genes was induced by the addition of 0.1 mM IPTG, while the expression of ics-mcherry and mcherry-divIVAMs in pJP108 and its derivative was induced with 0.05% arabinose for 1 h.
pETDuet derivatives possessing parBCc-cfp or parBCg-cfp with mcherry-ics, mcherry-DivIVAMs, or divIVACg-mcherry were cultured to log phase in the presence of ampicillin. Gene expression was induced by the addition of 0.1 mM IPTG for 1 h.
After induction, 10 μl of culture was smeared on microscopic slides and mounted with 5 μl of phosphate-buffered saline (PBS)-glycerol (1:1) solution. Cells were examined by a Leica DM6 B fluorescence microscope equipped with a 100x objective with a DFC7000 GT camera. Images were analysed using LAS X 3.6.0.20104, Fiji and “R” software (ggplot2 package; Wickham, 2009).
ATPase Activity Assays
The ATPase activity of ParA proteins was measured using a colorimetric ATPase/GTPase activity assay kit (Sigma–Aldrich, MAK113) according to the manufacturer’s instructions. Briefly, 10 μl of 4 mM ATP was added to 30 μl reaction mixtures containing kit assay buffer and proteins of interest (diluted in assay buffer to a 2 μM concentration, in technical triplicates) in a 96-well plate. The plate was incubated for 30 min at 30°C, and then the reaction mixtures were transferred to another plate containing 200 μl of colorimetric reagent. The mixtures were further incubated for 30 min at room temperature followed by absorption measurement at 630 nm (A630). The obtained results were plotted against a standard curve for free phosphate. The experiment was performed in two independent replicates. All studied ParB proteins were confirmed not to exhibit ATPase activity.
Mycobacterium smegmatis Growth and Microscopic Analysis
Mycobacterium smegmatis strains were grown either in Middlebrook liquid 7H9 medium (Difco) supplemented with 10% ADC [albumin-dextrose-catalase (BD)] and 0.05% Tween 80 or on solid 7H10 supplemented with 10% OADC [oleic acid, albumin-dextrose-catalase (BD)], 0.5% glycerol, and 0.05% Tween 80.
For growth curve analyses, M. smegmatis strains were inoculated from glycerol stocks and grown to log phase (OD600 0.3–0.4). Next, the cultures were diluted in fresh medium with and without addition of acetamide to an OD600 of 0.05, and 300 μl of diluted culture was loaded into the wells of a Bioscreen C-compatible honeycomb plate. The microplate cultures were incubated at 37°C with continuous shaking using Bioscreen C [Automated Growth Curves Analysis System, Growth Curves (Alab)], and the culture optical density was measured every 20 min. The results were analysed in Excel.
For snapshot microscopy, M. smegmatis strains were inoculated from glycerol stocks and grown overnight. After that, cultures were diluted to OD600 of 0.01 in fresh medium with and without addition of acetamide and cultivated until mid-log phase (OD600 0.5). For DNA staining, cells were treated with DAPI (2 μg ml−1) for 2 h. After centrifugation (5,000 rpm, 5 min), the cells were resuspended in PBS, and clumps were disrupted by a vortex mixer. Ten microlitres of culture was smeared on microscopic slides, dried and mounted with 5 μl of PBS-glycerol (1:1) solution. Cells were examined by a Leica DM6 B fluorescence microscope equipped with a 100x objective with a DFC7000 GT camera. Images were analysed using LAS X 3.6.0.20104 and Fiji.
Corynebacterium glutamicum Strain Growth and Microscopic Analysis
For the growth analysis, C. glutamicum strains were grown overnight in brain heart infusion [BHI medium (OxoidTM)] with kanamycin (25 μg/ml), and the cultures were diluted with a mixture of CGXII (Keilhauer et al., 1993) and BHI medium (1:1) with kanamycin to an OD of 0.3 and cultivated overnight. Next, the overnight cultures were used to set up Bioscreen cultures with starting OD600—0.2 in CGXII:BHI (3:1) medium with addition of IPTG (to a final concentration of 0.1 mM) or without addition of IPTG. Three hundred microlitres of culture was loaded into the wells of a Bioscreen C-compatible honeycomb plate in three replicates for each strain. The microplate cultures were incubated at 37°C with continuous shaking using Bioscreen C [Automated Growth Curves Analysis System, Growth Curves (Alab)], and the optical density was measured every 20 min. The results were analysed in Excel.
For snapshot microscopy, C. glutamicum strains were grown overnight in CGXII:BHI (OxoidTM) medium with kanamycin. Next, they were cultured in 1 ml of CGXII medium to an OD600 of 0.5 and then induced with IPTG (final concentration of 0.1 mM) or cultured further without the addition of IPTG. Next, the cells were washed twice with PBS and resuspended in 0.2 ml of PBS with the addition of FM4-64 (10 μg/ml) and Hoechst3342 (1 μg/ml). After 15 min of incubation, the cells were mounted on microscopic slides with agarose pads and analysed using a Zeiss Axio-Imager M1 fluorescence microscope (Carl Zeiss) with an EC Plan Neofluar ×100/1.3 oil Ph3 objective and a 2.5x optovar. Images were analysed using Fiji.
Caulobacter crescentus Growth and Microscopic Analysis
For microscopic experiments, C. crescentus strains were inoculated from glycerol stocks and grown overnight in PYE (peptone-yeast extract) medium with 1 μg/ml gentamycin. The next day, cultures were diluted 30 times to fresh medium and either induced with 0.5 mM vanillate or cultured without induction. After 5 h of further growth, 10 μl of culture was mounted on microscopic slides with agarose pads (1.2% agarose in PYE). Cells were examined by a Zeiss alpha plan achromatic 100x/1.46 oil phase 3 on an Axio Imager M2 microscope with an appropriate filter (Visitron Systems GmbH) and a cooled CCD camera (Photometrics, CoolSNAP HQ2) controlled through Metamorph (Molecular Devices).
For plating assays, C. crescentus strains were inoculated from glycerol stocks and grown overnight in PYE medium with 1 μg/ml gentamycin. The next day, cultures were diluted to OD = 1 in fresh PYE medium, and serial tenfold dilutions were made until 106 dilution was achieved. Eight microliters of each diluted culture was transferred to PYE agar plates containing gentamycin and a specific concentration of inducer (vanillate). The plates were incubated at 30°C for 2 days.
For flow cytometry analysis, C. crescentus cultures were treated as previously described for microscopic experiments, but triple repetition of each strain was performed. After 5 h of cultivation, 100 μl of each culture was transferred into 900 μl of 77% ethanol on ice and stored at −20°C until further use.
To estimate chromosome number, 20 μg ml−1 rifampicin was added to the rest of the culture for 3 h at 30°C and then fixed with ethanol as previously described. Fixed cells were resuspended in FACS staining buffer, pH 7.2 (10 mM Tris–HCl, 1 mM EDTA, 50 mM NaCitrate, and 0.01% Triton X-100), stained with 0.5 μM SYTOX Green nucleic acid stain solution (Invitrogen), and then analysed using a BD Accuri C6 flow cytometer instrument (BD Biosciences). Flow cytometry data were acquired and analysed using CFlow Plus V1.0.264.15 software (Accuri Cytometers Inc.). A total of 20,000 cells were analysed from each biological sample. The forward scattering (FSC-A) and green fluorescence (FL1-A) parameters were used to estimate cell sizes and cell chromosome contents, respectively.
Results
ParA-ParB Interactions Are Conserved and Retained Between Homologues From the Same Phyla
While most bacteria employ the ParABS system to segregate their chromosomes, in some bacterial species, ParA and ParB are also involved in specific interactions with other proteins, particularly those polarly localised. Here, our aim was to understand the conservation of ParA and ParB interactions. We hypothesised that dimerisation of both ParA and ParB as well as ParA-ParB interactions are conserved and may be sustained even between proteins from distantly related species. On the other hand, we expected that the interactions with cell-pole-localised protein partners would be strictly species-specific. To explore the ParA engagement with protein partners, we chose proteins from four different microorganisms that possess the ParABS system. Two of them, M. smegmatis and C. glutamicum, are Gram-positive bacteria belonging to the Actinomycetota (formerly Actinobacteria) phylum, and two, C. crescentus and R. sphaeroides, are Gram-negative Pseudomonadota (formerly Proteobacteria).
First, we analysed the homology between the studied proteins. In both proteins ParA and ParB, the homology is highest within domains responsible for key roles in chromosome segregation (such as ATP binding, hydrolysis, DNA binding in ParA, and DNA binding in ParB; Figures 1A,B; Autret et al., 2001; Leonard et al., 2004, 2005; Hester and Lutkenhaus, 2007; Pióro et al., 2019; Jalal and Le, 2020; Kawalek et al., 2020). As expected, for both ParA and ParB homologues, proteins from species that belong to the same phylum are highly conserved (up to 66% identity and 80% similarity), while the homologues from distantly related species (e.g., C. crescentus and M. smegmatis) show lower identity (approximately 30–50%; Figure 1C). Interestingly, ParA proteins are more conserved than ParB proteins and modelling of three ParA homologues (C. crescentus, C. glutamicum, and M. smegmatis) shows high similarity of their spatial structures (Figure 1D). The main structural difference between ParA homologues results from 20 to 25 amino acid-long N-terminal extension in the Actinomycetota proteins. Interestingly, ParB proteins also differ substantially in the N-terminal region, which is the protein fragment engaged in interactions with ParA.
