- Produce Safety and Microbiology Research Unit, Western Regional Research Center, Agricultural Research Service, United States Department of Agriculture, Albany, CA, United States
Chlorine dioxide (ClO2) and sodium hypochlorite (NaClO) are two chlorinated oxidizing agents that are implemented in water treatment and postharvest processing of fresh produce. While the antibacterial mechanisms of NaClO have been investigated, there are comparatively few studies that have looked at how ClO2 kills bacteria. Therefore, the objective of this study was to compare the inactivation pathways of ClO2 and NaClO against Escherichia coli O157:H7. Treatments consisted of 2.5, 5, and 10 ppm ClO2 or 50, 100, and 200 ppm NaClO for 5, 10, and 15 min. Maximum log reductions of E. coli O157:H7 were 5.5 and 5.1 after treatment with ClO2 or NaClO, respectively. Bacterial inactivation was measured using log reductions, intracellular reactive oxygen species (ROS) using with 2′,7′–dichlorofluorescin diacetate (DCFDA) or aminophenyl fluorescein (APF) probes, relative values of NAD+, NADH, NADP+, and NADPH cofactors. Additionally, the expression of three key genes involved in ROS stress was measured via RT-PCR. Levels of intracellular ROS measured by DCFDA after ClO2 treatment were significantly higher than those found after treatment in NaClO. Additionally, NaClO treatment resulted in upregulation of ROS-defense genes, while expression of the same genes was typically at base levels or downregulated after ClO2 treatment. As the concentrations of both treatments increased, the NADP+:NADPH ratio shifted to the cofactor being predominantly present as NADP+. These data indicate that ClO2 and NaClO damage E. coli O157:H7 via measurably different mechanisms and that ClO2 does not appear to cause substantial oxidative stress to E. coli O157:H7 directly.
Introduction
Public health experts recognize agricultural and municipal water as a potential vector of enteric pathogens. Chlorinated sanitizers such as sodium hypochlorite (NaClO) and chlorine dioxide (ClO2) are commonly used as antimicrobials for wastewater sanitation, surface decontamination, and agricultural water treatment. Industries rely heavily on NaClO as their primary sanitizer because of its relatively low cost. While treatments with NaClO have been demonstrated on numerous occasions to reduce microbial populations, the overuse of NaClO has significant drawbacks, creating a need for suitable alternatives. From an efficacy standpoint, NaClO treatments deteriorate outside a narrow pH range and in environments with high organic loads, creating significant issues for the food industry (Goodburn and Wallace, 2013). Additionally, the formation of carcinogenic byproducts and the potential ability to increase the spread of antibiotic-resistance genes has led to occupational and consumer safety concerns (Deborde and von Gunten, 2008; Liu et al., 2018). One potential alternative to NaClO is ClO2 which has recently expanded to be utilized as a sanitizer in the food industry (Ofori et al., 2018). Numerous studies have demonstrated that the ClO2 disinfection properties are comparable or superior to that of NaClO, especially in situations of higher organic load (Gómez-López et al., 2009; Wu, 2016; Bridges and Wu, 2018; Praeger et al., 2018; Tadepalli et al., 2018; Bridges et al., 2020). Additionally, ClO2 has demonstrated more targeted damage to pathogens and subsequent inactivation than NaClO (Praeger et al., 2018). Despite these observations, the antimicrobial mechanisms of ClO2 compared to NaClO are poorly understood.
The mechanisms in which NaClO inactivates microorganisms are well known and have been described in great detail (Rodgers et al., 2004; Fukuzaki, 2006; Rajkovic et al., 2010; Gray et al., 2013). In aqueous solutions, NaClO dissociates into hypochlorous acid (HOCl) and hypochlorite (OCl–). Because HOCl is uncharged, it is membrane permeable, while the charged OCl– cannot permeate undamaged cell membranes (Fukuzaki, 2006). This makes the antibacterial properties of NaClO largely attributed to the concentration of HOCl in a solution. Once present, HOCl will rapidly react with proteins with sulfur-containing amino acids, such as cysteine or methionine, forming highly reactive intermediates, leading to protein inactivation or degradation (Gray et al., 2013). Additionally, HOCl can also react with nucleotides and lipids, albeit less preferentially than amino acids, leading to DNA and RNA strand breakage, lipid peroxidation, and other damages that can contribute to cell death (Niki, 2009; Stanley et al., 2010; Gray et al., 2013). Although HOCl can damage bacterial cells through numerous mechanisms, it is likely that lethality occurs via damage resulting in the inhibition of ion, metabolite, and protein transport across bacterial membranes (Albrich et al., 1986; McKenna and Davies, 1988; Gray et al., 2013).
Chlorine dioxide is another chlorinated sanitizer that is commonly used in water treatment (Gómez-López et al., 2009; Wu, 2016; Bridges and Wu, 2018). Like HOCl, ClO2 has demonstrated powerful antimicrobial properties, and numerous studies and reviews described its antibacterial efficacy (Rodgers et al., 2004; Gómez-López et al., 2009; Wu, 2016; Bridges and Wu, 2018; Praeger et al., 2018; Sun et al., 2019). However, there are few studies that have investigated the molecular inactivation pathways of ClO2 against pathogens. The putative mechanism of ClO2 inactivation involves a similar pathway to HOCl, reacting with sulfur-containing amino acids and proteins (Berg et al., 1986; Praeger et al., 2018) causing increased membrane permeability and subsequent cell death. However, multiple studies that have visually examined cells after ClO2 treatment found no obvious cellular deformities or cell wall damage (Ofori et al., 2017, 2018; Bridges et al., 2020). Exemplifying this, Bridges et al. (2020) demonstrated that treatment of Escherichia coli O157:H7 with concentrations of ClO2 ≤ 15 ppm did not significantly interfere with the maintenance of membrane polarity, indicating that damages from ClO2 were not localized at the membrane level (10). These results suggest that lethal damages caused by ClO2 are not superficially localized, and routes of ClO2-inactivation could be intracellular.
Despite both HOCl and ClO2 being chlorine-based oxidizers, it is plausible that the responses that bacteria employ when responding to each sanitizer are measurably different. By examining how both chlorinated sanitizers affect multiple physiological metrics, fundamental differences in antimicrobial mechanisms might become apparent. Therefore, the objective of this study was to evaluate E. coli O157:H7 after exposure to either NaClO or ClO2 to determine how ClO2 affects bacteria cells differently from NaClO. Specifically, levels of intracellular reactive oxygen species (ROS), expression of select genes, and the state of specific cofactors were measured to highlight important differences in how each sanitizer kills bacterial cells.
Materials and Methods
Preparation of Bacteria
Escherichia coli O157:H7 (ATCC® 35150) was maintained at −80°C throughout the study. Due to differences between strains, only one strain of E. coli was selected for use to reduce variance in collected data. Before experimentation, 10 ml of tryptic soy broth (TSB) was inoculated using a culture maintained in frozen conditions and incubated overnight at 37°C. This first culture was then used to inoculate a second 10 ml tube of TSB and was incubated overnight at 37°C. Following incubation, the culture was streaked onto slants of tryptic soy agar (TSA), incubated overnight at 37°C, and transferred to storage at 4°C to serve as working stocks. The day prior to experimentation, cultures maintained at 4°C were used to inoculate 10 ml tubes of TSB incubated for 16–18 h overnight at 37°C. Following incubation, the cultures were centrifuged for 10 min at 10,000 × g, and the resultant pellet was washed twice with peptone water (0.1%) and resuspended in 9 ml of peptone water.
Preparation of Aqueous Sanitizers
Stock solutions of NaClO and ClO2 were prepared as described previously (Tadepalli et al., 2018; Bridges et al., 2019). In short, working solutions of 500, 1,000, and 2,000 ppm NaClO were made by diluting bleach solution (6% NaClO) with sterile deionized (DI) water on the day of experimentation. A stock solution of ClO2 was generated using a dry media method provided by ICA TriNova, LLC (Forest Park, GA, United States). The generated ClO2 stock solution was diluted with DI water to generate working solutions of 25, 50, and 100 ppm ClO2 on the day of experimentation, and ClO2 was measured by the DPD (N, N-diethyl-r-phenylenediamine) method using a Hatch DR 900 colorimeter as utilized in previous studies (Wu and Kim, 2007; Tadepalli et al., 2018; Bridges et al., 2019).
