- 1Federal Rural University of Amazonia (UFRA), Belém, Brazil
- 2Graduate Program in Biodiversity and Biotechnology (BIONORTE), Federal University of Tocantins (UFT), Palmas, Brazil
- 3Graduate Program in Biotechnology and Environmental Monitoring, Federal University of São Carlos (UFSCar), Sorocaba, Brazil
- 4Cell and Molecular Biology Laboratory, Center for Nuclear Energy in Agriculture, University of São Paulo (USP), Piracicaba, Brazil
- 5Princeton Institute for International and Regional Studies, Princeton University, Princeton, NJ, United States
- 6Graduate Program in Agricultural Microbiology, Federal University of Viçosa, Viçosa, Brazil
- 7Graduate Program in Environmental Sciences, University Brazil, Fernandópolis, Brazil
- 8Department of Physics, Chemistry, and Mathematics, Federal University of São Carlos (UFSCar), Sorocaba, Brazil
- 9Department of Microbial Ecology, Netherlands Institute of Ecology (NIOO-KNAW), Wageningen, Netherlands
Ammonia oxidation is the rate-limiting first step of nitrification and a key process in the nitrogen cycle that results in the formation of nitrite (NO2–), which can be further oxidized to nitrate (NO3–). In the Amazonian floodplains, soils are subjected to extended seasons of flooding during the rainy season, in which they can become anoxic and produce a significant amount of methane (CH4). Various microorganisms in this anoxic environment can couple the reduction of different ions, such as NO2– and NO3–, with the oxidation of CH4 for energy production and effectively link the carbon and nitrogen cycle. Here, we addressed the composition of ammonium (NH4+) and NO3–—and NO2–—dependent CH4-oxidizing microbial communities in an Amazonian floodplain. In addition, we analyzed the influence of environmental and geochemical factors on these microbial communities. Soil samples were collected from different layers of forest and agroforest land-use systems during the flood and non-flood seasons in the floodplain of the Tocantins River, and next-generation sequencing of archaeal and bacterial 16S rRNA amplicons was performed, coupled with chemical characterization of the soils. We found that ammonia-oxidizing archaea (AOA) were more abundant than ammonia-oxidizing bacteria (AOB) during both flood and non-flood seasons. Nitrogen-dependent anaerobic methane oxidizers (N-DAMO) from both the archaeal and bacterial domains were also found in both seasons, with higher abundance in the flood season. The different seasons, land uses, and depths analyzed had a significant influence on the soil chemical factors and also affected the abundance and composition of AOA, AOB, and N-DAMO. During the flood season, there was a significant correlation between ammonia oxidizers and N-DAMO, indicating the possible role of these oxidizers in providing oxidized nitrogen species for methanotrophy under anaerobic conditions, which is essential for nitrogen removal in these soils.
Introduction
Methane (CH4) is a greenhouse gas with approximately 34 times greater global warming potential than carbon dioxide (CO2) over 100 years, and since 1750, atmospheric levels of CH4 have increased by 157% (Myhre et al., 2013; World Meteorological Organization [WMO], 2021). Some of the largest natural sources of CH4 are wetlands, which account for 175–217 Tg CH4 yr–1 (Ciais et al., 2009; Kirschke et al., 2013), which is approximately 30% of the total emissions (Bloom et al., 2010, 2012). Tropical and subtropical wetlands are important sources of CH4 because of their elevated net primary productivity and high temperatures, producing 50–60% of all wetland CH4 emissions (Walter et al., 2001; Bloom et al., 2010, 2012).
Methane is the net result of the production and consumption of methane by methanogenic and methanotrophic microorganisms, respectively. This balance can be shifted by the flood pulse that changes the soil water saturation and favors methanogenic or methanotrophic activity depending on climatic conditions, land use, and anthropogenic activities (Steudler et al., 1996; Dalal and Allen, 2008; Meyer et al., 2017). When the soil is flooded, anoxic conditions induce CH4 production by methanogenic microorganisms (Agostinetto et al., 2002). However, under aerobic and anaerobic conditions, CH4 can be oxidized to CO2 by methanotrophic microorganisms, which act as the primary biological sinks of this greenhouse gas (Knittel and Boetius, 2009).
Anaerobic oxidation of methane is an important sink of this atmospheric gas (Conrad, 2009), as it plays an essential role in global warming mitigation. AOM was first found to be coupled with sulfate reduction and mediated by anaerobic methanotrophic archaea (ANME) and sulfate-reducing bacteria (SRB) (Boetius et al., 2000; Cui et al., 2015). Currently, AOM has been observed to be coupled with different terminal electron acceptors, such as nitrate (NO3–) (Haroon et al., 2013), nitrite (NO2–) (Ettwig et al., 2010), and other metals, including iron and manganese (Beal et al., 2009; Gabriel et al., 2020), which effectively link nitrogen and/or metal cycles to CH4 consumption.
Nitrate/nitrite-dependent anaerobic methane oxidation (N-DAMO) is mediated by the members of the bacterial and archaeal domains. Nitrite-dependent AOM is mediated by Candidatus Methylomirabilis oxyfera, a member of the NC10 bacterial phylum (Raghoebarsing et al., 2006; Ettwig et al., 2009), while nitrate-dependent AOM is catalyzed by anaerobic archaea belonging to the ANME-2d clade, Candidatus Methanoperedens nitroreducens (Haroon et al., 2013). The latter uses a reverse methanogenesis pathway for CH4 oxidation, with methyl-coenzyme M (methyl-CoM) reductase as the key enzyme, followed by NO3– reduction to NO2– (Haroon et al., 2013; Arshad et al., 2015; Ettwig et al., 2016).
A study in the Amazonian floodplains revealed a disproportionate role of the NC10 phylum in CH4 mitigation across soil layers, forest, and agricultural sites, with seasonal flooding in the different water types of the floodplains and the presence of Candidatus M. nitroreducens in the clear water floodplain of the Tocantins River (Bento et al., 2021). The nitrate-reducing archaeon Candidatus M. nitroreducens can not only reduce NO3– to NO2– but can also perform dissimilatory nitrate reduction to ammonium (DNRA) (Ettwig et al., 2016). It also shows syntrophic relationships with anammox (anaerobic ammonium oxidation) bacteria and Candidatus M. oxyfera depending on the substrate availability (Haroon et al., 2013).
In this study, we revealed the composition of active ammonia-oxidizing and nitrogen-dependent anaerobic methane-oxidizing communities using high-throughput sequencing of 16S rRNA transcript amplicons recovered from flooded and non-flooded forest and agroforest soils in an Amazonian clear water river floodplain. We hypothesized that (i) soil chemical factors, depth, land use, and seasonality modulate the active AOA and AOB communities and the occurrence of nitrogen-dependent anaerobic methane oxidizers in these soils, and (ii) increases in AOA and AOB abundance are coupled to an increase in abundance of nitrogen-dependent anaerobic methane oxidizers in flooded and non-flooded soils.
Materials and methods
Site description and soil sampling
Sampling sites were identified as primary forests (i.e., one forest site with no visible indications of human activities or significant disturbance of ecological processes) or traditional farming systems (i.e., one traditional cocoa-based agroforestry site) and characterized using: (1) Shuttle Radar Topography Mission (SRTM) satellite images at a resolution of 1 arc-sec (30 m), (2) Landsat 8 satellite data, (3) Google Earth images with Universal Transverse Mercator (UTM) projection, (4) SIRGAS referencing (UTM zone 22S to 20S), (5) field visits, and (6) QGIS v.2.18 “Las Palmas” geoprocessing tools. These sites are located in the lower Tocantins basin, municipality of Baião (2°40′51″ S, 49°39′05″ W) in the state of Pará, Brazil, in the hydrographic basin of the Tocantins River. This basin extends from 46° to 55°W and 2° to 18°S, with a total drainage area of 918 822 km2, and this represents 11% of the total Brazilian territory (Agência Nacional de Águas [ANA], 2015). Both sites are in an area that is seasonally flooded with clear water from the Tocantins River.
The study area is downstream of the Tucuruí Hydroelectric Power Plant reservoir, the largest Brazilian hydropower dam, with a flooded area of 2,850 km2 (Agência Nacional de Águas [ANA], 2009). Both sites are in an area seasonally flooded with clear water from the Tocantins River. After the construction of the Tucuruí dam, a reduction was recorded in the extension of the areas flooded by the river bed downstream, as a consequence of the regulation of the flow caused by the damming of the natural course of the river (Junk and Mello, 1987). The lower Tocantins River has a semi-diurnal tidal effect that alters river hydrodynamics (De Mérona et al., 2010). These tidal effects increase the water’s residence time and connection between the riparian zone, floodplains, and the main river channel. The topography of the basin is dominated by gently sloping upland plateaus, and the soils consist of Oxisols (24%), Ultissol (17%), Entisols (23%), and Entisol Plinthic (14%) (Agência Nacional de Águas [ANA], 2009).
The climate in this basin is tropical (hot and humid), with strong seasonality (Gomes et al., 2019). The annual average temperature is 26°C and precipitation is 2,533 mm (Agência Nacional de Águas [ANA], 2009). According to the Köppen climate classification, four distinct climate types are identified in the basin: Af in the northern region (tropical climate without dry season), Am in the northwest (with a moderate drought season between July and September), and Aw in the northwest, central-west, and east regions, which have an annual rainfall of approximately 1,700 mm, with a dry season between June and August. The last climate type is Cwa, humid subtropical with a dry winter and hot summer, which corresponds to the savanna area (Alvares et al., 2013).
In the primary forest and the adjacent traditional cocoa-based agroforest, soil samples were collected at three points that were a minimum of 14 m apart along a transect. Undeformed soil cores were taken from 0 to 30 cm topsoil layer in the dry (October 2017) and flood (May 2018) regimes of the floodplains. During the dry season, soil samples were collected in an aseptic cylindrical core (0–30 cm deep and 10 cm diameter) with a threaded partition at 15 cm (Supplementary Figure 1A). During the flood season, underwater soil cores (0–30 cm deep and 10 cm diameter) were collected using a universal hand core sediment sampler with a 0–15 cm adjustable core cutter (Aquatic Research Instruments, ID, United States) (Supplementary Figure 1B).
Soil cores collected in both the dry and flood regimes of the floodplains were sliced into two 15 cm layers (0–15 cm depth and 15–30 cm depth), totaling 24 soil samples (two soil layers × three sampling points × two land uses × two seasons). A subsample containing 3 g of soil was collected from each of the two 15 cm soil layers per point, immediately preserved in LifeGuard Soil Preservation Solution (Qiagen, Hilden, Germany), transported to the laboratory on ice, and stored at −80°C until further processing within 72 h after sampling. The remaining soil from each layer was used for the physicochemical analysis.