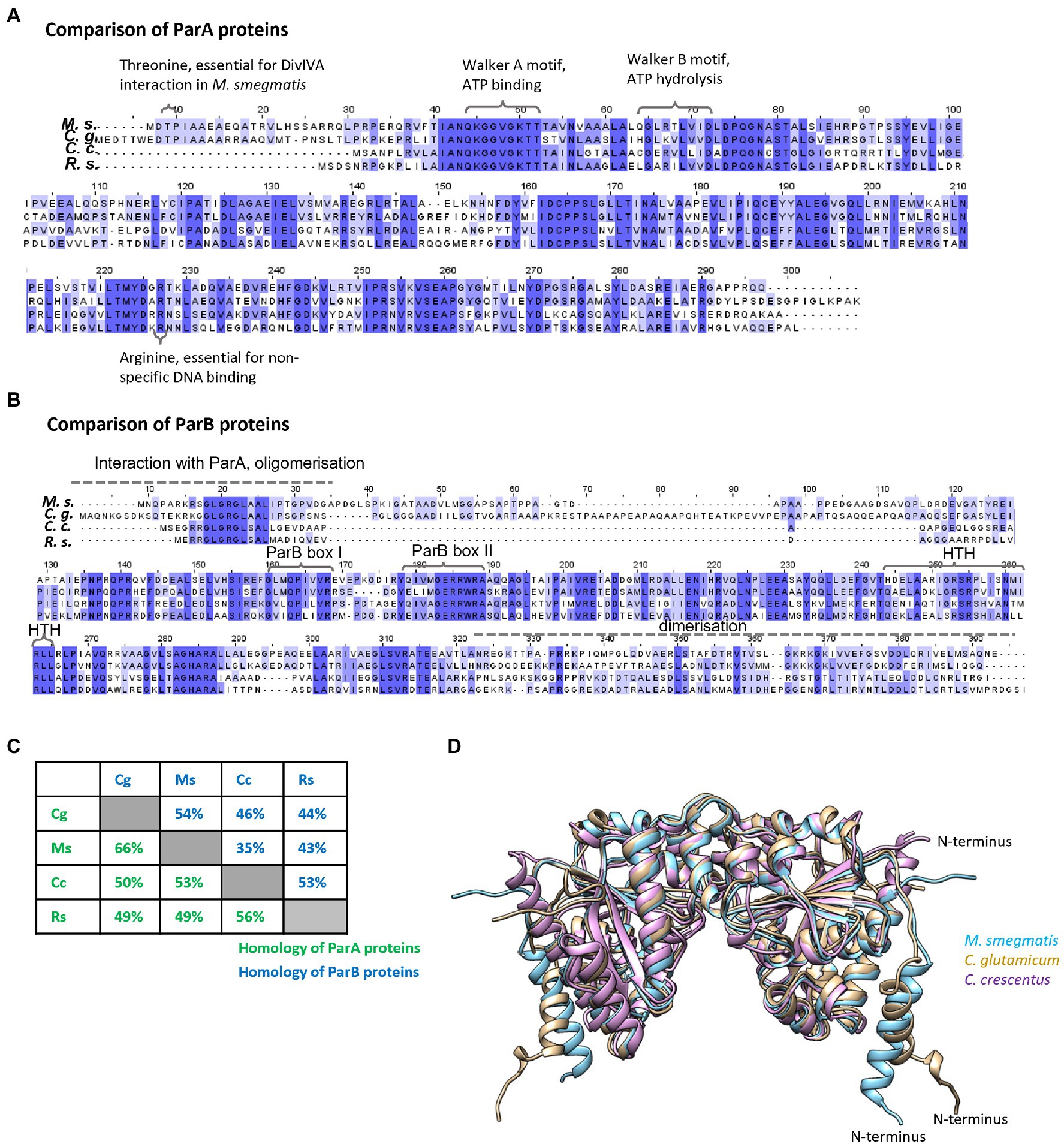
Figure 1. Homologies between ParA and ParB proteins from two Pseudomonadota species, Caulobacter crescentus and Rhodobacter sphaeroides, and two Actinomycetota species, Mycobacterium smegmatis and Corynebacterium glutamicum. (A) Sequence alignment of ParA proteins from M. smegmatis (Ms), C. glutamicum (Cg), and C. crescentus (Cc) and ParA1 from R. sphaeroides (Rs) aligned by Clustal Omega, visualised in Jalview, and coloured by BLOSOM62 score. Amino acids and motifs engaged in interactions are marked. (B) Sequence alignment of ParB proteins from M. smegmatis (Ms), C. glutamicum (Cg), and C. crescentus (Cc) and ParB1 from R. sphaeroides (Rs) aligned by Clustal Omega, visualised in Jalview, and coloured by BLOSOM62 score. Motifs engaged in interactions are marked. (C) Protein identities calculated in BLASTp. The green and blue numbers show identity between ParA and ParB proteins, respectively, from different species [M. smegmatis (Ms), C. glutamicum (Cg), C. crescentus (Cc), and R. sphaeroides (Rs)]. (D) The structure of ParA dimer proteins predicted by AlphaFold Colab (Jumper et al., 2021) compared and visualised by Chimera program (Pettersen et al., 2004). The blue colour indicates M. smegmatis ParA, brown—C. glutamicum ParA and violet—C. crescentus ParA. N-terminal of ParA from one monomer is marked.
To examine the specificity of the ParA and ParB protein interactions, we employed the bacterial two-hybrid (BTH) adenylate cyclase-based system in E. coli (Karimova et al., 1998). The BTH system was previously used in our studies to demonstrate the interaction of M. smegmatis ParA with DivIVA (Ginda et al., 2013). Escherichia coli does not possess the ParABS system, which facilitates the use of these bacteria as heterologous hosts for studies of segregation proteins. The analysed proteins were coproduced in E. coli as fusions with T18 and T25 adenylate cyclase subunits. Importantly, none of the genes encoding ParB homologue contained parS sequence. T18 fusions with T25 (vector only) or T25 fusions with T18 (empty vector) served as negative controls. Formation of the blue colonies on indicator media (LB-X-gal plates) results from restored adenylate cyclase activity and induction of the cAMP-CRP-dependent lac operon and suggests the interaction between T18 and T25 fusion proteins.
Colour of the colonies in which analysed proteins were coproduced indicated that all studied ParA and ParB proteins dimerise and they interact with their homologues from closely related species forming heterodimers (M. smegmatis with C. glutamicum proteins and R. sphaeroides with C. crescentus proteins; Figure 2A, within the black box), while interphyla interactions were less evident (pale blue colonies) or not detectable (Figure 2A). Similarly to dimerisation, the interactions between ParA and ParB were detected only for homologues from the same bacterial phyla (with one exception which is M. smegmatis ParA and R. sphaeroides ParB1 interaction). Thus, heterologous dimerisation and ParA-ParB interactions are preserved between the conserved proteins from bacteria from the same phyla.
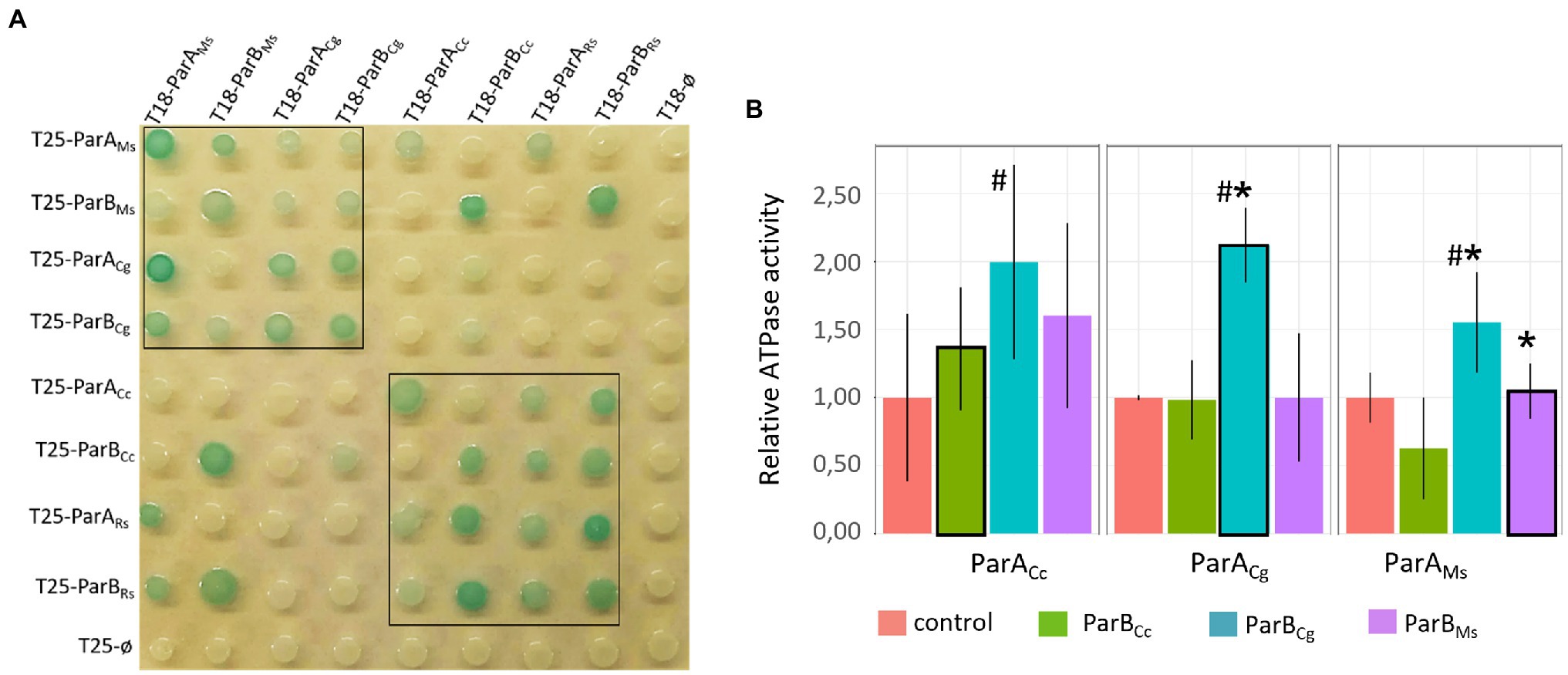
Figure 2. Intergenus interactions between ParA and ParB proteins.(A) Bacterial two-hybrid (BTH) assays testing heterodimerisation (ParA-ParA or ParB-ParB interactions) and interactions between ParA and ParB proteins from M. smegmatis (ParAMs, ParBMs), C. glutamicum (ParACg, ParBCg), C. crescentus (ParACc, ParBCc), and ParA1 and ParB1 from R. sphaeroides (ParARs, ParBRs) fused to either the T18 or T25 subdomain of adenylate cyclase (as indicated) expressed from pKT25 or pUT18C plasmids. Empty pKT25 and pUT18C vectors (T25Ø or T18Ø) served as negative controls. The blue colour of the colonies indicates interactions. The black box frames the colonies co-producing proteins from the same phyla. (B) ATPase assay measuring the influence of different ParB proteins [M. smegmatis (ParBMs), C. glutamicum (ParBCg), and C. crescentus (ParBCc)] on the ATPase activity of ParAs from M. smegmatis (ParAMs), C. glutamicum (ParACg), and C. crescentus (ParACc). ParBs showed no ATPase activity and ATPase activities of ParA proteins in absence of ParB (shown in Supplementary Figure S1A) were normalised to 1. The plot shows the average from three replicates with SE. Stimulation of the ATPase activity of ParA by cognate ParB is marked with black frames. Statistically significant differences are marked with # (p < 0.15, test T-Student). Asterisks indicate ParA-ParB interactions confirmed by BTH (A).
ParA ATPase activity was earlier shown to be stimulated by ParB (Easter and Gober, 2002). Thus, we used in vitro assay to measure the ATPase activity of selected ParA homologues (M. smegmatis, closely related C. glutamicum and distantly related C. crescentus) and to examine if it is stimulated by ParB proteins from closely or distantly related species. The ATPase assay of ParA alone indicated that M. smegmatis ParA has the strongest activity and C. crescentus ParA the weakest (Supplementary Figure S1A). We also confirmed that ParB proteins had the ability to weakly enhance activity of their cognate ParAs, but the stimulation varied dependent on the ParB-ParA pair tested (Figure 2B). Corynebacterium glutamicum ParB was most efficient at stimulation of ParA activity as it affected its cognate ParA as well as M. smegmatis ParA. Surprisingly, the ATPase activity of C. crescentus ParA was slightly increased by all studied ParB proteins.
To summarise, we demonstrated that both ParA and ParB homologues from the same bacterial phyla form heterodimers almost as efficiently as homodimers. However, the ParA-ParB interactions are sustained for proteins from closely related species. Moreover, these interactions may be sufficient to stimulate ATPase activity of ParA.
ParA and ParB Interactions With Polar Partner Proteins Are Genus Specific
Our earlier studies showed that the N-terminal region of M. smegmatis ParA is responsible for the interaction with the polar growth determinant DivIVA (Wag31; Pióro et al., 2019). Surprisingly, in closely related C. glutamicum, the DivIVA homologue was shown to interact with the ParB protein (Donovan et al., 2012). Interestingly, in C. crescentus and R. sphaeroides, ParB was shown to interact with MipZ (Thanbichler and Shapiro, 2006; Dubarry et al., 2019). Here, we set out to systematically analyse the interactions between ParA and ParB proteins from M. smegmatis, C. glutamicum, and C. crescentus as well as R. sphaeroides with polar partner proteins from the same and from other species using, as described above, BTH analysis (E. coli does not possess DivIVA and PopZ homologues).
