- 1Photosynthesis Research Center, Hangzhou Normal University, Hangzhou, China
- 2Department of Biochemistry and Molecular Biology, School of Basic Medical Sciences, The Affiliated Hospital of Hangzhou Normal University, Hangzhou, China
Roseiflexus castenholzii is an ancient green non-sulfur bacteria that absorbs the solar energy through bacteriochlorophylls (BChls) bound in the only light harvesting (LH) complex, and transfers to the reaction center (RC), wherein primary charge separation occurs and transforms the energy into electrochemical potentials. In contrast to purple bacteria, R. castenholzii RC-LH (rcRC-LH) does not contain an H subunit. Instead, a tightly bound tetraheme cytochrome c subunit is exposed on the P-side of the RC, which contains three BChls, three bacteriopheophytins (BPheos), two menaquinones, and one iron for electron transfer. These novel structural features of the rcRC-LH are advantageous for enhancing the electron transfer efficiency and subsequent photo-oxidation of the c-type hemes. However, the photochemical properties of rcRC-LH and its applications in developing the photo-bioelectrochemical cells (PBECs) have not been characterized. Here, we prepared a PBEC using overlapped fluorine-doped tin oxide (FTO) glass and Pt-coated glass as electrodes, and rcRC-LH mixed with varying mediators as the electrolyte. Absence of the H subunit allows rcRC-LH to be selectively adhered onto the hydrophilic surface of the front electrode with its Q-side. Upon illumination, the photogenerated electrons directly enter the front electrode and transfer to the counter electrode, wherein the accepted electrons pass through the exposed c-type hemes to reduce the excited P+, generating a steady-state current of up to 320 nA/cm2 when using 1-Methoxy-5-methylphenazinium methyl sulfate (PMS) as mediator. This study demonstrated the novel photoelectric properties of rcRC-LH and its advantages in preparing effective PBECs, showcasing a potential of this complex in developing new type PBECs.
Introduction
Photosynthesis is the process that converts solar energy into electrochemical energy and supports almost all life on Earth. It originated from bacteria, and gradually appeared in algae and higher plants through genetic transfer. Diverse photosynthetic systems have evolved to achieve higher energy transformation efficiency. The membrane-bound protein-pigment complexes in these systems are capable of catalyzing the photochemical charge separation with a quantum efficiency of close to 100% (Blankenship et al., 2011; Singh et al., 2018), which has inspired the exploration and development of the third-generation solar cells (O’Regan and Grätzel, 1991; Wang et al., 2020, 2021). The third-generation solar cells prepared by plant and bacterial photosynthetic complexes are collectively known as photo-bioelectrochemical cells (PBECs) (Feng et al., 2016; Sekar et al., 2016; Musazade et al., 2018; Zhou et al., 2019; Lee et al., 2020).
In contrast to algae, cyanobacteria and higher plants that have evolved oxygenic photosynthesis, anoxygenic phototrophs are prokaryotes with primitive photosynthetic systems. In the model organism purple bacteria, light energy is absorbed by bacteriochlorophyll (BChl) B880 bound in the light harvesting complex 1 (LH1) either directly or from the peripheral antenna LH2 and transferred to the reaction center (RC), where charge separation occurs and transforms the energy into electrochemical potentials. The RC is composed of L, M subunits that each contains five transmembrane helices, and the H subunit comprising a cytoplasmic globular domain anchored to the membrane by an N-terminal transmembrane helix (Supplementary Figures 1A,B). The RC accommodates a special pair (P) of BChls, two accessary BChls and two bacteriopheophytins (BPheos), as well as an iron and two ubiquinone (UQ) molecules for electron transfer (Supplementary Figures 1C,D). Excitation of the special pair (a dimer of coupled BChls that are arranged in a slipped face-to-face π-π-stacking, which acts as primary donor in the initial steps of the charge separation) on the P-side of the RC initiates transfer of an electron along the A-branch BChl (BA), BPheo (HA), QA quinone, and iron to QB quinone. Two electrons transferred from the charge separation fully reduces the quinone to hydroquinone, which diffuses into the membrane pool and initiates cyclic electron transfer between the RC and cytochrome bc1 to build up a proton motive force for ATP synthesis. This highly stable and relatively simple RC-LH1 complex of purple bacteria has been extensively studied to develop new type of PBECs (Ravi and Tan, 2015).
To prepare an efficient PBEC, the photosynthetic complexes are required to constantly generate electrons and continuously inject these photogenerated electrons into electrodes (Paul et al., 2020; Buscemi et al., 2021). The efficiency of the photoelectric conversion is correlated with the transfer path of electrons inside the PBEC. In most PBECs prepared from purple bacteria RC-LH1, the RC is attached to the front electrode with its P-side, which enables the Q-side and cytoplasmic domain of the H subunit exposed in the mediator. The photogenerated electrons are transferred through the mediator to the counter electrode, wherein the accepted electrons pass through an external circuit to the front electrode to reduce the excited P+ and form a steady-state current (Supplementary Figure 1E). However, transfer of the photogenerated electrons with a mediator often decreases the electron transfer efficiency and results in loss of the photocurrent (Takshi et al., 2010). To address this problem, Masaharu et al. connected the H subunit of RC to the electrode using a connector N-(1-Pyrenyl) Iodoacetamide, which allowed the photogenerated electrons flow out of QB and be directly transferred into the electrode (Trammell et al., 2006). Unfortunately, presence of the relatively thick H subunit (24Å) and the connector (4Å) increased the actual distance between QB and the electrode to 28Å, which resulted in a relatively low electron transfer efficiency of this system.
Beatty et al. then shortened the distance between QB and the electrode by genetically truncating the H subunit of Rhodobacter sphaeroides. The shortest mutant (45M RC) contains 45 amino acids left at the N-terminal of H subunit, it retained the main function of charge separation (formation of P+ and QA–) but lacked a bound quinone at QB site. When the H-truncated RC (45M-M229QB–) was attached to a gold electrode via a Cys engineered near the QA site, the steady-state currents increased to 200 nA/cm2 when using 20 mM hydroquinone as the sole mediator (Jun et al., 2018). The photocurrents were increased and decreased in about 0.2 s, a rate that is one or two orders of magnitude faster than previously reported values obtained using soluble mediators (Trammell et al., 2006; Terasaki et al., 2009). However, truncation of the H subunit increased the conformational or electrostatic changes of the quinone binding pockets and decreased the binding affinity of QB (Sun et al., 2015), causing the photogenerated electrons transferred only from QA to the electrode (Supplementary Figure 1F; Jun et al., 2018). Notably, complete removal of the H subunit from an intact RC severely reduced the electron transfer from QA to QB by approximately 102 to 103-fold, the resultant LM RC retained 95 ± 3% electron transfer to the primary QA (Debus et al., 1985). Consistently, the electron transfer rate from HA– to QA was decreased by approximately 4-fold in the LM dimer (Sun et al., 2016). Therefore, a RC-LH that lacks the H subunit but maintains intact electron transfer capacity is required to enhance the electron transfer efficiency of the PBECs.