Soil chemical factor measurement and analysis
Chemical measurements were performed on each of the 24 soil samples. Soil pH was measured using a soil suspension in 0.01 M CaCl2 (1:5, w/v). Soil moisture was determined using the gravimetric method described by Silva (2009), dissolved organic carbon (DOC) content using a Shimadzu TOC-5000A analyzer (Shimadzu, Columbia, MD, United States), and total carbon (C) and nitrogen (N) contents by dry combustion using a CHNS/O elemental analyzer (PerkinElmer, Waltham, MA, United States) from the soil samples. The analyses were performed using 5–7 mg of dry soil sieved through a 0.15-mm mesh. Sulfate was extracted and determined according to Cantarella and Prochnow (2001). Soil ammonium and nitrate were extracted using 2 M potassium chloride (KCl) and quantified by spectrophotometry as described by Krom (1980) and Norman et al. (1985), respectively.
Soil RNA isolation, cDNA synthesis, and 16S rRNA gene amplicon sequencing
Total RNA was isolated from 2 g of soil (wet weight) of each of the 24 soil samples using the RNeasy PowerSoil Total RNA Kit (Qiagen) according to the manufacturer’s instructions. RNA concentration and purity were assessed spectrophotometrically (Nanodrop ND-1000, Nanodrop Technologies, Inc., Wilmington, DE, United States) to determine the absorbance at 230, 260, 280, and 320 nm. The concentration of isolated RNA was 100–200 ng μL–1. RNA extraction was performed in duplicate for each sample (two technical replicates) and stored at −80°C until further use.
Complementary DNA (cDNA) was synthesized from single-stranded RNA using a QuantiNova Reverse Transcription Kit (Qiagen) with integrated removal of genomic DNA contamination following the manufacturer’s instructions.
Amplicon libraries were prepared using 16S Metagenomic Sequencing Library Preparation,1 with cDNA as a template in the amplification reactions and specific primers for the archaeal and bacterial 16S ribosomal RNA (rRNA) genes. The primer pair SD-Arch-0349-aS-17 (5′-GYGCASCAGKCGMGAAW-3′)/SD-Arch-0519-aA-16 (5′-TACCGCGGCKGCTG-3′) (Klindworth et al., 2013) was used to obtain amplicons of approximately 185 bp from the V3 region of the archaeal 16S rRNA gene. A fragment of approximately 390 bp of the V4 region of the bacterial 16S rRNA gene was amplified using the primers 515F (5′-GTGYCAGCMGCCGCGGTAA-3′) (Parada et al., 2016) and 806R (5′-GGACTACNVGGGTWTCTAA-3′) (Apprill et al., 2015).
Each reaction contained 2.5 μL of 10 × reaction buffer, 1 μL of MgCl2 (50 mM), 1 μL of dNTP (10 mM), 1 μL of bovine serum albumin (BSA; 1 mg mL–1), 0.5 μL of each primer (10 μM), 10 ng of template cDNA, 0.5 μL of Platinum Taq DNA Polymerase (5 U μL–1) (Thermo Fisher Scientific, Waltham, MA, United States), and sterile water to obtain a final volume of 25 μL. The thermocycling conditions were as follows: initial denaturation for 3 min at 95°C, 30 cycles of 30 s at 95°C, 30 s at 58°C for archaea (60°C for bacteria), 30 s at 72°C, and a final extension for 10 min at 72°C. All primers contained the Illumina adapter sequences. PCR products were purified using Agencourt AMPure XP beads (Beckman Coulter, Inc., Brea, CA, United States) and resuspended in nuclease-free water.
Amplicons were quantified using the 1000 Assay of the Agilent 2100 Bioanalyzer System (Agilent Technologies, Santa Clara, CA, United States). Amplicons from the 24 samples were indexed in a PCR reaction with 20 cycles using the Nextera XT Index Kit (Illumina, San Diego, CA, United States) and KAPA HiFi HotStart ReadyMix (Roche, Pleasanton, CA, United States). The 24 multiplexed samples were pooled at equal volumes by library, which was performed separately for archaea and bacteria. The pooled libraries were purified using HighPrep™ PCR Magnetic Beads (MagBio Genomics, Gaithersburg, MD, United States) and subsequently assayed on an Agilent 2100 Bioanalyzer System (Agilent Technologies) to estimate library size. Libraries were quantified using the Qubit™ dsDNA HS (High Sensitivity) Assay Kit on a Qubit 2.0 fluorometer (Life Technologies, Carlsbad, CA, United States) and KAPA SYBR® FAST qPCR Master Mix and Illumina standards and primer premix (KAPA Biosystems, Wilmington, MA, United States), according to the Illumina suggested protocol.
The resulting 16S rRNA amplicon libraries were denatured with 10 μL of NaOH, diluted to 8 pM with the Illumina HT1 buffer, and spiked with 1 % PhiX (Illumina). Equal concentrations of libraries were loaded independently for the 16S rRNA amplicons of archaea and bacteria using the MiSeq Reagent Kit v3 (Illumina). The equipment used for 16S rRNA amplicon sequencing was a MiSeq Personal Sequencing System (Illumina) operated in a rapid run mode to generate 2 × 300 bp paired-end reads.
16S rRNA amplicon sequence data preprocessing and taxonomic determination
All 16S rRNA gene sequence reads were processed and analyzed using QIIME v.1.9.1 (Quantitative Insights into Microbial Ecology) software (Caporaso et al., 2010a). Briefly, fastq files with forward and reverse reads were merged using the UPARSE algorithm (Edgar, 2013). Sequences that did not merge with this algorithm were merged using VSEARCH v.2.10.4, with 2 bp as the minimum length for overlap. The merged reads were further preprocessed by (i) quality control of the consensus sequences using a Phred quality score of 20 for each base call (Ewing and Green, 1998), (ii) removal of artificial sequences, such as primers and adapters, (iii) disposal of short length reads with less than 100 bp (archaea) and 200 bp (bacteria), and (iv) removal of ambiguous sequences. USEARCH (Edgar, 2010) was then employed to remove chimeras from the preprocessed reads. After chimera removal, the preprocessed reads were aligned using PyNAST (Caporaso et al., 2010b) with SILVA Release 1322 and sorted with >97% similarity into operational taxonomic units (OTUs) using a closed-reference OTU picking approach.
16S rRNA amplicon sequence data analysis
To reveal the active nitrogen-dependent anaerobic methane-oxidizing (N-DAMO) and ammonia-oxidizing microorganisms, the number of 16S amplicon sequences in each library was normalized using the OTU table (2,500 sequences for archaea and 20,000 sequences for bacteria) and the rarefaction method of QIIME v.1.9.1. The OTU tables obtained for the 16S rRNA gene sequences of archaea and bacteria were used separately to filter Candidatus Methanoperedens nitroreducens and Candidatus Methylomirabilis oxyfera clusters, as well as the archaeal and bacterial ammonia-oxidizing communities using the “filter_taxa_from_OTU_table.py” command of QIIME v.1.9.1 and the script described by Pylro et al. (2014). The relative abundances of active ammonia oxidizers and N-DAMO taxa were estimated by dividing the number of sequences belonging to each by the total number classified as ammonia oxidizers or N-DAMO per sample.
Statistical analyses
Statistical analyses were conducted using PAST software (Hammer et al., 2001) and R studio 4.0.5 (RStudio Team, 2018). Statistical analysis and graphical visualization in the R studio were performed using “vegan” 2.5-7 (Oksanen et al., 2022), “ggpubr” 0.4.0 (Kassambara, 2020), and “ggplot2” 3.3.5 (Wickham and Chang, 2016). Tukey’s HSD post-hoc analysis was used to determine the significance of the differences between the non-flood and flood seasons of the floodplain, and between the forest (FOR) and agroforest (TFS) for each soil factor within each soil layer (0–15 cm and 15–30 cm). The similarity between samples regarding their soil chemical factors and ammonia-oxidizing and nitrogen-dependent anaerobic methane-oxidizing community composition was assessed using nonmetric multidimensional scaling (NMDS) and permutational multivariate analysis of variance (PERMANOVA), using Euclidean distance for the chemical attributes and Bray-Curtis distance for community composition. Further evaluation of the contribution of land use, soil depth, and seasonality to the total variation in ammonia-oxidizing and nitrogen-dependent anaerobic methane oxidizer communities was determined through variance partitioning analysis (Borcard et al., 2018). The significance of each chemical factor was assessed using PERMANOVA. Pearson’s correlation was used to confirm the relationship between ammonia-oxidizing and nitrogen-dependent anaerobic methane oxidizers.
Results
Soil chemical profile
Lower pH values (p < 0.05) were observed in the FOR site compared to the TFS site in both flood and non-flood seasons, whereas sulfate and the C:N ratio were significantly higher at the FOR site, primarily during the non-flood season. Other chemical factors varied across seasons, sites, and depths (Table 1). Nonmetric multidimensional scaling-based multivariate analysis indicated that the contrasting seasons (R2 = 0.30, p = 0.001), sites (R2 = 0.10, p = 0.001), and depths (R2 = 0.23, p = 0.001) had highly different chemical profiles. The interaction between depth and season and between depth and sites were also significant (R2 = 0.095 and 0.055; p = 0.004 and 0.021, respectively), although only a small portion of the variance was explained (Figure 1 and Table 2).