Bacterial two-hybrid analysis revealed that M. smegmatis ParA interacted only with its cognate DivIVA and did not interact with C. glutamicum DivIVA or PopZ (Figure 3A). Markedly, the closely related C. glutamicum ParA did not interact with its cognate or with M. smegmatis DivIVA. We were also not able to detect an interaction between C. glutamicum ParB and its cognate DivIVA (this interaction was previously not detectable in BTH but was confirmed by another technique; Donovan et al., 2012) or M. smegmatis DivIVA. Surprisingly, C. crescentus ParB (but not ParA) interacted with all analysed polar partner proteins, i.e., both DivIVA homologues and PopZ, while R. sphaeroides ParB1 interacted only with M. smegmatis DivIVA, but not with PopZ (Figure 3A). Finally, C. crescentus and R. sphaeroides ParB, but not ParB from Actinomycetota, interacted with MipZ (Figure 3B). Moreover, BTH analysis showed that all studied polar partner proteins (both DivIVA and PopZ) form dimers or heterodimers (Supplementary Figure S1B). Thus, BTH analysis indicated that ParB homologues from C. crescentus and R. sphaeroides may interact with DivIVA but the interaction between ParA and DivIVA is specific for M. smegmatis proteins.
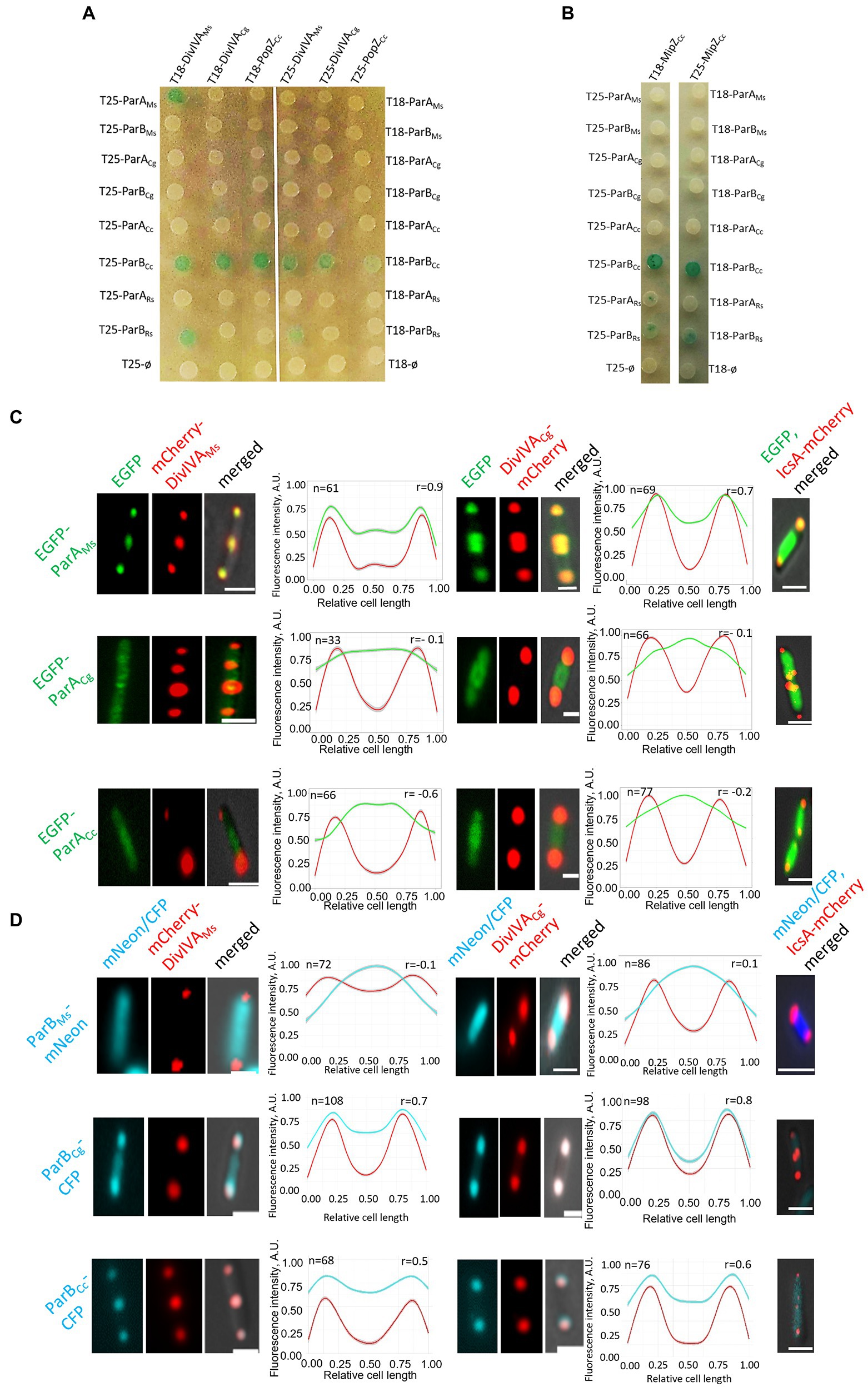
Figure 3. Genus-specific interactions between ParA or ParB proteins and polar partner proteins DivIVA and PopZ. (A) BTH assays testing interactions between M. smegmatis and C. glutamicum DivIVA (DivIVAMs and DivIVACg) or C. crescentus PopZ and ParA or ParB proteins from M. smegmatis (ParAMs, ParBMs), C. glutamicum (ParACg, ParBCg), C. cresentus (ParACc, ParBCc), as well as ParA1 and ParB1 from R. sphaeroides (ParARs, ParBRs) fused to either the T18 or T25 subdomain of adenylate cyclase (as indicated) expressed from pKT25 or pUT18C plasmids. Empty pKT25 and pUT18C vectors (T25Ø or T18Ø) served as negative controls. The blue colour of the colonies indicates interactions. (B) BTH assays testing the interaction between C. crescentus MipZ (MipZCc) and ParA or ParB proteins from M. smegmatis (ParAMs, ParBMs), C. glutamicum (ParACg, ParBCg), C. crescentus (ParACc, ParBCc) as well as ParA1 and ParB1 from R. sphaeroides (ParARs, ParBRs) fused to either the T18 or T25 subdomain of adenylate cyclase (as indicated) expressed from pKT25 or pUT18C plasmids. Empty pKT25 and pUT18C vectors (T25Ø or T18Ø) served as negative controls. The blue colour of the colonies indicates interactions. (C) Microscopy analysis of the colocalisation of ParA proteins from M. smegmatis (ParAMs), C. glutamicum (ParACg), to C. crescentus (ParACc) with M. smegmatis and C. glutamicum DivIVA (DivIVAMs and DivIVACg) in E. coli. Representative images of E. coli cells coproducing EGFP-ParA (green) with mCherry-DivIVA or Ics-mCherry (red) merged with DIC (grey). E. coli cells coproducing Ics-mCherry (red) and one of studied fusion proteins, as indicated, served as the negative control (right panel). Scale bar: 1 μm. Graphs show green and red fluorescence intensity profiles along the cell length (number of cells analysed indicated as n). Lines represent models fitted using a Loess algorithm implemented in the R program. The Pearson correlation coefficient “r,” as the measure of colocalisation, is indicated. (D) Microscopic colocalisation of ParB proteins from M. smegmatis (ParBMs), C. glutamicum (ParBCg), and C. crescentus (ParBCc) with M. smegmatis and C. glutamicum DivIVA (DivIVAMs and DivIVACg) in E. coli. Representative images of E. coli cells coproducing M. smegmatis ParB-mNeon, C. glutamicum and C. crescentus ParB-CFP and mCherry-DivIVA merged with DIC. E. coli cells coproducing Ics-mCherry (red) and one of studied fusion proteins, as indicated, served as the control the (right panel). Scale bar: 1 μm. Graphs show blue and red fluorescence intensity profiles along the cell length (number of cells analysed indicated as n). Lines represent models fitted using a Loess algorithm implemented in the R program. The Pearson correlation coefficient “r,” as the measure of colocalisation, is indicated.
Previous studies showed that the exchange of the third amino acid in M. smegmatis ParA (threonine exchange for alanine) abolished its interaction with its cognate DivIVA, suggesting that the extended N-terminal fragment of this ParA homologue is involved in DivIVA binding (Pióro et al., 2019). To check whether the N-terminal fragment of M. smegmatis ParA was sufficient to promote DivIVA binding by the other ParA homologue, we constructed a hybrid protein that consisted of an M. smegmatis N-terminal ParA fragment (amino acids 1–20) fused to R. sphaeroides ParA and tested the interaction of the hybrid protein with M. smegmatis DivIVA (Supplementary Figures S1C,D). BTH system studies showed that while hybrid protein dimerized, suggesting it was functional, the N-terminal fragment of M. smegmatis ParA was not sufficient to induce the R. sphaeroides ParA interaction with M. smegmatis DivIVA.
Since the previously shown interactions of C. glutamicum ParB and DivIVA were not detectable in BTH, as a complementary approach to study the interactions between actinobacterial segregation proteins and DivIVA, we analysed their colocalisation in E. coli cells. For this experiment, the studied proteins were tagged with fluorescent proteins (mCherry, mNeon, CFP, or EGFP), and their localisation was studied by fluorescence microscopy. The polarly localised Ics-mCherry fusion served as the negative control (Figures 3C,D; Supplementary Figure S1E; Ptacin et al., 2010).
Further analysis confirmed the colocalisation of M. smegmatis ParA with M. smegmatis DivIVA (Ginda et al., 2013) and showed its colocalisation with C. glutamicum DivIVA (the latter interaction not detected in BTH; Figure 3C). Markedly, the other studied ParA homologues (C. glutamicum and C. crescentus) did not colocalise with any of the analysed DivIVA homologues, confirming that the ParA-DivIVA interaction is a specific and unique feature of M. smegmatis proteins.
Additionally, E. coli microscopy studies showed that C. glutamicum ParB colocalised with C. glutamicum DivIVA, confirming earlier observations (Figure 3D; Donovan et al., 2012). Moreover, C. glutamicum ParB also colocalised partially with M. smegmatis DivIVA, suggesting that the ability to colocalise with DivIVA is an intrinsic feature of the C. glutamicum ParB protein and not DivIVA. Importantly, M. smegmatis ParB did not colocalise with any of the studied DivIVA homologues, consistent with the BTH result. Caulobacter crescentus ParB colocalised with both studied DivIVA homologues, confirming the results obtained with the BTH system. The negative control experiments showed that none of the analysed ParA and ParB homologues colocalised with polarly localised Ics-mCherry (Figures 3C,D; Supplementary Figure S1E).
In summary, analyses of ParA and ParB colocalisation in E. coli revealed that the interactions of M. smegmatis ParA with DivIVA are unique to this genus. Notably, DivIVA protein is also bound by the ParB homologue from C. crescentus as well as C. glutamicum ParB.