Roseiflexus castenholzii (R. castenholzii) is an ancient green non-sulfur bacteria that contains a unique photosynthetic system. In contrast to purple bacteria, R. castenholzii does not contain a peripheral LH2. It contains only one LH that encircles the L, M, and cyt c subunits composed RC to form an opened elliptical ring (Yamada et al., 2005; Majumder et al., 2016; Xin et al., 2018). Especially, R. castenholzii RC-LH (rcRC-LH) lacks the H subunit, it tightly associates a cytochrome (cyt) c subunit through a novel transmembrane helix (c-TM), with the tetraheme binding domain protruding into the periplasmic space, which can accept electrons transferred from the periplasmic electron donors. The c-TM further inserts into the gap of the LH ring, in together with a newly identified transmembrane helix X to form a quinol shuttling channel (Figure 1A and Supplementary Figure 2A). The RC contains three BChls and three BPheos (Collins et al., 2010), instead of four BChls and two BPheos in purple bacteria. Additionally, two menaquinone-11 (MQ) molecules have been identified within the RC, instead of the UQs bound in purple bacteria RC (Figures 1A,B and Supplementary Figures 1A–D). These novel structural features of rcRC-LH are beneficial for enhancing the electron transfer efficiency and subsequent photo-oxidation of the c-type hemes (Deisenhofer and Michel, 1989). Importantly, absence of the H subunit in rcRC-LH provides a prerequisite for direct transfer of the photogenerated electrons from the Q-side to the electrodes. However, the photochemical properties of rcRC-LH and its applications in developing PBECs have not been characterized.
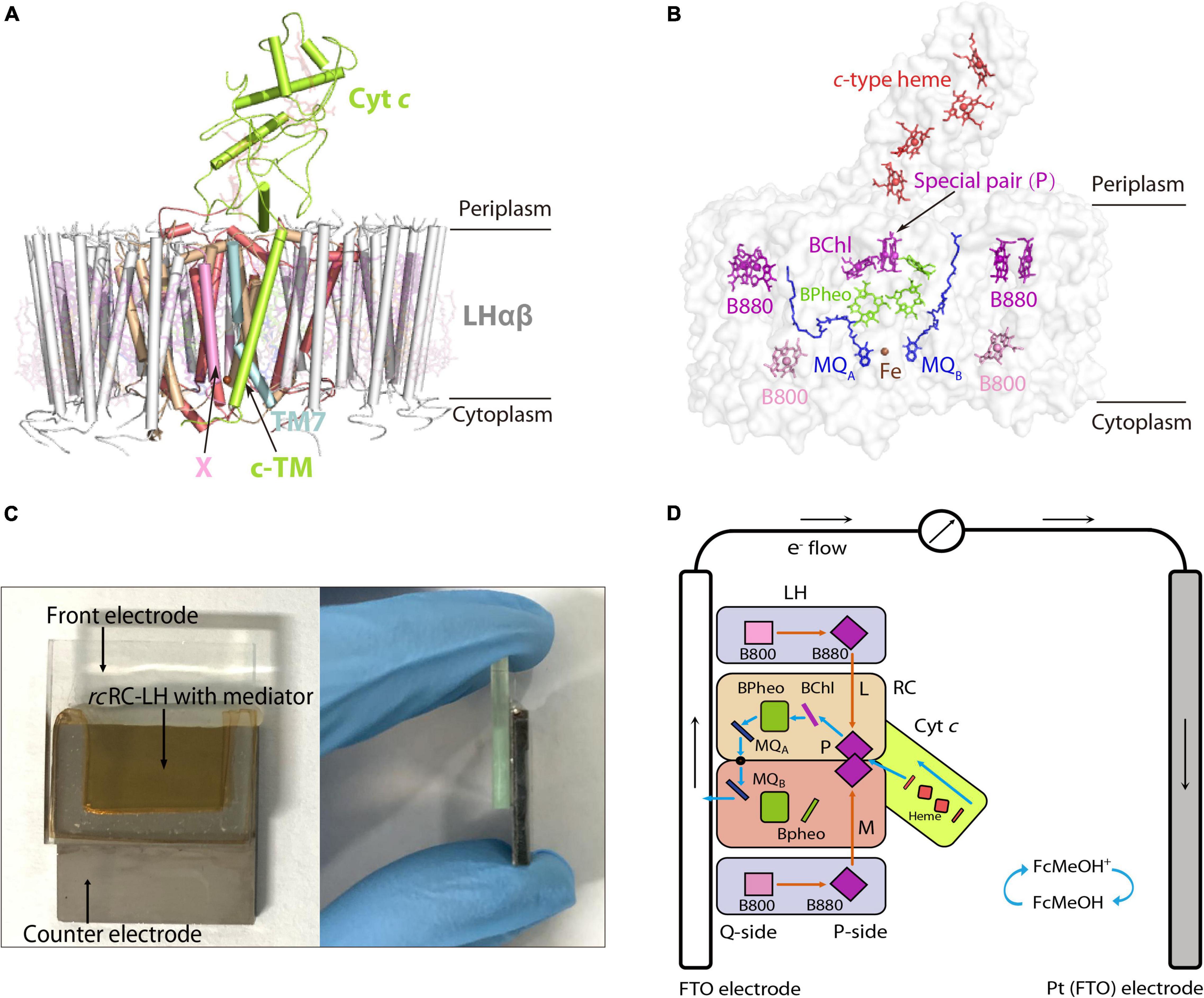
Figure 1. Preparation of an FTO-Pt PBEC using the RC-LH from R. castenholzii (rcRC-LH) rc. (A) Overall structure of the rcRC-LH (PDB ID: 5YQ7) is shown at the side view. The light harvesting (LH) complex (white) is composed of 15 LHαβ heterodimers in the form of an opened elliptical ring, which encircles the L (wheat), M (salmon), and cyt c subunit (limon) composed RC. The tetraheme (red sticks) binding domain of the cyt c subunit is exposed at the periplasmic side. Especially, a novel transmembrane helix of the cyt c (c-TM) inserts into the gap of the LH ring, and form a quinol shuttling channel in together with a newly identified X subunit (pink). An unassigned TM7 transmembrane helix (light cyan) is identified near the transmembrane helices of the L and M subunits in the RC. All the cofactors are shown as stick models with a transparency at 80%. (B) Spatial organization of the pigments and electron carriers in the rcRC-LH. Each LHαβ non-covalently binds two B880s (purple) at the periplasmic side, one B800 (pink) at the cytoplasmic side. The RC accommodates a special pair of BChls (P, purple), an accessary BChl (purple), and three BPheos (chartreuse), as well as an iron (brown sphere) and two menaquinone-11 (MQ, blue) molecules. The four c-type hemes bound in the cyt c subunit are exposed in the periplasmic side. All the cofactors except the iron are shown as stick models. (C) Construction of the rcRC-LH based FTO-Pt PBEC. The PBEC is composed of a FTO glass as the front electrode and a Pt-coated FTO glass as the counter electrode, a mixed solution of rcRC-LH with the mediator is injected into the cavity between the two electrodes as the electrolyte. A front (left) and side (right) view of the apparatus is shown. (D) Diagram of the operating mechanism of the rcRC-LH prepared FTO-Pt PBEC. The electron donor FcMeOH (hydroxymethylferrocene) was used as the mediator. Orange arrows indicate the route of light energy transfer, and blue arrows indicate the route of electron transfer. The P-side represents the direction of the rcRC-LH wherein the special pair (P) is located, and the direction where the MQA and MQB located is named the Q-side. The photogenerated electrons released from the excited P+ are transferred along the accessary BChl, BPheo to QA, an iron, then to QB. The electrons at the QB directly enter the front electrode (FTO) and are transferred to the counter electrode (Pt), wherein the accepted electrons pass through the c-type hemes via the mediator to reduce the excited P+ and form a steady-state current.