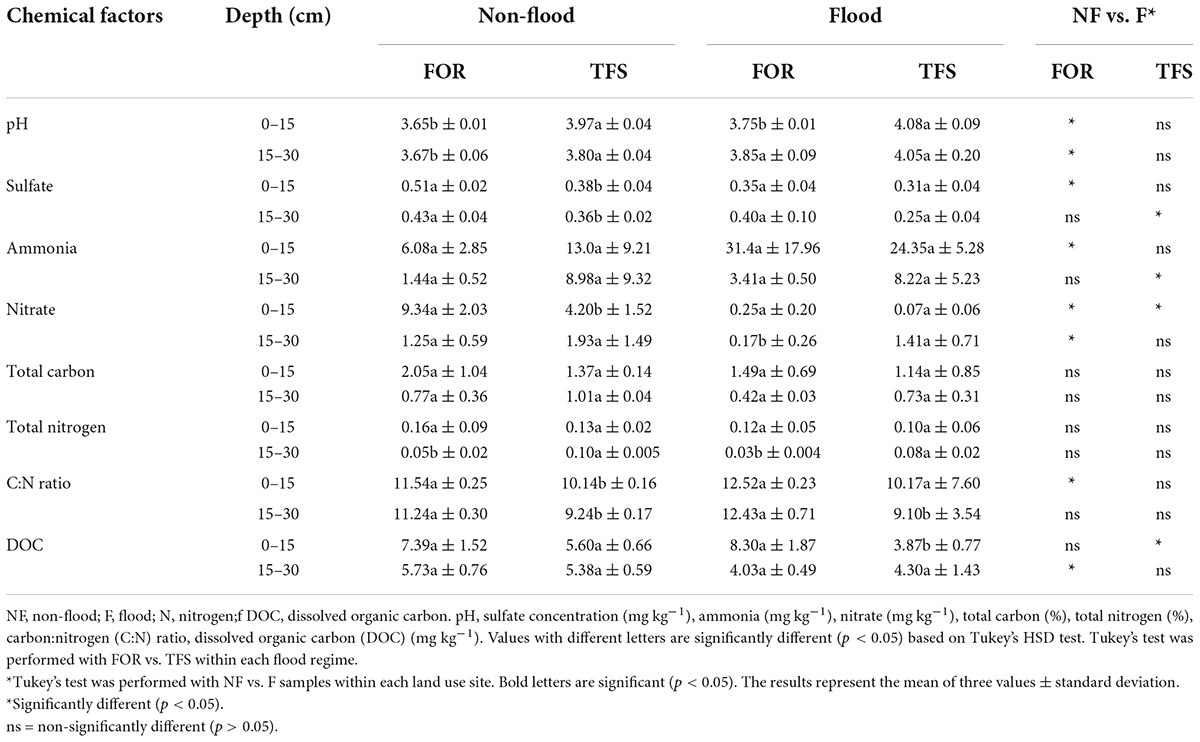
Table 1. Chemical attributes of the soil samples at each depth (0–15 and 15–30 cm), site [Forest (FOR) and Agroforest (TFS)], and season [Non-flood (NF) and Flood (F)] in the Tocantins River plain.
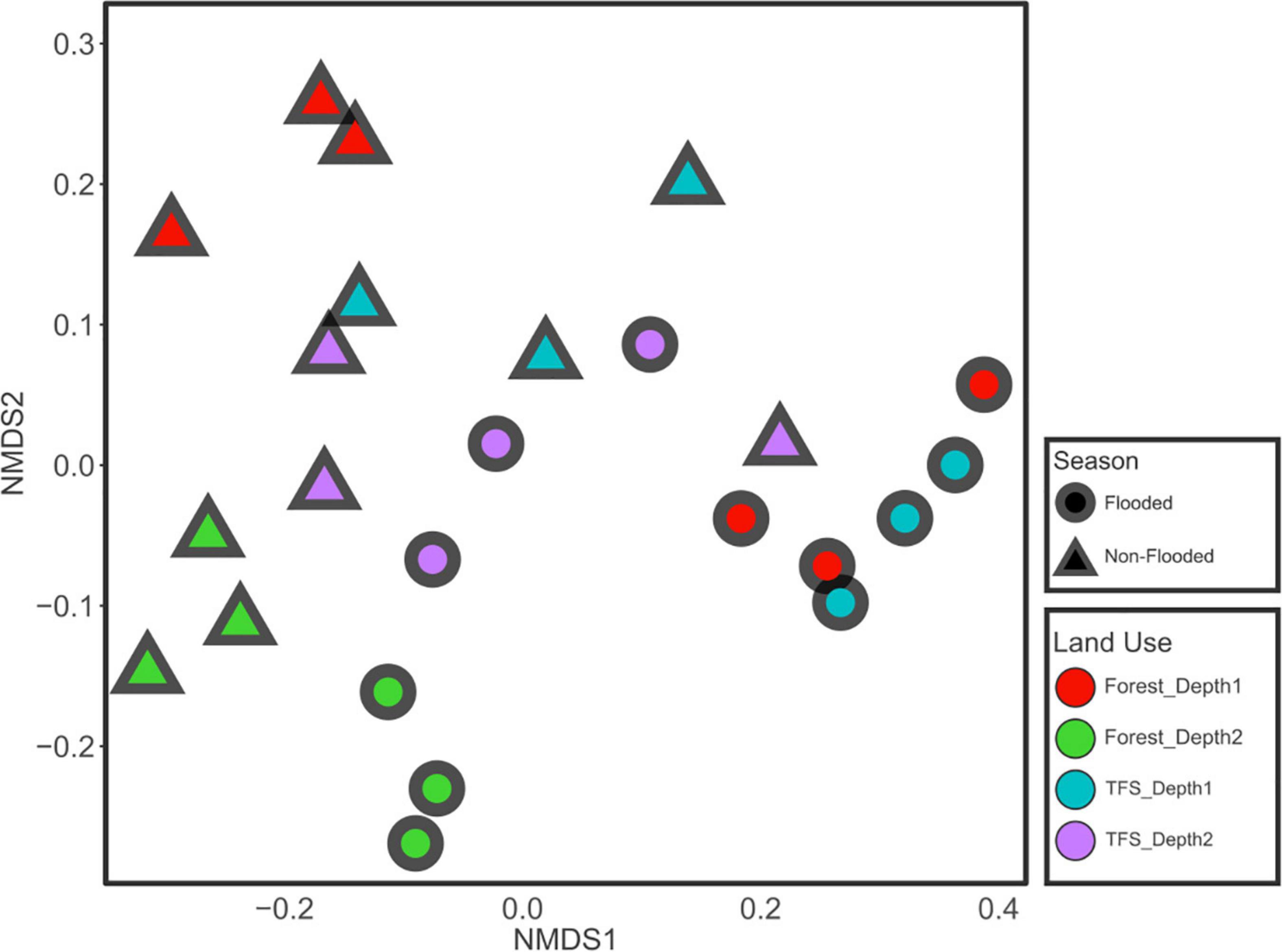
Figure 1. Clustering of soil chemical properties during different seasons (flood and non-flood) and different combinations of sites and depths. The plot is based on nonmetric multidimensional scaling (NMDS) using the Euclidean distance index.
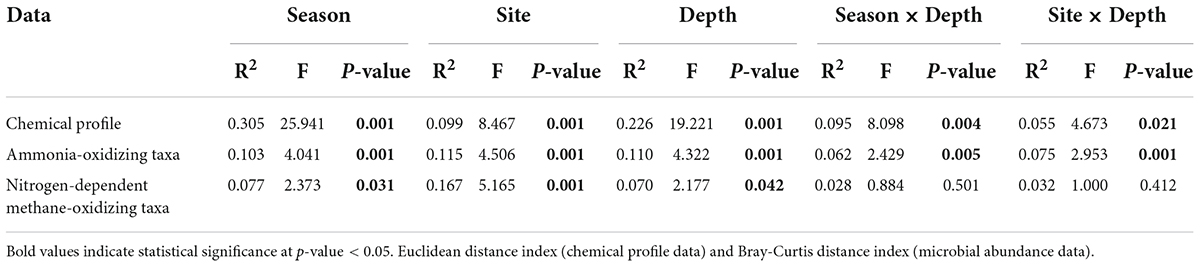
Table 2. Permutational multivariate analysis of variance of the chemical properties and the microbial community profiles in the floodplain of the Tocantins River.
Ammonia-oxidizing microbial community
Analysis of the soil layers [0–15 cm (D1) and 15–30 cm (D2)] in the FOR and TFS sites in the flood and non-flood seasons revealed the active presence of both ammonia-oxidizing archaea (AOA) and bacteria (AOB). The AOA community was composed of two distinct groups belonging to the archaeal phylum Thaumarchaeota, genus Nitrososphaera and genus Nitrosotaleacea, while the AOB community was composed of the members of the Nitrosomonas genus (Figure 2B). The archaeal and bacterial community composition is shown in the Supplementary Tables 1, 2.
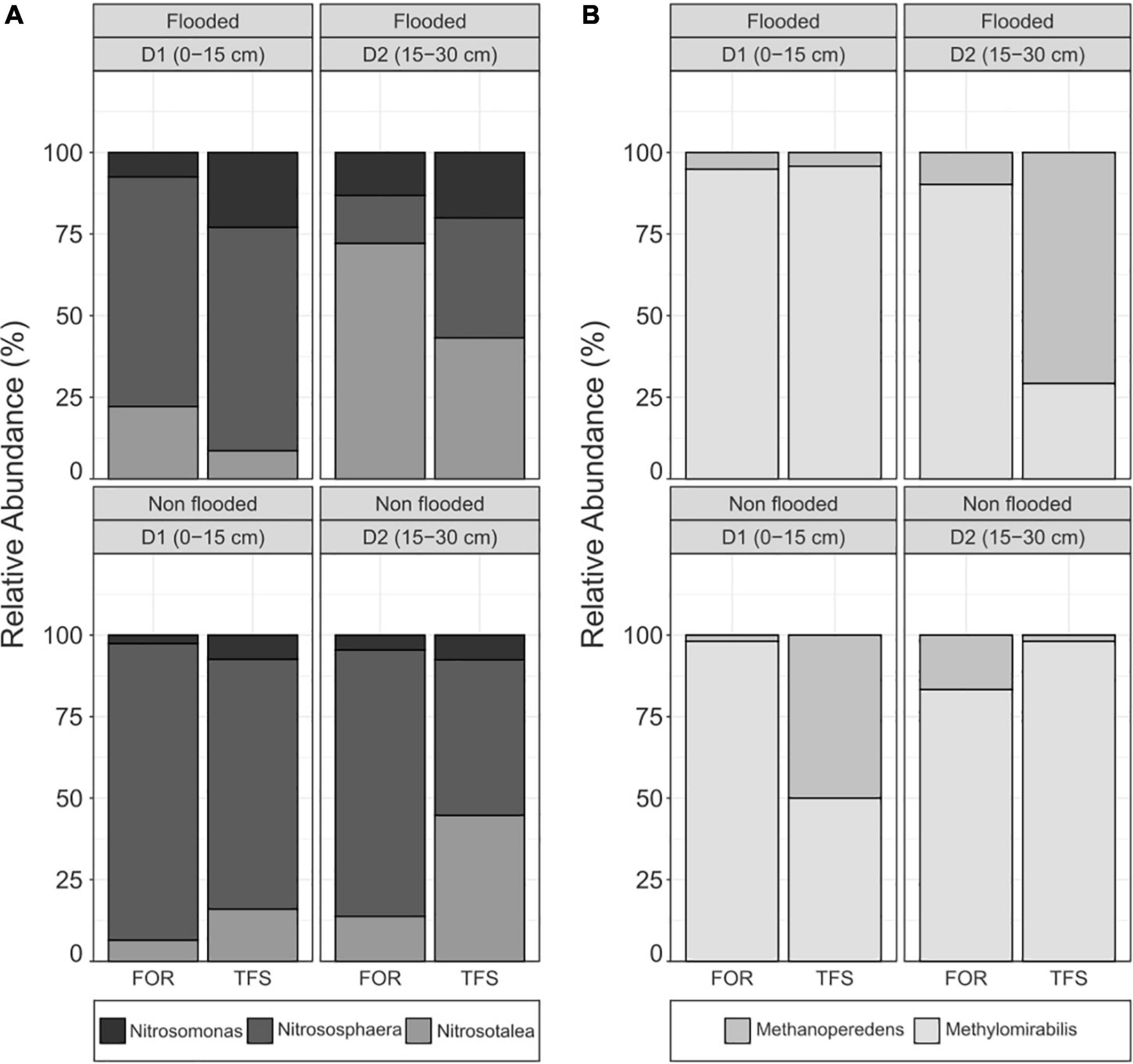
Figure 2. Relative abundance of (A) active ammonia-oxidizing genera and (B) active nitrogen-dependent anaerobic methane-oxidizing genera in each depth (0–15 and 15–30 cm), and season [Non-flood (NF) and Flood (F)], for contrasting land uses [Agroforest (TFS) and Forest (FOR)] in the Tocantins River plain.
