- 1Biozentrum, University of Basel, Basel, Switzerland
- 2Department of Biochemistry, Faculty of Biology and Medicine, Université de Lausanne, Epalinges, Switzerland
- 3Maucher Jenkins, Freiburg, Germany
- 4Institute of Medical Microbiology and Hygiene, Interfaculty Institute of Microbiology and Infection Medicine (IMIT), University of Tübingen, Tübingen, Germany
- 5Section of Cellular and Molecular Microbiology, Interfaculty Institute of Microbiology and Infection Medicine (IMIT), University of Tübingen, Tübingen, Germany
- 6Excellence Cluster “Controlling Microbes to Fight Infections” (CMFI), Tübingen, Germany
- 7Partner-site Tübingen, German Center for Infection Research (DZIF), Tübingen, Germany
Bartonella spp. are Gram-negative facultative intracellular pathogens that infect diverse mammals and cause a long-lasting intra-erythrocytic bacteremia in their natural host. These bacteria translocate Bartonella effector proteins (Beps) into host cells via their VirB/VirD4 type 4 secretion system (T4SS) in order to subvert host cellular functions, thereby leading to the downregulation of innate immune responses. Most studies on the functional analysis of the VirB/VirD4 T4SS and the Beps were performed with the major zoonotic pathogen Bartonella henselae for which efficient in vitro infection protocols have been established. However, its natural host, the cat, is unsuitable as an experimental infection model. In vivo studies were mostly confined to rodent models using rodent-specific Bartonella species, while the in vitro infection protocols devised for B. henselae are not transferable for those pathogens. The disparities of in vitro and in vivo studies in different species have hampered progress in our understanding of Bartonella pathogenesis. Here we describe the murine-specific strain Bartonella taylorii IBS296 as a new model organism facilitating the study of bacterial pathogenesis both in vitro in cell cultures and in vivo in laboratory mice. We implemented the split NanoLuc luciferase-based translocation assay to study BepD translocation through the VirB/VirD4 T4SS. We found increased effector-translocation into host cells if the bacteria were grown on tryptic soy agar (TSA) plates and experienced a temperature shift immediately before infection. The improved infectivity in vitro was correlating to an upregulation of the VirB/VirD4 T4SS. Using our adapted infection protocols, we showed BepD-dependent immunomodulatory phenotypes in vitro. In mice, the implemented growth conditions enabled infection by a massively reduced inoculum without having an impact on the course of the intra-erythrocytic bacteremia. The established model opens new avenues to study the role of the VirB/VirD4 T4SS and the translocated Bep effectors in vitro and in vivo.
Introduction
Bartonellae are Gram-negative facultative intracellular pathogens, which infect diverse mammals including humans. Clinically relevant infections with Bartonella are caused by zoonotic Bartonella henselae, the agent of the cat scratch disease (CSD; Huarcaya et al., 2002; Khalfe and Lin, 2022) or human-specific species such as Bartonella bacilliformis, the agent of the life-threatening Carrion’s disease, and Bartonella quintana, which causes trench fever (Maguina et al., 2009; Mada et al., 2022). Bartonellae are highly host-restricted pathogens. After transmission by an arthropod vector, the bacteria enter the dermis and eventually seed into the blood stream where they cause a long-lasting intra-erythrocytic bacteremia as hallmark of infection in their natural host (Seubert et al., 2002; Chomel et al., 2009; Harms and Dehio, 2012). Infections of incidental hosts are not associated with intra-erythrocytic persistence but clinical manifestations caused by several zoonotic species can range from mild symptoms to severe diseases (Chomel and Kasten, 2010; Wagner and Dehio, 2019).
Previous studies demonstrated that the progression to the blood stream requires a functional VirB/VirD4 type 4 secretion system (T4SS; Schulein and Dehio, 2002). T4SS are multi-protein complexes embedded into the cell envelop. In Bartonellae, VirB2-11 are assembled to the functional T4SS that facilitates substrate translocation. The ATPase VirD4, also referred to as the type 4 secretion (T4S) coupling protein (T4CP), is essential for substrate recognition and entry into the T4SS (Berge et al., 2017; Waksman, 2019). In Bartonella species, the virB2 promoter (PvirB2) drives expression of the virB2-11 operon. This promoter and the separate promoter of virD4 are controlled by the BatR/BatS two-component system. Upregulated expression of the VirB/VirD4 T4SS in B. henselae is linked to the BatR/BatS two-component system activated at physiological pH and the alternative sigma factor RpoH1. Induction of RpoH1 is mediated by the stringent response, which relies on the accumulation of the second messenger guanosine tetra- and pentaphosphate (both referred to as ppGpp) in the bacterial cytosol (Quebatte et al., 2010, 2013).