Treatment of Escherichia coli O157:H7 With Sodium Hypochlorite or Chlorine Dioxide and Measurement of Viability
Due to the fact that NaClO and ClO2 are known to have different oxidative strengths and dissimilar antibacterial efficacies at comparable concentrations, treatment concentrations and times were selected based on commonly utilized conditions in published literature and preliminary trials aimed at achieving similar ranges of E. coli O157:H7 reduction (Tadepalli et al., 2018, 2019; Bridges et al., 2019). One milliliter of NaClO solution (500, 1,000, or 2,000 ppm) or ClO2 solution (25, 50, or 100 ppm) was added to 9 ml of ∼8 log CFU/ml E. coli O157:H7 culture to make final concentrations of 50, 100, or 200 ppm NaClO and 2.5, 5, or 10 ppm ClO2. Treatments lasted for 5, 10, or 15 min, and the bacterial cultures were vortexed every 5 min. At the end of the treatment, 1 ml of 1% sodium thiosulfate (Na2S2O3) was added to the culture, making a final solution of 0.09% to inactivate any remaining sanitizer as utilized previously (Bridges et al., 2020). A treatment with sterile DI water was included as a control in every experiment. The cultures were then serially diluted in peptone water and plated on MacConkey’s sorbitol agar supplemented with 0.05 mg/l cefixime and 2.5 mg/l potassium tellurite (CT-SMAC) and overlaid with TSA [Thin Agar Layer (TAL) method] to aid in the recovery of sub-lethally injured bacteria as described previously (Wu, 2008).
Measurement of Intracellular Reactive Oxygen Species With 2′,7′–Dichlorofluorescin Diacetate or Aminophenyl Fluorescein
Levels of intracellular ROS were measured using the DCFDA and APF probes from Life Technologies (Carlsbad, CA, United States). For the ROS assays, one ml of E. coli O157:H7 was incubated in 20 μM DCFDA for 1 h at 37°C in darkness or 10 μM APF at room temperature for 30 min in darkness as described previously (Cossu et al., 2017). After incubation, the bacterial cells were centrifuged for 3 min at 10,000 × g, washed twice, and resuspended in 900 μL of peptone water. One hundred microliters of ClO2 or NaClO were then added to the bacterial culture to start experiments. After the treatment time, 100 μl of Na2S2O3 was added to inactivate the remaining sanitizer. The treated cells were centrifuged for 3 min at 10,000 × g and resuspended in peptone water. Two hundred microliters of bacteria were then transferred to clear-bottomed, black-sided 96-well plates, and fluorescence intensity was measured at excitation/emissions of 495/527 nm or 490/515 nm for DCFDA and APF, respectfully, using a SpectraMax M2 Microplate Reader (Molecular Devices, San Jose, CA, United States).
Expression of Select Genes After Treatment With Sodium Hypochlorite or Chlorine Dioxide
Bacterial RNA was extracted after 15 min treatments and neutralization with Na2S2O3 using a Quick-RNA Fungal/Bacterial Kit (Zymo Research, Irvine, CA, United States) with an additional DNase treatment to remove genomic DNA. Expression of superoxide dismutase (sodA), hydrogen peroxide-inducible genes activator (oxyR), and redox-sensitive transcriptional activator (soxR) were selected as key genes to represent oxidative stress response due to their well-known involvement in ROS defense processes (24, 34, 35). Additionally, expression of universal stress protein A (uspA), RNA polymerase σ factor (rpoS), and outer membrane porin C (ompC) were selected to represent the response to general stress, and 16S rRNA served as the controller gene. Primer sequences were selected from previous studies (Mei et al., 2015; Yang et al., 2018) and are presented in Supplementary Table 1. RNA reverse-transcription and quantification were performed using the iTaq™ Universal SYBR® Green One-Step Kit (Bio-Rad, Hercules, CA, United States) in a CFX96 Real-Time PCR Detection System (Bio-Rad) as follows: reverse transcription for 10 min at 50°C, polymerase activation, and DNA denaturation for 1 min at 95°C, and 40 cycles of denaturation at 95°C for 10 s followed by annealing/extension for 30 s at 60°C. Fluorescence was measured after each cycle. After cycling, melt curve analysis was performed from 65 to 95°C with 0.5°C increments every 2 s.
Levels of NAD+, NADH, NADP+, and NADHP After Treatment With Sodium Hypochlorite or Chlorine Dioxide
After the 15-min treatments and neutralization with Na2S2O3, individual levels of NAD+, NADH, NADP+, and NADH were measured using the luciferase-based NAD/NADH-Glo™ and NADP/NADPH- Glo™ kits (Promega, Madison, WI, United States). Following manufacturer-provided protocols, 50 μl of cells in phosphate buffered saline (PBS) were lysed using 50 μl 0.2 N NaOH with 1% dodecyltrimethylammonium bromide (DTAB). For NAD+ or NADP+ analysis, 50 μl of lysed cells were combined with 25 μl of 0.4 N HCl and heated at 60°C for 15 min. After heating, the sample was incubated at room temperature for 10 min, and 25 μl of 0.5 M Trizma Base was added. For NADH or NADPH analysis, 50 μl of lysed cells were heated at 60°C for 15 min. After heating, the samples were incubated at room temperature for 10 min, and 50 μl of 0.2 N HCl/0.25 M Trizma Base was added. Fifty microliters of each sample were combined with 50 μl of NAD/NADH-Glo™ or NADP/NADPH-Glo™ detection reagent for NAD+/NADH or NADP+/NADPH, respectfully, in individual wells of 96-well white-walled tissue culture plate. The plate was placed in the dark for 30 min, and relative luminescence (RLU) was measured using a Synergy HTX plate reader (Biotek, Winooski, VT, United States).
Data Analysis
The relative expression of individual genes was analyzed using the 2–ΔΔCt method (Livak and Schmittgen, 2001). All experiments were performed in triplicate, and statistical analysis was performed using JMP (ver.12) or Sigmaplot (ver. 14) software with α = 0.05. Raw bacterial counts were log-transformed, and log reductions were determined by subtracting the populations of bacteria recovered after treatment from untreated controls. One-way ANOVAs coupled with Tukey’s HSD post-hoc tests were used to determine significant differences in viable cell counts, ROS levels, relative gene expression, and cofactor levels among the treatment conditions.
Results
Bacterial Viability and General Stress After Treatment
The average reductions of E. coli after treatments with ClO2 or NaClO are presented in Figure 1. For ClO2, maximum log reductions of 0.3, 1.4, and 5.5 were achieved after 15 min treatments with 2.5, 5, or 10 ppm treatments, respectively (Figure 1A). All NaClO treatments achieved their maximum reductions at different time points, with 50 ppm reaching 0.69 after 5 min, while 100 and 200 ppm treatments resulted in maximum log reductions of 3.3 and 5.1, respectively, after 10 min (Figure 1B).