The overall ammonia-oxidizing microbial community was mainly composed of AOA members, which presented a clear dominance over AOB in terms of absolute and relative abundances (Table 3 and Figure 2A). While highly dominant in every season, site, and depth, AOA was relatively more abundant during the non-flood season, where all sites had >90% of the ammonia-oxidizing community composed of AOA, with maximum relative abundance in D1 of the FOR site (97.46%). Unlike the AOA community, the AOB community displayed a higher relative abundance during the flood season, reaching up to 20% relative abundance at both depths of the TFS site, in contrast to the 7.36 and 7.58% abundance of AOB in the same site in the non-flood season for D1 and D2, respectively (Figure 2A).
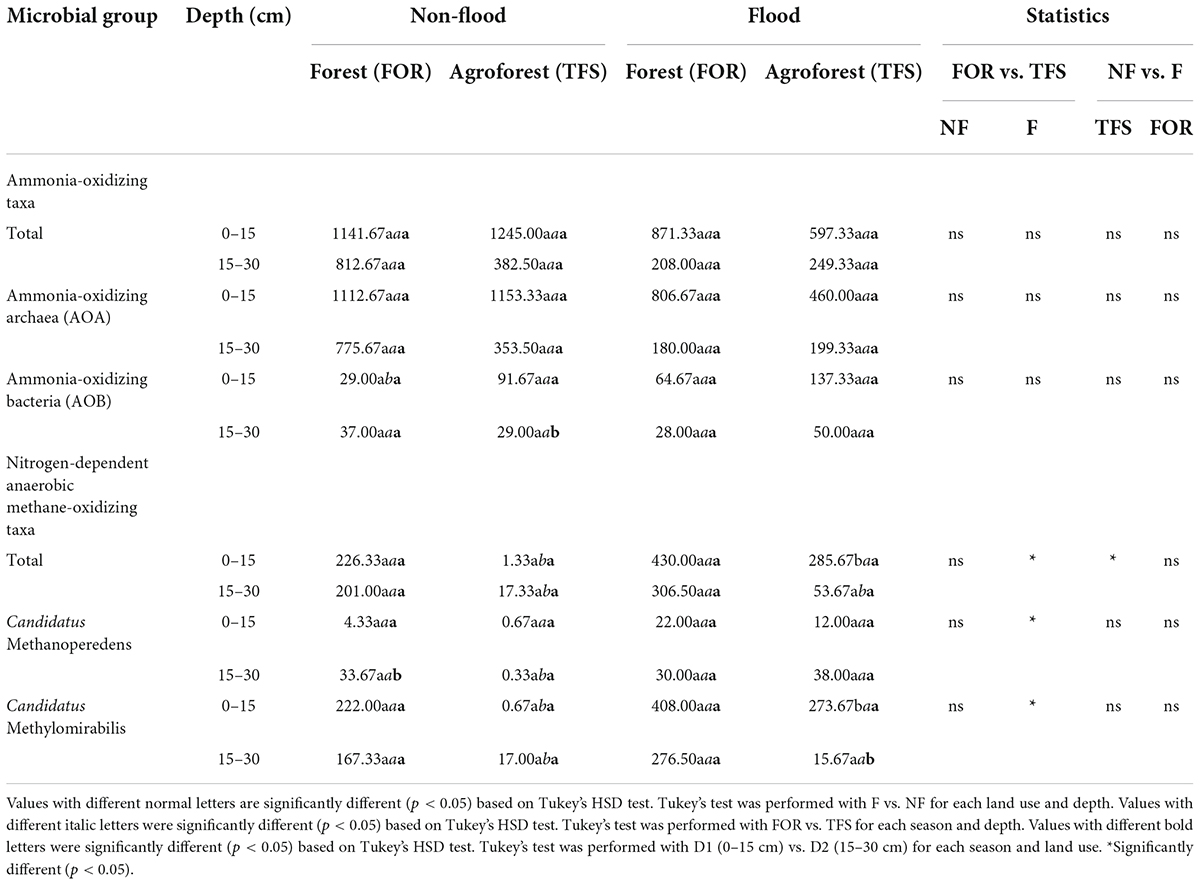
Table 3. Absolute sequence abundance of active ammonia-oxidizing and anaerobic methane-oxidizing bacteria and archaea in each depth, site, and season in the Tocantins River plain based on 16S rRNA gene amplicon sequencing data.
Variance in AOB community composition was mainly affected by the interrelation between edaphoclimatic and soil chemical factors. The interaction between land use and soil chemical attributes had the highest influence on the variance (11.718%), whereas depth (7.273%), seasonality in combination with soil chemical attributes (3.545%), and seasonality in combination with land use (3.377%) also exhibited high contributions. The intersection between all four factors (0.360%) and between all three edaphic factors (0.122%) had the lowest values (Figure 3B). The majority of the AOB variance was considered residual, which corresponded to unaccounted factors (87.107%). Different variables were grouped to form the “soil chemical factors,” which contributed largely to AOB variance, and to better evaluate the impacts of these individual variables on the variance of AOB, a permutation analysis of variance (PERMANOVA) of each component of the soil chemical attributes was performed. The PERMANOVA analysis indicated that pH had the highest influence on the variance in AOB community composition within the pool of chemical variables, along with other factors (Figure 3B).
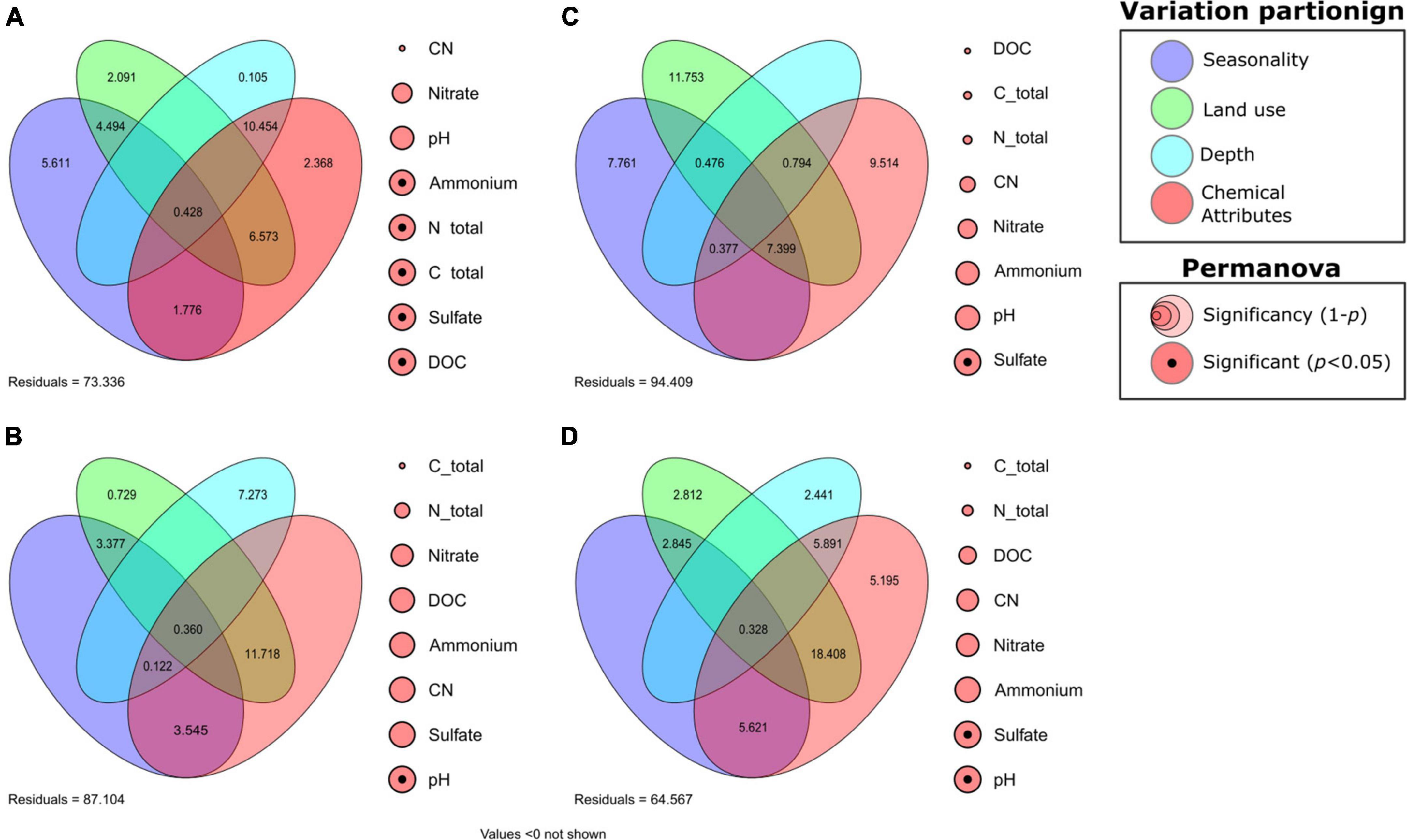
Figure 3. Variance partitioning of the active ammonia-oxidizing and nitrogen-dependent anaerobic methane-oxidizing communities, followed by the permutational analysis of variance of each chemical attribute. (A) Ammonia-oxidizing archaea (AOA), (B) ammonia-oxidizing bacteria (AOB), (C) Methanoperedens, and (D) Methylomirabilis.