In this study, we made the first attempt to prepare a PBEC using the rcRC-LH complex (Figures 1C,D). The extracted rcRC-LH showed excellent photoreduction activity in catalyzing the reduction of the electron acceptor methylviologen (MV2+). The PBEC was prepared using an overlapped fluorine-doped tin oxide (FTO) glass as the front electrode, a Pt-coated glass as the counter electrode, and the purified rcRC-LH mixed with a mediator as the electrolyte. Photoelectric measurements characterized the immobilization of rcRC-LH on the electrode, the electron transfer path, and photocurrent intensities of the PBECs using different mediators. The results of this study revealed the photochemical properties and potentials of rcRC-LH in preparing an effective PBEC, and will contribute to the development of new type PBECs in the future.
Materials and Methods
Extraction and Purification of the rcRC-LH Complex
The rcRC-LH complex was extracted and purified from photoheterotrophically grown R. castenholzii as previous reported (Xin et al., 2018). The homogeneity and purity of the complex was monitored at each stage of the preparation by recording the absorption spectrum from 250–900 nm. The final 880 nm to 280 nm absorption ratio for the purified rcRC-LH complex was above 1.25.
Measurement of the Stability and Photoreduction Activity of the rcRC-LH Complex
To test the stability of the purified rcRC-LH complex, light energy was simulated with a 200 W incandescent lamp. The freshly prepared rcRC-LH complex was placed 40 cm away from the light source and exposed under light for 12 h at 25 °C. Then, the absorption spectrum over the range 700–1000 nm before and after the illumination was measured via UV-Vis spectrometry (Mapada P6, China). The photoreduction activity of the rcRC-LH was measured by mixing the rcRC-LH complex (20 μM) with 20 mM methylviologen (Aladdin Chemical Reagent Co., Ltd., United States), 0.2 mM polyvinyl pyrrolidone (Macklin Biochemical Co., Ltd., United States), 35 mM L-Lysine (Biosharp Life Sciences Co., Ltd., China), and 500 mM 2-Hydroxy-1-ethanethiol (Aladdin Chemical Reagent Co., Ltd., United States) to a final volume of 1 mL. Using deionized water as a negative control group, the absorbance of the mixed solution at 605 nm was recorded with and without light exposure. All the measurements were performed independently three times. The average and standard deviations of the values were calculated.
Preparation of an FTO-Pt PBEC Using the rcRC-LH Complex
We prepared the FTO-Pt PBECs according to previous literatures (Tan et al., 2012a,b). The FTO conducting glass (TEC 7 ohm/sq, 20 mm square × 2.2 mm thick; Shangzhuo Technology Co., Ltd., China) was dipped into acetone and isopropanol successively for 30 min and dried to serve as the front electrode. The counter electrode was comprised of a second piece of FTO conducting glass covered with a layer of 25 nm-thick Pt that was deposited by magnetron sputtering technology (MSP-300C, China). The conductive film of the two electrodes was checked with scanning electron microscope (Hitachi S-4800, Japan), and the water contact angles of the surfaces were measured using Drop Shape Analyser (Kruss DSA100, Germany). The two electrodes were then connected by a hot melt adhesive film (300 μm-thick; Xingxia Polymer Products Co., Ltd., China) to form a cavity of about 1 cm2 between the boundaries of the two electrodes. A 30 μL electrolyte solution containing 40 μM of the rcRC-LH complex and varying mediators (each at 250 μM concentration) such as hydroxymethylferrocene (FcMeOH), methylviologen, N, N, N′, N′-Tetramethyl-p-phenylene-diamine dihydrochloride (TMPD), and 1-Methoxy-5-methylphenazinium methyl sulfate (PMS) (Aladdin Chemical Reagent Co., Ltd., United States) was injected into the cavity. Finally, the opening was sealed by hot glue gun.
Characterizations of the Photoelectric Properties of the FTO-Pt PBEC
To measure the photostability of the prepared FTO-Pt PBEC, light energy was simulated with a 200 W incandescent lamp. The FTO-Pt PBEC was placed 40 cm away from the light source and exposed under light for 12 h at 25 °C. Then, the absorption spectrum of rcRC-LH in FTO-Pt PBEC over the range 700–1000 nm before and after the illumination was measured via UV-Vis spectrometry. The prepared FTO-Pt PBEC was exposed to a light source that was simulated using a 200 W incandescent lamp at a distance of 40 cm. The photocurrent intensities in the cell were measured by an electrochemical workstation (Lanlink LK2005A, China), with an applied voltage at 0 mV and the current intensity in the dark environment as the baseline. Since the active areas of the cells were slightly different, an opaque mask with a 0.1 cm2 hole was placed on the outer surface of front electrode before measurement, which ensured that the intensity of the incident light in each cell reached approximately 1.5 mW/cm2.
Results
The Cofactors Arrangement and Electron Transfer Routes in the rcRC-LH Complex
In our previously reported cryo-EM structure of rcRC-LH (Xin et al., 2018), the only LH is composed of 15 LHαβ heterodimers in the form of an opened elliptical ring. Each LHαβ non-covalently binds two B880s at the periplasmic side, one B800 at the cytoplasmic side, and one keto-γ-carotene spanning the interface between LHαβ for light harvesting and transfer (Figure 1A and Supplementary Figures 2A,B). The L and M subunits of RC accommodate a special BChl dimer P (B865), a BChl (B818), and three BPheos, as well as an iron and two MQ-11 molecules that are positioned within 14Å of each other (Figure 1B and Supplementary Figure 3A), a distance necessary for efficient electron orbital coupling and energy resonance (Osyczka et al., 2004). The distance between the special pair (P) and the nearest c-type heme is approximately 10.4Å (Supplementary Figure 3A), which is adequate for the subsequent reduction of the excited P+, through accepting the electrons transferred back from the four c-type hemes.
The light energy absorbed by the coupled pigments (B800, B880, and keto-γ-carotene) in LH are transferred to the special pair (P) of the RC. Once excited, the state P+ is stabilized by transferring an electron to the primary electron acceptor, BChl, within several picoseconds, and then passed through BPheo, MQA, and iron to MQB (P+QB–) (Supplementary Figure 3B). P+ can be reduced by electrons transferred back from the c-type hemes. Transfer of two electrons from the photochemical charge separation forms P+QA–QB–, which is fully reduced to hydroquinone (QH2) after accepting the second photogenerated electron and incorporating two protons from the cytoplasm (Supplementary Figure 3B). The tightly bound tetraheme cyt c subunit endows the R. castenholzii RC a much higher efficiency for the energy transfer and photo-oxidation of the c-type hemes, which is significantly different from the soluble and monoheme cyt c in purple bacteria.
The Stability and Photoreduction Activity of the rcRC-LH Complex
The rcRC-LH complex was extracted and purified from the heterotrophically grown R. castenholzii. Spectroscopic analyses revealed typical Qy bands of the LH-bound BChls at 800 nm (B800) and 880 nm (B880), and a Qx band of BChls at 594 nm. The RC-bound BPheos were determined at 760 nm, and the carotenoids showed absorption at 457, 482, and 519 nm. Near this region, an absorption peak corresponding to the oxidized cyt c was observed at 410 nm, as well as the split peaks of BChls at 374 nm (Supplementary Figure 4A). The absorption spectrum of rcRC-LH barely changed after exposure to simulated light energy for 12 h, indicating the excellent photo-thermal stability of the complex (Supplementary Figure 4B).