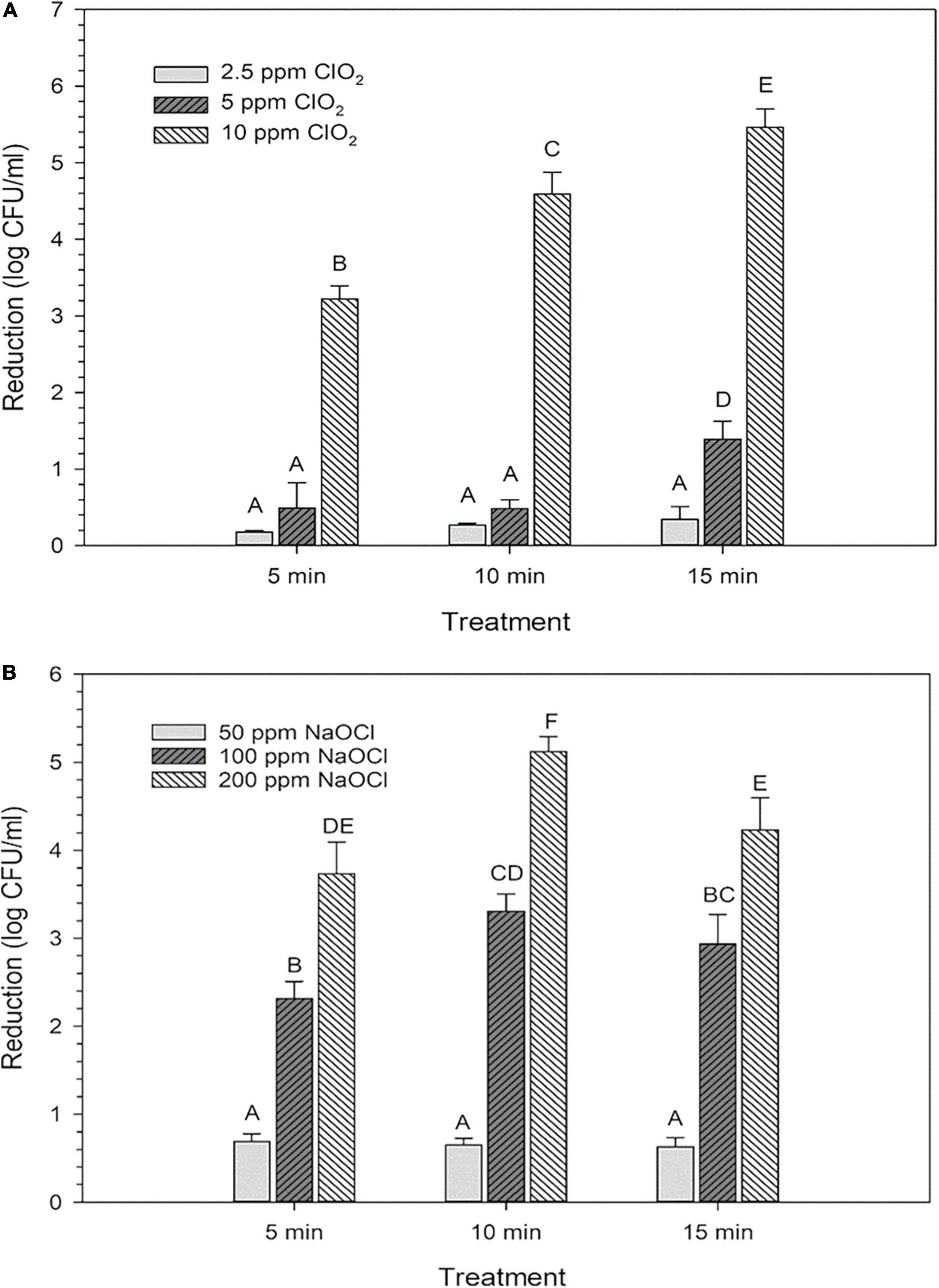
Figure 1. Log reductions of Escherichia coli O157:H7 after 2.5, 5, or 10 ppm ClO2 treatment for 5, 10, or 15 min compared (A) and treatment with 50, 100, or 200 ppm NaClO for 5, 10, or 15 min (B). Data are presented as means ± standard deviations, and significant differences (P ≤ 0.05) in bacteria reductions observed after each treatment are represented by different letters (e.g., A–F).
Monitoring gene expression to general stress as a control was performed by measuring levels of uspA mRNA. Expression of uspA increased accordingly with NaClO concentration, indicating a concentration-dependent stress response (Table 1). However, there was no change in uspA expression after ClO2 concentration increased. Levels of rpoS and ompC expression were additionally monitored to observe any potential changes in the regulation of stress responses or the presence of osmotic stress. Expression of rpoS decreased from 1.1 log to 0.3 log as NaClO concentration increased, while ClO2 treatment resulted in either base-level or downregulation of rpoS. The only examined gene that showed a similar trend after both treatments was ompC, indicating that both treatments resulted in osmotic stress.
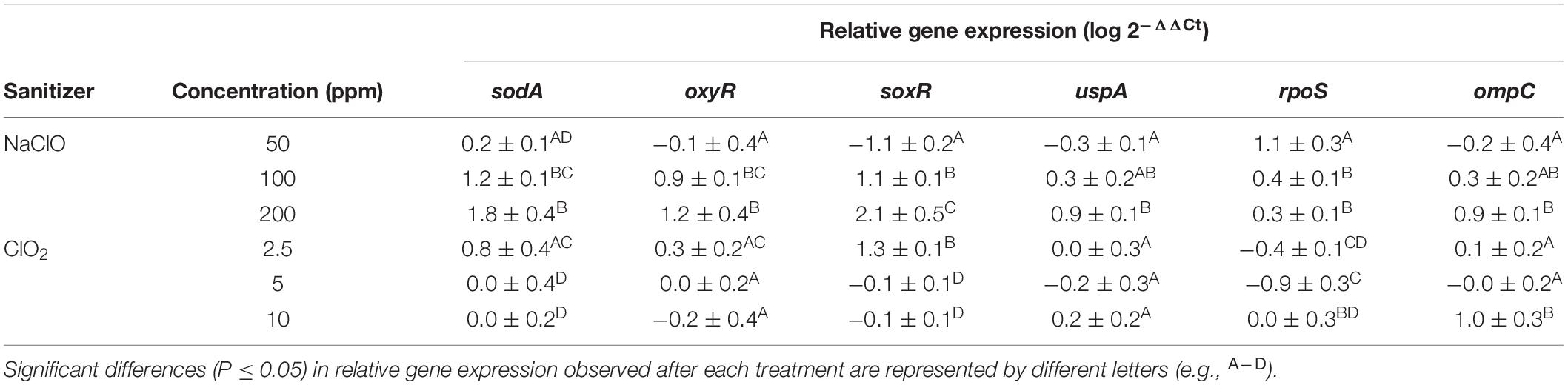
Table 1. Log relative expression of select genes after treatment of Escherichia coli O157:H7 with NaClO or ClO2.
Internal Reactive Oxygen Species Levels and Expression of Key Reactive Oxygen Species-Stress Genes After Treatment
Expression of select genes involved in ROS defense (sodA, oxyR, soxR) were all significantly (P > 0.05) upregulated after exposure to 100 and 200 ppm NaClO indicating that the cells were responding to the ROS stress at a gene expression level (Table 1). For ClO2 treatments, there were only 0.8, 0.3, and 1.3-fold increases for sodA, oxyR, and soxR expression, respectively, after treatment with 2.5 ppm. After treatment with 5 or 10 ppm ClO2, there were no significant changes in expression of the examined ROS-stress genes. Because there were significant log reductions after the 5 and 10 ppm treatment and little change in ROS-stress gene expression observed, these findings indicate that the cells are responding to NaClO and ClO2 treatments differently at the gene expression level.
Internal ROS measured by 2′,7′–dichlorofluorescin diacetate (DCFDA) after ClO2 significantly increased (P < 0.05) as the treatment concentration increased. However, increasing the exposure time did not influence internal ROS levels after ClO2 treatment (Figure 2A). Compared to ClO2 treatment, the ROS measured by DCFDA after NaClO treatment was relatively minimal (Figure 2B). The levels of ROS as measured by aminophenyl fluorescein (APF) did not significantly change with ClO2 treatment concentration (Figure 2C). In comparison, there was a significant increase in ROS levels measured by APF after the 200 ppm NaClO treatments (Figure 2D).