The soil chemical attributes alone accounted for little of the variance in the AOA community (2.368%), but their interaction with the other edaphic factors contributed to the majority of the AOA variance (21.599%). Depth in combination with soil chemical factors (10.454%), land use with soil chemical attributes (6.573%), seasonality (5.611%), and seasonality with land use (4.494%) had major contributions to AOA variance. In comparison, land use (2.091 %), seasonality in combination with soil chemical factors (1.776%), all four factors (0.428%), and depth (0.105%) had subtle effects on variance (Figure 3A). In contrast to AOB, the PERMANOVA analysis of the chemical attributes of AOA was significantly influenced by other soil chemical variables, such as dissolved organic carbon (DOC), total carbon, total nitrogen, sulfate, and ammonium (Figure 3A).
Nitrogen-dependent anaerobic methane-oxidizing microbial community
We observed the presence of anaerobic methanotrophic archaea from the Methanoperedens genus and anaerobic methanotrophic bacteria from the Methylomirabilis genus in the soils from the floodplain of the Tocantins River across all seasons, land uses, and depths analyzed (Table 3 and Figure 2B). Methylomirabilis members were dominant across most samples, except for the D2 layer of the TFS site during the flood season, where Methanoperedens had a higher abundance (Figure 2B). Both methanotrophs had a higher absolute abundance during the flood season, especially in the D1 soil layer (Table 3).
The variance partition of the Methanoperedens members revealed that land use (11.753%), soil chemical factors (9.514%), and seasonality (7.761%) had strong influences on variance, as did the factors in combination (7.399%). The joint effect of land use with depth and soil chemical factors (0.794%), seasonality with land use and depth (0.476%), and seasonality with depth and soil chemical factors (0.377%) had small or negligible effects on variation (Figure 3C). PERMANOVA analysis was conducted to analyze the significance of each variable within the group of soil chemical attributes, and only sulfate was significant for Methanoperedens variation (Figure 3C).
For the variance partition of Methylomirabilis members, the joint effect of land use in combination with depth and soil chemical factors had the greatest influence on variation (18.408%). However, depth in combination with soil chemical factors (5.891%), soil chemical factors (5.195%), and seasonality in combination with soil chemical attributes (5.621 %) also contributed. Land use in combination with seasonality (2.845%), land use (2.812%), depth (2.441%), and the intersection of all four factors (0.328%) had a minor influence on the variation (Figure 3D). Finally, the PERMANOVA results revealed the significance of sulfate and pH.
Interactions between ammonia oxidizers and nitrogen-dependent anaerobic methane oxidizers
The Pearson correlation between the ammonia-oxidizing and nitrogen-dependent anaerobic methane-oxidizing groups during the different seasons showed a positive and significant linear correlation in both groups only during the flood season (R2 = 0.80, p = 0.002). This positive correlation was accompanied by a decrease in the relative abundance of the bacterial phyla Actinobacteria, Planctomycetes, Firmicutes, Verrucomicrobia, and Bacteroidetes (Supplementary Table 3). A positive trend was also observed during the non-flood season, but it did not present a high correlation coefficient or significance at the 5% level (R2 = 0.23, p = 0.47) (Figure 4).
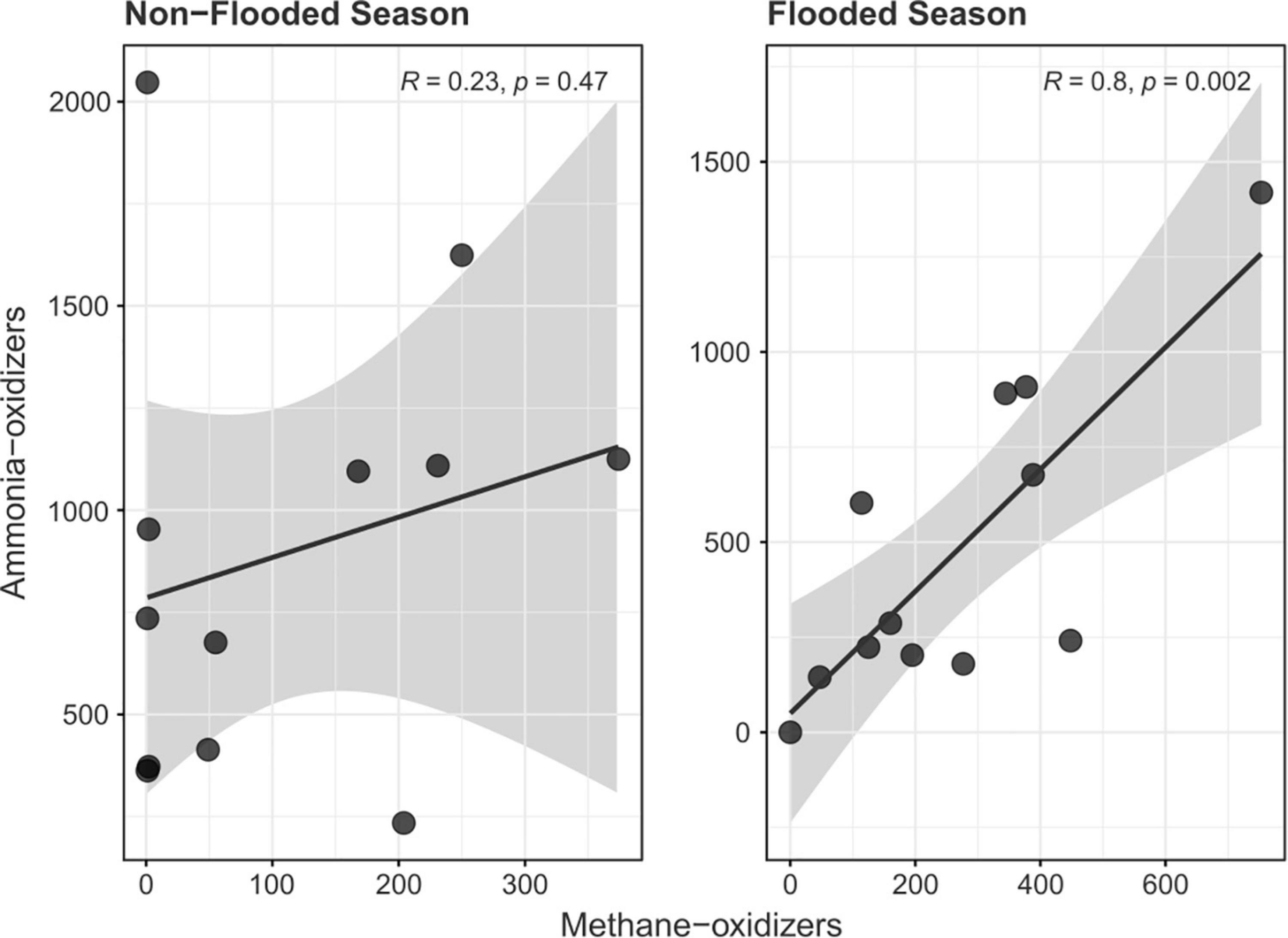
Figure 4. Correlation between active ammonia-oxidizing and nitrogen-dependent anaerobic ammonia-oxidizing communities during the flood and non-flood season.
Discussion
The results from this study reveal the importance of ammonia oxidizers in the production of oxidized nitrogen species for the N-DAMO community under the anoxic environment seasonally created by the flood of the Tocantins River. The importance of seasonal environmental changes is highlighted, as well as changes in depth and forest composition on the ammonia-oxidizing and N-DAMO community. Changes in both microbial communities were also notably tied to the soil chemical composition and to seasonal changes in the soil water saturation.
Soil chemical profile
Cluster analysis of the chemical profiles of the Tocantins River floodplain soils showed significant dissimilarity in the chemical compositions in response to variations caused by seasonal changes, land use, and depth (Figure 1 and Table 2). Clear-water rivers, such as the Tocantins, drain areas with low erosion, are low in sediments and dissolved solids, and have intermediate fertility floodplains (Ríos-Villamizar et al., 2013). The low concentration of minerals in these rivers cannot provide significant fertility to the floodplain soils in the flood season; thus, most of the seasonal variations could relate to microbe-mediated processes that are enabled by flooding or by changes in the forest structure from FOR to TFS.
Under an anoxic environment created by increasing depth or flooding, nitrate (NO3–) is the preferred electron acceptor for the decomposition of organic matter, followed closely by nitrite (NO2–), manganese (IV), and iodate (IO3–) in terms of the expected energy yield (Froelich et al., 1979; Torres-Alvarado et al., 2005; Lam and Kuypers, 2011). Sulfate (SO42–) can also be used as a terminal electron acceptor after all others have been removed, although it yields significantly less free energy (ΔG°) (Lam and Kuypers, 2011). Thus, NO3– and SO42– concentrations decreased in the soil, with NO3– being primarily consumed in both soil layers in the FOR and the D1 soil layer of the TFS site, whereas SO42– consumption was restricted to the D1 layer of the FOR and the D2 layer of the TFS site (Table 1). Both nitrate and sulfate reduction use H+ ions, which can be related to the increase in pH that occurs during the flood season, indicating the use of both ions as electron acceptors. Bento et al. (2021) also detected the presence of common nitrifiers, such as Nitrososphaera and Nitrospira, through amplicon sequencing analysis of the 16S rRNA gene, highlighting the importance of alternative electron acceptors for biogeochemical cycles during the flood season in the Amazonian floodplains. This chemical transformation can only occur in the absence of oxygen (O2), which can be achieved by an increase in depth and by flooding, which explains the high proportion of the variability explained by the changes in season and depth (Table 2).
Nitrification is a two-step process whereby ammonia (NH3+) is first oxidized to NO3–, which is then further oxidized to NO2– (Prosser, 1990). In the first step of nitrification, ammonia oxidation is performed by the oxidation of NH3+ to hydroxylamine through the enzyme ammonia monooxygenase (AMO) (Hooper et al., 1997). O2 is also one of the requirements for the ammonia oxidation reaction, which can be limited by the lack of O2 caused by flooding. Thus, the accumulation of NH3+/ NH4+ during the flood season (Table 1) can also be explained by microbial activity, or in this case, the lack thereof.