Methylviologen (MV2+) is an electron acceptor that can be easily reduced to MV+ upon accepting a single electron (Wang et al., 2021). Using 2-Hydroxy-1-ethanethiol as the electron donor and MV2 + as the electron acceptor, we measured the photochemical activity of rcRC-LH through recording the absorbance of MV+ at 605 nm (Figure 2A). In presence of the rcRC-LH, the absorbance of MV+ at 605 nm continually increased under illumination and kept constant when the illumination was turned off. Once re-exposed to the simulated light, the absorbance quickly increased, indicating that the rcRC-LH was excited by the light energy to initiate charge separation and subsequent electron transfer, releasing electrons to reduce MV2+ to MV+. Therefore, the extracted rcRC-LH possessed considerable photoreduction activity in vitro.
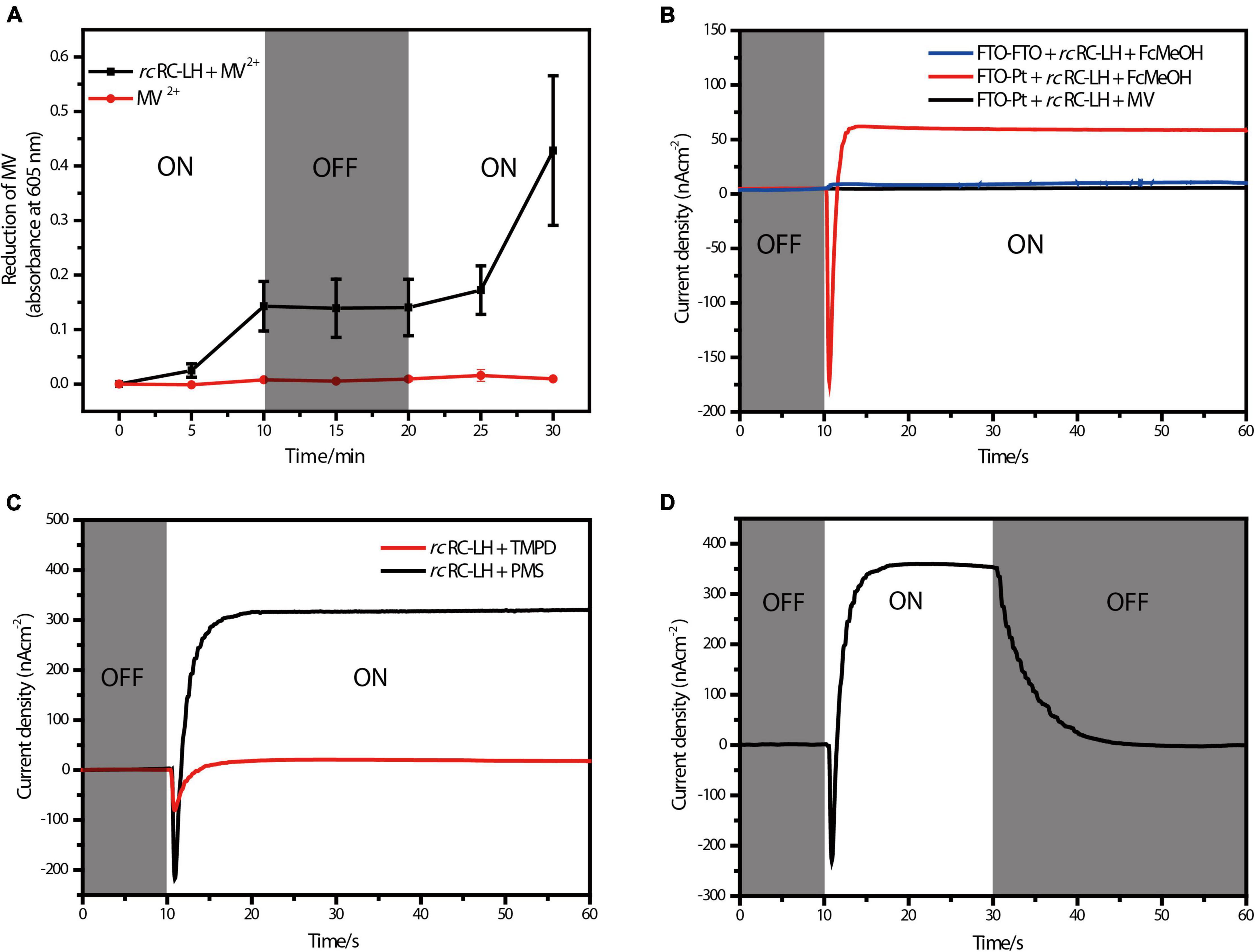
Figure 2. Photoelectric characterizations of the FTO-Pt PBEC. (A) Photoreduction activity of the rcRC-LH complex measured by using MV2+ as the electron acceptor, the absorbance of MV+ at 605 nm was recorded in the presence and absence of the rcRC-LH. The illumination period is indicated in the white sections of graph, and the dark period is indicated in gray. (B) Measurements of the photocurrent intensities of alternative PBECs. The photocurrent intensities were plotted over time with (white background) or without (gray background) illumination. One PBEC was prepared using FTO-glass as both electrodes (FTO-FTO), the other PBEC was prepared with only FTO-glass as the front electrode (FTO-Pt). FcMeOH (hydroxymethylferrocene) and MV (methylviologen) were used as the mediators in these PBECs. (C) Comparison of the photocurrent intensities of the FTO-Pt PBECs prepared using TMPD and PMS as the mediators. White graph background indicates a period of illumination, gray represents the period of darkness. (D) Changes of the photocurrent intensities of the PMS FTO-Pt PBEC upon alternating illumination conditions. White graph background indicates a period of illumination, gray represents the period of darkness.
Preparation of an FTO-Pt PBEC Using the rcRC-LH Complex
To prepare PBECs, the photosynthetic complex is often fixed to the working electrode by physical adsorption or chemical coupling after a long period of incubation with the electrode (Tan et al., 2012a). Before preparation, the working electrode usually needs to be pre-processed by high-temperature sensitization, chemical coupling, or modification of the surfaces (Lebedev et al., 2008; Terasaki et al., 2009). Here, a piece of FTO conducting glass deposited with a 350 nm-thick conductive film of fluorine-doped tin oxide was dipped into acetone and isopropanol successively for 30 min, then dried to serve as the front electrode. The surface of the FTO-glass is roughly textured (Supplementary Figure 5A), as a result of the low symmetric tetragonal crystal structures of fluorine-doped tin oxide (Zhang et al., 2018). This structure is similar to the wrinkles on the surface of the bacterial plasma membrane, which facilitates attachment of the photosynthetic complexes (Csiki et al., 2018).