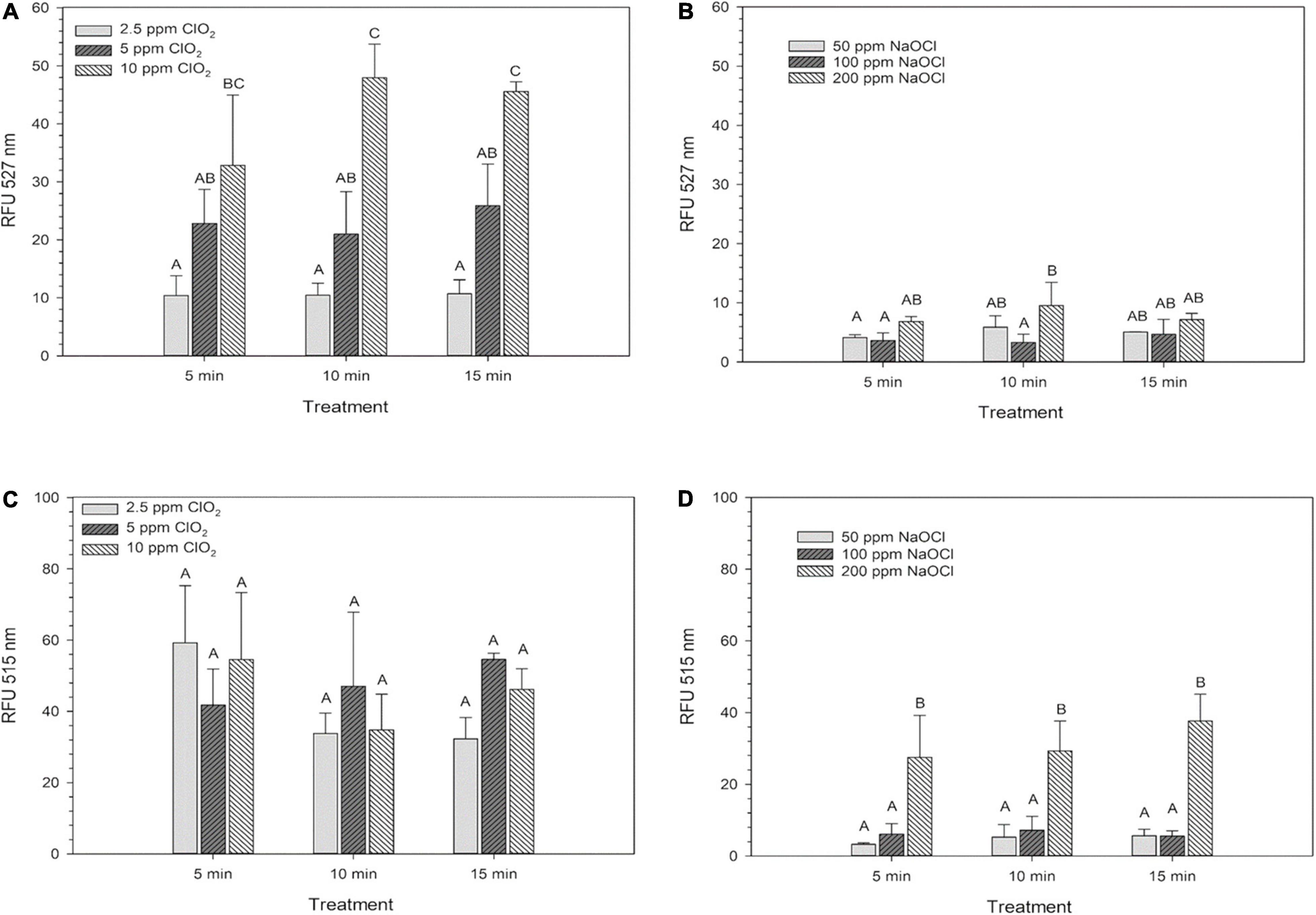
Figure 2. Intracellular ROS of Escherichia coli O157:H7 measured by DCF-DA after treatment with ClO2 (A) or NaClO (B) and the ROS levels as measured by APF after treatment with ClO2 (C) or NaClO (D). Data are presented as means ± standard deviations, and significant differences (P ≤ 0.05) in relative fluorescence units (RFUs) observed after each treatment are represented by different letters (e.g., A–C).
Levels of NAD+ and NADH After Treatment
The changes in base levels of NAD+ are presented in Figure 3A. The 50 ppm NaClO treatment resulted in an insignificant (P > 0.05) decrease in luminosity compared to distilled water, while both the 100 and 200 ppm concentrations had significant (P < 0.05) decreases. All three ClO2 treatments resulted in similar NAD+ levels. Also, there were significant decreases in NADH luminosity intensity as concentration increased for both NaClO and ClO2 (Figure 3B). Despite these changes, the overall ratio of NAD+:NADH remained relatively the same, with 99% ≥of the cofactor in the oxidized form (NAD+) and ≤1% in the reduced (NADH) state for all ClO2 or NaClO treatments (Table 2).
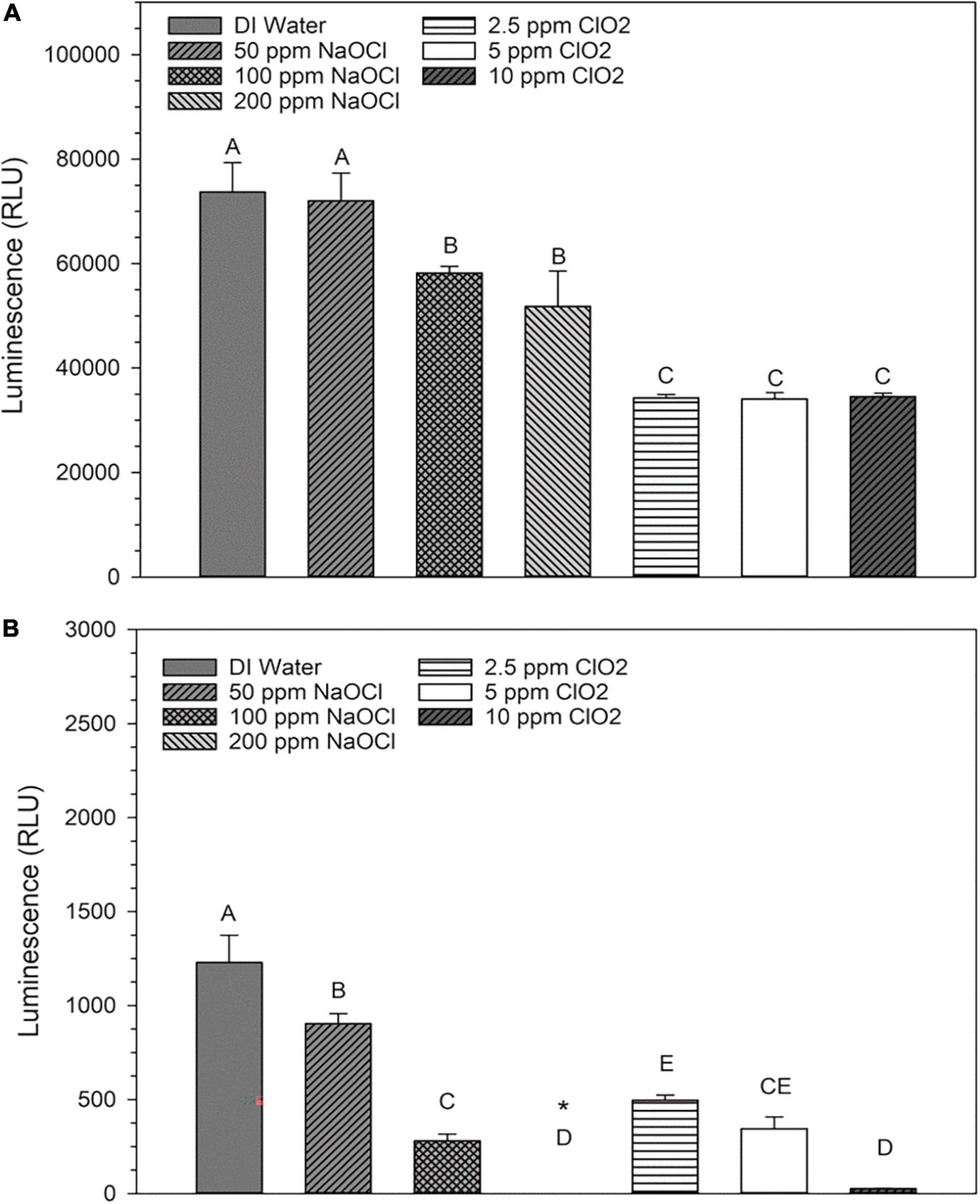
Figure 3. Relative levels of NAD+ (A) or NADH (B) present in Escherichia coli O157:H7 after treatment with NaClO or ClO2. Data are presented as means ± standard deviations, and significant differences (P ≤ 0.05) in relative luminosity units (RLUs) observed after each treatment are represented by different letters (e.g., A–E). The symbol “*” represents that the RLU was below the detection limit of the assay.
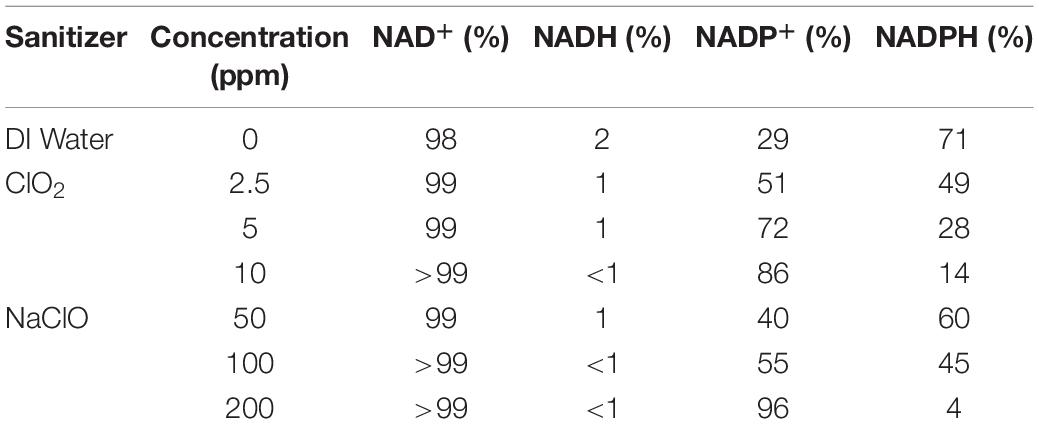
Table 2. The average percentage of reduced or oxidized forms of NAD+/NADH or NADP+/NADPH were measured after treatment with ClO2 or NaClO.