The VirB/VirD4 T4SS is important to translocate multiple Bartonella effector proteins (Beps) into mammalian host cells to subvert host cellular functions, e.g., to dampen innate immune responses (Wagner et al., 2019; Fromm and Dehio, 2021). Different Bartonella species translocate discrete cocktails of Beps into host cells (Harms et al., 2017). While some orthologs share a conserved function (Sorg et al., 2020), other Beps seem to vary in a species-specific manner (Schmid et al., 2006a; Wang et al., 2019). Extensive studies focusing on the role of the VirB/VirD4 T4SS and the translocated Beps have been performed in vitro and in vivo. However, experimental studies on these bacteria in the natural host share the problem that either the host as model is hardly available or protocols for in vitro studies are missing. B. henselae is among the best-characterized Bartonella species and in vitro infection protocols using various cell lines or primary cells were published (Musso et al., 2001; McCord et al., 2005; Sorg et al., 2020; Marlaire and Dehio, 2021). Investigating B. henselae in its natural host, the cat, is laborious and expensive (Chomel et al., 1996; Foil et al., 1998). In a mouse infection model, B. henselae failed to establish long-lasting intra-erythrocytic bacteremia and pathology also differed from infections in the natural host (Regnath et al., 1998; Kunz et al., 2008). On the other hand, several rodent infection models with rodent-specific species were published that recapitulate the long-lasting intra-erythrocytic infection course characteristic for the natural host (Boulouis et al., 2001; Koesling et al., 2001; Schulein and Dehio, 2002; Deng et al., 2016; Siewert et al., 2022). However, besides an erythrocyte invasion model no in vitro protocols were established to study the interaction of those species with cells of their natural hosts (Vayssier-Taussat et al., 2010). Establishing for at least one Bartonella strain an experimental model for in vitro and in vivo studies would help studying the role of the VirB/VirD4 T4SS and its translocated Beps in the context of infection in the natural reservoir.
In this study, we investigated Bartonella taylorii IBS296 as a model organism to study VirB/VirD4 and Bep effector-related functions in vitro and in vivo. We confirmed that B. taylorii is dampening the innate immune response in vitro in a VirB/VirD4 and BepD-dependent manner. Further, we established the split NanoLuc luciferase-based translocation assay (Westerhausen et al., 2020) to study BepD translocation through the VirB/VirD4 T4SS. In addition, we improved the previously established mouse infection model for B. taylorii (Siewert et al., 2022) by lowering the inoculum by several orders of magnitude without affecting course of bacteremia. Our findings provide the basis for more extensive studies focusing on the immunomodulatory function of B. taylorii in vitro and in vivo.
Materials and Methods
Bacterial Strains and Growth Conditions
All bacterial strains used in this study are listed in Supplementary Table S1. Escherichia coli strains were cultivated in lysogeny broth (LB) or on solid agar plates (LA) supplemented with appropriate antibiotics at 37°C overnight.
Bartonella strains were grown at 35°C and 5% CO2 on Columbia blood agar (CBA) or tryptic soy agar (TSA) plates supplemented with 5% defibrinated sheep blood and appropriate antibiotics. Bartonella strains stored as frozen stocks at −80°C were inoculated on CBA or TSA plates for 3 days and subsequently expanded on fresh plates for 2 days. Prior to infection Bartonella strains were cultured in M199 medium supplemented with 10% heat-inactivated fetal calf serum (FCS) for 24 h at an optical density (OD600 nm) of 0.5 at 28°C or 35°C and 5% CO2.
Antibiotics or supplements were used in the following concentrations: kanamycin at 30 μg/ml, streptomycin at 100 μg/ml, isopropyl-b-D-thiogalactoside (IPTG) at 100 μM and diaminopimelic acid (DAP) at 1 mM.
Construction of Strains and Plasmids
DNA manipulations were performed according to standard techniques and all cloned inserts were DNA sequenced to confirm sequence integrity. For protein, complementation/overexpression in Bartonella selected genes were cloned into plasmid pBZ485_empty under the control of the taclac promoter. Chromosomal deletions or insertions of B. taylorii were generated by a two-step gene replacement procedure as previously described (Schulein and Dehio, 2002). A detailed description for the construction of each plasmid is presented in Supplementary Table S2. The sequence of all oligonucleotide primers used in this study is listed in Supplementary Table S3.
Plasmids were introduced into Bartonella strains by conjugation from E. coli strain MFDpir using two-parental mating as described previously (Harms et al., 2017).
