- 1Department of Microbiology, Immunobiology and Genetics, Max Perutz Labs, Vienna Biocenter (VBC), University of Vienna, Vienna, Austria
- 2Molecular Systems Biology, Department of Functional and Evolutionary Ecology, Faculty of Life Sciences, University of Vienna, Vienna, Austria
- 3Research Group Bioinformatics and Computational Biology, Faculty of Computer Science, University of Vienna, Vienna, Austria
- 4Department of Theoretical Chemistry, University of Vienna, Vienna, Austria
- 5Vienna Metabolomics Center, University of Vienna, Vienna, Austria
In Pseudomonas aeruginosa, the RNA chaperone Hfq and the catabolite repression protein Crc act in concert to regulate numerous genes during carbon catabolite repression (CCR). After alleviation of CCR, the RNA CrcZ sequesters Hfq/Crc, which leads to a rewiring of gene expression to ensure the consumption of less preferred carbon and nitrogen sources. Here, we performed a multiomics approach by assessing the transcriptome, translatome, and proteome in parallel in P. aeruginosa strain O1 during and after relief of CCR. As Hfq function is impeded by the RNA CrcZ upon relief of CCR, and Hfq is known to impact antibiotic susceptibility in P. aeruginosa, emphasis was laid on links between CCR and antibiotic susceptibility. To this end, we show that the mexGHI-opmD operon encoding an efflux pump for the antibiotic norfloxacin and the virulence factor 5-Methyl-phenazine is upregulated after alleviation of CCR, resulting in a decreased susceptibility to the antibiotic norfloxacin. A model for indirect regulation of the mexGHI-opmD operon by Hfq is presented.
Introduction
Pseudomonas aeruginosa (Pae) is associated with a broad spectrum of acute and chronic infections (Kerr and Snelling, 2009; Williams et al., 2010), and notorious for its resistance toward a variety of antibiotics (Pang et al., 2019). The pathogenic potential of Pae is based on its metabolic versatility, permitting fast adaptation to changing environmental conditions and to different niches such as soil, marine habitats, or different organisms (Gellatly and Hancock, 2013).
In Pae, the global regulator Hfq is involved in small RNA-mediated riboregulation as well as in carbon catabolite repression (CCR; Sonnleitner and Bläsi, 2014; Ferrara et al., 2015; Moreno et al., 2015; Sonnleitner et al., 2017). The function of Hfq in riboregulation of Gram-negative bacteria has been well studied. Hfq is known to stabilize sRNAs and to facilitate their annealing with target mRNAs (Santiago-Frangos and Woodson, 2018). Moreover, in Pae, Hfq was shown to bind directly to mRNAs that contain (AAN)n or (ARN)n repeats, in which A is an adenine, R is a purine (A/G), and N is any nucleotide. When present in the translation initiation region, these motifs can engage the tripartite binding pockets on the distal face of Hfq (Link et al., 2009; Malecka et al., 2021) to block translation during CCR (Sonnleitner and Bläsi, 2014). Hereby, the catabolite repression protein Crc acts as a co-repressor by stabilizing the Hfq-RNA complex (Sonnleitner et al., 2018; Pei et al., 2019; Malecka et al., 2021). Transcriptome analyses with PAO1Δhfq and PAO1Δcrc revealed many overlapping mRNA targets, regulation of which is governed by both, Hfq and Crc, during CCR (Sonnleitner et al., 2018). In this way, Hfq/Crc ensure a hierarchical use of nutrients by precluding the synthesis of uptake- and degradative functions required for metabolization of C/N sources other than the available preferred one(s), such as C4-dicarboxylates. Furthermore, a ChIP-seq approach showed that Hfq and Crc co-associate with nascent transcripts, whereas Crc was not observed on RNAs in the absence of Hfq (Kambara et al., 2018). These in vivo findings are in accord with previous results, showing that Crc is devoid of RNA binding activity (Milojevic et al., 2013), and that the association of Crc with RNA requires Hfq (Sonnleitner et al., 2018). Moreover, a RNA-seq based comparative transcriptome analysis of the Pae strains PAO1Δhfq and PAO1ΔhfqΔcrc strongly suggested that Crc does not exert an independent regulatory function (Malecka et al., 2021).
Recent structural studies have revealed that Hfq/Crc complexes formed on individual mRNAs vary in form and composition (Dendooven, 2020). It is therefore conceivable that Hfq/Crc mediated regulation results in differential translational repression of target genes. This hypothesis may explain variations in translational control of individual genes in Pae crc deletion strains (Corona et al., 2018), which can result in a change of the order of metabolite utilization (La Rosa et al., 2016). In fact, the absence of Crc caused an unbalanced metabolism with poorly optimized metabolic fluxes in P. putida (La Rosa et al., 2015; Molina et al., 2019).
The response to different C-sources is coordinated by the RNA CrcZ (Sonnleitner et al., 2009; Valentini et al., 2014). CrcZ sequesters Hfq and/or Hfq/Crc when CCR is alleviated (Sonnleitner and Bläsi, 2014; Sonnleitner et al., 2018), which in turn leads to the metabolization of non- or less preferred C sources, such as mannitol. CrcZ expression is under control of the alternative sigma factor RpoN and the two-component system CbrA/B (Sonnleitner et al., 2009; Abdou et al., 2011). The signal responsible for CbrA activation remains unknown, but it is thought to be related to the internal energy status of the cell (Valentini et al., 2014). Furthermore, as CrcZ acts as a decoy for Hfq, CrcZ does not only interfere with direct translational repression by Hfq/Crc (Sonnleitner and Bläsi, 2014) but also with Hfq-mediated riboregulation by sRNAs (Sonnleitner et al., 2017). Moreover, Hfq-mediated regulation is linked to complex behavior including the control of virulence genes (Sonnleitner et al., 2003, 2006), quorum sensing (Kimyon et al., 2016; Yang and Lan, 2016), biofilm formation (Pusic et al., 2016) as well as antibiotic susceptibility (Pusic et al., 2018; Sonnleitner et al., 2020).
Most studies on Hfq/Crc mediated regulation in Pae have been performed during growth on specific carbon sources (Corona et al., 2018; Kambara et al., 2018; Sonnleitner et al., 2018; Molina et al., 2019). Comparatively, little is known on the dynamic rewiring of gene expression during diauxic growth in the simultaneous presence of a preferred carbon source, such as succinate, and a non-preferred one, such as mannitol (Valentini et al., 2014). Here, we aimed at obtaining a picture on the re-programing of regulatory networks and genes by using a multiomics approach, revealing changes in the transcriptome (RNA-seq), translatome (Ribo-seq), and proteome (MS, mass spectrometry). On the one hand, it was expected that these studies verify and validate transcripts subject to Hfq/Crc regulation during CCR. On the other hand, we anticipated that these studies reveal novel target genes that are differentially regulated after relief of CCR, i.e., after titration of Hfq/Crc by the RNA CrcZ, the levels of which are known to rise when the preferred carbon source is exhausted (Valentini et al., 2014). Here, we report an upregulation of the mexGHI-opmD operon encoding an efflux pump that expels the antibiotic norfloxacin and the virulence factor 5-Methyl-phenazine (5-Me-PCA). A model is presented for an indirect upregulation of the mexGHI-opmD operon after CrcZ mediated titration of Hfq.
Materials and Methods
RNA-seq and Ribo-seq Library Preparation and Analyses
Three biological replicates of P. aeruginosa strain O1 (PAO1; Holloway et al., 1979) were grown at 37°C in 100 ml Basal-Salt medium [BSM; 30.8 mM K2HPO4, 19.3 mM KH2PO4, 15 mM (NH4)SO4, 1 mM MgCl2, and 2 μM FeSO4] supplemented with 5 mM succinate and 40 mM mannitol (Sonnleitner et al., 2009). Total RNA was prepared from each of the three biological replicates after they reached an OD600 of 0.4 [time 1 (T1)], 0.7 [time 2 (T2)], and 1.2 [time 3 (T3); Figure 1A], respectively. 10 ml samples were withdrawn from each culture, the cells were harvested by centrifugation at 4,000 g for 15 min at 4°C and frozen in liquid nitrogen. Total RNA was extracted using the TRIzol reagent (Ambion) according to the manufacturer’s instructions. DNA was removed using DNAse I (Roche), followed by phenol-chloroform purification and ethanol precipitation with 1/10 volume of 3 M NaOAc, pH 5.5. To deplete ribosomal RNAs, the Ribo-Zero™ Magnetic Kit for Gram-negative Bacteria (Epicentre) was used. The libraries were constructed using the NEBNext® Ultra™ Directional RNA Library Preparation Kit from Illumina. Around 100 base pair single end sequence reads were generated using the Illumina HiSeq V4 platform at the Vienna Biocenter Campus Science Support Facility.1 Quality control assessment, sequencing adapter removal, and mapping of the samples against the PAO1 reference genome (NCBI accession number NC_002516.2) were performed as described previously with Segemehl using default parameters (Hoffmann et al., 2009). Reads mapping to regions annotated as either rRNA or tRNA were discarded. The mapped sequencing data were prepared for visualization using the ViennaNGS tool box, and visualized with the UCSC Genome Browser (Wolfinger et al., 2015). Reads per gene were counted using BEDTools (Quinlan and Hall, 2010) and the Refseq annotation of P. aeruginosa (NC_002516.2). Differential gene expression analysis was performed with DESeq (Anders and Huber, 2010). The levels of transcripts with a fold-change (FC) greater than 2 and a multiple testing adjusted value of p below 0.05 were considered to be significant (shown in bold in Supplementary Table S1).