Overexpression of the Endogenous or Closely Related ParA Homologues Is Detrimental
Given that the interactions between ParA and ParB are retained for homologues from bacterial species belonging to the same phylum, but interactions with polar partner proteins are genus specific, we set out to investigate the importance of the latter interactions by testing the functionality of ParA protein homologues in heterologous hosts. To this end, we overproduced homologues from closely and distantly related species in wild type C. crescentus and M. smegmatis cells.
It was previously shown that overproduction of ParA in C. crescentus and M. smegmatis results in the formation of elongated cells and disturbed chromosome segregation (10% of anucleate cells; Mohl and Gober, 1997; Ginda et al., 2013). Here, we compared C. crescentus strains overproducing the endogenous ParA compared to those overproducing the most closely related ParA from R. sphaeroides and one of distantly related protein (M. smegmatis ParA) apart from wild type homologue (an additional gene copy expressed from a vanillate inducible promoter). Similarly, we analysed the growth of the M. smegmatis strain overproducing its own ParA homologue as compared to the strain overproducing closely related C. glutamicum or distantly related C. crescentus ParA (all parA genes were overexpressed in the wild-type background from the acetamide-induced promoter).
The influence of heterologous ParA proteins on C. crescentus growth and chromosome segregation was assayed during growth on solid medium with or without inducer and with serial dilutions of the studied strains (Figure 4A). This analysis showed that overproduction of the endogenous ParA and closely related R. sphaeroides homologue inhibited C. crescentus colony growth, while overproduction of M. smegmatis ParA had no effect. Microscopy analysis showed that cells of C. crescentus strains overproducing ParA from Alphaproteobacteria were much longer than those of the control strain (with empty vector; Figure 4B), confirming the results of growth analysis. Analysis of chromosome number by flow cytometry revealed that longer cells of strain overproducing C. crescentus and R. sphaeroides ParA contained an increased number of chromosomal copies (Figure 4C). Finally, we tested the influence of ParA overproduction on the polar localisation of MipZ-YFP in C. crescentus (Supplementary Figure S2). Consistent with the above-described results, overproduction of ParA from either C. crescentus or R. sphaeroides, but not M. smegmatis, affected the positioning of MipZ-YFP, leading to appearance of additional non-polar complexes. Thus, the overexpression of R. sphaeroides parA gene as additional parA copy had the same detrimental effect on C. crescentus growth as overexpression of the native gene.
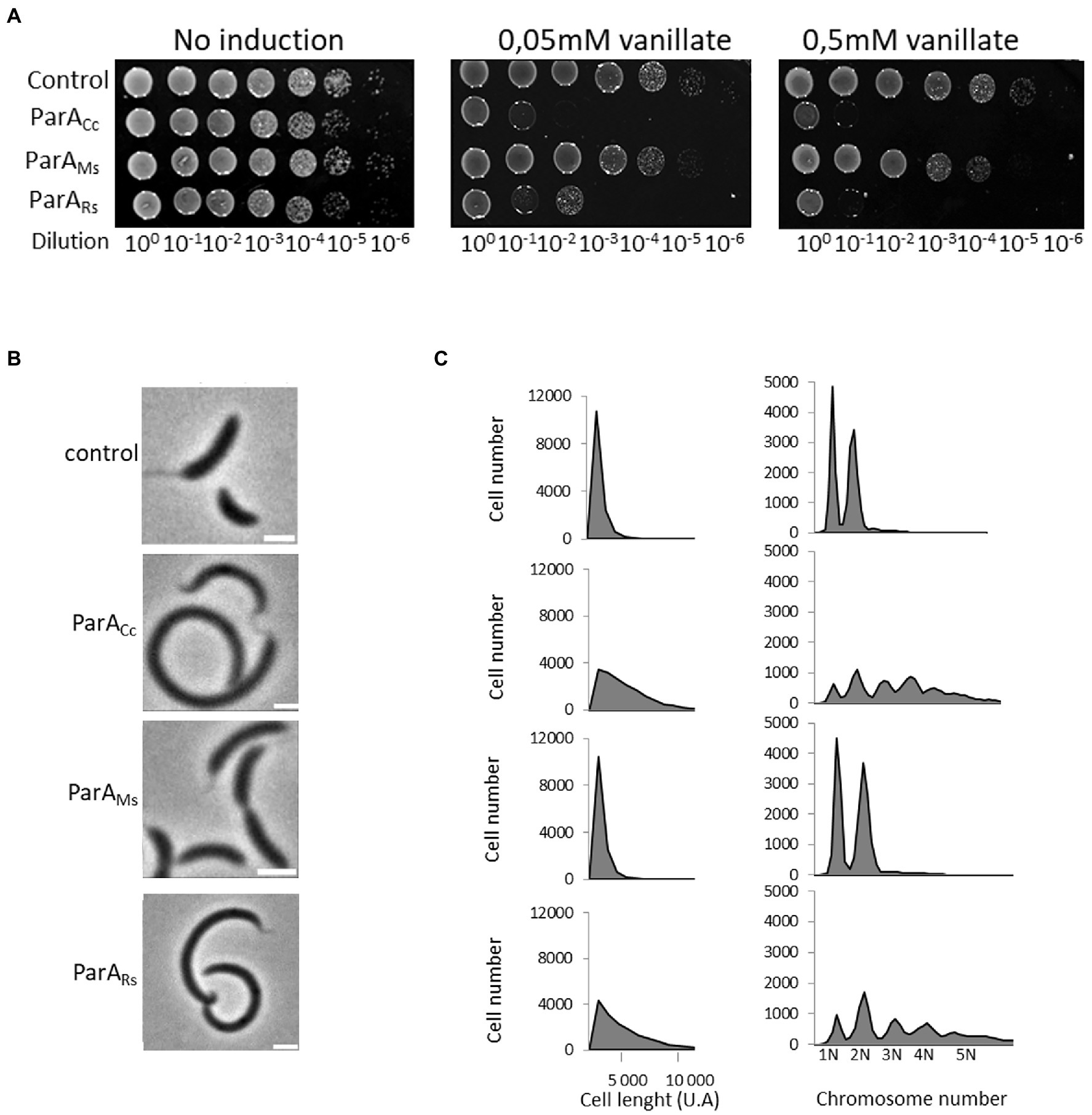
Figure 4. Negative effects of overproduction of the endogenous and closely related R. sphaeroides ParA on C. crescentus growth and cell morphology. (A) Spot plating assay to determine viability of C. crescentus strains overproducing M. smegmatis ParA (ParAMs) and R. sphaeroides ParA1 (ParARs) compared to the C. crescentus strain overproducing endogenous ParA (ParACc; all in wild type background and additional parA copy under the control of the vanillate promoter with and without induction, as indicated). The C. crescentus strain containing empty vector served as a control. (B) Phase contrast images of C. crescentus strains overproducing M. smegmatis ParA (ParAMs) and R. sphaeroides ParA1 (ParARs) compared to C. crescentus strain overproducing endogenous ParA (ParACc; as described above) and to the control strain with empty vector (control). Scale bar: 2 μm. (C) Flow cytometry profiles showing the cell length and chromosome numbers for C. crescentus strains overproducing M. smegmatis ParA (ParAMs) and R. sphaeroides ParA1 (ParARs) compared to the C. crescentus strain overproducing endogenous (ParACc; as described above) and to the control strain with empty vector (control).
Overproduction of all three ParA homologues (own and heterologous proteins) in M. smegmatis cells decreased the liquid culture growth rate although to various extents (Figure 5A). The most efficient inhibition of culture growth was observed when the endogenous ParA protein was overproduced, while overproduction of the related C. glutamicum ParA resulted in lesser growth inhibition. Overproduction of C. crescentus ParA also affected the M. smegmatis growth curve, most visibly during the later growth stage. Analysis of chromosome segregation (DNA staining with DAPI) showed that overproduction of C. glutamicum ParA had a milder effect than overproduction of M. smegmatis ParA, which is consistent with the effect on the growth curve (Figures 5A–C). Surprisingly, the largest effect on chromosome segregation was observed in M. smegmatis overproducing C. crescentus ParA (40% without DNA). Overproduction of M. smegmatis as well as C. crescentus ParA homologues also increased the M. smegmatis cell length.
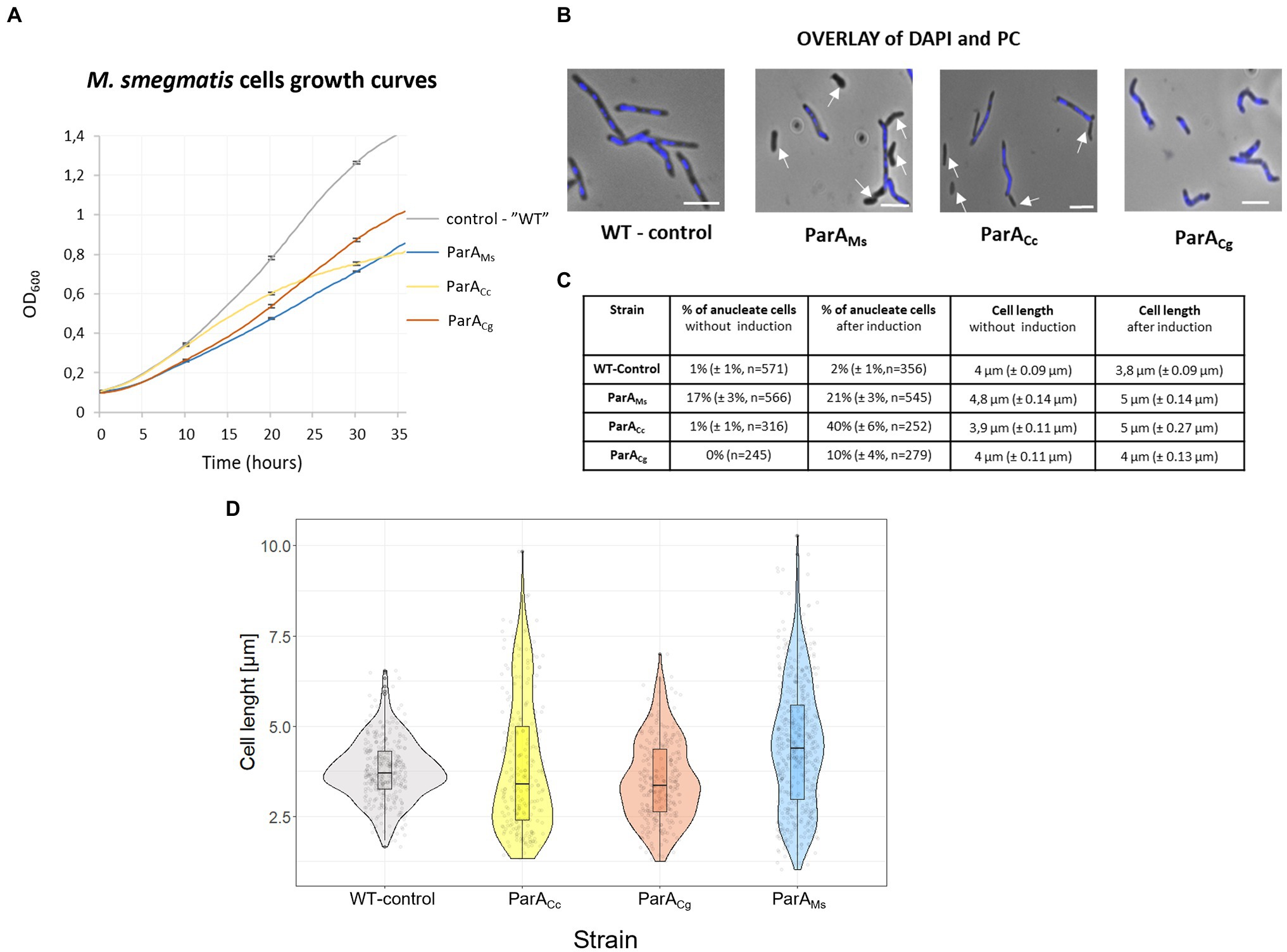
Figure 5. The negative effects of overproduction of the endogenous and heterologous (C. crescentus and C. glutamicum) ParA on M. smegmatis growth and cell morphology. (A) M. smegmatis culture growth rate of strains overproducing the endogenous (ParAMs), C. crescentus (ParACc), and C. glutamicum ParA (ParACg). parA was overexpressed from the parA gene (the additional gene copy under the pami promoter in the pMV vector in the wild type background) induced with 1% acetamide. M. smegmatis WT with empty vector pMVpami served as a control. The results represent the average of three independent experiments obtained using a Bioscreen C instrument. Bars indicate SEs. (B) M. smegmatis cells overproducing endogenous (ParAMs), C. crescentus (ParACc), or C. glutamicum ParA (ParACg; as descried above). Phase contrast images are merged with blue fluorescence of nucleoids stained with DAPI. WT with empty vector pMVpami served as the control. Scale bar: 3 μm. Arrows indicate anucleate cells. (C) Chromosome content and cell length of M. smegmatis cells overproducing endogenous (ParAMs), C. crescentus (ParACc), or C. glutamicum ParA (ParACg; as descried above) with and without overnight induction of 1% acetamide. M. smegmatis WT with empty vector pMVpami served as a control. Cell length was measured only in cells possessing DNA (stained with DAPI). The experiment was performed in two independent biological replicates. The number of calculated cells is indicated as n. The 95% of CI was indicated as ±. (D) Violin plot showing the length of M. smegmatis cells overproducing their endogenous (ParAMs), C. crescentus (ParACc) and C. glutamicum ParA (ParACg; as descried above) after overnight induction with 1% acetamide. M. smegmatis WT with empty vector pMVpami served as a control. The experiment was performed in two independent biological replicates. Cell length was measured in all cells (with and without nucleoids).