The counter electrode is comprised of a second piece of FTO conducting glass covered with a layer of 25 nm-thick Pt, which can evenly cover the surface of the FTO-glass without affecting its electrical conductivity (Supplementary Figure 5B). Moreover, coating of the Pt layer also enabled the two electrodes to show distinct wettability (Supplementary Figures 5C,D). Notably, the surface of the front electrode is hydrophilic with a water contact angle of 45.1°, whereas the Pt surface of the counter electrode is more hydrophobic (with a contact angle of 91.6°), which would affect the attachment of the rcRC-LH onto the electrodes. An electrolyte containing 40 μM rcRC-LH and alternative mediator was injected into the cavity formed between the two electrodes to prepare the FTO-Pt PBEC (Figures 1C,D). The absorption spectrum of the rcRC-LH stored in the FTO-Pt PBEC were barely changed after exposure to the simulated light energy for 12 h (Supplementary Figure 4C), indicating a moderate photostability of the FTO-Pt PBEC.
By constructing a PBEC containing two electrodes with distinct wettability, the rcRC-LH was expected to selectively adhere to the hydrophilic surface of the front electrode (Tan et al., 2013). To test which electrode the rcRC-LH was attached to, we constructed two PBECs using the electron donor hydroxymethylferrocene (FcMeOH) as the mediator. One PBEC was constructed using FTO-glass for both electrodes (FTO-FTO), and the other was constructed with FTO-glass as the front electrode only (FTO-Pt). The current intensities of the two PBECs were measured via an electrochemical workstation under illumination. Without illumination, the current intensities of both FTO-FTO and FTO-Pt were zero. However, the photogenerated current of the FTO-Pt was much higher than that of FTO-FTO under illumination (Figure 2B), indicating the rcRC-LH was selectively adhered to the hydrophilic surface of the FTO glass, and that it was active in catalyzing the photochemical conversion reactions to generate electrons at the front electrode under illumination. In the FTO-FTO PBEC, the photogenerated electrons were probably being neutralized, due to the comparable binding capacity of the rcRC-LH complex onto the two electrodes with the same wettability. Therefore, we successfully immobilized the rcRC-LH complex onto the hydrophilic front electrode of the FTO-Pt PBEC through controlling the wettability of the surfaces instead of utilizing a complicated pre-processing procedure, thus eliminating a time- and resource-consuming step.
Photoelectric Characterizations of the FTO-Pt PBEC
When using electron donor FcMeOH as the mediator, a reverse current spike was generated once the PBEC was exposed to illumination (Figure 2B), indicating the photogenerated electrons were flowing out of the front electrode. The current spike was then deflected to form a steady-state current after about a 5 s illumination (Figure 2B), indicating the electrons were flowing into the front electrode from the rcRC-LH. The appearance of the reverse current spike was intriguing, as in the PBECs prepared from purple bacteria RC-LH1, the reduction rate of P+ is usually thousands of times higher than the oxidation rate of QB– (Agalidis and Velthuys, 1986; Tan et al., 2012a). During photochemical electron transfer of the rcRC-LH prepared FTO-Pt PBEC, the excited P+ is reduced by the electrons transferred from the four c-type hemes via the mediator FcMeOH (Figure 1D). As the rate of electrons flow more quickly into the P-side than out of the Q-side, the electron carriers in the RC serve as an electron sink. The over-oxidized FcMeOH extracts electrons from the front electrode, generating a reverse current spike. When the electron carriers in the RC are fully occupied and FcMeOH+ is spreading inside the PBEC to reach an equilibration, the photogenerated electrons continuously flow into the front electrode to form a steady-state current (Figure 1D).
To allow the photogenerated electrons directly flow into the electrode, the QB of the RC are required to face the electrode (Page et al., 1999; Winkler and Gray, 2014; Jun et al., 2018). Therefore, attachment orientation of the RC-LH on the electrode is essential for direct electron transfer into the front electrode. In the PBECs prepared by connecting the P-side of RC-LH1 to the front electrode (Supplementary Figure 1E), the mediator MV2+ accepted the photogenerated electrons from the Q-side, and transferred the electrons to the counter electrode to form a steady-state current (Kondo et al., 2007). To check the attachment direction of rcRC-LH on the electrodes, we replaced the electron donor FcMeOH with electron acceptor MV2+. As shown in Figure 2B, when using MV2+ as a mediator, the FTO-Pt PBEC did not generate a current under illumination, indicating that no photogenerated electrons were transferred to the counter electrode via MV2+. Since the tetraheme binding domain of the cyt c subunit is exposed on the P-side of the rcRC-LH, if it is attached to the front electrode with the P-side, QB– would reduce the electron acceptor MV2+ to ensure continuous photocurrent generation. Therefore, lost of the photocurrent indicated that the rcRC-LH complex was selectively attached to the front FTO electrode with its Q-side. In contrast to the previously reported PBECs, the photogenerated electrons in our FTO-Pt PBEC can directly enter the front electrode without assistance of a mediator, avoiding the current losses that result from mediated electron transport.
The mediator also plays a significant role in the electron transfer of the PBECs, as it continuously supplies electrons to the excited P+ through the c-type hemes, in order to ensure continuous photocurrent generations (Figure 1D). Both N, N, N′, N′-Tetramethyl-p-phenylene-diamine dihydrochloride (TMPD) and 1-Methoxy-5-methylphenazinium methyl sulfate (PMS) can serve as electron donors for the rcRC-LH. The vacuum potentials of TMPD and cyt c are −4.7 eV, whereas PMS, with a vacuum potential of −4.5 eV, is more favorable to provide electrons for the cyt c (Yaghoubi et al., 2014). As shown in Figure 2C, when TMPD and PMS were used as mediators, a steady-state current was generated in 10 s after illumination. The efficiency of the solar cell is strongly dependent on the short-circuit photocurrent density (JSC) and the associated open-circuit voltage (VOC). Specifically, the PMS PBEC could reach a steady-state JSC of 320 nA/cm2 and VOC of 50 mV, which is much higher than that generated by the TMPD PBEC. These results indicated that the photocurrent intensities of the PBEC can be increased by changing the vacuum potentials of the mediator. In addition, the photocurrent of PMS PBEC started to decrease and gradually reached 0 nA/cm2 when the light was turned off (Figure 2D). The gradual decrease of the photocurrent was a result of the flowing of the stored photogenerated electrons into the electron carriers of the RC, when the reduction rate of P+ is much higher than the oxidation rate of QB–.
Discussion and Future Perspective
In this study, we prepared a PBEC using the RC-LH complex from an ancient green non-sulfur bacteria R. castenholzii, which are phylogenetically distant from other anoxygenic photosynthetic bacteria. Different from the well-studied purple bacteria, R. castenholzii does not have a peripheral antenna LH2, instead it contains only one LH, which is composed of 15 αβ-polypeptides to form an opened elliptical ring. The open conformation of the LH ring structurally resembles that of the purple bacteria LH1, which also contain an LH1 ring with gap produced by PufX, protein-W, or special transmembrane helices (Qian et al., 2021a,b; Swainsbury et al., 2021). However, the c-TM and the newly identified X in rcRC-LH share no sequence identity and spatial organizations with any of these proteins. Compared to the monomeric RC-LH1 of Rba. Sphaeroides, both c-TM and X are located at different positions with distinct conformations (Figure 1A and Supplementary Figures 1A,B). Specifically, the c-TM and X are almost parallel with the LHαβ heterodimers, whereas the PufX is tilted with the LHαβs at approximately 60°, and the protein-U is formed by two transmembrane helices in a U-shape (Figure 1A and Supplementary Figures 1A,B; Qian et al., 2021b). Besides, incorporation of the B800s at the cytoplasmic side of rcLH combines the spectroscopic properties of purple bacteria LH1 and LH2, which are beneficial for enhancing the light harvesting efficiency of the rcRC-LH.