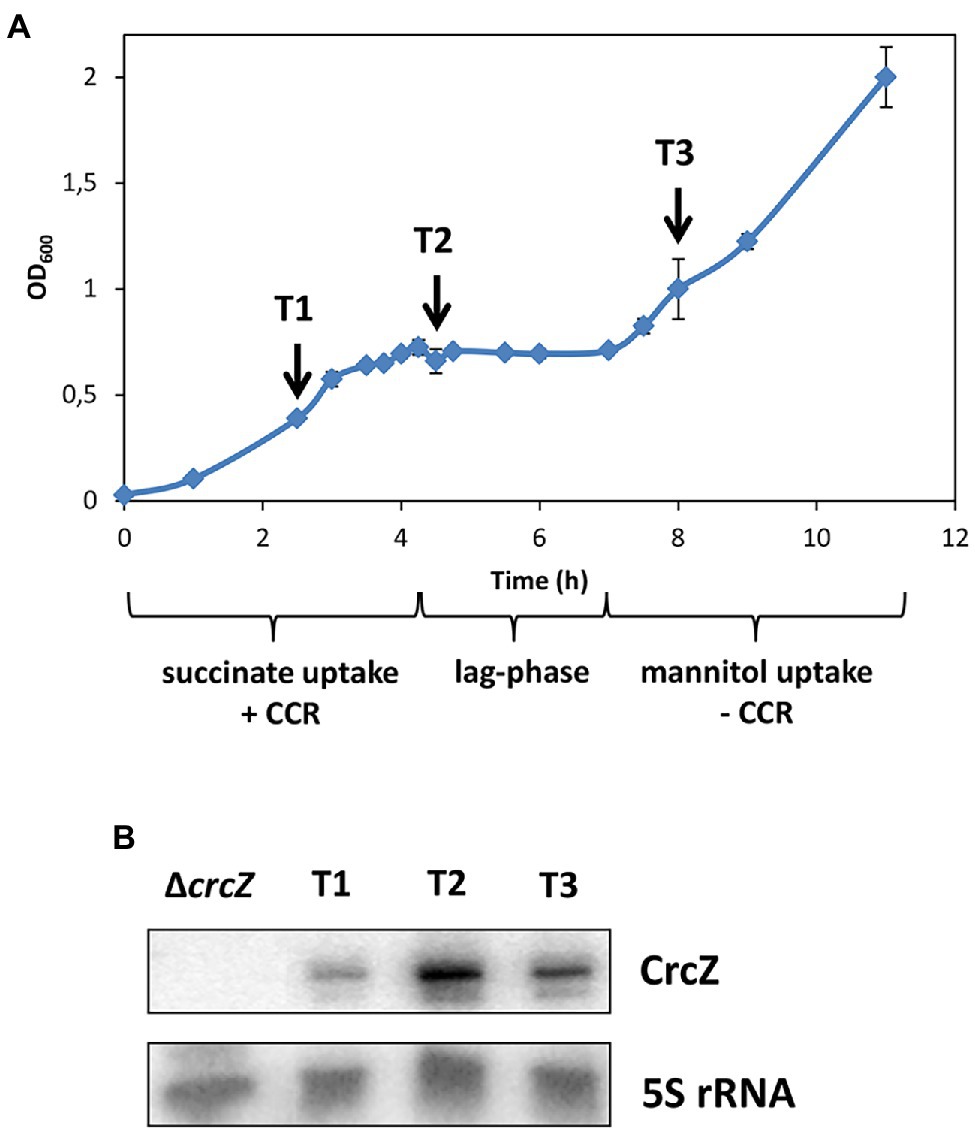
Figure 1. (A) Growth of strain PAO1 in Basal-Salt medium (BSM) supplemented with 5 mM succinate and 40 mM mannitol. The arrows indicate the times of sample withdrawal during carbon catabolite repression (CCR; T1), at the beginning of the lag-phase (T2) and after relief of CCR (T3). (B) The steady state levels of the regulatory RNA CrcZ were monitored by Northern blot analysis at T1, T2, and T3, respectively. 5S RNA was used as a loading control.
Ribosome profiling of elongating ribosomes (Ribo-seq; Ingolia et al., 2009) was performed with the same 100 ml cultures as used for the RNA-seq analyses. 10 ml samples were withdrawn from each culture after growth to an OD600 of 0.4 [time 1 (T1)], 0.7 [time 2 (T2)], and 1.2 [time 3 (T3); Figure 1A], respectively, transferred to new flasks and treated for 10 min with chloramphenicol (300 μg/ml) to stop translation. The cells were then harvested by centrifugation at 4,000 g for 15 min at 4°C, the pellet was washed in 50 ml ice cold lysis buffer (10 mM MgOAc, 60 mM NH4Cl, 10 mM TRIS–HCl, and pH 7.6), again pelleted by centrifugation at 5,000 g for 15 min at 4°C, and then frozen in liquid nitrogen. The pellets were re-suspended in 1 ml ice cold lysis buffer containing 0.2% Triton X-100, 100 μg/ml chloramphenicol, and 100 U/ml DNAse I, frozen in liquid nitrogen and cryogenically pulverized by repeated cycles of grinding in a pre-chilled mortar and freezing in a dry ice/ethanol bath. The lysates were centrifuged at 15,000 g for 30 min at 4°C to remove cellular debris. About 100 μl aliquots of the cleared lysates were treated with 4 μl of Micrococcal Nuclease (MNase, NEB) and 6 μl of the RiboLock RNase inhibitor (Thermo Scientific) for 1 h at 25°C with continuous shaking at 450 rpm. The lysates were layered onto 10%–40% linear sucrose density gradients in lysis buffer and centrifuged at 256,000 g for 3 h at 4°C. About 500 μl gradient fractions were collected by continuously monitoring the absorbance at 260 nm. The RNA was extracted from fractions containing 70S ribosomes with phenol-chloroform-isoamyl alcohol (25:24:1), and precipitated with ethanol. The samples were treated with DNase I (TURBO™ DNase, Thermo Scientific) and separated on a 15% polyacrylamide gel containing 8 M urea. Ribosome protected mRNA fragments ranging in size from 20 to 40 nucleotides were removed and eluted from the polyacrylamide gel by overnight incubation in elution buffer (0.3 M NaOAc, 1 mM EDTA) at 4°C, which was followed by another phenol-chloroform-isoamyl alcohol (25:24:1) extraction and ethanol precipitation. The quality of the RNA samples was subsequently analyzed with a 2100 Bioanalyzer and an Agilent RNA 6000 Pico Kit (Agilent Technologies). The RNA was further processed into cDNA libraries with NEBNext™ Small RNA Library Prep Set for Illumina® and their quality was assessed with the 2100 Bioanalyzer and a High Sensitivity DNA Kit (Agilent Technologies). Pipin Prep™ was used to purify the 140–160 bp cDNA products which corresponded to adapter-ligated 20–40 nucleotide long ribosomal footprints. RNA sequencing and data processing were performed as described above. The raw RNA-seq and Ribo-seq data were deposited in the ENA under accession number PRJEB52258.
Extraction of Cellular Proteins
Cellular proteins were extracted from the same 100 ml cultures of two biological replicates as used for the RNA-seq and Ribo-seq analyses. 10 ml samples were withdrawn from each culture after growth to an OD600 of 0.4 [time 1 (T1)], 0.7 [time 2 (T2)], and 1.2 [time 3 (T3); Figure 1A], respectively, transferred to new flasks and treated for 10 min with chloramphenicol (300 μg/ml) to stop translation. The cells were harvested by centrifugation at 4,000 g at 4°C for 15 min and frozen in liquid nitrogen. The pellets were re-suspended in 200 μl 1x Laemmli buffer per 0.5 OD600 unit. The cell suspensions were sonicated for 1 min at 40% power for 40 cycles (Bandelin Sonopuls GM70), and then boiled for 5 min at 95°C. The cell debris was removed by centrifugation at 24,000 g for 10 min at room temperature. The samples (100 μg/lane) were loaded onto a 12.5% SDS polyacrylamide gel, which was stained with colloidal coomassie stain (Roth). The gel slices were cut into pieces and placed in 1.5 ml microcentrifuge tubes. About 1 ml of 200 mM ammonium bicarbonate (AmBic) in 50% acetonitrile (ACN) was added to each sample followed by incubation for 1 h at 37°C. The supernatant was discarded and the samples were further incubated with 500 μl of 50 mM AmBic in 5% ACN for 15 min at 37°C. The solution was removed and the gels slices were incubated two times in 500 μl of 100% ACN for 10 min at 37°C to remove water. The dried proteins were digested with 100 μl of trypsin (Thermo; 25 ng/μl), re-suspended in 25 mM AmBic in 10% ACN and 5 mM CaCl2, and further incubated for 16 h at 37°C. The peptides were extracted with 150 μl of 50% ACN/1% of formic acid (FA) by sonication in a low intensity ultrasound bath for 3 min, after which the supernatants were transferred to 1.5 ml Eppendorf tubes. The gel was further incubated with 100 μl 90% ACN/1% FA for 15 min at room temperature. The supernatant was transferred to the same tubes as in the previous step and dried in a vacuum concentrator. The protein digests were desalted with C18 solid phase extraction (SPE; Agilent Technologies) and carbon graphite SPE as described by Furuhashi et al. (2014). After desalting, the corresponding eluates were pooled and dried in a vacuum concentrator.
Liquid Chromatography-Mass Spectrometry Analysis
The samples were analysed with an Orbitrap Elite hybrid ion trap-orbitrap mass spectrometer (MS; Thermo Fisher Scientific) coupled to an UltiMate 3000 RSLCnano system (Thermo Fisher Scientific). The peptides were re-suspended in 99% of ACN and 1% of FA to a final concentration of 0.2 μg/μl and separated on a 50 cm EASY-Spray C18 LC column (Thermo Fisher Scientific). The peptides were eluted with a 120 ml gradient from 2 to 40% of solvent B (90% ACN, 0.1% FA) at a flow rate of 300 μl/min. Full scans were obtained with an Orbitrap mass analyzer. The scan range was set to 350–1800 Th, the resolution to 120,000 at 400 m/z, the AGC target to 1,000,000 ions, and the maximum injection time to 100 ms. Up to 20 most intense precursor ions (charge ≥ 2) were selected for CID fragmentation (normalized collision energy 35%, activation time 10 ms, isolation width 2.0 Th, and minimum signal threshold 1,000) in an ion trap. The MS2 AGC target was set to 5,000 ions and the maximum injection time to 50 ms. The dynamic exclusion of fragmented precursors was set to 30 s.