Ammonia-oxidizing and nitrogen-dependent anaerobic methane-oxidizing community
The ammonia-oxidizing community had a clear dominance of archaea over bacteria in all samples (Table 3 and Figure 2). This is expected in acidic soils, such as those found in the floodplains of the Tocantins River (Table 1), since most of cultivated AOB are highly sensitive to low pH environments (Zhang et al., 2012). The pH value was the major chemical attribute affecting AOB variance (Figure 3B), which is believed to be due to the low ammonia (NH3+) availability at low pH levels, as NH3+ is exponentially ionized to ammonium (NH4+), and NH3+ rather than NH4+ is thought to be the substrate for the ammonia monooxygenase enzyme, which catalyzes ammonia oxidation (Suzuki et al., 1974; Lehtovirta-Morley et al., 2011). Furthermore, kinetic studies on the isolated AOA Nitrosopumilus maritimus (strain SCM1) indicated a high ammonia affinity, suggesting that Nitrosopumilus-like AOA could not only out-compete AOB in ammonium-depleted environments, such as acidic soils, but could also effectively compete with other heterotrophs and phytoplankton for ammonia under these conditions (Martens-Habbena et al., 2009), The analysis of the membrane structure and genome of the acidophilic AOA isolate Candidatus Nitrosotalea devanaterra also indicated the presence of mechanisms for low pH tolerance, such as cation uptake, cytoplasmic buffering, and a cell membrane composition distinct from that of neutrophilic AOA (Lehtovirta-Morley et al., 2016). AOA members also encode a different type of N transporter, amt-NH4+ transporter, which differs from the Rh-NH3 transporters present in AOB, indicating better adaptation of AOA to acidic environments, such as those found throughout the Amazon floodplains (Offre et al., 2014; Lehtovirta-Morley et al., 2016).
Tropical soils are subjected to many environmental constraints, and the related microbes have a strong selective pressure to withstand and colonize these harsh and highly dynamic environments. Tropical soil bacterial communities contain more closely related taxa at the tip of the phylogenetic tree from more distantly related clades, suggesting a higher proportion of niche specialists (Bahram et al., 2018). Ammonia-oxidizing archaeal communities could behave in a similar way regarding the phylogenetic composition of the community, since, in acidic soils, ammonia oxidation is dominated by highly adapted archaea. These archaea are from closely related taxa of two evolutionarily distant acidophilic clades within the Thaumarchaeota phylum, one of which is related to the genus Nitrosotalea (Clusters 14 and 15) and the other to the genus Nitrososphaera (Cluster 11) (Gubry-Rangin et al., 2011, 2015; Macqueen and Gubry-Rangin, 2016). The evolution of these acidophilic clades appears to be driven mainly by pH, which is expected, as pH is one of the principal factors that determine niche differentiation between ammonia-oxidizing microorganisms (Gubry-Rangin et al., 2015).
Ammonia-oxidizing archaea are chemolithotrophs that can couple the oxidation of ammonia to C fixation, and the total nitrogen and ammonium/ammonia (hereafter referred to as “ammonia”) concentrations can directly influence this process (Stahl and de la Torre, 2012). AOA have a high affinity for ammonia and are well adapted to low nitrogen environments (Martens-Habbena et al., 2009). The AOA are autotrophs and they can fix carbon through a modified version of the 3- hydroxypropionate/4-hydroxybutyrate pathway (Walker et al., 2010; Könneke et al., 2014). AOB members of the Nitrosomonadaceae family are obligate autotrophs that can only fix carbon through the Calvin cycle (Clark et al., 2021). This could represent another evolutionary advantage for AOA in tropical floodplain soils, as DOC and total carbon had a significant effect on their variance (Figure 3A).
The nitrogen-dependent methane-oxidizing community had a higher abundance of Methylomirabilis than Methanoperedens, which can occur because of the absence of anammox taxa in our samples, which decreased the competition for NO2– in the anoxic environment created by the flood, thus favoring other NO2– reducers. NO2– is especially important for Methylomirabilis oxyfera, as it couples NO2– reduction to an intra-aerobic methane oxidation pathway (Ettwig et al., 2010), and has previously been identified in the floodplains of Amazonian rivers (Gabriel et al., 2020). Additionally, excessive competition can be the cause of the low abundance of Methanoperedens, as NO3– is the preferred electron acceptor for anaerobic decomposition of organic material (Torres-Alvarado et al., 2005) and can also be used for nitrogen assimilation into the biomass of a multitude of organisms, including plants (Kuypers et al., 2018). In addition, NO3– has high water solubility and can be easily lost in the soil profile through runoff (Robertson and Vitousek, 2009).
Members of both the Methanoperedenaceae and Methylomirabilaceae families have been previously co-enriched (Raghoebarsing et al., 2006), and in the absence of ammonia, which would inhibit anaerobic ammonia oxidation (anammox) activity and NO2– consumption, the dominant microbial consortium in an anaerobic bioreactor fed with CH4 and NO3– was that of the archaeon Candidatus Methanoperdens nitroreducens and the bacterium Candidatus Methylomirabilis oxyfera (Haroon et al., 2013), which were also detected in this floodplain (Gabriel et al., 2020; Bento et al., 2021; Gontijo et al., 2021).
The model of interaction between Methanoperdens nitroreducens and Methylomirabilis oxyfera, described by Haroon et al. (2013), may also be applied to the Tocantins River floodplain soils where high CH4 availability (Bento et al., 2021) and highly acidic (pH < 4) soils with low ammonia availability could inhibit anammox activity (Bai et al., 2015; Daverey et al., 2015) and allow for the cooperation between both methanotrophs. Methanoperdens nitroreducens can couple methane oxidation to reduce NO3– to NO2– (Raghoebarsing et al., 2006), which would fuel Methylomirabilis oxyfera as it couples methane oxidation to NO2– reduction (Ettwig et al., 2009).
The AOM processes conducted by the members of both the Methanoperedens and Methylomirabilis genera can only occur under anaerobic conditions, and the total abundance of the nitrogen-dependent anaerobic methane oxidizers was considerably reduced during the non-flood season, approaching zero in D1 of the TFS site (Table 3). In contrast, the total abundance of ammonia oxidizers was reduced during the flood season due to O2 limitations caused by flooding but remained more abundant during the flood season than both nitrogen-dependent anaerobic methane oxidizers combined.
Ammonia-oxidizing archaea have been detected in oxygen-minimum zones (O2 ≤ 20 μM) and are considered providers of oxidized nitrogen species (nitrate and nitrite) to microbes in oxygen-minimum zones (Stahl and de la Torre, 2012). The supply of such nitrogen species can also fuel nitrogen-dependent anaerobic methane oxidation, especially in acidic environments such as the Tocantins River floodplain, where ammonium rather than ammonia is the most abundant ionic species, which could inhibit anammox activity. This inhibition would allow Methanoperedens and Methylomirabilis to undertake the process of nitrogen removal in the anaerobic and oxygen minimum zones, while AOA would remain active as they are better adapted to low pH and ammonium-rich environments and could present significantly higher oxygen affinities to withstand the flooded environment (Stahl and de la Torre, 2012). This interaction between both groups can only occur during the flood season, as biogeochemistry would not favor this combination in the non-flood season (Figure 4).
Conclusion
The results of the current study showed that both ammonia oxidation and methanotrophy were deeply tied to the floodplain hydrological cycle, which is influenced by seasonal changes. In addition, both communities were influenced by forest composition and soil depth. Seasonal changes in soil water saturation were the main drivers of community composition, which in turn had significant effects on soil chemical factors. The ammonia-oxidizing community presented certain resilience to seasonal changes despite the relatively aerobic nature of the detected ammonia oxidizers and was also dominated by AOA members, as expected for acidic soils. The nitrogen-dependent anaerobic CH4-oxidizing community presented a clear difference between seasons, with greater abundance during the flood season, and was affected by changes in forest composition and soil depth. Based on 16S rRNA transcript amplicon sequencing data and statistical analysis, aerobic ammonia oxidizers were significantly related to the nitrogen-dependent anaerobic CH4-oxidizing community during the flood season, as ammonia oxidation can provide oxidized nitrogen members that can be coupled to anaerobic CH4 oxidation. We suggest that these theoretical relationships can be validated in future studies to provide insight into the complexity of the carbon and nitrogen cycles in the Amazonian floodplain and the carbon and nitrogen cycling microbial community responses to environmental changes, which can include those related to ongoing climate change.
Data availability statement
The datasets presented in this study can be found in online repositories. The name of the repository and accession number can be found below: National Center for Biotechnology Information (NCBI) BioProject, https://www.ncbi.nlm.nih.gov/bioproject/, PRJNA732086.
Author contributions
AN, PB, and VN contributed to the conception and design of the study. AN, GM, and DB organized the database. GM, GV, and TV performed the statistical analysis. GM, AN, and GG wrote the first draft of the manuscript. AV, LO, VN, PB, CM, and ST wrote sections of the manuscript. All authors contributed to manuscript revision, read, and approved the submitted version.
Funding
This study was financed by a grant from Fundação de Amparo à Pesquisa do Estado de São Paulo, Brasil (FAPESP) (2016/16687-3). DB was supported by the Coordenação de Aperfeiçoamento de Pessoal de Nível Superior, Brasil (CAPES), Financial Code 001. GG was supported by CAPES, Financial Code 001 (process number 88882.306808/2018-01). AV was supported by FAPESP (2019/25931-3) and is currently supported by the Fung Global Fellows Program of the Princeton Institute for International and Regional Studies (PIIRS, Princeton University). AN was supported by FAPESP (2017/03575–5). AN, CM, and ST would like to thank CNPq for the Research Productivity Grants (309746/2021-3, 315190/2021-3, and 314806/2021-0).
Acknowledgments
We thank Marília de Souza Bento and Leonardo Machado Pitombo for their overall support. We also thank Ivanildo L. S. Gaia, Glauber Altrão Carvalho, and Domickson Costa for their support during fieldwork.
Conflict of interest
The authors declare that the research was conducted in the absence of any commercial or financial relationships that could be construed as a potential conflict of interest.
Publisher’s note
All claims expressed in this article are solely those of the authors and do not necessarily represent those of their affiliated organizations, or those of the publisher, the editors and the reviewers. Any product that may be evaluated in this article, or claim that may be made by its manufacturer, is not guaranteed or endorsed by the publisher.
Supplementary material
The Supplementary Material for this article can be found online at: https://www.frontiersin.org/articles/10.3389/fmicb.2022.913453/full#supplementary-material
Footnotes
References
Agência Nacional de Águas [ANA] (2009). Plano Estratégico de Recursos Hídricos da Bacia Hidrográfica dos rios Tocantins e Araguaia: Relatório Síntese. Brasília: ANA.
Agência Nacional de Águas [ANA] (2015). Conjuntura dos recursos hídricos no Brasil: regiões hidrográficas brasileiras – Edição Especial. Brasília: ANA.