Compared to the LH that contains different compositional and structural features, the core components of the RC, including the L, M-subunits and cofactors are structurally conserved with that of purple bacteria (Supplementary Figures 1A–D, 2A,B). Notably, purple bacteria usually contain an H subunit that is important for regulating the assembly and electron transfer of the RC (Debus et al., 1985; Sun et al., 2015; Sun, 2017; Cao et al., 2022). However, presence of the H subunit often increased the distance between Q-side of the RC and the electrode, and decreased the electron transfer efficiency in PBECs. In contrast, absence of the H subunit allows the rcRC-LH to be selectively adhered to the hydrophilic surface of the front electrode with its Q-side, then the photogenerated electrons directly enter the electrode without assistance from any mediators (Figure 1D). Moreover, R. castenholzii RC contains a membrane-bound cyt c subunit that is tightly associated with the L and M subunits, with its tetraheme binding domain exposed at the P-side (Figure 1A and Supplementary Figure 2A). Exposure of the tetraheme binding domain endows rcRC-LH a higher photo-oxidation rate of the c-type hemes, which accepted the electrons transferred from the counter electrode and reduced the excited P+ to form a continuous photocurrent (Figure 1D). Therefore, the novel structural features of rcRC-LH provide a prerequisite for constructing effective PBECs.
The PBEC in this study was prepared using overlapped FTO glass and Pt-coated glass as electrodes, and the rcRC-LH mixed with a mediator as the electrolyte. As shown in Figure 2C, when using PMS as the mediator, the PBEC produced continuous photocurrent in 10 s after illumination (Figure 2C). A steady-state JSC of up to 320 nA/cm2 was generated with VOC at 50 mV and the incident light intensities at 1.5 mW/cm2. In the FTO-Pt PBEC prepared using Rba. sphaeroides RC-LH1 (Supplementary Figure 1E), generation of the steady-state current took about 20 s after illumination. However, the steady-state JSC of this PBEC could reach 900 nA/cm2 for the fresh PMS, with a VOC of 80 mV and the incident light intensities at 10 mW/cm2 (Tan et al., 2012b). In respect to the power conversion efficiency that is calculated from the product of the VOC and JSC divided by the incident light intensities, a higher power conversion efficiency of 1.07 × 10–3% was obtained for the rcRC-LH based PBEC than that prepared by Rba. sphaeroides RC-LH1 (7.2 × 10–4%). Therefore, although the rcRC-LH prepared FTO-Pt PBEC generated lower VOC and JSC, but it achieved a relatively faster and higher power conversion efficiency than the RC-LH1 based FTO-Pt PBEC.
Difference of the power conversion efficiency are mainly resulted from the distinct structural features and attachment orientations of the rcRC-LH and RC-LH1. It has been shown that removal of the H subunit from purple bacteria RC results in enhanced exposure of the semiquinone sites in the LM dimer (Sun, 2017), indicating the cytoplasmic domain of the H subunit shields the internal quinones of the RC-LH1. Therefore, presence of the H subunit hindered electron transfer from the Q-side of the RC to the electrode and decreased the electron transfer efficiency in the RC-LH1 prepared PBECs, no matter the RC-LH1 was attached to the electrode with its P-side or Q-side (Supplementary Figures 1E,F). Genetic truncation of the cytoplasmic domain of the H subunit indeed shortened the distance between QB and electrode to about 12Å, a steady-state JSC of up to 200 nA/cm2 was generated when using hydroquinone as the mediator (Jun et al., 2018). However, the photogenerated electrons were transferred only from QA to the electrode (Supplementary Figure 1F), since the QB binding affinity was dramatically decreased due to truncation of the H subunit (Sun et al., 2015). In contrast, the rcRC-LH is a natural protein complex bound with endogenous menaquinones, the conformations of the quinone binding pockets were not affected during preparation of the PBECs. As a result, the photogenerated electrons could transfer from the excited P+ to both the QA and QB in the PBECs. Therefore, absence of the H subunit in rcRC-LH is an advantageous structural feature that not only effectively exposes the Q-side of the RC for direct electron transfer to the electrode, but also enhances the power conversion efficiency of the PBECs.
However, the steady-state VOC and JSC of the rcRC-LH prepared FTO-Pt PBEC are relatively low, as compared with the dye-sensitized solar cells that commonly generate VOC at several hundreds of mV and JSC in mA/cm2 range. The redox potential difference between the electrolyte and the photo-oxidized BChls in the RC (P/P+) is a major determinant of the VOC. In Rba. Sphaeroides RC-LH1 prepared PBEC, the VOC scaled approximately linear with the measured potential of the electrolyte. On the other side, the JSC was also increased by the vacuum potentials of the mediator. The FTO-Pt PBECs containing fresh TMPD (−4.73 eV) typically produced steady VOC of approximately 7 mV and JSC of 150 nA/cm2, which are much lower than that generated by the fresh PMS (−4.51 eV) (Tan et al., 2012b). Consistently, both the VOC and JSC of the rcRC-LH prepared PBEC were remarkably increased when PMS was used as the mediator (Figure 2C). Therefore, it is possible to increase the VOC and JSC of the rcRC-LH based PBECs by simple manipulation of the electrolyte or alternating the potential of the coupled P/P+ through engineering of the RC. In addition, the photocurrent output can also be improved by optimizing the coverage and assemble form of the photoactive proteins on the electrode, exploiting the use of different electrode materials, fabrication procedures, and alternative incident light intensities of these PBECs. Besides, the electrolyte in our FTO-Pt PBEC could still have the loss of volatilization during long-time exposure to the light. Therefore, a more stable electrolyte with minimal volatilization rate also needs to be investigated in the future.
Conclusion
We prepared a novel FTO-Pt PBEC that converts the simulated light energy into steady-state current, using the natural rcRC-LH complex as the photovoltaic module. In contrast to the purple bacteria RC-LH1, the rcRC-LH does not contain the H subunit. Instead, a tightly bound tetraheme cyt c subunit is exposed on the P-side of the RC, which allows the rcRC-LH to selectively adhere onto the hydrophilic surface of the front electrode with its Q-side. As a result, the photogenerated electrons can directly enter the front electrode without assistance of a mediator, avoiding decrease of the electron transfer efficiency and loss of the photocurrent. Additionally, the photocurrent intensities of this FTO-Pt PBEC can be enhanced by changing vacuum potentials of the mediators. A steady-state current of up to 320 nA/cm2 was generated in the presence of PMS as the mediator. The results of this study provide a new perspective for preparing new type PBECs using the unique structural features of the rcRC-LH complex.
Data Availability Statement
The original contributions presented in this study are included in the article/Supplementary Material, further inquiries can be directed to the corresponding author.
Author Contributions
XX initiated the project and supervised all experiments. JD prepared the PBECs and characterized its photoelectrical properties. JX and ML purified the RC-LH complex from R. castenholzii. XZ, HH, and JW assisted in measurement of the photoelectric properties of the rcRC-LH based PBECs. XX and JD analyzed the data and wrote the manuscript. All authors contributed to the article and approved the submitted version.