Peptide Identification and Quantification
The peptide identification and protein quantification was done with MaxQuant (version 1.5; Cox and Mann, 2008; Cox et al., 2011). The spectra were searched against the P. aeruginosa protein database.2 The following settings were used: maximum two missed cleavages were allowed, methionine oxidation and protein N-terminal acetylation were set to variable modifications and mass tolerance for precursor mass was set to 4.5 ppm and for fragment masses to 0.6 Da. The maximum FDR for peptides and proteins was 1%. Match between runs function was used with 20 and 0.7 min alignment and matching time window, respectively. Protein quantification was done with a peptide count ≥2. Filtering and further data processing and statistics were done with Perseus 1.5 software and Excel.
Northern Blot Analyses
Total RNA was isolated from strain PAO1 at OD600 = 0.4 (T1), 0.7 (T2), and 1.2 (T3) during diauxic growth in BSM medium supplemented with succinate (5 mM) and mannitol (40 mM), from strain PAO1 (pME10011) after growth to an OD600 of 2.0 in BSM medium supplemented with succinate (40 mM) and mannitol (40 mM), respectively, as well as from strains PAO1 (pME10011; pMMB67HE) and PAO1 (pME10011; pMMBcrcZ) after growth to an OD600 of 2.0 in BSM medium supplemented with succinate (40 mM). The corresponding RNAs were purified using the TRIZol reagent (Ambion) according to the manufacturer’s instructions. The levels of the CrcZ RNA and 5S RNA (control) were determined by Northern-blotting using 5 μg of total RNA. The RNA samples were resuspended in 1x RNA loading dye (95% formamide, 10 mM EDTA pH 8.0, 0.025% xylene cyanol FF, and 0.025% bromophenol blue), denatured for 5 min at 98°C, separated on 6% polyacrylamide/8 M Urea gel, and then transferred onto a nylon membrane (Amersham) by electro-blotting. The RNAs were cross-linked to the membrane by exposure to UV light. The membranes were hybridized with gene-specific 32P-end-labeled oligonucleotides [CrcZ: K3 (5’GCT GGA GTC GTT ACG TGT TG-3`); 5S rRNA: I26 (5`-CCC CAC ACT ACC ATC GGC GAT GCG TCG-3`); Sonnleitner et al., 2018]. The hybridization signals were visualized using a PhosphoImager (Molecular Dynamics). The normalization was performed with ImageQuant TL version 8.1.
Determination of the Minimal Inhibitory Concentration
PAO1 was grown to an OD600 of 0.3–0.4 in BSM supplemented with 40 mM succinate and mannitol, respectively. 2 ml of the cultures were transferred to 10 ml glass tubes, containing 50 μl norfloxacin in serial dilutions (concentration range 78 μg/L–10 mg/L). The cultures were shaken for 16 h at 37°C followed by measurement of the OD600. The MIC corresponds to the lowest concentration of antibiotics that impacted growth.
Candida albicans Bioassay
300 μl of overnight culture of C. albicans was mixed with 10 ml YPD agar medium [2% Yeast Extract (Sigma-Aldrich), 4% peptone (Sigma-Aldrich), 4% Dextrose (Sigma-Aldrich), and 0.75% Agar (Merk)], poured into Petri dishes and incubated for 36 h at 37°C. 10 μl of the PAO1 culture grown in BSM (see above) supplemented with 40 mM succinate or 40 mM mannitol (OD600 = 3.0) were spotted on top of the C. albicans lawns, and then incubated for 48 h at 37°C.
Determination of the Pyocyanin Levels
Strain PAO1 was grown at 37°C for 24 h in 20 ml of BSM supplemented with 40 mM succinate and mannitol, respectively. The strains PAO1 [pMMB67HE (vector control); Fürste et al., 1986] and PAO1 [pMMBcrcZ (Ptac driven constitutive expression of crcZ); Sonnleitner and Bläsi, 2014], respectively, were grown at 37°C for 24 h in 20 ml of BSM supplemented with 40 mM succinate. Pyocyanin was extracted from 4 ml of culture supernatant with 2 ml of chloroform, followed by an extraction with 1 ml of 0.1 M HCl. The concentration of pyocyanin was measured at A520, and is given as A520/ml of the culture supernatant.
β-Galactosidase Assays
The β-galactosidase activities were determined as described by Miller (1972). The cells were permeabilized with 5% toluene. The β-galactosidase units in the different experiments were derived from three independent experiments.
Results and Discussion
CrcZ Levels During Diauxic Growth
To monitor in parallel changes in the transcriptome, translatome, and proteome during diauxic growth, strain PAO1 was grown in BSM minimal medium supplemented with 5 mM succinate (preferred carbon source) and 40 mM mannitol (less preferred carbon source). Under these conditions, the cells grew first exponentially as CCR is anticipated to prioritize metabolization of succinate (Figure 1A). After exhaustion of succinate, a lag-phase was observed after which growth resumed (Figure 1A).
In Pae, translational regulation by Hfq/Crc is predominantly negative during CCR (Sonnleitner and Bläsi, 2014; Kambara et al., 2018; Sonnleitner et al., 2018). After alleviation of CCR, the levels of the regulatory RNA CrcZ were expected to increase and to act as a decoy to abrogate Hfq/Crc-mediated translational repression (Sonnleitner and Bläsi, 2014; Valentini et al., 2014; Sonnleitner et al., 2018). As anticipated from studies that assessed CrcZ synthesis during growth on different carbon sources (Sonnleitner et al., 2009; Valentini et al., 2014), the levels of CrcZ RNA were the lowest during CCR, i.e., during growth on succinate (Figure 1B; T1), were augmented at the beginning of the lag-phase (Figure 1B; T2), and leveled off when growth resumed apparently on mannitol (Figure 1B; T3). As the levels of CrcZ varied during diauxic growth, the experimental set-up was deemed suitable to capture Hfq-dependent changes in the transcriptome, translatome, and proteome after relief of CCR.
Data Analysis
Several studies on the Hfq/Crc regulon are based on RNA-seq based transcriptomics (Sonnleitner et al., 2012, 2018) or proteomics (Linares et al., 2010), which do not provide information on post-transcriptional regulation when analyzed alone. Here, we have used a multiomics approach to compare the transcriptomes, translatomes, and proteomes of cells harvested at T3 and T2 with that of T1, respectively. The same dataset was generated for the comparison of T3 with T2. The following criteria were applied for differential gene expression analysis of the multiomics data. Only annotated protein coding genes deposited in the Pseudomonas genome database (Winsor et al., 2016) were considered for comparison. For the Venn diagrams (Supplementary Figure S1) all genes with a low expression rate (less than 100 RNA-seq or 50 Ribo-seq reads) were disregarded. In addition, a value of p (adjusted for multiple testing) of 0.05 was set as a threshold for significance and the difference in FC (fold-change) had to exceed ±2 for a given gene.
We did not quantitatively compare the three datasets in terms of the correlation between gene expression- and protein fold-changes. Nevertheless, we consider this study as a useful resource for the Pae community interested in studying CCR regulated genes. Owing to Hfq/Crc-mediated translational repression of many genes during CCR (Corona et al., 2018; Sonnleitner et al., 2018), we primarily focused in follow-up studies on differentially regulated genes/proteins implicated in antibiotic susceptibility after relief of CCR.
Data at a Glance
RNA-seq
The comparison of T2 with T1 revealed 3,302 (1,620 downregulated/1,682 upregulated) differentially abundant transcripts, whereas the comparisons of T3 with T1 and T3 with T2 revealed 1,880 (739 downregulated/1,141 upregulated) and 2,698 (782 downregulated/1,916 upregulated) differentially abundant transcripts, respectively (Supplementary Table S1; Supplementary Figure S1).
Ribo-seq
The comparison of T2 with T1 revealed 383 (133 downregulated/250 upregulated) differential changes in ribosomal occupancy, whereas the comparisons of T3 with T1 and T3 with T2 revealed 1,520 (715 downregulated/805 upregulated) and 1,052 (492 downregulated/560 upregulated) differential changes in ribosomal occupancy, respectively (Supplementary Table S1; Supplementary Figure S1).
Proteomics
When compared with the RNA-seq and Ribo-seq analyses, only ~600 proteins were found to be differentially abundant in the T2/T1, T3/T1, and T3/T2 datasets (Supplementary Table S1; Supplementary Figure S1). The low coverage of the proteome might result from the gel-based protein digestion, which is not well suited for the identification of low-abundant peptides and insoluble membrane proteins (Abhyankar et al., 2011; Mayne et al., 2016).
When compared with the RNA-seq data, the number of differentially expressed genes was lower in Ribo-seq. Discrepancies in the number of de-regulated genes in RNA-seq when compared to Ribo-seq data have been reported before (Blevins et al., 2018). Thus, our data show once more the importance of parallel application of these methods for assessment of gene expression. Notwithstanding with RNA-seq and Ribo-seq data, the number of de-regulated proteins was even lower. This observation agreed with other studies, where a poor correlation between mRNA and protein levels have been reported (Greenbaum et al., 2003; Maier et al., 2009).
As shown in the Venn diagrams (Supplementary Figure S1), with 176 genes/proteins the overlap for upregulated genes/proteins with all three approaches was most prominent when T3 was compared with T1, whereas this number decreased to 71 and 16, when T3/T2 and T2/T1, respectively, were compared. In contrast, only a few overlaps were observed for down-regulated genes/proteins in the corresponding data sets (Supplementary Figure S1).
A meta-analysis of normalized synthesis/expression of differentially abundant proteins/transcripts was therefore performed for the T3/T1 dataset. For this purpose, the genes were grouped into the corresponding KEGG pathways (Bolger et al., 2014). As shown in the heat-map, the protein, Ribo-seq and RNA-seq profiles of the T3/T1 dataset were related at the level of the KEGG pathways, although the upregulation of some degradative pathways was more apparent from the proteome data when compared to the Ribo-seq and RNA-seq analyses (Supplementary Figure S2).