Agostinetto, D., Fleck, N. G., Rizzardi, M. A., and Balbinot, A. A. Jr. (2002). Potencial de emissão de metano em lavouras de arroz irrigado. Ciênc. Rural 32, 1073–1081. doi: 10.1590/s0103-84782002000600026
Alvares, C. A., Stape, J. L., Sentelhas, P. C., de Moraes Gonçalves, J. L., and Sparovek, G. (2013). Köppen’s climate classification map for Brazil. Meteorol. Z. 22, 711–728. doi: 10.1127/0941-2948/2013/0507
Apprill, A., McNally, S., Parsons, R., and Weber, L. (2015). Minor revision to V4 region SSU rRNA 806R gene primer greatly increases detection of SAR11 bacterioplankton. Aquat. Microb. Ecol. 75, 129–137. doi: 10.3354/ame01753
Arshad, A., Speth, D. R., De Graaf, R. M., Op den Camp, H. J. M., Jetten, M. S. M., and Welte, C. U. (2015). A metagenomics-based metabolic model of nitrate-dependent anaerobic oxidation of methane by methanoperedens-like archaea. Front. Microbiol. 6:1423. doi: 10.3389/fmicb.2015.01423
Bahram, M., Hildebrand, F., Forslund, S. K., Anderson, J. L., Soudzilovskaia, N. A., Bodegom, P. M., et al. (2018). Structure and function of the global topsoil microbiome. Nature 560, 233–237. doi: 10.1038/s41586-018-0386-6
Bai, R., Xi, D., He, J. Z., Hu, H. W., Fang, Y. T., and Zhang, L. M. (2015). Activity, abundance and community structure of anammox bacteria along depth profiles in three different paddy soils. Soil Biol. Biochem. 91, 212–221. doi: 10.1016/j.soilbio.2015.08.040
Beal, E. J., House, C. H., and Orphan, V. J. (2009). Manganese- and iron-dependent marine methane oxidation. Science 325, 184–187. doi: 10.1126/science.1169984
Bento, M., de, S., Barros, D. J., Araújo, M. G., da, S., Da Róz, R., et al. (2021). Active methane processing microbes and the disproportionate role of NC10 phylum in methane mitigation in Amazonian floodplains. Biogeochemistry 156, 293–317. doi: 10.1007/s10533-021-00846-z
Bloom, A. A., Palmer, P. I., Fraser, A., and Reay, D. S. (2012). Seasonal variability of tropical wetland CH4 emissions: the role of the methanogen-available carbon pool. Biogeosciences 9, 2821–2830. doi: 10.5194/bg-9-2821-2012
Bloom, A. A., Palmer, P. I., Fraser, A., Reay, D. S., and Frankenberg, C. (2010). Large-scale controls of methanogenesis inferred from methane and gravity spaceborne data. Science 327, 322–325. doi: 10.1126/science.1175176
Boetius, A., Ravenschlag, K., Schubert, C. J., Rickert, D., Widdel, F., Gleeske, A., et al. (2000). A marine microbial consortium apparently mediating AOM. Nature 407, 623–626. doi: 10.1073/pnas.072210299
Borcard, D., Gillet, F., and Legendre, P. (2018). “Canonical Ordination,” in Numerical Ecology with R, eds D. Borcard, F. Gillet, and P. Legendre (Cham: Springer International Publishing), 203–297.
Cantarella, H., and Prochnow, L. I. (2001). “Determinaçãoo de sulfato em solos,” in Análise Química para Avaliação da Fertilidade de Solos Tropicais, eds B. van Raij, J. C. Andrade, H. C. Cantarella, and J. A. Quaggio (Campinas: Instituto Agronômico de Campinas), 225–230.
Caporaso, J. G., Bittinger, K., Bushman, F. D., DeSantis, T. Z., Andersen, G. L., and Knight, R. (2010a). PyNAST: a flexible tool for aligning sequences to a template alignment. Bioinformatics 26, 266–267. doi: 10.1093/bioinformatics/btp636
Caporaso, J. G., Kuczynski, J., Stombaugh, J., Bittinger, K., Bushman, F. D., Costello, E. K., et al. (2010b). QIIME allows analysis of high-throughput community sequencing data. Nat. Methods 7, 335–336. doi: 10.1038/nmeth.f.303
Ciais, P., Sabine, C., Bala, G., Bopp, L., Brovkin, V., Canadell, A., et al. (2009). “Carbon and Other Biogeochemical Cycles BT - Climate Change The physical Science Basis. Contribution of Working Group I to the Fifth Assessment Report of the intergovernmental Panel on Climate Change,” in Climate Change The Physical Science Basis. Contribution of Working Group I to the Fifth Assessment Report of the Intergovernmental Panel on Climate Change (Peter), 465–570. Available online at: http://ebooks.cambridge.org/ref/id/CBO9781107415324A023%0Apapers3://publication/doi/10.1017/cbo9781107415324.015 [accessed Dec 17, 2020].
Clark, I. M., Hughes, D. J., Fu, Q., Abadie, M., and Hirsch, P. R. (2021). Metagenomic approaches reveal differences in genetic diversity and relative abundance of nitrifying bacteria and archaea in contrasting soils. Sci. Rep. 11:15905. doi: 10.1038/s41598-021-95100-9
Conrad, R. (2009). The global methane cycle: recent advances in understanding the microbial processes involved. Environ. Microbiol. Rep. 1, 285–292. doi: 10.1111/j.1758-2229.2009.00038.x
Cui, M., Ma, A., Qi, H., Zhuang, X., and Zhuang, G. (2015). Anaerobic oxidation of methane: an “active” microbial process. Microbiologyopen 4, 1–11. doi: 10.1002/mbo3.232
Dalal, R. C., and Allen, D. E. (2008). Greenhouse gas fluxes from natural ecosystems. Aust. J. Bot. 56:369. doi: 10.1071/BT07128
Daverey, A., Chei, P. C., Dutta, K., and Lin, J. G. (2015). Statistical analysis to evaluate the effects of temperature and pH on anammox activity. Int. Biodeterior. Biodegrad. 102, 89–93. doi: 10.1016/j.ibiod.2015.03.006
De Mérona, B., Juras, A. A., Santos, G. M. D., and Cintra, I. H. A. (2010). Os Peixes e a Pesca no Baixo Rio Tocantins: Vinte Anos Depois da UHE Tucuruí. São Carlos: Eletrobrás Eletronorte.
Edgar, R. C. (2010). Search and clustering orders of magnitude faster than BLAST. Bioinformatics 26, 2460–2461. doi: 10.1093/bioinformatics/btq461
Edgar, R. C. (2013). UPARSE: highly accurate OTU sequences from microbial amplicon reads. Nat. Methods 10, 996–998. doi: 10.1038/nmeth.2604
Ettwig, K. F., Butler, M. K., Le Paslier, D., Pelletier, E., Mangenot, S., Kuypers, M. M. M., et al. (2010). Nitrite-driven anaerobic methane oxidation by oxygenic bacteria. Nature 464, 543–548. doi: 10.1038/nature08883
Ettwig, K. F., Van Alen, T., Van De Pas-Schoonen, K. T., Jetten, M. S. M., and Strous, M. (2009). Enrichment and molecular detection of denitrifying methanotrophic bacteria of the NC10 phylum. Appl. Environ. Microbiol. 75, 3656–3662. doi: 10.1128/AEM.00067-09
Ettwig, K. F., Zhu, B., Speth, D., Keltjens, J. T., Jetten, M. S. M., and Kartal, B. (2016). Archaea catalyze iron-dependent anaerobic oxidation of methane. Proc. Natl. Acad. Sci. U.S.A. 113, 12792–12796. doi: 10.1073/pnas.1609534113
Ewing, B., and Green, P. (1998). Base-calling of automated sequencer traces using phred. ii. error probabilities. Genome Res. 8, 186–194. doi: 10.1101/gr.8.3.186
Froelich, P. N., Klinkhammer, G. P., Bender, M. L., Luedtke, N. A., Heath, G. R., Cullen, D., et al. (1979). Early oxidation of organic matter in pelagic sediments of the eastern equatorial Atlantic: suboxic diagenesis. Geochim. Cosmochim. Acta 43, 1075–1090. doi: 10.1016/0016-7037(79)90095-4
Gabriel, G. V. M., Oliveira, L. C., Barros, D. J., Bento, M. S., Neu, V., Toppa, R. H., et al. (2020). Methane emission suppression in flooded soil from amazonia. Chemosphere 250:126263. doi: 10.1016/j.chemosphere.2020.126263
Gomes, E. P., Blanco, C. J. C., and Pessoa, F. C. L. (2019). Identification of homogeneous precipitation regions via Fuzzy c-means in the hydrographic region of tocantins–araguaia of brazilian amazonia. Appl. Water Sci. 9, 1–12. doi: 10.1007/s13201-018-0884-6
Gontijo, J. B., Paula, F. S., Venturini, A. M., Yoshiura, C. A., Borges, C. D., Moura, J. M. S., et al. (2021). Not just a methane source: amazonian floodplain sediments harbour a high diversity of methanotrophs with different metabolic capabilities. Mol. Ecol. 30, 2560–2572. doi: 10.1111/mec.15912
Gubry-Rangin, C., Hai, B., Quince, C., Engel, M., Thomson, B. C., James, P., et al. (2011). Niche specialization of terrestrial archaeal ammonia oxidizers. Proc. Natl. Acad. Sci. U.S.A. 108, 21206–21211. doi: 10.1073/pnas.1109000108
Gubry-Rangin, C., Kratsch, C., Williams, T. A., McHardy, A. C., Embley, T. M., Prosser, J. I., et al. (2015). Coupling of diversification and pH adaptation during the evolution of terrestrial Thaumarchaeota. Proc. Natl. Acad. Sci. U.S.A. 112, 9370–9375. doi: 10.1073/pnas.1419329112
Hammer, Ø, Harper, D. A. T., and Ryan, P. D. (2001). PAST: paleontological statistics software package for education and data analysis. Palaeontol. Electron 4:9.
Haroon, M. F., Hu, S., Shi, Y., Imelfort, M., Keller, J., Hugenholtz, P., et al. (2013). Anaerobic oxidation of methane coupled to nitrate reduction in a novel archaeal lineage. Nature 500, 567–570. doi: 10.1038/nature12375
Hooper, A. B., Vannelli, T., Bergmann, D. J., and Arciero, D. M. (1997). Enzymology of the oxidationof ammonia to nitrate by bacteria. Antonie van Leeuwenhoek 71, 59–67.