Levels of NADP+ and NADPH After Treatment
Changes in NADP+ levels after NaClO treatment are shown in Figure 4A. Compared to the distilled water control treatment, there was an increase in NADP+ luminosity after treatment with 50 or 100 ppm NaClO. Conversely, the 200 ppm treatment resulted in NADP+ luminosity similar to that of distilled water. All three ClO2 treatments resulted in an increased level of NADP+ compared to the control. Like the trend observed in NADH levels after treatment, NADPH levels significantly decreased as concentration increased for both treatments (Figure 4B). Additionally, as both ClO2 and NaClO increased in concentration, there was a shift in the NADP+:NADPH ratio favoring the reduced state (Table 2). After 2.5 ppm ClO2 treatment, the NADP+:NADPH ratio was 51:49% which shifted to 86%:14% after the 10 ppm treatment. Similarly, there was a shift from 40:60% to 96:4% as the NaClO treatment concentration increased.
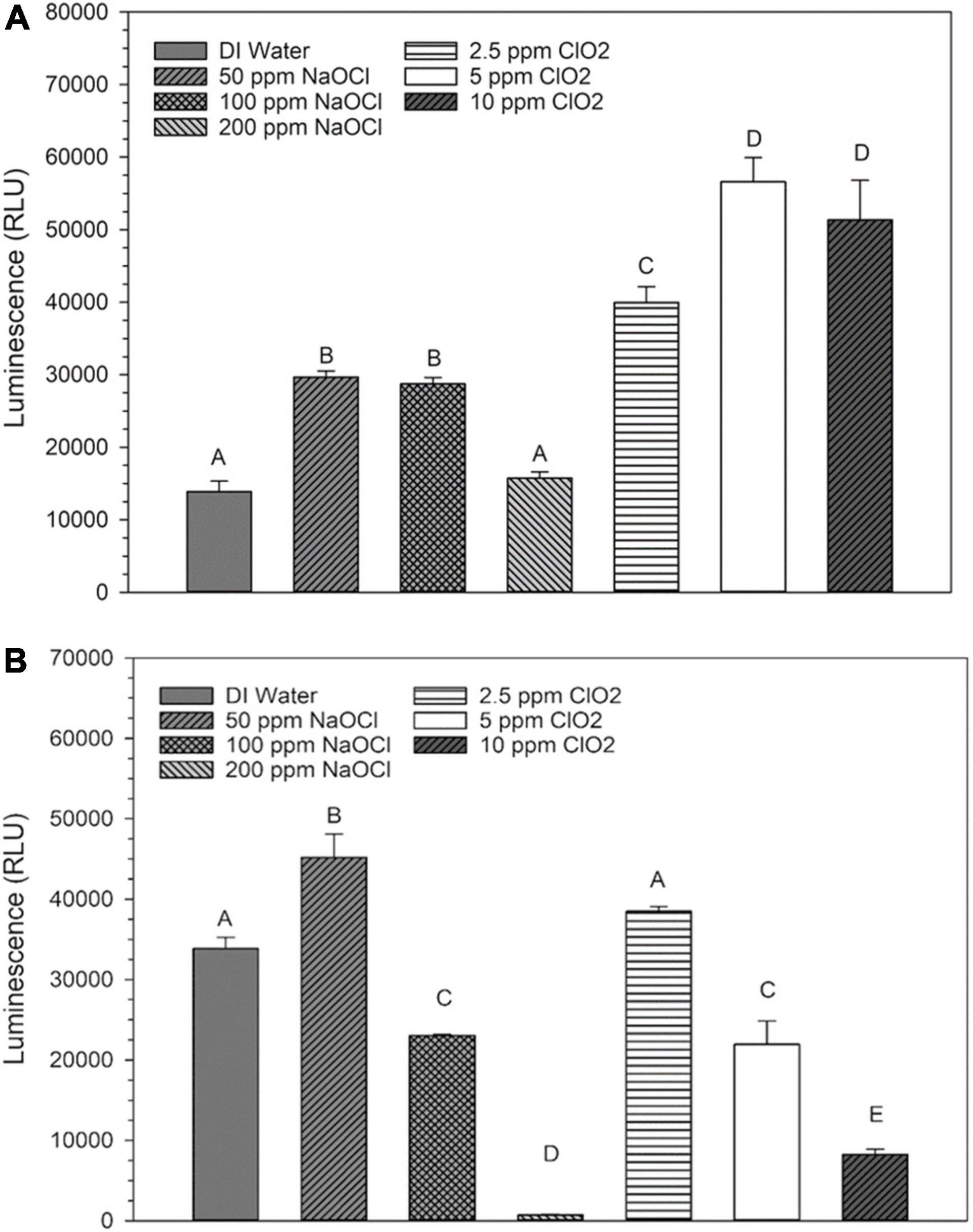
Figure 4. Relative levels of NADP+ (A) or NADPH (B) present in Escherichia coli O157:H7 after treatment with NaClO or ClO2. Data are presented as means ± standard deviations, and significant differences (P ≤ 0.05) in relative luminosity units (RLUs) observed after each treatment are represented by different letters (e.g., A–E).
Discussion
The present study demonstrates the distinctions between two commonly used chlorinated water treatment sanitizers. Management of ROS is vital to the survival of every cell, and measuring relative levels of intracellular ROS can provide insight into the physiological state of a cell. In this study, ClO2 treatments resulted in increased concentrations of measurable ROS without significantly increasing ROS-related gene expression. In comparison, exposure to E. coli O157:H7 with NaClO elicited a clear transcriptional response to oxidative stress, presumably resulting in reduced intercellular ROS levels detected. It is possible that the increased expression of ROS-defense genes ultimately allowed the cells to reduce the internal levels of ROS after NaClO treatment. In contrast, with ClO2, there was relatively little ROS-defense gene activity and a noticeable increase in ROS levels. Based on these results alone, it appears that ClO2 and NaClO caused cell death utilizing different mechanisms (Figure 5). Furthermore, the observation that ClO2 treatment resulted in the downregulation of key ROS-defense genes and increased the levels of intracellular ROS directly implies that the antibacterial potency of ClO2 does not appear to be simply due to direct oxidation by ClO2. While ClO2 is an oxidizing agent, the antibacterial effect of ClO2 is more complicated than widespread oxidation, as observed with NaClO.