Validation of the Omics Data
Most Pseudomonas species lack phosphofructokinase, an enzyme which converts fructose-6-phosphate into fructose-1,6-biphosphate (Temple et al., 1998). The absence of this enzyme impedes assimilation of many carbon sources directly through the glycolytic pathway (Lessie and Phibbs, 1984). In Pae, the assimilation of, e.g., glucose and mannitol proceeds through the pentose phosphate (PP) and Entner-Doudoroff (ED) pathways (Dolan et al., 2020; Park et al., 2020). Hence, growth on mannitol requires a rewiring of gene expression during the lag-phase (Park et al., 2020). To validate the omics data, we first assessed the abundance of key genes/proteins required for mannitol conversion and of the PP and ED pathways. The mtlD and mtlZ genes encode enzymes required for the initial steps of mannitol catabolism (Supplementary Figure S3). The abundance of the mtlD and mtlZ transcripts increased in T2 when compared to T1. Although there was no change in the Ribo-seq reads, there was an apparent increase of the corresponding proteins. The zwf transcript and the encoded enzyme of the PP pathway were upregulated in T2 when compared with T1 (Supplementary Figure S3). Similarly, the key genes of the ED pathway edaA, edd, and pgl as well as the encoded enzymes were up-regulated in T2 when compared with T1 (Supplementary Figure S3). These results are in line with previous studies, showing that pathways required for growth on non-glycolytic carbon sources are repressed in the presence of succinate (Rojo, 2010; Dolan et al., 2020).
Analyses of the T3/T1 Dataset Reveals an Indirect Regulation of the MexGHI-OpmD Efflux Pump by Hfq
In Pae, the function of CCR is not limited to the hierarchical assimilation of carbon sources. It has been reported that distinct C-sources can affect antibiotic susceptibility (Linares et al., 2010; Martinez and Rojo, 2011; Pusic et al., 2018) and other virulence traits (O'Toole et al., 2000; Pusic et al., 2016). In fact, it was shown that the absence of Hfq or titration of Hfq by the RNA CrcZ leads to an increased susceptibility towards several different classes of antibiotics (Pusic et al., 2018; Sonnleitner et al., 2020).
To search for antibiotic resistance determinants affected by CCR, we focused on genes/functions previously implicated in antibiotic susceptibility that were found to be either up- or downregulated in the RNA-seq, Ribo-seq and proteome analyses (Supplementary Figure S1). The best match between the three datasets was obtained for genes/proteins constituting the MexGHI-OpmD efflux pump, which were found to be upregulated upon growth on mannitol at T3 when compared with T1 (Table 1). The MexGHI-OpmD pump has been implicated in the export of xenobiotics, the antibiotic norfloxacin, and the dye acriflavine (Sekiya et al., 2003; Aendekerk et al., 2005). Besides, a precursor of pyocyanin, 5-methylphenazine-1-carboxylate (5-Me-PCA) was shown to be exported through MexGHI-OpmD (Sakhtah et al., 2016), which is important for self-resistance against this highly reactive compound. Like pyocyanin (Dietrich et al., 2006), 5-Me-PCA was shown to induce the mexGHI-opmD operon through activation of the redox-active transcription factor SoxR (Sakhtah et al., 2016).
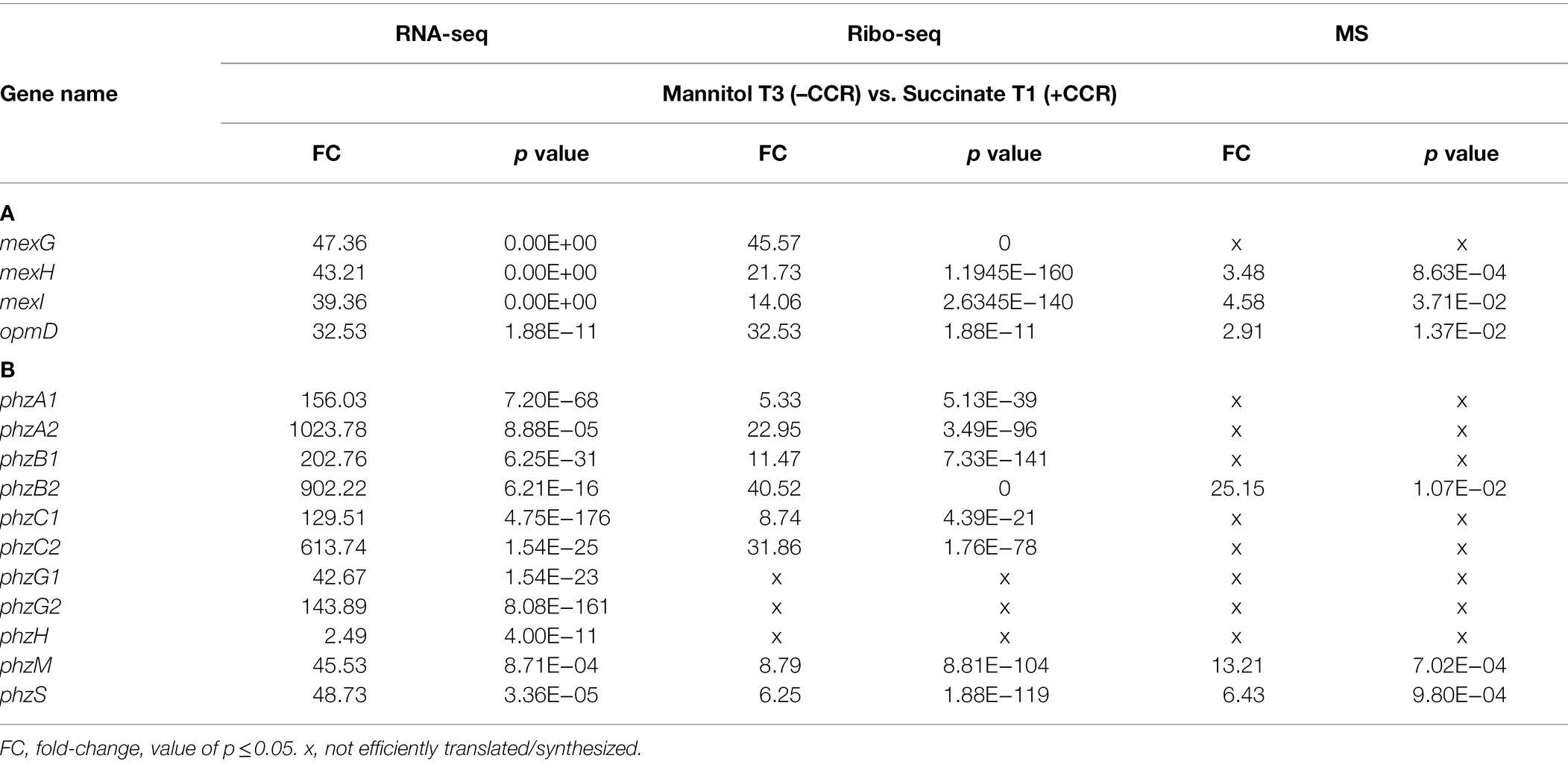
Table 1. T3/T1 datasets of the transcriptome (RNA-seq), translatome (Ribo-seq), and proteome (MS) analyses of genes encoding the (A) MexGHI-OpmD efflux pump and (B) functions required for synthesis of 5-Methyl-phenazine (5-Me-PCA) and pyocyanin.
As the MexGHI-OpmD pump was shown to affect the susceptibility toward norfloxacin (Sekiya et al., 2003), we initially asked whether the observed upregulation of the mexGHI-opmD operon in T3 vs. T1 (Table 1) would result in a reduced susceptibility toward the antibiotic during growth in the presence of mannitol. PAO1 cells grown in BSM medium supplemented with mannitol (MIC = 1.25 μg/ml) were ~ 4-fold more resistant toward the antibiotic when compared to PAO1 grown in the presence of succinate (0.31 μg/ml) as the sole C-source.
As 5-Me-PCA was shown to be exported through MexGHI-OpmD in the clinical strain PA14 (Sakhtah et al., 2016), we further tested whether the increased production of the MexGHI-OpmD pump during growth on mannitol (Table 1; T3/T1) correlates with an enhanced efflux of 5-Me-PCA. For this purpose, a 5-Me-PCA bioassay was used, in which Pae was grown on top of C. albicans lawns. Under these conditions C. albicans can import 5-Me-PCA, which is then modified to a red fluorescent derivative (Gibson et al., 2009; Morales et al., 2010; Sakhtah et al., 2016). As shown in Figure 2A, when the cells were grown in BSM medium containing mannitol and then spotted onto the C. albicans lawn, a red pigmentation was visible around the colony. This was hardly visible when the cells were first grown in BSM medium supplemented with succinate. Hence, the outcome of the bioassay can be reconciled with the omics data in that the MexGHI-OpmD pump is upregulated during growth on mannitol.