In summary, we demonstrated that C. crescentus growth is impaired by the overproduction of endogenous ParA as well as by closely related ParA homologue from R. sphaeroides. In M. smegmatis, the impact of overproduction was dependent on ParA homologues; the most detrimental was overproduction of endogenous protein, while overproduction of C. glutamicum homologue had lesser influence. This may reflect the ability of ParA homologues to engage with non-cognate ParB and indicate that some intracellular ParA interactions are maintained for closely related proteins.
Heterologous parA Does Not Complement Corynebacterium glutamicum and Mycobacterium smegmatis parA Mutants
While genes encoding ParA and ParB homologues are essential in C. crescentus, in C. glutamicum and M. smegmatis, both genes can be deleted. A deletion mutant of parA in M. smegmatis leads to slowed growth and the formation of anucleate cells (30% of anucleate cells; Ginda et al., 2013), while abolished ParA binding to DivIVA slightly decreased the average cell length and increased the cell elongation rate (Pióro et al., 2019). As indicated by experiments above, C. glutamicum ParA was partially functional in M. smegmatis cells, however it did not interact with DivIVA, and its affinity to ParB seemed to be lower than that of cognate ParA, all of which should be expected to diminish C. glutamicum ParA functionality in M. smegmatis. We sought to confirm these results by a trying to rescue the phenotype of a parA deletion by complementation with parA from C. glutamicum.
Analysis of the M. smegmatis parA deletion strain complemented with C. glutamicum parA showed that the production of heterologous ParA (Supplementary Figure S3) did not revert the mutant phenotype. The growth curve of M. smegmatis complemented with C. glutamicum parA was similar to that of the parA deletion strain (Figure 6A). Moreover, the induction of C. glutamicum ParA production did not restore the chromosome segregation defect compared to parA deletion (18% of cells were still anucleate with and without parA induction; Figures 6B,E). Complementation of M. smegmatis parA deletion with its own parA reduced segregation defect (3% anucleate cells after induction of parA gene expression). Thus, our experiment indicated that C. glutamicum ParA could not functionally substitute the endogenous ParA in M. smegmatis.
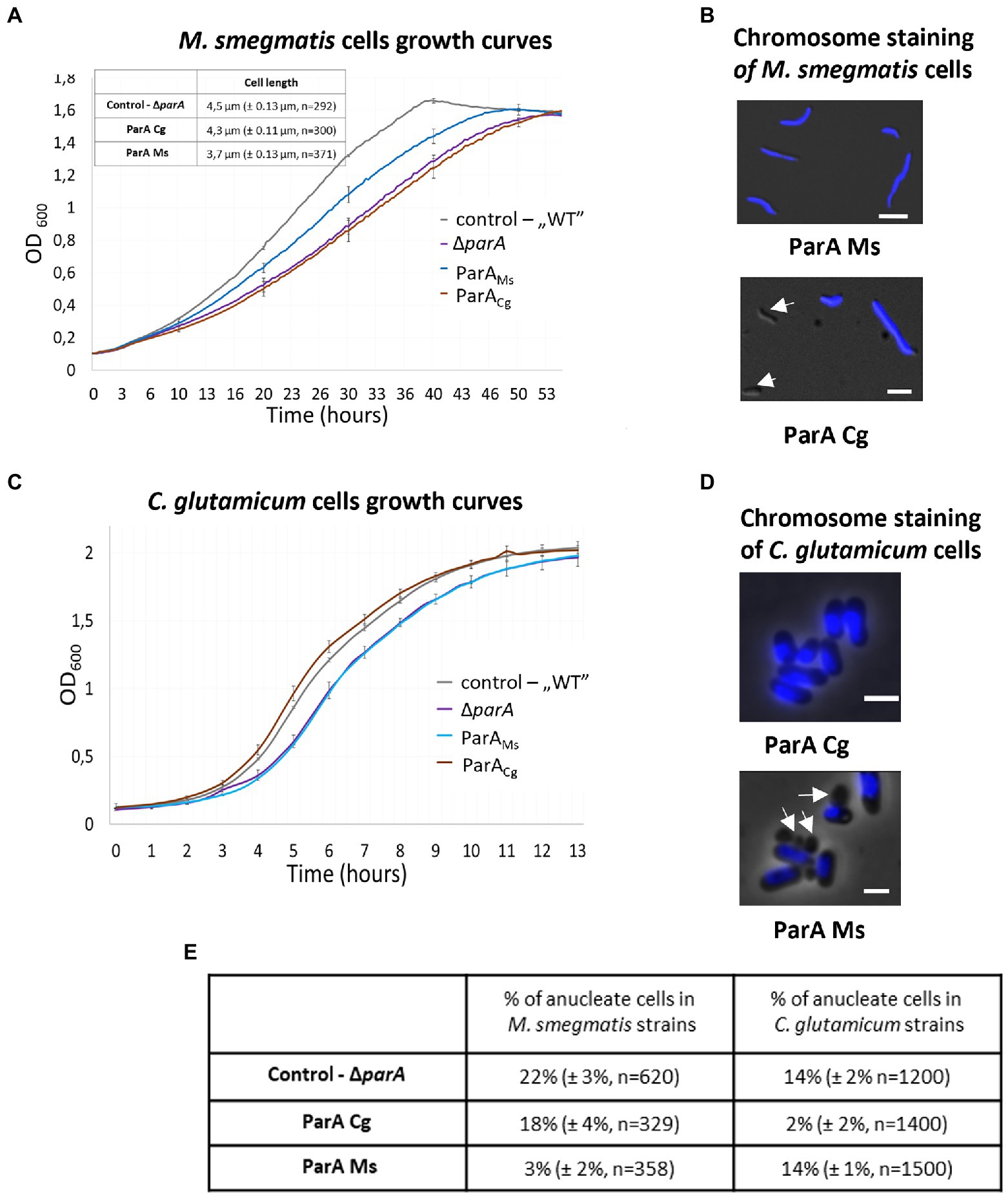
Figure 6. Lack of complementation of the C. glutamicum and M. smegmatis parA deletion strains with the heterologous parA. (A) Culture growth curves of the M. smegmatis parA deletion strain complemented with its own (ParAMs) and C. glutamicum (ParACg) parA gene. M. smegmatis WT with empty vector pMVpami and parA mutant served as controls. The results represent the average of three independent experiments obtained using a Bioscreen C instrument. Bars indicate SEs. Inset: cell length of M. smegmatis strains obtained by microscopy. M. smegmatis ΔparA served as a control (the number of cells analysed, n, is indicated). (B) Microscopic images of M. smegmatis parA mutants complemented with its own (ParAMs) and C. glutamicum (ParACg) parA gene. Blue fluorescence of nucleoids stained with DAPI is merged with DIC. Arrows indicate anucleate cells. Scale bar: 2 μm. (C) Culture growth curves of C. glutamicum parA mutants complemented with its own (ParACg) and M. smegmatis (ParAMs) parA gene. C. glutamicum WT with empty vector pEKEX and parA mutant served as controls. The results represent the average of three independent experiments obtained using a Bioscreen C instrument. Bars indicate SEs. (D) Microscopy images of the C. glutamicum parA deletion strain complemented with its own (ParACg) and M. smegmatis (ParAMs) parA gene. DIC images are merged with blue fluorescence of nucleoids stained with Hoechst3342. Arrows indicate anucleate cells. Scale bar: 2 μm. (E) The percentage of anucleate cells identified in the images of the DAPI-stained cells of analysed M. smegmatis and C. glutamicum parA mutant strains complemented with M. smegmatis (ParAMs) and C. glutamicum (ParACg) parA. Deletion strains served as control. The experiment was performed in two independent biological replicates. The number of calculated cells is indicated as n. The 95% of CI was indicated as ±.
We also tested reciprocal complementation of the C. glutamicum parA deletion strain by M. smegmatis parA (Supplementary Figure S3). In C. glutamicum, parA deletion slows growth, leads to formation anucleate cells (16% in LB medium) and alters cell length (Donovan et al., 2010). Knowing that M. smegmatis ParA interacts with C. glutamicum ParB and that its ATPase activity is stimulated by this interaction, we expected that it should functionally complement parA deletion in C. glutamicum, even though unlike their native ParA it has ability to bind DivIVA.
We found that M. smegmatis parA did not complement C. glutamicum parA deletion. The growth curve of the strain with induced expression of M. smegmatis parA was similar to the growth curve of the parA deletion strain (Figure 6C). The number of anucleate cells in the C. glutamicum parA deletion complemented with M. smegmatis parA was the same as that in the parA deletion strain (approximately 14% with and without parA induction). In contrast, induced expression of C. glutamicum parA reverted parA deletion phenotype (number of anucleate cells lowered to 2%; Figures 6D,E). Interestingly, although the number of anucleate cells in the C. glutamicum parA deletion strain complemented with M. smegmatis parA was the same as that in the parA deletion, the fractions of cells with 1 and 2 ParB-YPet complexes differed significantly, with more cells with 1 or 2 ParB complexes (34 and 44%, respectively) observed in the complemented strain than in the parA deletion strain (17 and 35%; Supplementary Figure S4). This may indicate that the production of M. smegmatis ParA in C. glutamicum may impair the overlapping chromosome replication cycles characteristic of C. glutamicum cells.