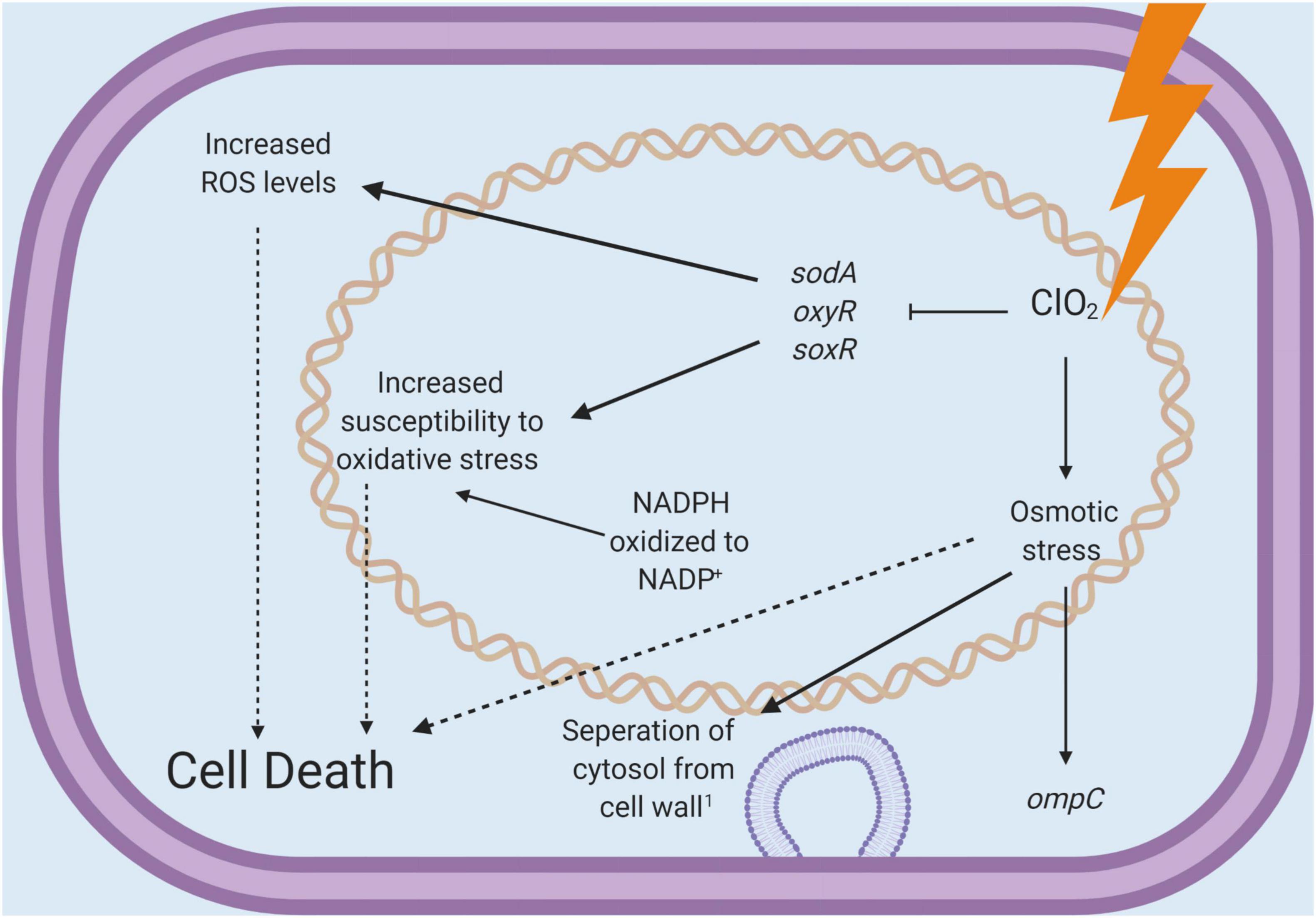
Figure 5. A schematic diagram for the antibacterial mechanisms of chlorine dioxide concluded from the present study.
The membrane-permeable DCFDA is a general oxidative stress indicator that can provide cursory information on bacterial ROS responses. Upon entry to cells, it is cleaved by cellular esterases at ester bonds which produces a polar, membrane-impermeable product that, upon oxidation, becomes fluorescent. While DCFDA is most sensitive to H2O2, it can also react with other oxidants (Eruslanov and Kusmartsev, 2010). The other ROS probe used, APF, is non-fluorescent until it reacts with hydroxyl radicals (.OH), peroxynitrite (ONOO–), or hypochlorite (ClO–). The relative increase in probe signal observed after ClO2 treatment indicates an intracellular ROS response, but it is unknown whether this increase was due to the direct generation of ROS by the treatments or through interference with typical ROS-defense systems. The difference in results between the two probes could be due to the generation of different species of ROS during or after treatment, differences in membrane permeability of the probes, and limitations of the sensitivity of the probes in general. Previously, Cossu et al. (2017) found a non-linear relationship between DCFDA fluorescence and E. coli O157:H7 viability after treatment with H2O2 and no relationship between APF fluorescence and viability. Like the APF results in the present study, they also did not find significant increases in fluorescence corresponding to increases in sanitizer concentration; the difference in ROS levels after treatment with each sanitizer could imply that the increase in ROS levels after ClO2 treatment could be due to damage to ROS management and defense processes. These findings help contextualize how ClO2 treatment can result in higher levels of microbial inactivation in water which has major public health implications.
All aerobic microorganisms manage ROS that accumulates in cells as products of the incomplete reduction of molecular oxygen. In this study, the expression of manganese superoxide dismutase (sodA) was used as an indicator of oxidative stress in E. coli O157:H7. The expression of sodA, and the other two ROS-defense-related genes monitored in this study, soxR, and oxyR, can be influenced by other external environmental factors, which result in predictable cellular responses to oxidative stress. Expression of sodA itself is regulated by SoxR regulon. Upon activation by superoxide, SoxR activates the transcription of soxS, which, in turn, regulates critical genes responsible for managing oxidative stress, which includes sodA. After treatment with NaClO, a corresponding upregulation of sodA expression increased as treatment concentration increased, indicating that NaClO treatment caused dose-dependent superoxide stress in E. coli O157:H7. However, there was no significant increase in the expression of these genes after treatment with ClO2. Previously, Mei et al. (2017) observed that treatment of E. coli O157:H7 on lettuce with 50 mM H2O2 resulted in a decrease in the expression of sodA, soxR, and oxyR. Additionally, the expression of soxR was used to indicate the incidence of oxidative stress in the present study. After treatment with 2.5 ppm ClO2, there was a noticeable increase in soxR expression and a corresponding slight increase in sodA expression. This indicates the formation of superoxide because of treatment. However, as treatment concentration increased, the levels of expression of these two genes remained at baseline, which is counter to the observation of oxygen radical activity observed with DCFDA.
The third ROS-defense gene monitored in this study, oxyR, is transcribed after oxidation of the OxyR regulon. In the presence of H2O2, OxyR is oxidized and then activates transcription of the OxyR regulon genes, which function to protect the cell against H2O2 (Gray et al., 2013). Conversely, the expression of oxyR did not change after ClO2 exposure either, which could indicate that there was no successful activation of the OxyR regulon. The fact that there was a significant increase in intracellular ROS after ClO2 treatment, but little ROS-defense gene induction, potentially represents ClO2-induced disruption of the normal signaling pathways which could result in the upregulation of these genes. Therefore, cells treated with ClO2 could potentially be more susceptible to oxidative damage and ROS accumulation. These findings further support that ClO2 causes different cellular responses from what is observed with treatment with a typical oxidizer like NaClO.
To serve as controls for other stresses, the expression of three other stress-related genes (uspA, rpoS, and ompC) was measured as well. Like the results observed with the ROS-defense genes, expression of uspA increased with NaClO concentration while ClO2 treatment resulted in little change. While the exact functions of UspA proteins are unclear, increased expression of uspA has been observed after numerous types of stresses and has been associated with improved survival rates during stress in E. coli (Vollmer and Bark, 2018). Likewise, rpoS is associated with multiple types of stress resistances, and rpoS mutants have been previously described as being sensitive to treatment with oxidizers (Battesti et al., 2011). In the present study, the relative levels of rpoS expression inversely to NaClO concentration. Mei et al. (2017) previously also reported downregulation of rpoS after treatment of Escherichia coli O157:H7 with 50 mM H2O2. However, it has been demonstrated that OxyS, a product of OxyR activation, negatively regulates rpoS translation (Zhang, 1998), indicating that the downregulation of rpoS observed in the present was a result of increased oxyR expression. The only investigated gene that demonstrated similar trends in expression after treatment with either NaClO or ClO2 was ompC, indicating that E. coli O157:H7 was undergoing osmotic stress during both types of treatments. Previously, Bridges et al. (2020) found that E. coli O157:H7 cells treated with 10 ppm ClO2 had visible sections where the cytoplasm was separated from the cell wall, further supporting that during high concentration ClO2 treatment, and NaClO as well, osmotic stress could contribute to bacterial mortality.
NADPH is essential for the function of catalases, superoxide dismutases, and glutathione peroxidases (Singh et al., 2008). The redox potential of NADP+/NADPH is almost identical to NAD+/NADH, but the key difference between them is that NADP+/NADPH is primarily used in anabolic redox reactions while NAD+/NADH is used in oxidation reactions (Spaans et al., 2015). In this study, the cofactor measurements after treatment demonstrate a shift in the overall ratio of NADP+:NADPH as the concentration of both antimicrobials increased. The average percentages of NADP+:NADPH after 50 ppm and 200 ppm NaClO treatments were 49 and 14%, while for 2.5 and 10 ppm ClO2, they were 60 and 4%. One possibility is that NADPH was oxidized directly during NaClO or the ClO2 exposure due to the abundance of NADP+ increasing with treatment concentration. However, given the differences in gene expression mentioned earlier, it is impossible to determine if the increased NADP+ levels are due to similar metabolic processes after each treatment. Regardless, these results do indicate the importance of NADPH in managing oxidative stress and are in agreement with other research on the topic. Christodoulou et al. (2018) previously demonstrated the rapid increase of NADPH during oxidative stress in E. coli and further explained the importance of NADPH regeneration via the pentose phosphate pathway. Additionally, Bitew et al. (2020) demonstrated that mutant Coxiella burnetii with diminished functionality of SdrA, an NADP regenerating enzyme, were more sensitive to oxidative stress in vitro.