Funding
This work was supported by grants from the National Natural Science Foundation of China (32171227, 31870740, and 31570738) and Zhejiang Provincial Natural Science Foundation of China under Grant No. LR22C020002 to XX.
Conflict of Interest
The authors declare that the research was conducted in the absence of any commercial or financial relationships that could be construed as a potential conflict of interest.
Publisher’s Note
All claims expressed in this article are solely those of the authors and do not necessarily represent those of their affiliated organizations, or those of the publisher, the editors and the reviewers. Any product that may be evaluated in this article, or claim that may be made by its manufacturer, is not guaranteed or endorsed by the publisher.
Acknowledgments
We thank Hualan Wang at the College of Material, Chemistry and Chemical Engineering for assistance during characterization of the photoelectric property of FTO-Pt PBEC.
Supplementary Material
The Supplementary Material for this article can be found online at: https://www.frontiersin.org/articles/10.3389/fmicb.2022.928046/full#supplementary-material
References
Agalidis, I., and Velthuys, B. R. (1986). Oxidation of QA- and of QB- of photosynthetic reaction centers by an artificial acceptor. FEBS Lett. 197, 263–266. doi: 10.1016/0014-5793(86)80339-8
Blankenship, R. E., Tiede, D. M., Barber, J., Brudvig, G. W., Fleming, G., Ghirardi, M., et al. (2011). Comparing photosynthetic and photovoltaic efficiencies and recognizing the potential for improvement. Science 332, 805–809. doi: 10.1126/science.1200165
Buscemi, G., Vona, D., Ragni, R., Comparelli, R., Trotta, M., Milano, F., et al. (2021). Polydopamine/ethylenediamine nanoparticles embedding a photosynthetic bacterial reaction center for efficient photocurrent generation. Adv. Sustain. Syst. 5:2000303. doi: 10.1002/adsu.202000303
Cao, P., Bracun, L., Yamagata, A., Christianson, B. M., Negami, T., Zou, B., et al. (2022). Structural basis for the assembly and quinone transport mechanisms of the dimeric photosynthetic RC-LH1 supercomplex. Nat. Commun. 13:1977. doi: 10.1038/s41467-022-29563-3
Collins, A. M., Qian, P., Tang, Q., Bocian, D. F., Hunter, C. N., and Blankenship, R. E. (2010). Light-harvesting antenna system from the phototrophic bacterium Roseiflexus castenholzii. Biochemistry 49, 7524–7531. doi: 10.1021/bi101036t
Csiki, R., Drieschner, S., Lyuleeva, A., Cattani-Scholz, A., Stutzmann, M., and Garrido, J. A. (2018). Photocurrent generation of biohybrid systems based on bacterial reaction centers and graphene electrodes. Diam. Relat. Mater. 89, 286–292. doi: 10.1016/j.diamond.2018.09.005
Debus, R. J., Feher, G., and Okamura, M. Y. (1985). LM complex of reaction centers from Rhodopseudomonas sphaeroides R-26: characterization and reconstitution with the H subunit. Biochemistry 24, 2488–2500. doi: 10.1021/bi00331a015
Deisenhofer, J., and Michel, H. (1989). The photosynthetic reaction center from the purple bacterium Rhodopseudomonas viridis. Science 245, 1463–1473. doi: 10.1126/science.245.4925.1463
Feng, H. J., Liang, Y. X., Guo, K., Li, N., Shen, D. S., Cong, Y. Q., et al. (2016). Hybridization of photoanode and bioanode to enhance the current production of bioelectrochemical systems. Water Res. 102, 428–435. doi: 10.1016/j.watres.2016.06.061
Jun, D., Dhupar, H. S., Mahmoudzadeh, A., Duong, F., Madden, J. D. W., and Beatty, J. T. (2018). In vivo assembly of a truncated H subunit mutant of the Rhodobacter sphaeroides photosynthetic reaction centre and direct electron transfer from the Q(A) quinone to an electrode. Photosynth. Res. 137, 227–239. doi: 10.1007/s11120-018-0493-0
Kondo, M., Nakamura, Y., Fujii, K., Nagata, M., Suemori, Y., Dewa, T., et al. (2007). Self-assembled monolayer of light-harvesting core complexes from photosynthetic bacteria on a gold electrode modified with alkanethiols. Biomacromolecules 8, 2457–2463. doi: 10.1021/bm070352z
Lebedev, N., Trammell, S. A., Tsoi, S., Spano, A., Kim, J. H., Xu, J., et al. (2008). Increasing efficiency of photoelectronic conversion by encapsulation of photosynthetic reaction center proteins in arrayed carbon nanotube electrode. Langmuir 24, 8871–8876. doi: 10.1021/la8011348
Lee, J., Cho, H., and Kim, S. (2020). Enhanced photocurrent generation from a single-mediated photo-bioelectrochemical cell using wild-type Anabaena Variabilis dispersed in solution. ChemElectroChem 7, 4075–4083. doi: 10.1002/celc.202001026
Majumder, E. L., Olsen, J. D., Qian, P., Collins, A. M., Hunter, C. N., and Blankenship, R. E. (2016). Supramolecular organization of photosynthetic complexes in membranes of Roseiflexus castenholzii. Photosynth. Res. 127, 117–130. doi: 10.1007/s11120-015-0179-9
Musazade, E., Voloshin, R., Brady, N., Mondal, J., Atashova, S., Zharmukhamedov, S. K., et al. (2018). Biohybrid solar cells: fundamentals, progress, and challenges. J. Photochem. Photobiol. C Photochem. Rev. 35, 134–156. doi: 10.1016/j.jphotochemrev.2018.04.001
O’Regan, B., and Grätzel, M. (1991). A low-cost, high-efficiency solar cell based on dye-sensitized colloidal TiO2 films. Nature 353, 737–740. doi: 10.1038/353737a0
Osyczka, A., Moser, C. C., Daldal, F., and Dutton, P. L. (2004). Reversible redox energy coupling in electron transfer chains. Nature 427, 607–612. doi: 10.1038/nature02242
Page, C. C., Moser, C. C., Chen, X., and Dutton, P. L. (1999). Natural engineering principles of electron tunnelling in biological oxidation–reduction. Nature 402, 47–52. doi: 10.1038/46972
Paul, N., Suresh, L., Vaghasiya, J. V., Yang, L., Zhang, Y., Nandakumar, D. K., et al. (2020). Self-powered all weather sensory systems powered by Rhodobacter sphaeroides protein solar cells. Biosens. Bioelectron. 165:112423. doi: 10.1016/j.bios.2020.112423
Qian, P., Croll, T. I., Hitchcock, A., Jackson, P. J., Salisbury, J. H., Castro-Hartmann, P., et al. (2021a). Cryo-EM structure of the dimeric Rhodobacter sphaeroides RC-LH1 core complex at 2.9 A: the structural basis for dimerisation. Biochem. J. 478, 3923–3937. doi: 10.1042/BCJ20210696
Qian, P., Swainsbury, D. J. K., Croll, T. I., Salisbury, J. H., Martin, E. C., Jackson, P. J., et al. (2021b). Cryo-EM structure of the monomeric Rhodobacter sphaeroides RC-LH1 core complex at 2.5 A. Biochem. J. 478, 3775–3790. doi: 10.1042/BCJ20210631