In summary, the complementation analyses show that even highly homologous ParA proteins, whose heterologous interactions are at least partially sustained, are not functional enough to convey chromosome segregation. This observation highlights the significance of all the species-specific interactions of chromosome segregation proteins.
Discussion
The bacterial chromosome segregation proteins ParA and ParB from various bacteria, although share basic biochemical properties, exhibit the ability to engage in unique, species-specific interactions. Our aim was to explore how conserved the interactions are between ParA and ParB and to determine whether they are sufficient to sustain the in vivo functionality of heterologous proteins. It should be kept in mind that using E. coli systems (BTH, co-localisation studies) for interaction studies may enhance or diminish the intermolecular interactions due to the lack of the biological context. However, application of two complementary systems allowed us to study combinations of interactions and delivered consistent results. Moreover, the application of the native strains as the host in overexpression or complementation analysis delivered information on the exogenous protein functionality (Table 1).
We showed that ParA and ParB proteins from different bacterial species form heterodimers (or higher order oligomers), particularly protein pairs from the same bacterial phylum. The ParA-ParB interactions are predominantly maintained within pairs of proteins from the same bacterial phyla. While these observations are consistent with the expectations, they are not fully supported by the observed influence of ParB on the ATPase activity of ParA. The difference in the N-terminal fragment of ParB, which was shown to be engaged in interaction with ParA (Autret et al., 2001), may account for the observed differences in ParB-ParA interactions. ParB ability to interact with ParA is supposed to affect formation of ParA gradient and efficiency of chromosome segregation (Vecchiarelli et al., 2014). Our analysis suggests the species-specific tuning of ParB—ParA interactions. Recently similar significance of species dependent interactions between ParB and SMC was reported (Bock et al., 2021).
An interesting feature of the ParAB system is its species-specific adjustment to interactions with polarly localised proteins. While in M. smegmatis ParA interacts with polar DivIVA, the closely related C. glutamicum ParA does not share this ability. In contrast, as shown before (Donovan et al., 2012) in C. glutamicum, ParB is involved in the interaction with DivIVA. We also detected ability of C. crescentus and R. sphaeroides ParB to interact with DivIVA. This interaction may result from similar structural features of DivIVA and PopZ, namely their ability to form higher order branched filamentous superstructure (Bowman et al., 2013; Holmes et al., 2016). We expected that the N-terminal extension of M. smegmatis ParA may play a role in species-specific interactions with DivIVA. Notably, 20 N-terminal amino acids in M. smegmatis ParA are not present in ParA homologues from other phyla and are only partially similar to the elongated N-terminus of C. glutamicum ParA. Previously, we showed that the exchange of the third amino acid in ParA abolishes its binding to DivIVA (Pióro et al., 2019). However, simply fusing 20 N-terminal amino acids from M. smegmatis ParA to R. sphaeroides protein did not promote its interactions with M. smegmatis DivIVA, indicating that the interaction interface is more complex and extends beyond the N-terminal fragment of M. smegmatis ParA. Markedly, M. smegmatis ParA is recruited to both M. smegmatis and C. glutamicum DivIVA. However, M. smegmatis DivIVA also weakly interacted with C. glutamicum ParB, which efficiently bound its cognate DivIVA. This indicates that the ability to bind DivIVA seems to be an intrinsic feature of the ParA or ParB protein.
The nonpreserved interactions between ParA and ParB from different bacterial phyla presumably account for the lack of functionality of distantly related homologues in heterologous systems (Figure 7). While the effects of overproduction of the highly homologous protein are similar to the effects of overproduction of cognate protein, although they may be less enhanced, the overproduction of distantly related proteins, i.e., M. smegmatis in C. crescentus, had no effect on growth and chromosome segregation. An exception to this rule was the toxic effect observed upon overproduction of C. crescentus ParA in the M. smegmatis background.
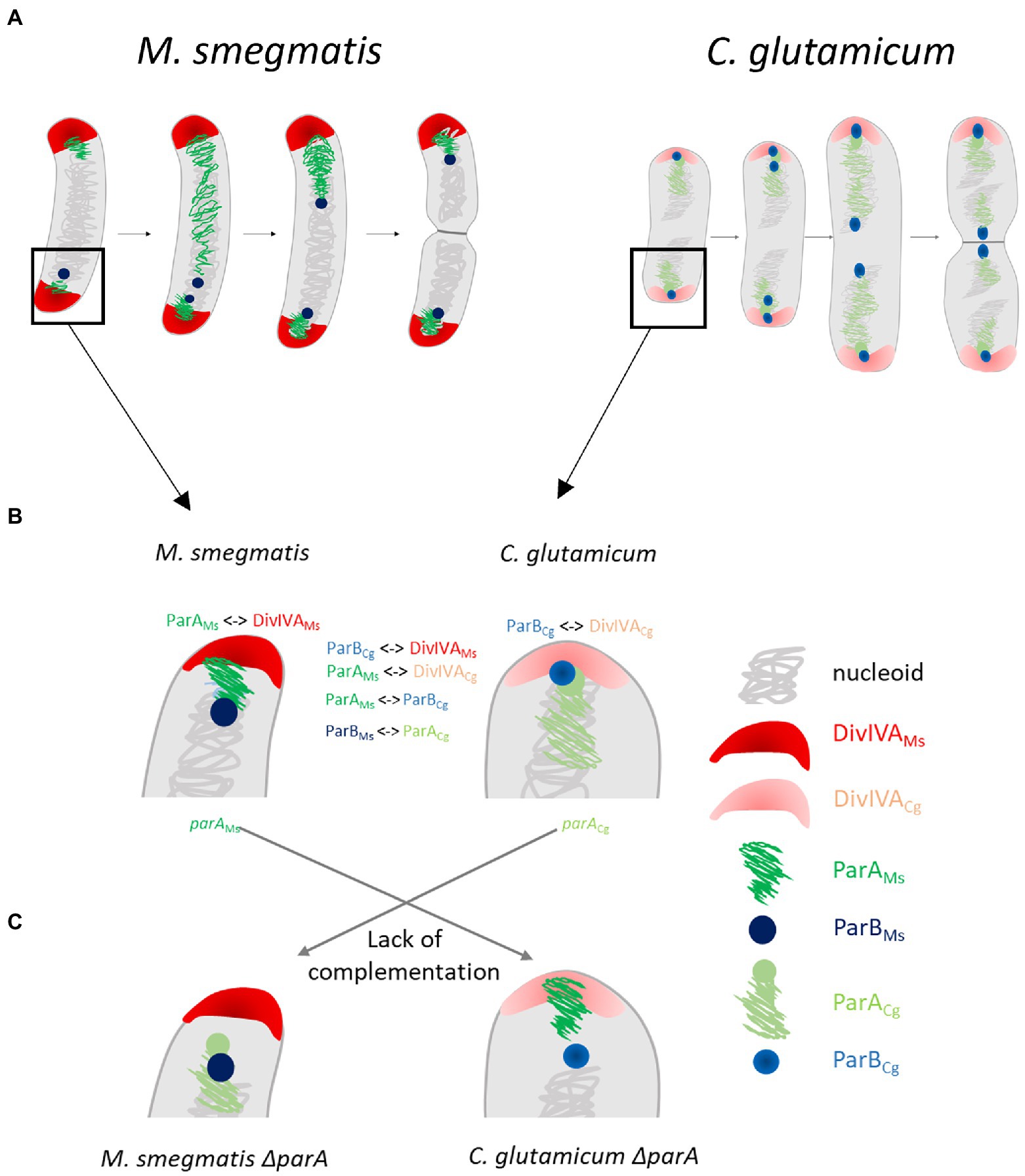
Figure 7. The species specific interactions of segregation and polarly localised proteins in Actinobacteria are critical for efficient chromosome segregation and may be linked with the different oriC positioning. (A) Scheme of differences in oriC positioning in closely related Mycobacterium smegmatis and Corynebacterium glutamicum cells which undergo non-overlapping (1 or 2 oriCs in cell in some distance from the pole) or overlapping rounds of replication (2 or 4 oriCs, polarly localised), respectively. (B) The interactions of segregation proteins and polar partner proteins (marked with symbol <−>) and detailed oriC positioning (polar or in distance to the pole). (C) The lack of complementation of parA deletion phenotype with heterologous parA.
Markedly, C. glutamicum ParA was not able to functionally substitute for the M. smegmatis homologue and in any extent did not restore the parA deletion phenotype. This finding could be considered surprising since C. glutamicum ParA is highly homologous to M. smegmatis protein, it interacted with M. smegmatis ParB in BTH system and its overproduction influenced M. smegmatis growth, similarly as overproduction of native protein even though to a lesser extent. Moreover, the lack of interaction between C. glutamicum ParA and M. smegmatis DivIVA cannot account for the severe segregation defect of the complemented strain since disruption of the DivIVA-ParA interaction was shown to only modestly affect chromosome segregation (Pióro et al., 2019). Speculatively, the lack of complementation could be explained by the inefficient enhancement of C. glutamicum ParA ATPase activity by M. smegmatis ParB.
However, even more surprisingly, the C. glutamicum parA deletion strain could not be complemented by homologous M. smegmatis ParA. The M. smegmatis ParA could be expected to be able to functionally substitute C. glutamicum ParA since its ATPase activity was somewhat enhanced by C. glutamicum ParB. However, M. smegmatis ParA, unlike C. glutamicum ParA, was shown to interact with C. glutamicum DivIVA, which might disturb the protein interaction network, specifically ParB-DivIVA interactions. This may suggest that all the complex interactions of segregation proteins, including those with polar protein partners, are crucial for their full functionality in vivo.
The surprising difference in DivIVA interaction partners (ParA in M. smegmatis and ParB in C. glutamicum) may explain not only the lack of heterologous protein functionality but also the different oriC region positions in these closely related species. It is worth mentioning that genus-specific interactions of ParA with TipN and PopZ in C. crescentus or bactofilins in Myxoccocus xanthus are crucial for specific oriC positioning (Ptacin et al., 2010; Schofield et al., 2010; Lin et al., 2017). Thus, in fast-growing C. glutamicum with overlapping replication cycles, ParB anchors oriC close to the cell pole (Donovan et al., 2012; Böhm et al., 2017), while in relatively slow-growing M. smegmatis, ParA recruitment to DivIVA positions oriC at some distance from the pole (Figure 7; Ginda et al., 2013; Pióro et al., 2019). Speculatively, ParA or, alternatively, ParB interaction with DivIVA may be one of the cell cycle checkpoints. This notion may be supported by the change in the number of ParB complexes in C. glutamicum producing M. smegmatis ParA instead of their own homologue. In those cells, ParA would likely be recruited to DivIVA, possibly affecting the DivIVA-ParB interaction and speculatively modifying the cell cycle. Thus, we infer that ParA or ParB interactions with DivIVA may be related to the requirements of the cell cycle or may even manifest some cell cycle features.
Our studies constitute the first interspecies analysis of segregation proteins and polarly localised protein interactions. We show the significance of the fine-tuning of the ParB-ParA interaction, which may be related to the stimulation of ParA ATPase activity. We also demonstrate that genus-specific recruitment of ParA or ParB to DivIVA is critical for efficient chromosome segregation and growth. Our studies reveal the interaction network of segregation proteins that is critical to their function and demonstrate that even closely related ParA and ParB homologues are engaged in genus-specific interactions related to specific requirements of the particular bacterial species cell cycle.