Increased susceptibility to oxidative stress provides opportunities for significant damage to the lipid bilayer and other regions prone to oxidative damage. Similar studies to the present one have indicated that lipid peroxidation plays a key role in disrupting the membrane fluidity of the bacterial cells wall of gram-positive microorganisms, such as Enterococcus faecalis (Ersoy et al., 2019) as well as the gram-negative E. coli (Bridges et al., 2020). The subsequent byproducts of lipid peroxidation, such as aldehydes, can penetrate the cytoplasm and target many proteins and nucleic acids within the cell. These byproducts may also uncouple electron transport across a cellular membrane and decrease cellular ATP. In addition, it is important to consider the sublethal mechanism of the injury, which would affect genomic expression. Although the TAL recovery method was implemented to increase bacterial recovery, it is impossible to rule out that there were viable but non-culturable (VNBC) cells present after treatment. The presence of VNBC could be contributing to the variability between different time points observed in the plate-count data. Furthermore, evidence of resistance to ClO2 treatment in E. coli O157:H7 has been previously demonstrated, indicating that injured and/or stressed cells can effectively respond to treatment (Shu et al., 2020). It is also important to note that VNBC cells would also influence the values of the data discussed below and be valuably contributing to these physiological metrics. Future studies will investigate the sublethal mechanism of bacterial recovery, especially in the presence of important antioxidants such as glutathione.
Sodium hypochlorite is an inexpensive sanitizer currently used in mass quantities for agriculture and municipal water treatment. Comparatively, the cost of generation, storage, and monitoring of alternative sanitizers, such as ClO2, are currently more expensive. However, the cost per liter or square meter is not the only area growers need to consider when applying pathogen mitigation strategies. There are several drawbacks to the ubiquitous use of NaClO, such as reduced antimicrobial efficacy and the formation of toxic byproducts. Therefore, the USDA National Organic Standards has formally recommended that the application dosage for hypochlorites to agricultural water not exceed the 4 ppm residue limit imposed by the Safe Drinking Water Act (Tiemann, 2014). In addition, NaClO has limited efficacy outside of specific pH and organic load. Furthermore, the reduced antimicrobial efficacy can lead to subpopulations of pathogenic microorganisms persisting in the environment and antibiotic resistance. Therefore, alternative sanitizers such as ClO2 can be a tool to consider for agricultural water safety because of the antimicrobial range at a significantly lower residual level.
Data Availability Statement
The original contributions presented in this study are included in the article/Supplementary Material, further inquiries can be directed to the corresponding author.
Author Contributions
DB performed experiments and wrote the draft of the manuscript. AL edited the drafts of the manuscript. VW conceived and supervised the study, designed experiments, and reviewed and edited drafts of the manuscript. All authors read and approved the final draft of the manuscript.
Funding
This study was funded by the United States Department of Agriculture National Institute of Food and Agriculture (USDA NIFA) award numbers 2015-69003-32075 and 2021-51181-35905. Chlorine dioxide precursor materials were generously provided by Joel Tenney (ICA TriNova).
Conflict of Interest
The authors declare that the research was conducted in the absence of any commercial or financial relationships that could be construed as a potential conflict of interest.
Publisher’s Note
All claims expressed in this article are solely those of the authors and do not necessarily represent those of their affiliated organizations, or those of the publisher, the editors and the reviewers. Any product that may be evaluated in this article, or claim that may be made by its manufacturer, is not guaranteed or endorsed by the publisher.
Supplementary Material
The Supplementary Material for this article can be found online at: https://www.frontiersin.org/articles/10.3389/fmicb.2022.923964/full#supplementary-material
References
Albrich, J. M., Gilbaugh, J. H., Callahan, K. B., and Hurst, J. K. (1986). Effects of the putative neutrophil-generated toxin, hypochlorous acid, on membrane permeability and transport systems of Escherichia coli. J. Clin. Invest. 78, 177–184. doi: 10.1172/JCI112548
Battesti, A., Majdalani, N., and Gottesman, S. (2011). The RpoS-Mediated General Stress Response in Escherichia coli. Annu. Rev. Microbiol 65, 189–213. doi: 10.1146/annurev-micro-090110-102946
Berg, J. D., Roberts, P. V., and Matin, A. (1986). Effect of chlorine dioxide on selected membrane functions of Escherichia coli. J. Appl. Bacteriol. 60, 213–220. doi: 10.1111/j.1365-2672.1986.tb01075.x
Bitew, M. A., Hofmann, J., De Souza, D. P., Wawegama, N. K., Newton, H. J., and Sansom, F. M. (2020). SdrA, an NADP (H)-regenerating enzyme, is crucial for Coxiella burnetii to resist oxidative stress and replicate intracellularly. Cell. Microbiol. 22. 5, e13154. doi: 10.1111/cmi.13154
Bridges, D. F., Lacombe, A., and Wu, V. C. H. (2020). Integrity of the Escherichia coli O157:H7 Cell Wall and Membranes after Chlorine Dioxide Treatment. Front. Microbiol. 11:888. doi: 10.3389/FMICB.2020.00888
Bridges, D. F., Tadepalli, S., Anderson, R., Zhang, R., and Wu, V. C. H. (2019). Reduction of Listeria monocytogenes and Salmonella Typhimurium on Blueberries through Brief Exposure to Antimicrobial Solutions Coupled with Freezing. J. Food Prot 82, 926–930. doi: 10.4315/0362-028X.JFP-18-433
Bridges, D. F., and Wu, V. C. H. (2018). Gaseous Chlorine Dio2018, Pages 243-252xide for Postharvest Treatment of Produce. Postharvest Disinfection of Fruits and Vegetables. 2018, 243–252. doi: 10.1016/b978-0-12-812698-1.00013-3
Christodoulou, D., Link, H., Fuhrer, T., Kochanowski, K., Gerosa, L., and Sauer, U. (2018). Reserve flux capacity in the pentose phosphate pathway enables Escherichia coli’s rapid response to oxidative stress. Cell Syst. 6, 569–578. doi: 10.1016/j.cels.2018.04.009
Cossu, A., Le, P., Young, G. M., and Nitin, N. (2017). Assessment of sanitation efficacy against Escherichia coli O157:H7 by rapid measurement of intracellular oxidative stress, membrane damage or glucose active uptake. Food Control 71, 293–300. doi: 10.1016/J.FOODCONT.2016.07.009
Deborde, M., and von Gunten, U. (2008). Reactions of chlorine with inorganic and organic compounds during water treatment-Kinetics and mechanisms: A critical review. Water Res. 42, 13–51. doi: 10.1016/j.watres.2007.07.025
Ersoy, Z. G., Dinc, O., Cinar, B., Gedik, S. T., and Dimoglo, A. (2019). Comparative evaluation of disinfection mechanism of sodium hypochlorite, chlorine dioxide and electroactivated water on Enterococcus faecalis. Lwt. 102, 205–213. doi: 10.1016/j.lwt.2018.12.041
Eruslanov, E., and Kusmartsev, S. (2010). Identification of ROS using oxidized DCFDA and flow-cytometry. Methods Mol. Biol. 594, 57–72. doi: 10.1007/978-1-60761-411-1_4
Fukuzaki, S. (2006). Mechanisms of Actions of Sodium Hypochlorite in Cleaning and Disinfection Processes. Biocontrol Sci. 11, 147–157. doi: 10.4265/bio.11.147