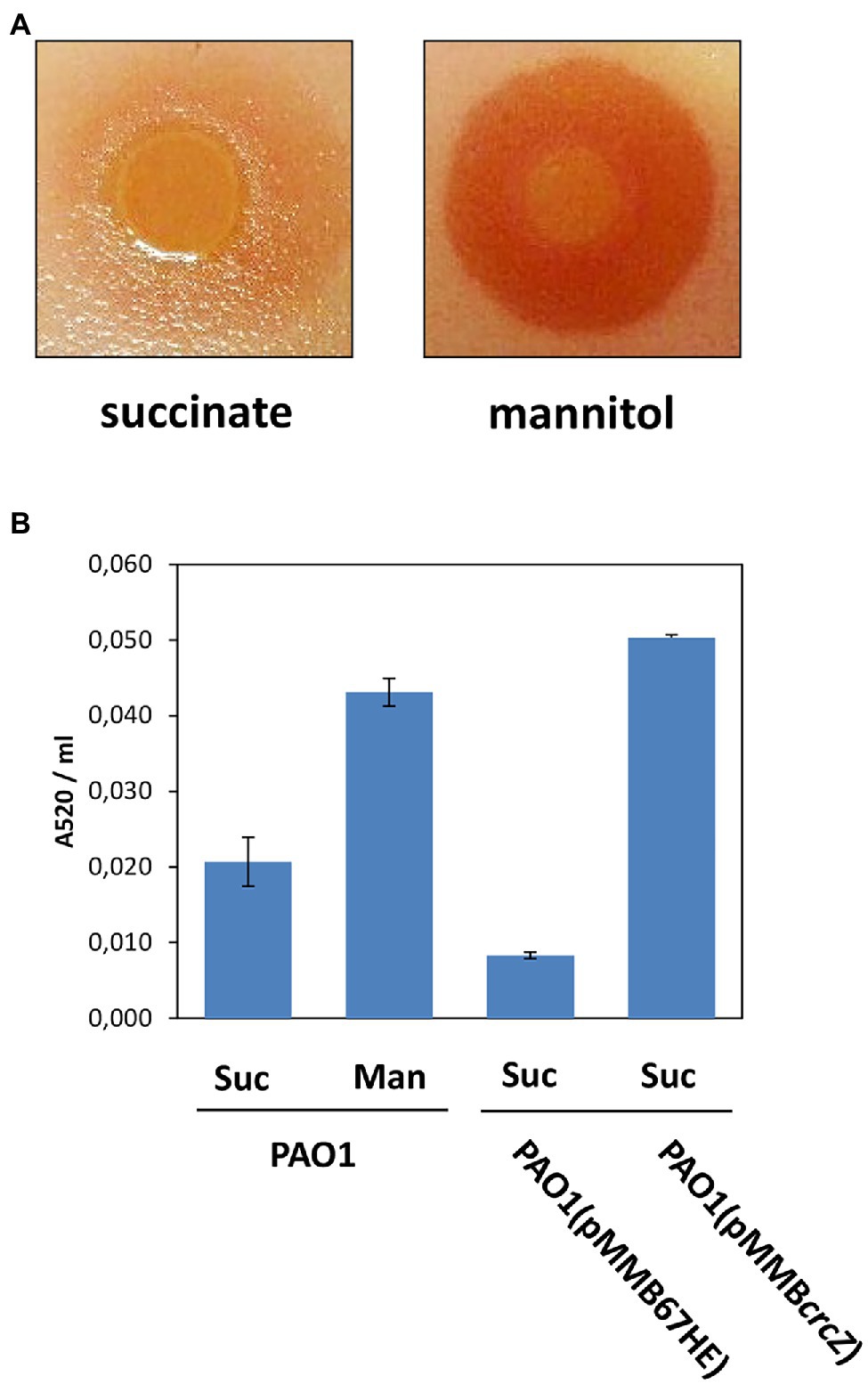
Figure 2. (A) Increased release of 5-Me-PCA results in red phenazine formation on top of Candida albicans lawns upon growth of PAO1 in BSM supplemented with mannitol. (B) Increased pyocyanin production in PAO1 and in PAO1 (pMMBcrcZ) after growth on mannitol and upon ectopic expression of the crcZ gene from plasmid pMMBcrcZ (Sonnleitner and Bläsi, 2014), respectively. The strain PAO1 (pMM67HE) harbors the parental vector of plasmid pMMcrcZ (Sonnleitner and Bläsi, 2014). Suc, succinate; Man, mannitol. The error bars represent SDs.
In addition to the mexGHI-opmD operon, the genes/functions of the phenazine operons phzA1/2—phzG1/2, phzM and phzS, which are required to convert chorismate into pyocyanin (Flood et al., 1972; Mavrodi et al., 2006), were also upregulated in the T3/T1 dataset (Table 1). However, with the exception of the RNA-seq data not all genes/functions could be detected in the Ribo-seq and proteome datasets. The upregulation of the mexGHI-opmD and of some genes of the phenazine pathway was also observed in previous microarray and RNA-seq studies with a PAO1∆hfq strain (Sonnleitner et al., 2006, 2020). Moreover, an enhanced pyocyanin production was observed in a PAO1∆hfq strain when compared with the parental strain (Sonnleitner et al., 2003). Accordingly, when compared with growth in BSM-succinate medium, the pyocyanin production was enhanced after growth of PAO1 in BSM-mannitol medium as well as upon plasmid-mediated overproduction of CrcZ RNA (Figure 2B). Thus, the absence of Hfq as well as conditions expected to titrate Hfq resulted in higher 5-Me-PCA and pyocyanin levels.
How does Hfq affect 5-Me-PCA and pyocyanin synthesis? The phzM gene, encoding the N-methyltransferase presumed to convert PCA to 5-Me-PCA (Parsons et al., 2007), was shown to be negatively regulated by Hfq at the post-transcriptional level in Pae M18 (Wang et al., 2012). In addition, owing to Hfq-binding motifs overlapping with the ribosome binding site, the phzM gene was shown to be translationally repressed by Hfq/Crc in strain PAO1 (Sonnleitner and Bläsi, 2014). Moreover, the phzM mRNA was more abundant after the anticipated titration of Hfq by CrcZ at T2 and T3, when compared with T1 (Supplementary Table S1; Table 1). Thus, we hypothesized that translational repression of phzM by Hfq/Crc in the presence of succinate leads to low levels of 5-Me-PCA as observed in the bioassay (Figure 2A). On the other hand, de-repression of phzM during growth on mannitol, i.e., after synthesis of CrcZ and relief of CCR, could explain the elevated levels of 5-Me-PCA (Figure 2A). To substantiate this hypothesis, we made use of a translational phzM::lacZ reporter gene mounted on plasmid pME10011 (Huang et al., 2012) and determined the synthesis of the PhzM-LacZ protein by measuring the β-galactosidase activity of strain PAO1 (pME10011) at T1 and T3 during diauxic growth (see Figure 1). As shown in Figure 3A, the PhzM-LacZ production was ~5-fold increased at T3 when compared with T1. In addition, the synthesis of the PhzM-LacZ protein was assessed in strain PAO1 (pME10011) after growth to an OD600 of 2.0 in BSM medium supplemented with succinate and mannitol, respectively. When compared with growth in the presence of succinate, phzM::lacZ translation and CrcZ levels were increased in the presence of mannitol (Figure 3B). Furthermore, the strains PAO1 (pMMBcrcZ; pME10011) and PAO1 (pMMB67HE; pME10011) were grown to an OD600 of 2.0 in BSM medium supplemented with succinate. When compared with strain PAO1 (pMMB67HE; pME10011), harboring the parental vector of plasmid pMMBcrcZ (Sonnleitner and Bläsi, 2014), ectopic expression of crcZ in strain PAO1 (pMMBcrcZ; pME10011) resulted in increased PhzM-LacZ production (Figure 3C). Hence, in contrast to Hfq/Crc-mediated translational repression of phzM during growth on succinate (Figure 4A), CrcZ-mediated titration of Hfq during growth on mannitol and the resulting upregulation of the phzM gene can explain the observed increase in 5-Me-PCA and pyocyanin levels (Figure 2). On the other hand, the 5-Me-PCA-dependent activation of SoxR (Sakhtah et al., 2016) can in turn explain the observed upregulation of the mexGHI-opmD operon after alleviation of CCR (Figure 4B). It is also worth mentioning that two other SoxR regulated transcripts, PA2274 and PA3718 (Palma et al., 2005), show a higher abundance after alleviation of CCR (Supplementary Table S1).
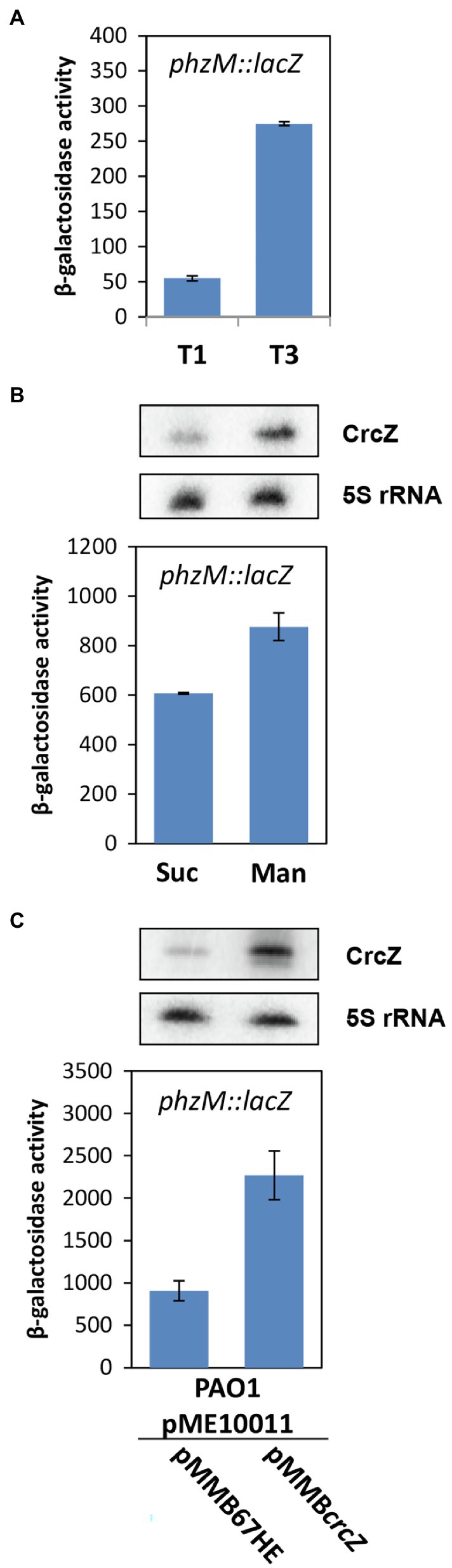
Figure 3. Increased levels of the RNA CrcZ result in upregulation of phzM translation. (A) Strain PAO1 (pME10011) was grown in BSM supplemented with 5 mM succinate and 40 mM mannitol. The β-galactosidase activity conferred by the translational phzM::lacZ reporter gene was determined at T1 and T3 (see Figure 1A), respectively. The corresponding steady state levels of the CrcZ RNA are shown in Figure 1B. (B) Increased phzM::lacZ translation during growth in BSM supplemented with mannitol. The synthesis of the PhzM-LacZ protein was assessed in strain PAO1 (pME10011) by monitoring the β-galactosidase activity after growth to an OD600 of 2.0 in BSM medium supplemented with succinate (Suc) and mannitol (Man), respectively. (C) Increased phzM::lacZ translation upon ectopic expression of the crcZ gene from plasmid pMMBcrcZ. The synthesis of the PhzM-LacZ protein was assessed in strains PAO1 (pME10011; pMMB67HE) and PAO1 (pME10011; pMMBcrcZ) by monitoring the β-galactosidase activity after growth to an OD600 of 2.0 in BSM medium supplemented with succinate. The error bars represent SDs. Top panels (B,C). The steady state levels of the CrcZ RNA were determined by Northern-blot analysis when the cultures reached an OD600 of 2.0. 5S rRNA served as a loading control.