Cell Lines and Culture Conditions
JAWS II (ATCC CRL-11904) cell line is a GM-CSF-dependent DC line established from bone marrow cells of a p53-knockout C57BL/6 mouse (Jiang et al., 2008). JAWS II cells were cultured at 37°C in 5% CO2 in complete culture medium consisting of MDM with 20% FCS, 4 mM L-glutamine, 1 mM sodium pyruvate, and 5 ng/ml GM-CSF. RAW 264.7 (ATCC TIB-71) cell line is a murine macrophage cell line originating from an adult male BALB/c mouse (Raschke et al., 1978). RAW 264.7 and RAW 264.7 LgBiT (obtained from E. Bohn, University Tübingen, Germany) cells were cultured at 37°C and 5% CO2 in DMEM Glutamax supplemented with 10% FCS. The RAW 264.7 LgBiT cell clone was generated exactly in the same manner as recently described for a HeLa cell clone (Westerhausen et al., 2020) with the exception that the RAW 267.4 LgBiT macrophages were treated with 3 ng/ml puromycin to select for stably transduced cells. In culture, the puromycin concentration was reduced to 2 ng/ml.
Cell Infections
B. henselae and B. taylorii strains were cultured as described above. One day before infection, 1 × 105 cells (JAWS II) or 5 × 105 cells (RAWs) were seeded per well in 12-well plates. 1 × 104 cells (RAW LgBiT) per well were seeded in white 96-well plates (Corning, Cat. CLS3610). One hour prior to infection bacterial cultures were supplemented with 100 μM IPTG to induce protein expression, if required. Cells were washed once with infection medium (DMEM Glutamax, supplemented with 1% FCS) and infected with a multiplicity of infection (MOI) of 50 bacteria per cell (Sorg et al., 2020), if not stated otherwise. Bacterial attachment was synchronized by centrifugation at 500 g for 3 min. Infected cells were incubated at 37°C and 5% CO2 for the indicated times. If indicated, cells were stimulated with 100 ng/ml LPS (lipopolysaccharides from E. coli O26:B6, impurities <3% protein, Sigma-Aldrich) during the last 2 h of infection at 37°C and 5% CO2. Supernatants were analyzed by Ready-SET-Go! ELISA kits for TNF-α. Adherent cells were harvested, lysed, and analyzed by immunoblot. For monitoring effector injection, luminescence reading was carried out in the Synergy H4 plate reader (BioTek).
Quantification of TNF-α Levels in Culture Supernatants
TNF-α was quantified in cell culture supernatants of infected cells by Ready-SET-Go! ELISA kits (Thermo Fisher Scientific, Cat. 88-7324-77) according to the manufacturer’s instructions. 96-well assay plates (Costar, Cat. 9018) were coated overnight at 4°C with capture antibody in coating buffer. The plates were washed with wash buffer (PBS containing 0.05% Tween-20) and incubated at RT for 1 h with assay diluent (provided in the kit) to block unspecific binding. Samples were added directly to the plate or after pre-dilution in assay diluent. Pre-dilutions used for TNF-α quantification: JAWS II (1 to 4 for samples with LPS stimulation; no pre-dilution for unstimulated samples), RAW 264.7 (1–6 for samples with LPS stimulation; no pre-dilution for unstimulated samples). Subsequently samples were diluted twice by serial 2-fold dilutions on the plate. The respective lyophilized standard was resolved as requested, added to the plate and diluted by serial 2-fold dilutions. The plate was incubated at 4°C overnight. Five wash steps were performed. After addition of the respective detection antibody, the plate was incubated 1 h at RT. Horseradish peroxidase-conjugated avidin was added for 30 min at room temperature after another five washing steps. After seven washes, ELISA substrate solution was added for 5–15 min at RT and the reaction stopped by adding 1 M H3PO4. Absorbance was read at 450 and 570 nm. At least, three independent experiments (n = 3) were performed in technical triplicates.
SDS-PAGE, Western Blotting, and Immunodetection
SDS-PAGE and immunoblotting were performed as described (Schulein et al., 2005). To verify expression levels of the protein of interest, JAWS II or RAW 264.7 cells were collected and washed in ice-cold PBS. Cell pellets were lysed by adding Novagen’s PhosphoSafe extraction buffer (Merck, Cat. 71296) complemented with cOmplete Mini EDTA-free protease inhibitor cocktail (Roche, Cat. 11836170001). Lysate protein concentrations were quantified using the Pierce BCA Protein Assay kit (Thermo Fisher Scientific, Cat. 23225). Lysates with equal protein concentrations were mixed with 5x SDS sample buffer and resolved on 4%–20% precast protein TGX gels (BioRad, Cat. 456-1093, Cat. 456-1096). Pre-stained Precision Plus Protein Dual Color Standard (BioRad, Cat. 1610374) was used as protein size reference. Proteins were transferred onto Amersham Protran® Nitocellulose Blotting membrane (0.45 μm pore size) or Amersham Hybond® PVDF membrane (0.2 μm pore size). Membranes were probed with primary antibodies directed against the protein of interest [α-actin (Millipore, Cat. MAB1501), α-pSTAT3 (Y705; Cell Signaling Technology, Cat. 9145), α-STAT3 (Cell Signaling Technology, Cat. 12640), α-FLAG (Sigma-Aldrich, Cat. F1804)]. Detection was performed with horseradish peroxidase-conjugated antibodies directed against rabbit or mouse IgG [HRP-linked α-mouse IgG (Cell Signaling Technology, Cat. 7076), HRP-linked α-rabbit IgG (Cell Signaling Technology, Cat. 7074)]. Immunoblots were developed using LumiGLO® chemiluminescent substrate (Seracare, Cat. 5430) and imaged using the Fusion FX device (Vilber). Signal quantification was performed using the Fusion FX7 Edge software. If required, images were adjusted in brightness and contrast using the ImageJ software.