Data Availability Statement
The original contributions presented in the study are included in the article/Supplementary Material, further inquiries can be directed to the corresponding authors.
Author Contributions
MP, MBr, and DJ contributed to conception and design of the study. MP wrote original draft, performed analysis, and visualized data. MP, IM, TŁ, AG, and PJ performed experiments. KB, MBr, JA, PV, and MBe delivered resources. MP, IM, and DJ supervised students. PV, MBr, and DJ reviewed and edited manuscript. DJ administrated project and raised funds. All authors contributed to the article and approved the submitted version.
Funding
This work was funded by OPUS grant 2017/27/B/NZ1/00823 and partially by Harmonia grant 2014/14/M/NZ1/00076 (results presented in Figure 5; Supplementary Figure S2) from the National Science Centre, Poland. DJ visit to the Marc Bramkamp laboratory at Ludwig Maximilian University, Munich, was funded by the Polish National Agency for Academic Exchange (NAWA, project number PPN/BEK/2018/1/00399). MB acknowledges funding from Deutsche Forschungsgemeinschaft (DFG) grant BR2915/6-2. The cost of publication was financed by University of Wrocław.
Conflict of Interest
The authors declare that the research was conducted in the absence of any commercial or financial relationships that could be construed as a potential conflict of interest.
Publisher’s Note
All claims expressed in this article are solely those of the authors and do not necessarily represent those of their affiliated organizations, or those of the publisher, the editors and the reviewers. Any product that may be evaluated in this article, or claim that may be made by its manufacturer, is not guaranteed or endorsed by the publisher.
Acknowledgments
We are grateful to Martin Thanbichler for the pET21aparACc construct and to Christopher W. Jones from Oxford University for help in the laboratory. We are thankful to Bernhard Kepplinger for critical reading and comments on the manuscript and Agnieszka Strzałka for help with R programming and statistical analyses.
Supplementary Material
The Supplementary Material for this article can be found online at: https://www.frontiersin.org/articles/10.3389/fmicb.2022.928139/full#supplementary-material
Supplementary Figure S1 | ParA protein interactions. (A) ATPase activity of sole ParA proteins measured in μmoles of free phosphate/min/L (U/L) according to the manufacturer’s protocol (Sigma-Aldrich), indicating the greatest activity of the Mycobacterium smegmatis ParA protein and the lowest activity in the case of the C. crescentus ParA protein. (B) BTH assays testing interactions between M. smegmatis (DivIVAMs) and Corynebacterium glutamicum (DivIVACg) DivIVA as well as C. crescentus (PopZCc) PopZ proteins fused to either the T18 or T25 subdomain of adenylate cyclase (Cya, as indicated) expressed from pKT25 or pUT18C plasmids. Empty pKT25 and pUT18C vectors (T25Ø or T18Ø) served as negative controls. The blue colour of the colonies indicates interactions. (C) Amino acid sequences of the N-terminus regions of the M. smegmatis and R. sphaeroides ParA proteins. The hybrid protein consists of the first 20 amino acids of M. smegmatis ParA (marked in blue), and the remaining protein is the R. sphaeroides sequence. (D) BTH assays testing the interaction of hydrid ParA fused with the T25 subdomain of adenylate cyclase with M. smegmatis DivIVA (DivIVAMs) or ParA (ParAMs) fused with T18. The positive control is M. smegmatis T25-ParA with T18-DivIVA. The blue colour of the colonies indicates interactions. (E) Lack of colocalisation of M. smegmatis EGFP-ParA and ParB-mNeon (ParAMs, ParBMs), C. glutamicum EGFP-ParA, ParB-CFP (ParACg, ParBCg), and C. crescentus EGFP-ParA, ParB-CFP (ParACc, ParBCc) with IcsA-mCherry. Graphs show green/blue and red fluorescence intensity profiles along the cell length (number of cells analysed indicated as n). Lines represent models fitted using a Loess algorithm implemented in the R program. The Pearson correlation coefficient “r,” as the measurement of colocalisation, is indicated.
Supplementary Figure S2 | Localisation of MipZ-YFP in C. crescentus cells. C. crescentus cells producing MipZ-YFP and overexpressing C. crescentus (ParACc), Mycobacterium smegmatis (ParAMs) parA genes or R. sphaeroides parA1 (ParARs) with and without induction with vannilate. Florescence images of MipZ-YPF fluorescence (yellow) merged with DIC. C. crescentus strain MT97 served as a control. Scale bar 2 μm.
Supplementary Figure S3 | Confirmation of ParA proteins production in Mycobacterium smegmatis and Corynebacterium glutamicum strain. (A) Western blot analysis of M. smegmatis ΔparA strains overexpressing own (Ms) and heterologous parA from C. glutamicum (Cg). ΔparA strain and mc2 served as controls. Strains were cultured overnight with 10% acetamide induction (marked as +) or without (−). Cells were lysed by sonication and 100 μg of total proteins were subjected to 10% SDS-PAGE electrophoresis and then transferred on nitrocellulose blotting membrane. The membrane was incubated with rabbit serum containing anti ParAMs antibody. As secondary antibody anti-rabbit IgG conjugated with alkaline phosphatase was used. Prestained protein ladder (Thermo Scientific) was used as a marker (marked as M). (B) Western blot analysis of cell lysate from C. glutamicum ΔparA strains complemented with own (ParACg) or heterologous parA from M. smegmatis (ParAMs), two clones of each strain were analysed. C. glutamicum ΔparA strain was used as the control (ΔparA). Cultures at logarithmic phase were induced with 0.05 mM IPTG for 2 h. Cells were lysed by sonication and 100 μg of proteins were subjected to 10% SDS-PAGE electrophoresis and then transferred on nitrocellulose blotting membrane. The membrane was incubated with rabbit serum containing anti-ParAMs antibody. As secondary antibody anti-rabbit IgG conjugated with alkaline phosphatase was used. Prestained protein ladder Thermo Scientific was used as a marker (marked as M).
Supplementary Figure S4 | ParB complexes in Corynebacterium glutamicum parA mutant strains. C. glutamicum parA deletion strain complemented with native parA gene (ParACg) and Mycobacterium smegmatis parA (ParAMs). Upper panel: microscopy images of examples of C. glutamicum cells producing ParB-YPet (yellow) with membranes stained with FM4-64 (red). Lower panel: percentage of cells possessing a specific number of ParB complexes. Scale bar: 1 μm.
References
Autret, S., Nair, R., and Errington, J. (2001). Genetic analysis of the chromosome segregation protein Spo0J of Bacillus subtilis: evidence for separate domains involved in DNA binding and interactions with Soj protein. Mol. Microbiol. 41, 743–755. doi: 10.1046/j.1365-2958.2001.02551.x
Babl, L., Giacomelli, G., Ramm, B., Gelmroth, A.-K., Bramkamp, M., and Schwille, P. (2022). CTP-controlled liquid-liquid phase separation of ParB. J. Mol. Biol. 434:167401. doi: 10.1016/j.jmb.2021.167401
Bates, D., and Kleckner, N. (2005). Chromosome and replisome dynamics in E. coli: loss of sister cohesion triggers global chromosome movement and mediates chromosome segregation. Cell 121, 899–911. doi: 10.1016/j.cell.2005.04.013
Bock, F. P., Anchimiuk, A., Diebold-Durand, M. -L., and Gruber, S. (2021). The ParB clamp docks onto Smc for DNA loading via a joint-ParB interface. biorxiv [Preprint]. doi: 10.1101/2021.12.15.472096
Böhm, K., Giacomelli, G., Schmidt, A., Imhof, A., Koszul, R., Marbouty, M., et al. (2020). Chromosome organization by a conserved condensin-ParB system in the actinobacterium Corynebacterium glutamicum. Nat. Commun. 11:1485. doi: 10.1038/s41467-020-15238-4
Böhm, K., Meyer, F., Rhomberg, A., Kalinowski, J., Donovan, C., and Bramkamp, M. (2017). Novel chromosome organization pattern in Actinomycetales—overlapping replication cycles combined with diploidy. MBio 8, e00511–e005117. doi: 10.1128/mBio.00511-17
Bowman, G. R., Perez, A. M., Ptacin, J. L., Ighodaro, E., Folta-Stogniew, E., Comolli, L. R., et al. (2013). Oligomerization and higher-order assembly contribute to sub-cellular localization of a bacterial scaffold. Mol. Microbiol. 90, 776–795. doi: 10.1111/mmi.12398
Chan, H., Söderström, B., and Skoglund, U. (2020). Spo0J and SMC are required for normal chromosome segregation in Staphylococcus aureus. MicrobiologyOpen 9:e999. doi: 10.1002/mbo3.999
Donovan, C., Schwaiger, A., Krämer, R., and Bramkamp, M. (2010). Subcellular localization and characterization of the ParAB system from Corynebacterium glutamicum. J. Bacteriol. 192, 3441–3451. doi: 10.1128/JB.00214-10
Donovan, C., Sieger, B., Krämer, R., and Bramkamp, M. (2012). A synthetic Escherichia coli system identifies a conserved origin tethering factor in Actinobacteria. Mol. Microbiol. 84, 105–116. doi: 10.1111/j.1365-2958.2012.08011.x
Dubarry, N., Willis, C. R., Ball, G., Lesterlin, C., and Armitage, J. P. (2019). In vivo imaging of the segregation of the 2 chromosomes and the cell division proteins of Rhodobacter sphaeroides reveals an unexpected role for MipZ. MBio 10, e02515–e02518. doi: 10.1128/mBio.02515-18
Easter, J., and Gober, J. W. (2002). ParB-stimulated nucleotide exchange regulates a switch in functionally distinct ParA activities. Mol. Cell 10, 427–434. doi: 10.1016/S1097-2765(02)00594-4
Ginda, K., Bezulska, M., Ziółkiewicz, M., Dziadek, J., Zakrzewska-Czerwińska, J., and Jakimowicz, D. (2013). ParA of Mycobacterium smegmatis co-ordinates chromosome segregation with the cell cycle and interacts with the polar growth determinant DivIVA. Mol. Microbiol. 87, 998–1012. doi: 10.1111/mmi.12146
Ginda, K., Santi, I., Bousbaine, D., Zakrzewska-Czerwińska, J., Jakimowicz, D., and McKinney, J. (2017). The studies of ParA and ParB dynamics reveal asymmetry of chromosome segregation in mycobacteria. Mol. Microbiol. 105, 453–468. doi: 10.1111/mmi.13712
Gruber, S., and Errington, J. (2009). Recruitment of condensin to replication origin regions by ParB/SpoOJ promotes chromosome segregation in B. subtilis. Cell 137, 685–696. doi: 10.1016/j.cell.2009.02.035