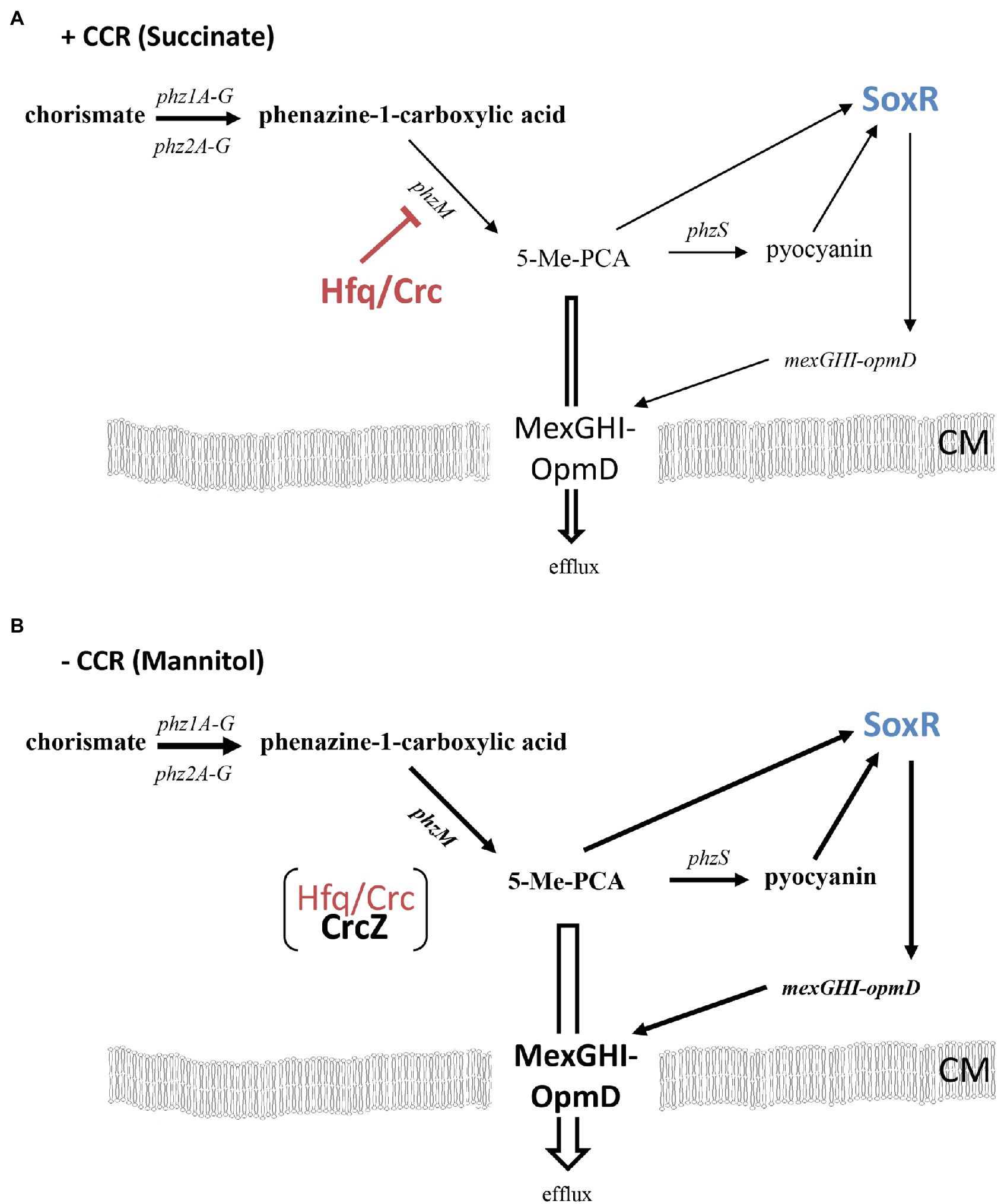
Figure 4. (A) Indirect downregulation of the mexGHI-opmD operon by Hfq during CCR (e.g., in the presence of succinate). The red bar indicates negative post-transcriptional regulation by Hfq/Crc of phzM (Sonnleitner and Bläsi, 2014), leading to moderate expression of the mexGHI-opmD operon (indicated by lean arrows and writing). (B) Increased synthesis of the mexGHI-opmD operon after relief of CCR (e.g., in the presence of mannitol). Titration of Hfq/Crc by CrcZ results in an increased synthesis of PhzM and hence in elevated levels of 5-Me-PCA and pyocyanin, which in turn results in activation of SoxR followed by transcriptional upregulation of the mexGHI-opmD operon (indicated by bold arrows and writing; Dietrich et al., 2006; Sakhtah et al., 2016). 5-Methyl-PCA efflux through MexGHI-OpmD (Sakhtah et al., 2016) protects the cells from the reactive compound. CM, cytoplasmic membrane.
Conclusion
In our experimental setup, we cannot exclude other factors such as an increase of byproducts of cellular metabolism or quorum sensing that might impact phenanzine synthesis (Lee and Zhang, 2015; Higgins et al., 2018), and thus mexGHI-opmD expression (Sakhtah et al., 2016) during diauxic growth. However, as phzM translation was de-repressed in a PAO1∆hfq deletion strain and CrcZ was shown to titrate Hfq (Sonnleitner and Bläsi, 2014), and induction of the phzM::lacZ reporter gene correlated with increased CrcZ levels (Figures 3B,C), a simple rationale for the observed upregulation of the mexGHI-opmD operon after relief of CCR is the sequestration of Hfq by the RNA CrcZ, leading to elevated synthesis of the PhzM enzyme.
We have previously shown that CrcZ-mediated titration of Hfq generally enhances the sensitivity toward antibiotics (Pusic et al., 2018). For instance, CrcZ-mediated abrogation of negative translational regulation by Hfq of the oprD and opdP porin genes results in increased carbapenem susceptibility (Sonnleitner et al., 2020). Although controlled synthesis of CrcZ can provide a means to (re)sensitize P. aeruginosa to certain antibiotics it apparently can also decrease the susceptibility to antibiotics, when relief of Hfq-mediated negative regulation results in the synthesis of metabolites, which in turn stimulate synthesis of resistance determinants, such as the mexGHI-opmD operon. Hence, distinct Hfq-dependent regulatory circuits either leading to an increased or a decreased susceptibility towards individual antibiotics should be considered when using sugars and other metabolites as adjuvants in antibiotic treatment of Pae infections (Meylan et al., 2017).
Data Availability Statement
The raw RNA-seq and Ribo-seq data were deposited in the ENA under accession number PRJEB52258.
Author Contributions
UB, MR, ES, and WW: conceived and designed the experiments. MR, EN, and ES: performed the experiments. FA, UB, MR, ES, MW, and WW: analyzed the data. All authors contributed to the article and approved the submitted version.
Funding
The work was supported by the Austrian Science Fund (www.fwf.ac.at/en) through project P28711-B22 (UB and ES). MR was supported through the FWF funded doctoral program RNA-Biology W-1207.
Conflict of Interest
The authors declare that the research was conducted in the absence of any commercial or financial relationships that could be construed as a potential conflict of interest.
Publisher’s Note
All claims expressed in this article are solely those of the authors and do not necessarily represent those of their affiliated organizations, or those of the publisher, the editors and the reviewers. Any product that may be evaluated in this article, or claim that may be made by its manufacturer, is not guaranteed or endorsed by the publisher.
Acknowledgments
We are grateful to Dr. L. Dietrich, Columbia University, New York, United States for providing materials and to Kerstin Altenburger for technical assistance.
Supplementary Material
The Supplementary Material for this article can be found online at: https://www.frontiersin.org/articles/10.3389/fmicb.2022.919539/full#supplementary-material
Footnotes
References
Abdou, L., Chou, H. T., Haas, D., and Lu, C. D. (2011). Promoter recognition and activation by the global response regulator CbrB in Pseudomonas aeruginosa. J. Bacteriol. 193, 2784–2792. doi: 10.1128/JB.00164-11
Abhyankar, W., Beek, A. T., Dekker, H., Kort, R., Brul, S., and de Koster, C. G. (2011). Gel-free proteomic identification of the Bacillus subtilis insoluble spore coat protein fraction. Proteomics 11, 4541–4550. doi: 10.1002/pmic.201100003
Aendekerk, S., Diggle, S. P., Song, Z., Hoiby, N., Cornelis, P., Williams, P., et al. (2005). The MexGHI-OpmD multidrug efflux pump controls growth, antibiotic susceptibility and virulence in Pseudomonas aeruginosa via 4-quinolone-dependent cell-to-cell communication. Microbiology 151, 1113–1125. doi: 10.1099/mic.0.27631-0
Anders, S., and Huber, W. (2010). Differential expression analysis for sequence count data. Genome Biol. 11:R106. doi: 10.1186/gb-2010-11-10-r106
Blevins, W.R., Tavella, T., Moro, S.G., Blasco-Moreno, B., Closa-Mosquera, A., Díez, J., et al. (2018). Using ribosome profiling to quantify differences in protein expression: a case study in Saccharomyces cerevisiae oxidative stress conditions. bioRxiv [Preprint]. doi: 10.1101/501478
Bolger, A. M., Lohse, M., and Usadel, B. (2014). Trimmomatic: a flexible trimmer for Illumina sequence data. Bioinformatics 30, 2114–2120. doi: 10.1093/bioinformatics/btu170
Corona, F., Reales-Calderon, J. A., Gil, C., and Martinez, J. L. (2018). The development of a new parameter for tracking post-transcriptional regulation allows the detailed map of the Pseudomonas aeruginosa Crc regulon. Sci. Rep. 8:16793. doi: 10.1038/s41598-018-34741-9
Cox, J., and Mann, M. (2008). MaxQuant enables high peptide identification rates, individualized p.p.b.-range mass accuracies and proteome-wide protein quantification. Nat. Biotechnol. 26, 1367–1372. doi: 10.1038/nbt.1511
Cox, J., Neuhauser, N., Michalski, A., Scheltema, R. A., Olsen, J. V., and Mann, M. (2011). Andromeda: a peptide search engine integrated into the MaxQuant environment. J. Proteome Res. 10, 1794–1805. doi: 10.1021/pr101065j
Dendooven, T. (2020). Fantastic Ribonucleoprotein Complexes and how to Study them. Architectural Principles of Regulatory Assemblies in bacteria. PhD thesis. Univeristy of Cambridge, UK.