Determination of Promoter Expression by Flow Cytometry
Bartonellae were grown on CBA or TSA plates as described above. Bacteria were resuspended in M199 + 10% FCS, diluted to a final concentration of OD600 nm = 0.5, and incubated for 24 h at 28°C or 35°C at 5% CO2. The bacteria were harvested, centrifuged at 1,900 g for 4 min, and washed in FACS buffer (2% FCS in PBS). After another centrifugation step, the supernatant was aspirated, bacteria fixed in 3.7% PFA for 10 min at 4°C, and finally resuspended in FACS buffer. Expression of the PvirB2:gfp promoter was evaluated by measuring the GFP fluorescence signal using the BD LSRFortessa. Data analysis was performed using FlowJo v10.6.2.
NanoLuc-Based Effector Translocation Assay
To assess whether the HiBiT-FLAG fragment, HiBiT-FLAG-BepDBhe, and HiBiT-FLAG-BepDBta can complement LgBiT to a functional luciferase, we used the Nano-Glo HiBiT lytic detection system (Promega, Cat. N3030). Bacteria were cultured as previously indicated. Around 5 × 108 bacteria were resuspended in 100 μl PBS and supplemented with 100 μl LSC buffer containing the substrate (1:50 v/v) and the LgBiT protein (1:100 v/v). The luminescent signal was measured using the Synergy H4 plate reader.
RAW LgBiT macrophages were infected as described previously. Effector translocation into macrophages was quantified by measuring the luminescent signal using the Synergy H4 plate reader. The Nano-Glo live cell reagent (Promega, Cat. N2011) was prepared as advised by manufacturer. After 24 h of infection, supernatant was aspirated and cells were gently washed in pre-warmed PBS. A final volume of 100 μl PBS per well was supplemented with 25 μl of the Nano-Glo live cell assay buffer containing the substrate for luminescence measurement in the Synergy H4 plate reader. The following settings were used: temperature 37°C, shaking sequence 30 s at 300–500 rpm, delay 10 min, autoscale, and integration time 5 s. At least, three independent experiments (n = 3) were performed in technical triplicates.
Mouse Experimentation
Mice handling was performed in strict accordance with the Federal Veterinary Office of Switzerland and local animal welfare bodies. All animal work was approved by the Veterinary Office of the Canton Basel City (license number 1741). All animals were housed at SPF (specific pathogen free) conditions and provided water and food ad libitum. The animal room was on a 12h light/12h dark cycle, and cage bedding changed every week. Female C57BL/6JRj mice were purchased from Janvier Labs.
Animals were infected i.d. with the indicated cfu bacteria in PBS. Blood was drawn in 3.8% sodium citrate from the tail vein several days post-infection. Whole blood was frozen at −80°C, thawed, and plated on CBA plates in serial dilutions to determine blood cfu count.
Statistical Analysis
Graphs were generated with GraphPad Prism 8. Statistical analyses were performed using one-way ANOVA with multiple comparisons (Tukey’s multiple comparison test). For the graphs presented in the figures, significance was denoted as non-significant (ns); p > 0.05; *p < 0.05; **p < 0.01; ***p < 0.001; ****p < 0.0001. Number of independent biological replicates is indicated as n in the figure legends.