Junk, W. J., and Mello, J. A. S. N. De (1987). “Impactos ecológicos das represas hidrelétricas na bacia amazônica brasileira,” in Homem e Natureza na Amazônia, eds G. Kohlhepp and A. Schrader (Tübingen: Universität Tübingen), 37–385.
Kassambara, A. (2020). ggubr: ‘ggplot2’ Based Publication Ready Plots. R Package Version 0.4.0. Vienna: R Foundation for Statistical Computing.
Kirschke, S., Bousquet, P., Ciais, P., Saunois, M., Canadell, J. G., Dlugokencky, E. J., et al. (2013). Three decades of global methane sources and sinks. Nat. Geosci. 6, 813–823. doi: 10.1038/ngeo1955
Klindworth, A., Pruesse, E., Schweer, T., Peplies, J., Quast, C., Horn, M., et al. (2013). Evaluation of general 16S ribosomal RNA gene PCR primers for classical and next-generation sequencing-based diversity studies. Nucleic Acids Res. 41:e1. doi: 10.1093/nar/gks808
Knittel, K., and Boetius, A. (2009). Anaerobic oxidation of methane: progress with an unknown process. Annu. Rev. Microbiol. 63, 311–334. doi: 10.1146/annurev.micro.61.080706.093130
Könneke, M., Schubert, D. M., Brown, P. C., Hügler, M., Standfest, S., Schwander, T., et al. (2014). Ammonia-oxidizing archaea use the most energy-efficient aerobic pathway for CO2 fixation. Proc. Natl. Acad. Sci. U.S.A. 111, 8239–8244. doi: 10.1073/pnas.1402028111
Krom, M. D. (1980). Spectrophotometric determination of ammonia: a study of a modified Berthelot reaction using salicylate and dichloroisocyanurate. Analyst 105:305. doi: 10.1039/an9800500305
Kuypers, M. M. M., Marchant, H. K., and Kartal, B. (2018). The microbial nitrogen-cycling network. Nat. Rev. Microbiol. 16, 263–276. doi: 10.1038/nrmicro.2018.9
Lam, P., and Kuypers, M. M. M. (2011). Microbial nitrogen cycling processes in oxygen minimum zones. Ann. Rev. Mar. Sci. 3, 317–345. doi: 10.1146/annurev-marine-120709-142814
Lehtovirta-Morley, L. E., Sayavedra-Soto, L. A., Gallois, N., Schouten, S., Stein, L. Y., Prosser, J. I., et al. (2016). Identifying potential mechanisms enabling acidophily in the ammonia-oxidizing archaeon “Candidatus Nitrosotalea devanaterra.”. Appl. Environ. Microbiol. 82, 2608–2619. doi: 10.1128/AEM.04031-15
Lehtovirta-Morley, L. E., Stoecker, K., Vilcinskas, A., Prosser, J. I., and Nicol, G. W. (2011). Cultivation of an obligate acidophilic ammonia oxidizer from a nitrifying acid soil. Proc. Natl. Acad. Sci. U.S.A. 108, 15892–15897. doi: 10.1073/pnas.1107196108
Macqueen, D. J., and Gubry-Rangin, C. (2016). Molecular adaptation of ammonia monooxygenase during independent pH specialization in Thaumarchaeota. Mol. Ecol. 25, 1986–1999. doi: 10.1111/mec.13607
Martens-Habbena, W., Berube, P. M., Urakawa, H., De La Torre, J. R., and Stahl, D. A. (2009). Ammonia oxidation kinetics determine niche separation of nitrifying Archaea and Bacteria. Nature 461, 976–979. doi: 10.1038/nature08465
Meyer, K. M., Klein, A. M., Rodrigues, J. L. M., Nüsslein, K., Tringe, S. G., Mirza, B. S., et al. (2017). Conversion of Amazon rainforest to agriculture alters community traits of methane-cycling organisms. Mol. Ecol. 26, 1547–1556. doi: 10.1111/mec.14011
Myhre, G., Shindell, D., Bréon, F., Collins, W., Fuglestvedt, J., Huang, J., et al. (2013). Anthropogenic and Natural Radiative Forcing: In Climate Change 2013: The Physical Science Basis. Contribution of Working Group I to the Fifth Assessment Report of the Intergovernmental Panel on Climate Change. Cambridge: Cambridge Univ. Press, 659–740.
Norman, R. J., Edberg, J. C., and Stucki, J. W. (1985). Determination of nitrate in soil extracts by dual-wavelength ultraviolet spectrophotometry. Soil Sci. Soc. Am. J. 49, 1182–1185. doi: 10.2136/sssaj1985.03615995004900050022x
Offre, P., Kerou, M., Spang, A., and Schleper, C. (2014). Variability of the transporter gene complement in ammonia-oxidizing archaea. Trends Microbiol. 22, 665–675. doi: 10.1016/j.tim.2014.07.007
Oksanen, J., Blanchet, F. G., Friendly, M., Kindt, R., Legendre, P., McGlinn, D., et al. (2022). Vegan: Community Ecology Package. R package version 2.5-7. 2020.
Parada, A. E., Needham, D. M., and Fuhrman, J. A. (2016). Every base matters: assessing small subunit rRNA primers for marine microbiomes with mock communities, time series and global field samples. Environ. Microbiol. 18, 1403–1414. doi: 10.1111/1462-2920.13023
Prosser, J. I. (1990). Autotrophic nitrification in bacteria. Adv. Microb. Physiol. 30, 125–181. doi: 10.1016/S0065-2911(08)60112-5
Pylro, V. S., Roesch, L. F. W., Morais, D. K., Clark, I. M., Hirsch, P. R., and Tótola, M. R. (2014). Data analysis for 16S microbial profiling from different benchtop sequencing platforms. J. Microbiol. Methods 107, 30–37. doi: 10.1016/j.mimet.2014.08.018
Raghoebarsing, A. A., Pol, A., Van De Pas-Schoonen, K. T., Smolders, A. J. P., Ettwig, K. F., Rijpstra, W. I. C., et al. (2006). A microbial consortium couples anaerobic methane oxidation to denitrification. Nature 440, 918–921. doi: 10.1038/nature04617
Ríos-Villamizar, E. A., Piedade, M. T. F., Da Costa, J. G., Adeney, J. M., and Junk, W. J. (2013). Chemistry of different amazonian water types for river classification: a preliminary review. WIT Trans. Ecol. Environ. 178, 17–28. doi: 10.2495/WS130021
Robertson, G. P., and Vitousek, P. M. (2009). Nitrogen in agriculture: balancing the cost of an essential resource. Annu. Rev. Environ. Resour. 34, 97–125. doi: 10.1146/annurev.environ.032108.105046
RStudio Team (2018). RStudio: Integrated Development for R. Available online at: http://www.rstudio.com/
Silva, F. C. (2009). Manual de Análises Químicas de Solos, Plantas e Fertilizantes. Distrito Federal: Embrapa Informação Tecnológica, 627.
Stahl, D. A., and de la Torre, J. R. (2012). Physiology and Diversity of Ammonia-Oxidizing Archaea. Annu. Rev. Microbiol 66, 83–101. doi: 10.1146/annurev-micro-092611-150128
Steudler, P. A., Melillo, J. M., Feigl, B. J., Neill, C., Piccolo, M. C., and Cerri, C. C. (1996). Consequence of forest-to-pasture conversion on CH4 fluxes in the brazilian amazon basin. J. Geophys. Res. Atmos. 101, 18547–18554. doi: 10.1029/96jd01551
Suzuki, I., Dular, U., and Kwok, S. C. (1974). Ammonia or ammonium ion as substrate for oxidation by Nitrosomonas europaea cells and extracts. J. Bacteriol. 120, 556–558. doi: 10.1128/jb.120.1.556-558.1974
Torres-Alvarado, R., Ramírez-vives, F., Fernández, F. J., and Barriga-sosa, I. (2005). Methanogenesis and methane oxidation in wetlands. Implications in the global carbon cycle Metanogénesis y metano-oxidación en humedales. Implicaciones en el ciclo del carbono global. 15, 327–349.
Walker, C. B., De La Torre, J. R., Klotz, M. G., Urakawa, H., Pinel, N., Arp, D. J., et al. (2010). Nitrosopumilus maritimus genome reveals unique mechanisms for nitrification and autotrophy in globally distributed marine crenarchaea. Proc. Natl. Acad. Sci. U.S.A. 107, 8818–8823. doi: 10.1073/pnas.0913533107
Walter, B. P., Heimann, M., and Matthews, E. (2001). Modeling modern methane emissions from natural wetlands 2. Interannual variations 1982-1993. J. Geophys. Res. Atmos 106, 34207–34219. doi: 10.1029/2001JD900164
Wickham, H., and Chang, W. (2016). ggplot2’: Create Elegant Data Visualisations Using the Grammar of Graphics. R package version 3.1.0.
World Meteorological Organization [WMO] (2021). WMO Greenhouse Gas Bulletin (GHG Bulletin) – No.17: The State of Greenhouse Gases in the Atmosphere Based on Global Observations Through 2020. Geneva: WMO. Available online at: https://library.wmo.int/index.php?lvl=notice_display&id=21975
Keywords: ammonia oxidation, methanotrophs, Thaumarchaeota, 16S rRNA sequencing, tropical floodplains
Citation: Monteiro GGTN, Barros DJ, Gabriel GVM, Venturini AM, Veloso TGR, Vazquez GH, Oliveira LC, Neu V, Bodelier PLE, Mansano CFM, Tsai SM and Navarrete AA (2022) Molecular evidence for stimulation of methane oxidation in Amazonian floodplains by ammonia-oxidizing communities. Front. Microbiol. 13:913453. doi: 10.3389/fmicb.2022.913453
Received: 05 April 2022; Accepted: 08 July 2022;
Published: 01 August 2022.
Edited by:
Antonio Castellano-Hinojosa, University of Florida, United StatesReviewed by:
J. Michael Beman, University of California, Merced, United StatesMiguel Hurtado Martínez, University of Granada, Granada, Spain
Copyright © 2022 Monteiro, Barros, Gabriel, Venturini, Veloso, Vazquez, Oliveira, Neu, Bodelier, Mansano, Tsai and Navarrete. This is an open-access article distributed under the terms of the Creative Commons Attribution License (CC BY). The use, distribution or reproduction in other forums is permitted, provided the original author(s) and the copyright owner(s) are credited and that the original publication in this journal is cited, in accordance with accepted academic practice. No use, distribution or reproduction is permitted which does not comply with these terms.
*Correspondence: Acacio A. Navarrete, YWNhY2lvbmF2YXJyZXRlQGdtYWlsLmNvbQ==