Hester, C. M., and Lutkenhaus, J. (2007). Soj (ParA) DNA binding is mediated by conserved arginines and is essential for plasmid segregation. Proc. Natl. Acad. Sci. U. S. A. 104, 20326–20331. doi: 10.1073/pnas.0705196105
Holmes, J. A., Follett, S. E., Wang, H., Meadows, C. P., Varga, K., and Bowman, G. R. (2016). Caulobacter PopZ forms an intrinsically disordered hub in organizing bacterial cell poles. Proc. Natl. Acad. Sci. U. S. A. 113, 12490–12495. doi: 10.1073/pnas.1602380113
Hu, L., Vecchiarelli, A. G., Mizuuchi, K., Neuman, K. C., and Liu, J. (2017). Brownian ratchet mechanisms of ParA-mediated partitioning. Plasmid 92, 12–16. doi: 10.1016/j.plasmid.2017.05.002
Jakimowicz, D., Brzostek, A., Rumijowska-Galewicz, A., Żydek, P., Dołzbłasz, A., Smulczyk-Krawczyszyn, A., et al. (2007). Characterization of the mycobacterial chromosome segregation protein ParB and identification of its target in Mycobacterium smegmatis. Microbiol. Read. Engl. 153, 4050–4060. doi: 10.1099/mic.0.2007/011619-0
Jalal, A. S. B., and Le, T. B. K. (2020). Bacterial chromosome segregation by the ParABS system. Open Biol. 10:200097. doi: 10.1098/rsob.200097
Jalal, A. S., Tran, N. T., and Le, T. B. (2020). ParB spreading on DNA requires cytidine triphosphate in vitro. elife 9:e53515. doi: 10.7554/eLife.53515
Jensen, R. B., Wang, S. C., and Shapiro, L. (2001). A moving DNA replication factory in Caulobacter crescentus. EMBO J. 20, 4952–4963. doi: 10.1093/emboj/20.17.4952
Jumper, J., Evans, R., Pritzel, A., Green, T., Figurnov, M., Ronneberger, O., et al. (2021). Highly accurate protein structure prediction with AlphaFold. Nature 596, 583–589. doi: 10.1038/s41586-021-03819-2
Karimova, G., Pidoux, J., Ullmann, A., and Ladant, D. (1998). A bacterial two-hybrid system based on a reconstituted signal transduction pathway. Proc. Natl. Acad. Sci. 95, 5752–5756. doi: 10.1073/pnas.95.10.5752
Kawalek, A., Wawrzyniak, P., Bartosik, A. A., and Jagura-Burdzy, G. (2020). Rules and exceptions: the role of chromosomal ParB in DNA segregation and other cellular processes. Microorganisms 8:E105. doi: 10.3390/microorganisms8010105
Keilhauer, C., Eggeling, L., and Sahm, H. (1993). Isoleucine synthesis in Corynebacterium glutamicum: molecular analysis of the ilvB-ilvN-ilvC operon. J. Bacteriol. 175, 5595–5603. doi: 10.1128/jb.175.17.5595-5603.1993
Lemon, K. P., and Grossman, A. D. (2000). Movement of replicating DNA through a stationary replisome. Mol. Cell 6, 1321–1330. doi: 10.1016/S1097-2765(00)00130-1
Leonard, T. A., Butler, P. J. G., and Löwe, J. (2004). Structural analysis of the chromosome segregation protein Spo0J from Thermus thermophilus. Mol. Microbiol. 53, 419–432. doi: 10.1111/j.1365-2958.2004.04133.x
Leonard, T. A., Butler, P. J., and Löwe, J. (2005). Bacterial chromosome segregation: structure and DNA binding of the Soj dimer—a conserved biological switch. EMBO J. 24, 270–282. doi: 10.1038/sj.emboj.7600530
Lim, H. C., Surovtsev, I. V., Beltran, B. G., Huang, F., Bewersdorf, J., and Jacobs-Wagner, C. (2014). Evidence for a DNA-relay mechanism in ParABS-mediated chromosome segregation. elife 3:e02758. doi: 10.7554/eLife.02758
Lin, L., Osorio Valeriano, M., Harms, A., Søgaard-Andersen, L., and Thanbichler, M. (2017). Bactofilin-mediated organization of the ParABS chromosome segregation system in Myxococcus xanthus. Nat. Commun. 8:1817. doi: 10.1038/s41467-017-02015-z
Livny, J., Yamaichi, Y., and Waldor, M. K. (2007). Distribution of centromere-like parS sites in bacteria: insights from comparative genomics. J. Bacteriol. 189, 8693–8703. doi: 10.1128/JB.01239-07
Minnen, A., Attaiech, L., Thon, M., Gruber, S., and Veening, J.-W. (2011). SMC is recruited to oriC by ParB and promotes chromosome segregation in Streptococcus pneumoniae. Mol. Microbiol. 81, 676–688. doi: 10.1111/j.1365-2958.2011.07722.x
Mohl, D. A., and Gober, J. W. (1997). Cell cycle-dependent polar localization of chromosome partitioning proteins in Caulobacter crescentus. Cell 88, 675–684. doi: 10.1016/S0092-8674(00)81910-8
Osorio-Valeriano, M., Altegoer, F., Steinchen, W., Urban, S., Liu, Y., Bange, G., et al. (2019). ParB-type DNA segregation proteins are CTP-dependent molecular switches. Cell 179, 1512–1524.e15. doi: 10.1016/j.cell.2019.11.015
Pettersen, E. F., Goddard, T. D., Huang, C. C., Couch, G. S., Greenblatt, D. M., Meng, E. C., et al. (2004). UCSF chimera--a visualization system for exploratory research and analysis. J. Comput. Chem. 25, 1605–1612. doi: 10.1002/jcc.20084
Pióro, M., and Jakimowicz, D. (2020). Chromosome segregation proteins as coordinators of cell cycle in response to environmental conditions. Front. Microbiol. 11:588. doi: 10.3389/fmicb.2020.00588
Pióro, M., Małecki, T., Portas, M., Magierowska, I., Trojanowski, D., Sherratt, D., et al. (2019). Competition between DivIVA and the nucleoid for ParA binding promotes segrosome separation and modulates mycobacterial cell elongation. Mol. Microbiol. 111, 204–220. doi: 10.1111/mmi.14149
Ptacin, J. L., Gahlmann, A., Bowman, G. R., Perez, A. M., von Diezmann, L., Eckart, M. R., et al. (2014). Bacterial scaffold directs pole-specific centromere segregation. Proc. Natl. Acad. Sci. U. S. A. 111, E2046–E2055. doi: 10.1073/pnas.1405188111
Ptacin, J. L., Lee, S. F., Garner, E. C., Toro, E., Eckart, M., Comolli, L. R., et al. (2010). A spindle-like apparatus guides bacterial chromosome segregation. Nat. Cell Biol. 12, 791–798. doi: 10.1038/ncb2083
Reyes-Lamothe, R., Nicolas, E., and Sherratt, D. J. (2012). Chromosome replication and segregation in bacteria. Annu. Rev. Genet. 46, 121–143. doi: 10.1146/annurev-genet-110711-155421
Reyes-Lamothe, R., Possoz, C., Danilova, O., and Sherratt, D. J. (2008). Independent positioning and action of Escherichia coli replisomes in live cells. Cell 133, 90–102. doi: 10.1016/j.cell.2008.01.044
Sambrook, J., and Russell, D. W. (2001). Molecular Cloning: A Laboratory Manual. 3rd Edn. Vol. 1. New York: Cold Spring Harbour.
Schofield, W. B., Lim, H. C., and Jacobs-Wagner, C. (2010). Cell cycle coordination and regulation of bacterial chromosome segregation dynamics by polarly localized proteins. EMBO J. 29, 3068–3081. doi: 10.1038/emboj.2010.207
Soh, Y.-M., Davidson, I. F., Zamuner, S., Basquin, J., Bock, F. P., Taschner, M., et al. (2019). Self-organization of parS centromeres by the ParB CTP hydrolase. Science 366, 1129–1133. doi: 10.1126/science.aay3965
Sullivan, N. L., Marquis, K. A., and Rudner, D. Z. (2009). Recruitment of SMC by ParB-parS organizes the origin region and promotes efficient chromosome segregation. Cell 137, 697–707. doi: 10.1016/j.cell.2009.04.044
Thanbichler, M. (2010). Synchronization of chromosome dynamics and cell division in bacteria. Cold Spring Harb. Perspect. Biol. 2:a000331. doi: 10.1101/cshperspect.a000331
Thanbichler, M., and Shapiro, L. (2006). MipZ, a spatial regulator coordinating chromosome segregation with cell division in Caulobacter. Cell 126, 147–162. doi: 10.1016/j.cell.2006.05.038
Toro, E., Hong, S.-H., McAdams, H. H., and Shapiro, L. (2008). Caulobacter requires a dedicated mechanism to initiate chromosome segregation. Proc. Natl. Acad. Sci. U. S. A. 105, 15435–15440. doi: 10.1073/pnas.0807448105
Trojanowski, D., Ginda, K., Pióro, M., Hołówka, J., Skut, P., Jakimowicz, D., et al. (2015). Choreography of the Mycobacterium replication machinery during the cell cycle. MBio 6, e02125–e02114. doi: 10.1128/mBio.02125-14
Trojanowski, D., Hołówka, J., and Zakrzewska-Czerwińska, J. (2018). Where and when bacterial chromosome replication starts: a single cell perspective. Front. Microbiol. 9:2819. doi: 10.3389/fmicb.2018.02819
Vecchiarelli, A. G., Neuman, K. C., and Mizuuchi, K. (2014). A propagating ATPase gradient drives transport of surface-confined cellular cargo. Proc. Natl. Acad. Sci. U. S. A. 111, 4880–4885. doi: 10.1073/pnas.1401025111
Viollier, P. H., Thanbichler, M., McGrath, P. T., West, L., Meewan, M., McAdams, H. H., et al. (2004). Rapid and sequential movement of individual chromosomal loci to specific subcellular locations during bacterial DNA replication. Proc. Natl. Acad. Sci. U. S. A. 101, 9257–9262. doi: 10.1073/pnas.0402606101
Webb, C. D., Teleman, A., Gordon, S., Straight, A., Belmont, A., Lin, D. C., et al. (1997). Bipolar localization of the replication origin regions of chromosomes in vegetative and sporulating cells of B. subtilis. Cell 88, 667–674. doi: 10.1016/S0092-8674(00)81909-1
Keywords: segregation proteins, ParA, ParB, DivIVA, polar recruitment
Citation: Pióro M, Matusiak I, Gawek A, Łebkowski T, Jaroszek P, Bergé M, Böhm K, Armitage J, Viollier PH, Bramkamp M and Jakimowicz D (2022) Genus-Specific Interactions of Bacterial Chromosome Segregation Machinery Are Critical for Their Function. Front. Microbiol. 13:928139. doi: 10.3389/fmicb.2022.928139
Edited by:
Jörg Stülke, University of Göttingen, GermanyReviewed by:
Sven Halbedel, Robert Koch Institute (RKI), GermanySusan Schlimpert, John Innes Centre, United Kingdom
Stephan Gruber, University of Lausanne, Switzerland
Copyright © 2022 Pióro, Matusiak, Gawek, Łebkowski, Jaroszek, Bergé, Böhm, Armitage, Viollier, Bramkamp and Jakimowicz. This is an open-access article distributed under the terms of the Creative Commons Attribution License (CC BY). The use, distribution or reproduction in other forums is permitted, provided the original author(s) and the copyright owner(s) are credited and that the original publication in this journal is cited, in accordance with accepted academic practice. No use, distribution or reproduction is permitted which does not comply with these terms.
*Correspondence: Monika Pióro, bW9uaWthLnBpb3JvQHV3ci5lZHUucGw=; Dagmara Jakimowicz, ZGFnbWFyYS5qYWtpbW93aWN6QHV3ci5lZHUucGw=
†Present addresses: Matthieu Bergé, CNRS Laboratoire de Chimie Bactérienne LCB (UMR 7283), Aix-Marseille Université, Institut de Microbiologie de la Méditerranée, Marseille, France
Kati Böhm, Roche Diagnostics GmbH, Penzberg, Germany