Results
Establishment of a Cell Culture Assay for BepD Translocation via the Bartonella taylorii VirB/VirD4 T4SS
In vitro infection protocols devised for B. henselae enable an efficient and fast translocation of Beps via the VirB/VirD4 T4SS into eukaryotic host cells (Schmid et al., 2006b; Sorg et al., 2020). Inside host cells, the Bartonella effector protein D of B. henselae (BepDBhe) interacts via phospho-tyrosine domains (pY domains) with the transcription factor Signal Transducer and Activator of Transcription 3 (STAT3) and the Abelson tyrosine kinase (c-ABL). c-ABL than phosphorylates STAT3 on Y705 resulting in a downregulation of pro-inflammatory cytokine secretion. The orthologue effector encoded in B. taylorii, BepDBta, is also translocated and exhibits a conserved function when ectopically expressed in B. henselae (Sorg et al., 2020). We ectopically expressed BepDBhe and BepDBta in B. henselae ΔbepA-G, a genomic deletion mutant lacking all bep genes. We infected the mouse dendritic cell line JAWS II at a multiplicity of infection (MOI) of 50 for 6 h. Bacteria expressing BepDBhe or BepDBta facilitated STAT3 phosphorylation to a similar extend (Sorg et al., 2020; Supplementary Figure S1A). To establish an in vitro infection protocol for B. taylorii, which enables the effector translocation via the VirB/VirD4 T4SS, we used the BepD-dependent STAT3 phosphorylation as sensitive readout.
We infected JAWS II cells with B. taylorii IBS296 SmR, used as wild-type strain, and the T4S-deficient ΔvirD4 mutant. Corresponding B. henselae strains served as controls. The bacteria were grown on Columbia blood agar (CBA) plates, and the VirB/VirD4 system was induced by overnight culturing in M199 + 10% FCS (Quebatte et al., 2013; Sorg et al., 2020). We performed time-course experiments to compare the STAT3 phosphorylation at early time-points after infection. We quantified the phosphorylated STAT3 over the total STAT3. Cells infected for 24 h with the translocation-deficient ΔvirD4 mutants served as controls and did not show enhanced STAT3 activation. B. henselae wild-type triggered STAT3 phosphorylation 1 h post-infection (hpi). However, B. taylorii did not induce STAT3 phosphorylation in JAWS II cells within 6 hpi (Figures 1A,B). STAT3 phosphorylation was observed only after 24 h in cells infected with B. taylorii (Supplementary Figure S1B). Since BepD of B. taylorii triggered the STAT3 phosphorylation at early time points when expressed in B. henselae (Supplementary Figure S1A), we speculated that other than described for the B. henselae VirB/VirD4 T4SS, the one of B. taylorii was not induced during the first hours of infection. To test whether the induction of VirB/VirD4 T4SS can be further enhanced, we optimized the culture conditions. In previous studies, different Bartonella species isolated from their natural hosts were cultured on tryptic soy agar (TSA) plates instead of CBA (Li et al., 2015; Stepanic et al., 2019). Compared to the ΔvirD4 mutant, B. taylorii wild-type harvested from TSA plates induced STAT3 phosphorylation already after 3 hpi. At 6 hpi cells infected with B. taylorii showed STAT3 phosphorylation to comparable levels as triggered by B. henselae grown on CBA (Figures 1C,D).