Dietrich, L. E., Price-Whelan, A., Petersen, A., Whiteley, M., and Newman, D. K. (2006). The phenazine pyocyanin is a terminal signalling factor in the quorum sensing network of Pseudomonas aeruginosa. Mol. Microbiol. 61, 1308–1321. doi: 10.1111/j.1365-2958.2006.05306.x
Dolan, S. K., Kohlstedt, M., Trigg, S., Vallejo Ramirez, P., Kaminski, C. F., Wittmann, C., et al. (2020). Contextual flexibility in Pseudomonas aeruginosa central carbon metabolism during growth in single carbon sources. MBio 11, e02684–e02719. doi: 10.1128/mBio.02684-19
Ferrara, S., Carloni, S., Fulco, R., Falcone, M., Macchi, R., and Bertoni, G. (2015). Post-transcriptional regulation of the virulence-associated enzyme AlgC by the sigma(22)-dependent small RNA ErsA of Pseudomonas aeruginosa. Environ. Microbiol. 17, 199–214. doi: 10.1111/1462-2920.12590
Flood, M. E., Herbert, R. B., and Holliman, F. G. (1972). Pigments of Pseudomonas species. V. Biosynthesis of pyocyanin and the pigments of Ps. Aureoaciens. J. Chem. Soc. Perkin. 4, 622–626. doi: 10.1039/p19720000622
Fürste, J. P., Pansegrau, W., Frank, R., Blocker, H., Scholz, P., Bagdasarian, M., et al. (1986). Molecular cloning of the plasmid RP4 primase region in a multi-host-range tacP expression vector. Gene 48, 119–131. doi: 10.1016/0378-1119(86)90358-6
Furuhashi, T., Nukarinen, E., Ota, S., and Weckwerth, W. (2014). Boron nitride as desalting material in combination with phosphopeptide enrichment in shotgun proteomics. Anal. Biochem. 452, 16–18. doi: 10.1016/j.ab.2014.01.005
Gellatly, S. L., and Hancock, R. E. (2013). Pseudomonas aeruginosa: new insights into pathogenesis and host defenses. Pathog. Dis. 67, 159–173. doi: 10.1111/2049-632X.12033
Gibson, J., Sood, A., and Hogan, D. A. (2009). Pseudomonas aeruginosa-Candida albicans interactions: localization and fungal toxicity of a phenazine derivative. Appl. Environ. Microbiol. 75, 504–513. doi: 10.1128/AEM.01037-08
Greenbaum, D., Colangelo, C., Williams, K., and Gerstein, M. (2003). Comparing protein abundance and mRNA expression levels on a genomic scale. Genome Biol. 4:117. doi: 10.1186/gb-2003-4-9-117
Higgins, S., Heeb, S., Rampioni, G., Fletcher, M. P., Williams, P., and Cámara, M. (2018). Differential regulation of the phenazine biosynthetic operons by quorum sensing in Pseudomonas aeruginosa PAO1-N. Front. Cell. Infect. Microbiol. 8:252. doi: 10.3389/fcimb.2018.00252
Hoffmann, S., Otto, C., Kurtz, S., Sharma, C. M., Khaitovich, P., Vogel, J., et al. (2009). Fast mapping of short sequences with mismatches, insertions and deletions using index structures. PLoS Comput. Biol. 5:e1000502. doi: 10.1371/journal.pcbi.1000502
Holloway, B. W., Krishnapillai, V., and Morgan, A. F. (1979). Chromosomal genetics of Pseudomonas. Microbiol. Rev. 43, 73–102. doi: 10.1128/mr.43.1.73-102.1979
Huang, J., Sonnleitner, E., Ren, B., Xu, Y., and Haas, D. (2012). Catabolite repression control of pyocyanin biosynthesis at an intersection of primary and secondary metabolism in Pseudomonas aeruginosa. Appl. Environ. Microbiol. 78, 5016–5020. doi: 10.1128/AEM.00026-12
Ingolia, N. T., Ghaemmaghami, S., Newman, J. R., and Weissman, J. S. (2009). Genome-wide analysis in vivo of translation with nucleotide resolution using ribosome profiling. Science 324, 218–223. doi: 10.1126/science.1168978
Kambara, T. K., Ramsey, K. M., and Dove, S. L. (2018). Pervasive targeting of nascent transcripts by Hfq. Cell Rep. 23, 1543–1552. doi: 10.1016/j.celrep.2018.03.134
Kerr, K. G., and Snelling, A. M. (2009). Pseudomonas aeruginosa: a formidable and ever-present adversary. J. Hosp. Infect. 73, 338–344. doi: 10.1016/j.jhin.2009.04.020
Kimyon, Ö., Ulutürk, Z. I., Nizalapur, S., Lee, M., Kutty, S. K., Beckmann, S., et al. (2016). N-Acetylglucosamine inhibits LuxR, LasR and CviR based quorum sensing regulated gene expression levels. Front. Microbiol. 7:1313. doi: 10.3389/fmicb.2016.01313
La Rosa, R., Behrends, V., Williams, H. D., Bundy, J. G., and Rojo, F. (2016). Influence of the Crc regulator on the hierarchical use of carbon sources from a complete medium in Pseudomonas. Environ. Microbiol. 18, 807–818. doi: 10.1111/1462-2920.13126
La Rosa, R., Nogales, J., and Rojo, F. (2015). The Crc/CrcZ-CrcY global regulatory system helps the integration of gluconeogenic and glycolytic metabolism in Pseudomonas putida. Environ. Microbiol. 17, 3362–3378. doi: 10.1111/1462-2920.12812
Lee, J., and Zhang, L. (2015). The hierarchy quorum sensing network in Pseudomonas aeruginosa. Protein Cell 6, 26–41. doi: 10.1007/s13238-014-0100-x
Lessie, T. G., and Phibbs, P. V. Jr. (1984). Alternative pathways of carbohydrate utilization in pseudomonads. Annu. Rev. Microbiol. 38, 359–388. doi: 10.1146/annurev.mi.38.100184.002043
Linares, J. F., Moreno, R., Fajardo, A., Martinez-Solano, L., Escalante, R., Rojo, F., et al. (2010). The global regulator Crc modulates metabolism, susceptibility to antibiotics and virulence in Pseudomonas aeruginosa. Environ. Microbiol. 12, 3196–3212. doi: 10.1111/j.1462-2920.2010.02292.x
Link, T. M., Valentin-Hansen, P., and Brennan, R. G. (2009). Structure of Escherichia coli Hfq bound to polyriboadenylate RNA. Proc. Natl. Acad. Sci. U. S. A. 106, 19292–19297. doi: 10.1073/pnas.0908744106
Maier, T., Guell, M., and Serrano, L. (2009). Correlation of mRNA and protein in complex biological samples. FEBS Lett. 583, 3966–3973. doi: 10.1016/j.febslet.2009.10.036
Malecka, E. M., Bassani, F., Dendooven, T., Sonnleitner, E., Rozner, M., Albanese, T. G., et al. (2021). Stabilization of Hfq-mediated translational repression by the co-repressor Crc in Pseudomonas aeruginosa. Nucleic Acids Res. 49, 7075–7087. doi: 10.1093/nar/gkab510
Martinez, J. L., and Rojo, F. (2011). Metabolic regulation of antibiotic resistance. FEMS Microbiol. Rev. 35, 768–789. doi: 10.1111/j.1574-6976.2011.00282.x
Mavrodi, D. V., Blankenfeldt, W., and Thomashow, L. S. (2006). Phenazine compounds in fluorescent Pseudomonas spp. biosynthesis and regulation. Annu. Rev. Phytopathol. 44, 417–445. doi: 10.1146/annurev.phyto.44.013106.145710
Mayne, J., Ning, Z., Zhang, X., Starr, A. E., Chen, R., Deeke, S., et al. (2016). Bottom-up proteomics (2013-2015): keeping up in the era of systems biology. Anal. Chem. 88, 95–121. doi: 10.1021/acs.analchem.5b04230
Meylan, S., Porter, C. B. M., Yang, J. H., Belenky, P., Gutierrez, A., Lobritz, M. A., et al. (2017). Carbon sources tune antibiotic susceptibility in Pseudomonas aeruginosa via tricarboxylic acid cycle control. Cell Chem. Biol. 24, 195–206. doi: 10.1016/j.chembiol.2016.12.015
Miller, J.H. (1972). Experiments in Molecular Genetics. Cold Spring Harbour: Cold Spring Harbour Press
Milojevic, T., Grishkovskaya, I., Sonnleitner, E., Djinovic-Carugo, K., and Bläsi, U. (2013). The Pseudomonas aeruginosa catabolite repression control protein Crc is devoid of RNA binding activity. PLoS One 8:e64609. doi: 10.1371/journal.pone.0064609
Molina, L., Rosa, R., Nogales, J., and Rojo, F. (2019). Pseudomonas putida KT2440 metabolism undergoes sequential modifications during exponential growth in a complete medium as compounds are gradually consumed. Environ. Microbiol. 21, 2375–2390. doi: 10.1111/1462-2920.14622
Morales, D. K., Jacobs, N. J., Rajamani, S., Krishnamurthy, M., Cubillos-Ruiz, J. R., and Hogan, D. A. (2010). Antifungal mechanisms by which a novel Pseudomonas aeruginosa phenazine toxin kills Candida albicans in biofilms. Mol. Microbiol. 78, 1379–1392. doi: 10.1111/j.1365-2958.2010.07414.x
Moreno, R., Hernandez-Arranz, S., La Rosa, R., Yuste, L., Madhushani, A., Shingler, V., et al. (2015). The Crc and Hfq proteins of Pseudomonas putida cooperate in catabolite repression and formation of ribonucleic acid complexes with specific target motifs. Environ. Microbiol. 17, 105–118. doi: 10.1111/1462-2920.12499