Ravi, S. K., and Tan, S. C. (2015). Progress and perspectives in exploiting photosynthetic biomolecules for solar energy harnessing. Energy Environ. Sci. 8, 2551–2573. doi: 10.1039/c5ee01361e
Sekar, N., Jain, R., Yan, Y., and Ramasamy, R. P. (2016). Enhanced photo-bioelectrochemical energy conversion by genetically engineered cyanobacteria. Biotechnol. Bioeng. 113, 675–679. doi: 10.1002/bit.25829
Singh, V. K., Ravi, S. K., Ho, J. W., Wong, J. K. C., Jones, M. R., and Tan, S. C. (2018). Biohybrid photoprotein-semiconductor cells with deep-lying redox shuttles achieve a 0.7 V Photovoltage. Adv. Funct. Mater. 28:1703689. doi: 10.1002/adfm.201703689
Sun, C. (2017). Removal of the H subunit results in enhanced exposure of the semiquinone sites in the LM dimer from Rhodobacter sphaeroides to oxidation by ferricyanide and by O2. Photosynth. Res. 133, 371–377. doi: 10.1007/s11120-017-0404-9
Sun, C., Carey, A. M., Gao, B. R., Wraight, C. A., Woodbury, N. W., and Lin, S. (2016). Ultrafast electron transfer kinetics in the LM dimer of bacterial photosynthetic reaction center from Rhodobacter sphaeroides. J. Phys. Chem. B 120, 5395–5404. doi: 10.1021/acs.jpcb.6b05082
Sun, C., Taguchi, A. T., Beal, N. J., O’malley, P. J., Dikanov, S. A., and Wraight, C. A. (2015). Regulation of the primary quinone binding conformation by the H subunit in reaction centers from Rhodobacter sphaeroides. J. Phys. Chem. Lett. 6, 4541–4546. doi: 10.1021/acs.jpclett.5b01851
Swainsbury, D. J. K., Qian, P., Jackson, P. J., Faries, K. M., Niedzwiedzki, D. M., Martin, E. C., et al. (2021). Structures of Rhodopseudomonas palustris RC-LH1 complexes with open or closed quinone channels. Sci. Adv. 7:eabe2631. doi: 10.1126/sciadv.abe2631
Takshi, A., Madden, J. D. W., Mahmoudzadeh, A., Saer, R., and Beatty, J. T. (2010). A photovoltaic device using an electrolyte containing photosynthetic reaction centers. Energies 3, 1721–1727. doi: 10.3390/en3111721
Tan, S. C., Crouch, L. I., Jones, M. R., and Welland, M. (2012a). Generation of alternating current in response to discontinuous illumination by photoelectrochemical cells based on photosynthetic proteins. Angew. Chem. Int. Ed. Engl. 51, 6667–6671. doi: 10.1002/anie.201200466
Tan, S. C., Crouch, L. I., Mahajan, S., Jones, M. R., and Welland, M. E. (2012b). Increasing the open-circuit voltage of photoprotein-based photoelectrochemical cells by manipulation of the vacuum potential of the electrolytes. ACS Nano 6, 9103–9109. doi: 10.1021/nn303333e
Tan, S. C., Yan, F., Crouch, L. I., Robertson, J., Jones, M. R., and Welland, M. E. (2013). Superhydrophobic carbon nanotube electrode produces a near-symmetrical alternating current from photosynthetic protein-based photoelectrochemical cells. Adv. Funct. Mater. 23, 5556–5563. doi: 10.1002/adfm.201301057
Terasaki, N., Yamamoto, N., Hiraga, T., Yamanoi, Y., Yonezawa, T., Nishihara, H., et al. (2009). Plugging a molecular wire into photosystem I: reconstitution of the photoelectric conversion system on a gold electrode. Angew. Chem. Int. Ed. Engl. 48, 1585–1587. doi: 10.1002/anie.200805748
Trammell, S. A., Spano, A., Price, R., and Lebedev, N. (2006). Effect of protein orientation on electron transfer between photosynthetic reaction centers and carbon electrodes. Biosens. Bioelectron. 21, 1023–1028. doi: 10.1016/j.bios.2005.03.015
Wang, X., Liu, C., Shi, Z., Pan, M., and Yu, D. (2020). Protein-encapsulated chlorophyll a molecules for biological solar cells. Mater. Des. 195:108983. doi: 10.1016/j.matdes.2020.108983
Wang, X., Pan, M., Shi, Z., Yu, D., and Huang, F. (2021). Protein nanobarrel for integrating chlorophyll a molecules and its photochemical performance. ACS Appl. Bio Mater. 4, 399–405. doi: 10.1021/acsabm.0c00208
Winkler, J. R., and Gray, H. B. (2014). Long-range electron tunneling. J. Am. Chem. Soc. 136, 2930–2939. doi: 10.1021/ja500215j
Xin, Y., Shi, Y., Niu, T., Wang, Q., Niu, W., Huang, X., et al. (2018). Cryo-EM structure of the RC-LH core complex from an early branching photosynthetic prokaryote. Nat. Commun. 9:1568. doi: 10.1038/s41467-018-03881-x
Yaghoubi, H., Li, Z., Jun, D., Lafalce, E., Jiang, X. M., Schlaf, R., et al. (2014). Hybrid Wiring of the Rhodobacter sphaeroides reaction center for applications in bio-photoelectrochemical solar cells. J. Phys. Chem. C 118, 23509–23518. doi: 10.1021/jp507065u
Yamada, M., Zhang, H., Hanada, S., Nagashima, K. V., Shimada, K., and Matsuura, K. (2005). Structural and spectroscopic properties of a reaction center complex from the chlorosome-lacking filamentous anoxygenic phototrophic bacterium Roseiflexus castenholzii. J. Bacteriol. 187, 1702–1709. doi: 10.1128/JB.187.5.1702-1709.2005
Zhang, J., Lou, Y., Liu, M., Zhou, H., Zhao, Y., Wang, Z., et al. (2018). High-performance dye-sensitized solar cells based on colloid–solution deposition planarized fluorine-doped tin oxide substrates. ACS Appl. Mater. Interfaces 10, 15697–15703. doi: 10.1021/acsami.8b01737
Keywords: photo-bioelectrochemical cell, reaction center, light harvesting complex, photosynthetic bacteria, electron transfer
Citation: Du J, Xin J, Liu M, Zhang X, He H, Wu J and Xu X (2022) Preparation of Photo-Bioelectrochemical Cells With the RC-LH Complex From Roseiflexus castenholzii. Front. Microbiol. 13:928046. doi: 10.3389/fmicb.2022.928046
Received: 25 April 2022; Accepted: 30 May 2022;
Published: 16 June 2022.
Edited by:
Weimin Ma, Shanghai Normal University, ChinaReviewed by:
Lijin Tian, Key Laboratory of Photobiology, Institute of Botany (CAS), ChinaWenda Wang, Institute of Botany (CAS), China
Qiang Wang, Henan University, China
Copyright © 2022 Du, Xin, Liu, Zhang, He, Wu and Xu. This is an open-access article distributed under the terms of the Creative Commons Attribution License (CC BY). The use, distribution or reproduction in other forums is permitted, provided the original author(s) and the copyright owner(s) are credited and that the original publication in this journal is cited, in accordance with accepted academic practice. No use, distribution or reproduction is permitted which does not comply with these terms.
*Correspondence: Xiaoling Xu, xuxl@hznu.edu.cn