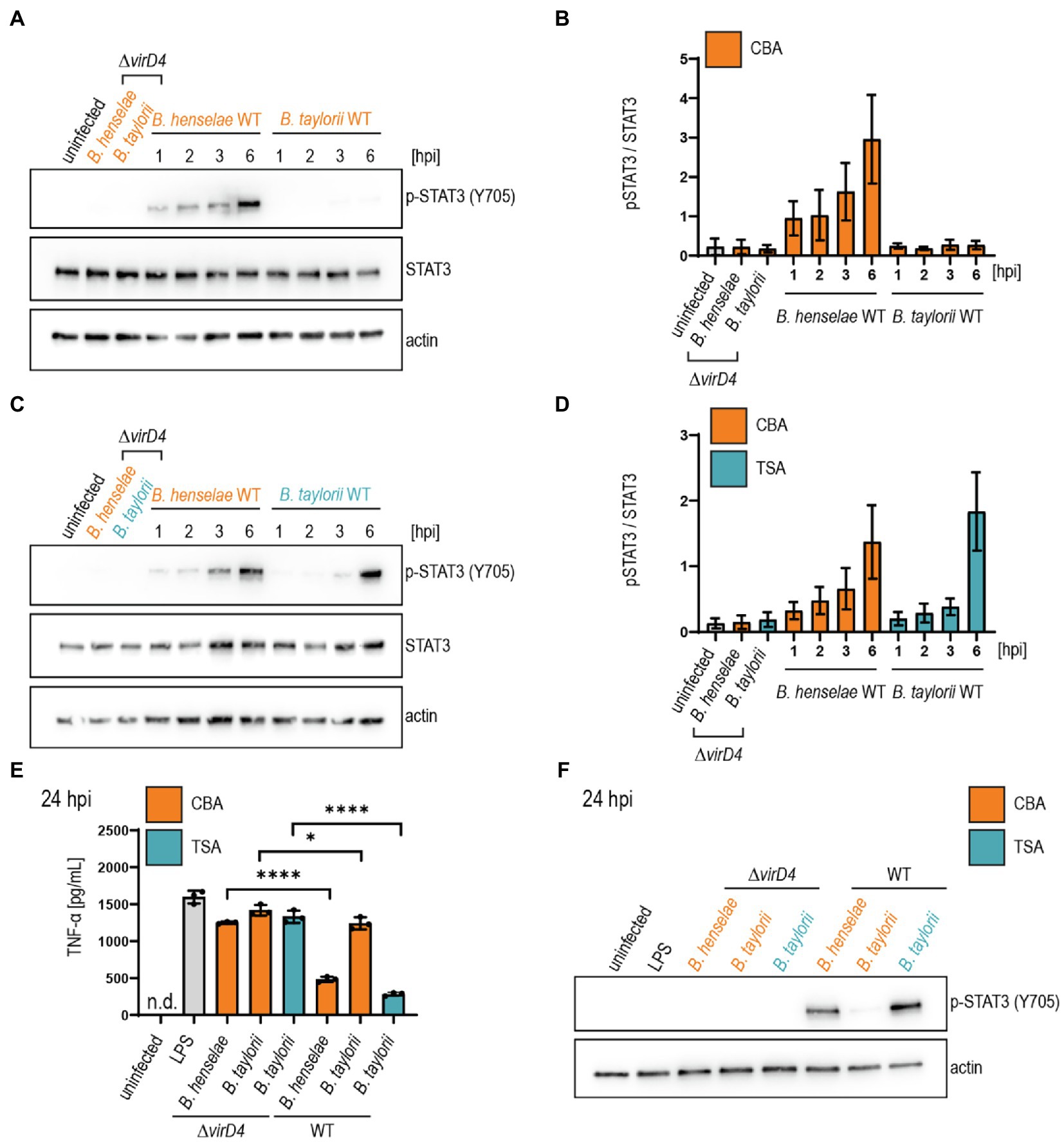
Figure 1. Bartonella taylorii grown on TSA trigger a stronger STAT3 activation compared to bacteria grown on CBA. JAWS II cells were infected at MOI 50. At the depicted time points, cells were harvested and analyzed by immunoblot with specific antibodies against p-STAT3 (Y705), STAT3, and actin. Phosphorylated STAT3 was quantified over the total amount of STAT3. (A) Shown is an immunoblot of JAWS II cell lysates infected with Bartonella henselae wild-type or ΔvirD4, B. taylorii wild-type or ΔvirD4 grown on CBA (orange). Cells were infected for 24 h with ΔvirD4 mutants. (B) Quantification of three independent immunoblots as shown in (A). (C) Shown is an immunoblot of JAWS II cell lysates infected with B. henselae wild-type or ΔvirD4, B. taylorii wild-type or ΔvirD4 grown on TSA (blue). Cells were infected for 24 h with ΔvirD4 mutants. (D) Quantification of three independent immunoblots as shown in (C). (E) JAWS II dendritic cells were infected as described in (A). During the last 2 h of infection, cells were treated with 100 ng/ml LPS. After 24 hpi TNF-α concentration in the cell culture supernatant was assessed by ELISA. (F) Cells in (E) were analyzed by Western Blot for phosphorylated STAT3 (Y705). Actin was used as loading control. Data for immunoblots were acquired by pooling three technical replicates. Data from one representative experiment (n = 3) are presented. Data were analyzed using one-way ANOVA with multiple comparisons (Tukey’s multiple comparison test), *p < 0.05 and ****p < 0.0001.
To exclude potential influence on the STAT3 phosphorylation mediated by other effectors present in B. taylorii, we infected JAWS II cells with mutants lacking single bep genes. Bartonella taylorii encodes five Beps, namely BepA-BepI. BepA, BepC, and BepI harbor an N-terminal FIC (filamentation induced by cyclic AMP) domain. BepD and BepF contain EPIYA-related motifs in their N-terminus (Supplementary Figure S1C). However, BepDBta is the only effector, which induces STAT3 phosphorylation in infected JAWS II cells (Supplementary Figure S1D).
Next, we tested if B. taylorii downregulates the secretion of pro-inflammatory cytokines in a VirB/VirD4 T4SS-dependent manner as previously published for B. henselae (Sorg et al., 2020). We infected JAWS II for 6 or 24 h with B. taylorii wild-type and the ΔvirD4 mutant. Corresponding B. henselae strains served as controls. During the last 2 h of infection, JAWS II cells were co-stimulated with E. coli LPS as potent TLR4 ligand to increase TNF-α secretion (Sorg et al., 2020). Cells infected with B. henselae wild-type secreted significantly lower TNF-α concentrations compared to the ΔvirD4 mutant at 6 and 24 hpi. While no discernable inhibitory effect was displayed 6 hpi, B. taylorii recovered from CBA plates impaired TNF-α secretion at 24 hpi in a VirD4-dependent manner. Surprisingly, bacteria recovered from TSA plates did not impair the TNF-α secretion after 6 hpi, although the STAT3 phosphorylation was induced (Supplementary Figures S1E,F). At 24 hpi, B. taylorii cultured on TSA reduced the TNF-α secretion much more efficiently compared to bacteria grown on CBA plates (Figures 1E,F).