O'Toole, G. A., Gibbs, K. A., Hager, P. W., Phibbs, P. V. Jr., and Kolter, R. (2000). The global carbon metabolism regulator Crc is a component of a signal transduction pathway required for biofilm development by Pseudomonas aeruginosa. J. Bacteriol. 182, 425–431. doi: 10.1128/jb.182.2.425-431.2000
Palma, M., Zurita, J., Ferreras, J. A., Worgall, S., Larone, D. H., Shi, L., et al. (2005). Pseudomonas aeruginosa SoxR does not conform to the archetypal paradigm for SoxR-dependent regulation of the bacterial oxidative stress adaptive response. Infect. Immun. 73, 2958–2966. doi: 10.1128/IAI.73.5.2958-2966.2005
Pang, Z., Raudonis, R., Glick, B. R., Lin, T. J., and Cheng, Z. (2019). Antibiotic resistance in Pseudomonas aeruginosa: mechanisms and alternative therapeutic strategies. Biotechnol. Adv. 37, 177–192. doi: 10.1016/j.biotechadv.2018.11.013
Park, H., McGill, S. L., Arnold, A. D., and Carlson, R. P. (2020). Pseudomonad reverse carbon catabolite repression, interspecies metabolite exchange, and consortial division of labor. Cell. Mol. Life Sci. 77, 395–413. doi: 10.1007/s00018-019-03377-x
Parsons, J. F., Greenhagen, B. T., Shi, K., Calabrese, K., Robinson, H., and Ladner, J. E. (2007). Structural and functional analysis of the pyocyanin biosynthetic protein PhzM from Pseudomonas aeruginosa. Biochemistry 46, 1821–1828. doi: 10.1021/bi6024403
Pei, X. Y., Dendooven, T., Sonnleitner, E., Chen, S., Bläsi, U., and Luisi, B. F. (2019). Architectural principles for Hfq/Crc-mediated regulation of gene expression. elife 8:e43158. doi: 10.7554/eLife.43158
Pusic, P., Sonnleitner, E., Krennmayr, B., Heitzinger, D. A., Wolfinger, M. T., Resch, A., et al. (2018). Harnessing metabolic regulation to increase Hfq-dependent antibiotic susceptibility in Pseudomonas aeruginosa. Front. Microbiol. 9:2709. doi: 10.3389/fmicb.2018.02709
Pusic, P., Tata, M., Wolfinger, M. T., Sonnleitner, E., Haussler, S., and Bläsi, U. (2016). Cross-regulation by CrcZ RNA controls anoxic biofilm formation in Pseudomonas aeruginosa. Sci. Rep. 6:39621. doi: 10.1038/srep39621
Quinlan, A. R., and Hall, I. M. (2010). BEDTools: a flexible suite of utilities for comparing genomic features. Bioinformatics 26, 841–842. doi: 10.1093/bioinformatics/btq033
Rojo, F. (2010). Carbon catabolite repression in Pseudomonas: optimizing metabolic versatility and interactions with the environment. FEMS Microbiol. Rev. 34, 658–684. doi: 10.1111/j.1574-6976.2010.00218.x
Sakhtah, H., Koyama, L., Zhang, Y., Morales, D. K., Fields, B. L., Price-Whelan, A., et al. (2016). The Pseudomonas aeruginosa efflux pump MexGHI-OpmD transports a natural phenazine that controls gene expression and biofilm development. Proc. Natl. Acad. Sci. U. S. A. 113, E3538–E3547. doi: 10.1073/pnas.1600424113
Santiago-Frangos, A., and Woodson, S. A. (2018). Hfq chaperone brings speed dating to bacterial sRNA. Wiley Interdiscip. Rev. RNA 9:e1475. doi: 10.1002/wrna.1475
Sekiya, H., Mima, T., Morita, Y., Kuroda, T., Mizushima, T., and Tsuchiya, T. (2003). Functional cloning and characterization of a multidrug efflux pump, mexHI-opmD, from a Pseudomonas aeruginosa mutant. Antimicrob. Agents Chemother. 47, 2990–2992. doi: 10.1128/AAC.47.9.2990-2992.2003
Sonnleitner, E., Abdou, L., and Haas, D. (2009). Small RNA as global regulator of carbon catabolite repression in Pseudomonas aeruginosa. Proc. Natl. Acad. Sci. U. S. A. 106, 21866–21871. doi: 10.1073/pnas.pnas.0910308106
Sonnleitner, E., and Bläsi, U. (2014). Regulation of Hfq by the RNA CrcZ in Pseudomonas aeruginosa carbon catabolite repression. PLoS Genet. 10:e1004440. doi: 10.1371/journal.pgen.1004440
Sonnleitner, E., Hagens, S., Rosenau, F., Wilhelm, S., Habel, A., Jäger, K. E., et al. (2003). Reduced virulence of a hfq mutant of Pseudomonas aeruginosa O1. Microb. Pathog. 35, 217–228. doi: 10.1016/s0882-4010(03)00149-9
Sonnleitner, E., Prindl, K., and Bläsi, U. (2017). The Pseudomonas aeruginosa CrcZ RNA interferes with Hfq-mediated riboregulation. PLoS One 12:e0180887. doi: 10.1371/journal.pone.0180887
Sonnleitner, E., Pusic, P., Wolfinger, M. T., and Bläsi, U. (2020). Distinctive regulation of Carbapenem susceptibility in Pseudomonas aeruginosa by Hfq. Front. Microbiol. 11:1001. doi: 10.3389/fmicb.2020.01001
Sonnleitner, E., Schuster, M., Sorger-Domenigg, T., Greenberg, E. P., and Bläsi, U. (2006). Hfq-dependent alterations of the transcriptome profile and effects on quorum sensing in Pseudomonas aeruginosa. Mol. Microbiol. 59, 1542–1558. doi: 10.1111/j.1365-2958.2006.05032.x
Sonnleitner, E., Sorger-Domenigg, T., and Bläsi, U. (2012). “Pseudomonas aeruginosa small regulatory RNAs,” in Regulatory RNAs in Prokaryotes. eds. W. R. Hess and A. Marchfelder (Vienna: Springer), 77–94.
Sonnleitner, E., Wulf, A., Campagne, S., Pei, X. Y., Wolfinger, M. T., Forlani, G., et al. (2018). Interplay between the catabolite repression control protein Crc, Hfq and RNA in Hfq-dependent translational regulation in Pseudomonas aeruginosa. Nucleic Acids Res. 46, 1470–1485. doi: 10.1093/nar/gkx1245
Temple, L. M., Sage, A. E., Schweizer, H. P., and Phibbs, P. V. (1998). “Carbohydrate catabolism in Pseudomonas aeruginosa,” in Pseudomonas. ed. T. C. Montie (Boston, MA: Springer US), 35–72.
Valentini, M., Garcia-Maurino, S. M., Perez-Martinez, I., Santero, E., Canosa, I., and Lapouge, K. (2014). Hierarchical management of carbon sources is regulated similarly by the CbrA/B systems in Pseudomonas aeruginosa and Pseudomonas putida. Microbiology 160, 2243–2252. doi: 10.1099/mic.0.078873-0
Wang, G., Huang, X., Li, S., Huang, J., Wei, X., Li, Y., et al. (2012). The RNA chaperone Hfq regulates antibiotic biosynthesis in the rhizobacterium Pseudomonas aeruginosa M18. J. Bacteriol. 194, 2443–2457. doi: 10.1128/JB.00029-12
Williams, B. J., Dehnbostel, J., and Blackwell, T. S. (2010). Pseudomonas aeruginosa: host defence in lung diseases. Respirology 15, 1037–1056. doi: 10.1111/j.1440-1843.2010.01819.x
Winsor, G. L., Griffiths, E. J., Lo, R., Dhillon, B. K., Shay, J. A., and Brinkman, F. S. (2016). Enhanced annotations and features for comparing thousands of Pseudomonas genomes in the Pseudomonas genome database. Nucleic Acids Res. 44, D646–D653. doi: 10.1093/nar/gkv1227
Wolfinger, M. T., Fallmann, J., Eggenhofer, F., and Amman, F. (2015). ViennaNGS: A toolbox for building efficient next-generation sequencing analysis pipelines. F1000Res 4:50. doi: 10.12688/f1000research.6157.2
Keywords: Pseudomonas aeruginosa, Hfq, CRC, carbon catabolite repression, multiomics, norfloxacin, phenazines, MexGHI-OpmD efflux pump
Citation: Rozner M, Nukarinen E, Wolfinger MT, Amman F, Weckwerth W, Bläsi U and Sonnleitner E (2022) Rewiring of Gene Expression in Pseudomonas aeruginosa During Diauxic Growth Reveals an Indirect Regulation of the MexGHI-OpmD Efflux Pump by Hfq. Front. Microbiol. 13:919539. doi: 10.3389/fmicb.2022.919539
Edited by:
Lin Zeng, University of Florida, United StatesReviewed by:
Weihui Wu, Nankai University, ChinaZachary Daniel Moye, University of Florida, United States
Copyright © 2022 Rozner, Nukarinen, Wolfinger, Amman, Weckwerth, Bläsi and Sonnleitner. This is an open-access article distributed under the terms of the Creative Commons Attribution License (CC BY). The use, distribution or reproduction in other forums is permitted, provided the original author(s) and the copyright owner(s) are credited and that the original publication in this journal is cited, in accordance with accepted academic practice. No use, distribution or reproduction is permitted which does not comply with these terms.
*Correspondence: Udo Bläsi, udo.blaesi@univie.ac.at; Elisabeth Sonnleitner, elisabeth.sonnleitner@univie.ac.at