Gómez-López, V. M., Rajkovic, A., Ragaert, P., Smigic, N., and Devlieghere, F. (2009). Chlorine dioxide for minimally processed produce preservation: a review. Trends Food Sci. Technol. 20, 17–26. doi: 10.1016/j.tifs.2008.09.005
Goodburn, C., and Wallace, C. A. (2013). The microbiological efficacy of decontamination methodologies for fresh produce: A review. Food Control 32, 418–427. doi: 10.1016/j.foodcont.2012.12.012
Gray, M. J., Wholey, W.-Y., and Jakob, U. (2013). Bacterial Responses to Reactive Chlorine Species. Annu. Rev. Microbiol. 67, 141–160. doi: 10.1146/annurev-micro-102912-142520
Liu, S. S., Qu, H. M., Yang, D., Hu, H., Liu, W. L., Qiu, Z. G., et al. (2018). Chlorine disinfection increases both intracellular and extracellular antibiotic resistance genes in a full-scale wastewater treatment plant. Water Res. 136, 131–136. doi: 10.1016/j.watres.2018.02.036
Livak, K. J., and Schmittgen, T. D. (2001). Analysis of Relative Gene Expression Data Using Real-Time Quantitative PCR and the 2−ΔΔCT Method. Methods 25, 402–408. doi: 10.1006/METH.2001.1262
McKenna, S. M., and Davies, K. J. A. (1988). The inhibition of bacterial growth by hypochlorous acid. Possible role in the bactericidal activity of phagocytes. Biochem. J. 254, 685–692. doi: 10.1042/bj2540685
Mei, G. Y., Tang, J., Bach, S., and Kostrzynska, M. (2017). Changes in gene transcription induced by hydrogen peroxide treatment of verotoxin-producing Escherichia coli O157:H7 and non-O157 serotypes on romaine lettuce. Front. Microbiol. 8:477. doi: 10.3389/fmicb.2017.00477
Mei, G. Y., Tang, J., Carey, C., Bach, S., and Kostrzynska, M. (2015). The effect of oxidative stress on gene expression of Shiga toxin-producing Escherichia coli (STEC) O157: H7 and non-O157 serotypes. Int. J. Food Microbiol 215, 7–15. doi: 10.1016/j.ijfoodmicro.2015.07.029
Niki, E. (2009). Lipid peroxidation: Physiological levels and dual biological effects. Free Radic. Biol. Med. 47, 469–484. doi: 10.1016/j.freeradbiomed.2009.05.032
Ofori, I., Maddila, S., Lin, J., and Jonnalagadda, S. B. (2017). Chlorine dioxide oxidation of Escherichia coli in water – A study of the disinfection kinetics and mechanism. J. Environ. Sci. Heal. Part A 52, 598–606. doi: 10.1080/10934529.2017.1293993
Ofori, I., Maddila, S., Lin, J., and Jonnalagadda, S. B. (2018). Chlorine dioxide inactivation of Pseudomonas aeruginosa and Staphylococcus aureus in water: The kinetics and mechanism. J. Water Process Eng. 26, 46–54. doi: 10.1016/J.JWPE.2018.09.001
Praeger, U., Herppich, W. B., and Hassenberg, K. (2018). Aqueous chlorine dioxide treatment of horticultural produce: Effects on microbial safety and produce quality–A review. Crit. Rev. Food Sci. Nutr. 58, 318–333. doi: 10.1080/10408398.2016.1169157
Rajkovic, A., Smigic, N., and Devlieghere, F. (2010). Contemporary strategies in combating microbial contamination in food chain. Int. J. Food Microbiol. 141, S29–S42. doi: 10.1016/J.IJFOODMICRO.2009.12.019
Rodgers, S. L., Cash, J. N., Siddiq, M., and Ryser, E. T. (2004). A Comparison of Different Chemical Sanitizers for Inactivating Escherichia coli O157:H7 and Listeria monocytogenes in Solution and on Apples, Lettuce, Strawberries, and Cantaloupe. J Food Prot 67, 721–731. doi: 10.4315/0362-028X-67.4.721
Shu, X., Singh, M., Karampudi, N. B. R., Bridges, D. F., Kitazumi, A., Wu, V. C., et al. (2020). Xenobiotic effects of chlorine dioxide to Escherichia coli O157: H7 on non-host tomato environment revealed by transcriptional network modeling: implications to adaptation and selection. Front. Microbiol 11:1122. doi: 10.3389/fmicb.2020.01122
Singh, R., Lemire, J., Mailloux, R. J., and Appanna, V. D. (2008). A novel strategy involved anti-oxidative defense: The conversion of NADH into NADPH by a metabolic network. PLoS One 3:e2682. doi: 10.1371/journal.pone.0002682
Spaans, S. K., Weusthuis, R. A., Van Der Oost, J., and Kengen, S. W. (2015). NADPH-generating systems in bacteria and archaea. Front. Microbiol 6:742. doi: 10.3389/fmicb.2015.00742
Stanley, N. R., Pattison, D. I., and Hawkins, C. L. (2010). Ability of hypochlorous acid and N -chloramines to chlorinate DNA and its constituents. Chem. Res. Toxicol. 23, 1293–1302. doi: 10.1021/tx100188b
Sun, X., Baldwin, E., and Bai, J. (2019). Applications of gaseous chlorine dioxide on postharvest handling and storage of fruits and vegetables – A review. Food Control 95, 18–26. doi: 10.1016/j.foodcont.2018.07.044
Tadepalli, S., Bridges, D. F., Anderson, R., Zhang, R., and Wu, V. C. H. (2019). Synergistic effect of sequential wash treatment with two different low-dosage antimicrobial washes in combination with frozen storage increases Salmonella Typhimurium and Listeria monocytogenes reduction on wild blueberries. Food Control 102, 87–93. doi: 10.1016/j.foodcont.2019.03.013
Tadepalli, S., Bridges, D. F., Driver, R., and Wu, V. C. H. (2018). Effectiveness of different antimicrobial washes combined with freezing against Escherichia coli O157:H7, Salmonella Typhimurium, and Listeria monocytogenes inoculated on blueberries. Food Microbiol. 74, 34–39. doi: 10.1016/j.fm.2018.02.018
Tiemann, M. (2014). Safe drinking water act (SDWA): a summary of the act and its major requirements. Washington,: Congressional Research Service.
Vollmer, A. C., and Bark, S. J. (2018). Twenty-Five Years of Investigating the Universal Stress Protein: Function, Structure, and Applications. in Advances in Applied Microbiology 102, 1–36. doi: 10.1016/bs.aambs.2017.10.001
Wu, V. C. H. (2008). A review of microbial injury and recovery methods in food. Food Microbiol. 25, 735–744. doi: 10.1016/J.FM.2008.04.011
Wu, V. C.-H. (2016). Chlorine Dioxide (ClO2),” in Postharvest Management Approaches for Maintaining Quality of Fresh Produce. Cham: Springer International Publishing, 209–218. doi: 10.1007/978-3-319-23582-0_12
Wu, V. C. H., and Kim, B. (2007). Effect of a simple chlorine dioxide method for controlling five foodborne pathogens, yeasts and molds on blueberries. Food Microbiol. 24, 794–800. doi: 10.1016/J.FM.2007.03.010
Yang, J., Tang, C. B., Xiao, J., Du, W. F., and Li, R. (2018). Influences of epigallocatechin gallate and citric acid on Escherichia coli O157:H7 toxin gene expression and virulence-associated stress response. Lett. Appl. Microbiol. 67, 435–441. doi: 10.1111/lam.13058
Keywords: chlorine dioxide, sodium hypochlorite, oxidative stress, Escherichia coli O157:H7, cellular oxidation
Citation: Bridges DF, Lacombe A and Wu VCH (2022) Fundamental Differences in Inactivation Mechanisms of Escherichia coli O157:H7 Between Chlorine Dioxide and Sodium Hypochlorite. Front. Microbiol. 13:923964. doi: 10.3389/fmicb.2022.923964
Received: 20 April 2022; Accepted: 30 May 2022;
Published: 17 June 2022.
Edited by:
Lin Lin, Jiangsu University, ChinaReviewed by:
Andrea Fin, University of Turin, ItalyLoris Pinto, Institute of Sciences of Food Production, Italian National Research Council, Italy
Copyright © 2022 Bridges, Lacombe and Wu. This is an open-access article distributed under the terms of the Creative Commons Attribution License (CC BY). The use, distribution or reproduction in other forums is permitted, provided the original author(s) and the copyright owner(s) are credited and that the original publication in this journal is cited, in accordance with accepted academic practice. No use, distribution or reproduction is permitted which does not comply with these terms.
*Correspondence: Vivian C. H. Wu, dml2aWFuLnd1QHVzZGEuZ292