The in vitro infection protocol devised for B. henselae is not transferable to B. taylorii. STAT3 phosphorylation and reduced TNF-α secretion was observed 6 hpi in cells infected with B. henselae. In contrast, the BepD-dependent phenotype in cells infected with B. taylorii grown on CBA was only observed 24 hpi. To improve the effector translocation, we cultured B. taylorii on TSA plates prior to cell infection. These bacteria induced STAT3 phosphorylation already after 3 hpi, but did not reduce the TNF-α concentration in the supernatant of infected JAWS II cells after 6 hpi. To facilitate a more efficient downregulation of the innate immune response, we aimed to further modify the bacterial culture conditions.
Temperature Shift Prior to Infection Increases Effector Translocation
We aimed at improving the effector translocation via the VirB/VirD4 T4SS of B. taylorii. Bartonellae are transmitted via blood-sucking arthropods and experience a temperature shift during their infection cycle from ambient temperature of the arthropod vector to warm-blooded body temperature of the mammalian hosts. Bartonella virulence factors have been shown to undergo differential regulation in response to temperature (Abromaitis and Koehler, 2013; Tu et al., 2016). To assess the influence of the temperature on the translocation efficiency, we cultivated the bacteria at 28°C or 35°C in M199 + 10% FCS before infection.
We investigated the influence of decreased temperature in B. taylorii overnight cultures on the BepD-dependent STAT3 activation in RAW macrophages. Different studies already demonstrated successful infection of murine macrophage cell lines by B. henselae (Musso et al., 2001; Sorg et al., 2020). While infection with the translocation-deficient ΔvirD4 mutant or the bacteria lacking bepD did not trigger STAT3 activation, we found higher levels of phosphorylated STAT3 at 3 hpi if B. taylorii wild-type was cultured at 28°C. The amount of pSTAT3 was quantified over STAT3 (Figures 2A,B). A similar phenotype was also observed 6 hpi (Supplementary Figures S2A,B), although less prominently. This might indicate that BepD translocation is improved at earlier time points if bacteria are cultured at lower temperatures. The incubation at 28°C prior to infection triggers a stronger STAT3 phosphorylation in vitro, which seems to correlate with a higher translocation of BepD into host cells.
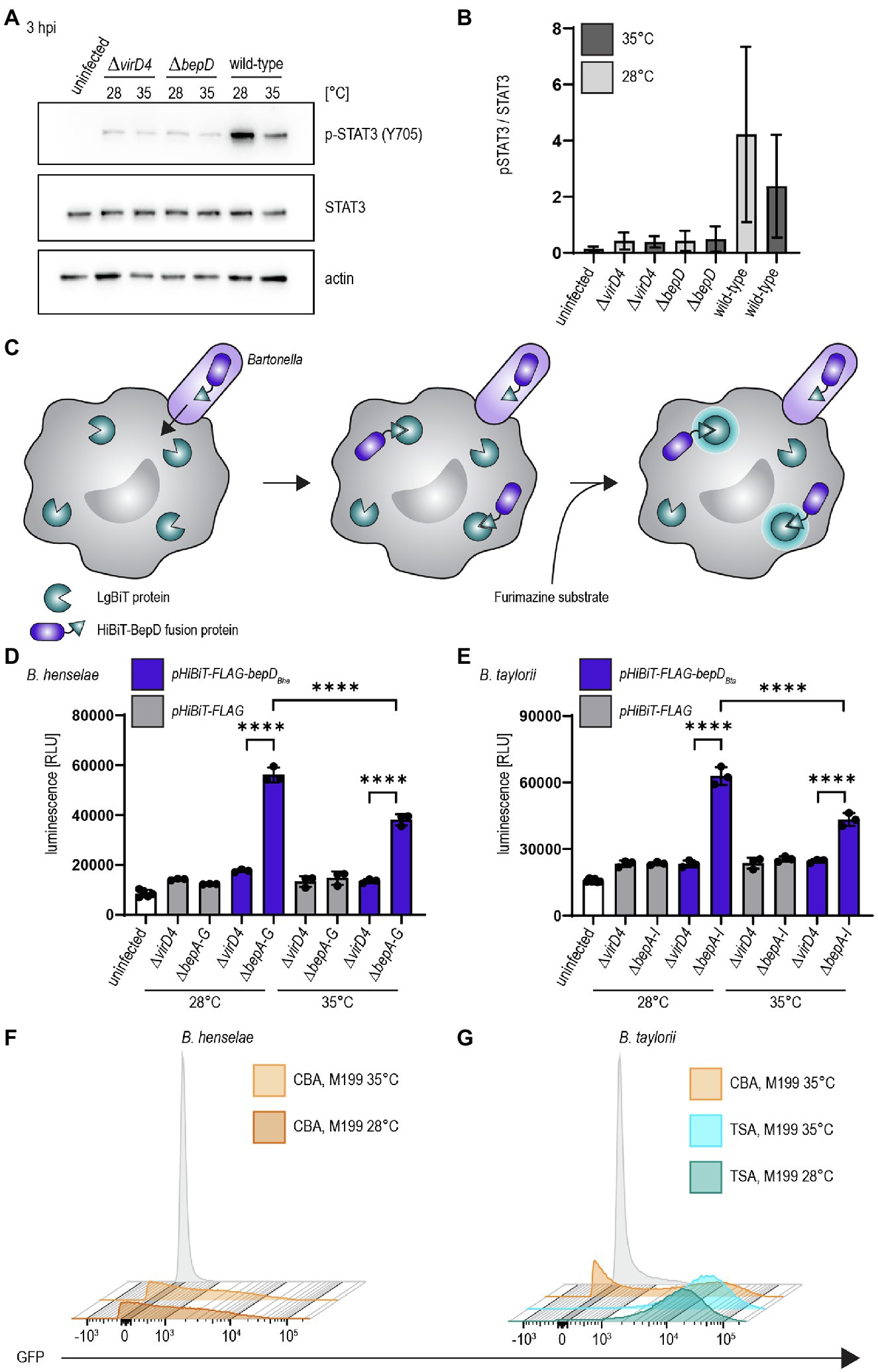
Figure 2. Temperature shift increases effector translocation (A) Bacteria were cultured in M199 + 10% FCS at 28°C or 35°C for 24 h prior to infection. RAW 264.7 macrophages were infected for 3 h at MOI 50 with B. taylorii wild-type, the BepD-deficient mutant ΔbepD or the translocation-deficient mutant ΔvirD4. Cell lysates were analyzed by Western Blot for phosphorylated STAT3 (Y705), STAT3, and actin. Shown is an immunoblot of RAW cell lysates infected for 3 h with bacteria grown at 28°C or 35°C. (B) Quantification of pSTAT3 signal over STAT3 control of three independent immunoblots as shown in (A). Bacteria were grown at 28°C (light grey) or 35°C (dark grey). (C) Schematic overview showing the split NLuc assay principle. Bacteria were allowed to infect RAW LgBiT macrophages for 24 h. HiBiT-BepD was translocated inside host cells via the VirB/VirD4 T4SS. The substrate Furimazine was added and luminescence measured. (D) Bacteria were cultured in M199 + 10% FCS for 24 h at 28°C or 35°C prior to infection. RAW LgBiT macrophages were infected at MOI 50 for 24 h with B. henselae ΔbepA-G or ΔvirD4 containing pHiBiT-bepDBhe (blue) or pHiBiT (grey). Luminescence of the complemented split NLuc was measured. (E) RAW LgBiT were infected for 24 h with B. taylorii ΔbepA-I or ΔvirD4 containing pHiBiT-bepDBta (blue) or the control pHiBiT-FLAG (grey) either cultured in M199 + 10% FCS at 28°C or 35°C for 24 h prior to infection. Luminescence of the complemented split NLuc was measured. (F,G) Bartonella henselae or B. taylorii expressing GFP under the corresponding virB2 promoter on a plasmid were grown on CBA (orange) or TSA (blue) plates and cultured for 24 h in M199 + 10% FCS at 28°C or 35°C. GFP expression was analyzed by FACS measurement. Bacteria containing the empty plasmid (pCD366, grey) were used as control. Data for immunoblots were acquired by pooling three technical replicates. All experiments were performed in three independent biological replicates. Data were analyzed using one-way ANOVA with multiple comparisons (Tukey’s multiple comparison test), ****p < 0.0001.
As a second read-out to study effector translocation under various conditions, we implemented the split NanoLuc (NLuc) luciferase-based translocation assay for the VirB/VirD4 T4SS in Bartonella. This assay was developed to assess effector injection via the type III secretion system of Salmonella enterica serovar Typhimurium in HeLa cells (Westerhausen et al., 2020). Split NLuc is composed of a small fragment (HiBiT, 1.3 kDa), which is fused to the bacterial effectors, and a larger fragment (LgBiT, 18 kDa), which is stably expressed in RAW264.7 macrophages (RAW LgBiT). Complementation of LgBiT with HiBiT to a functional luciferase should only occur if host cells were infected with Bartonella strains harboring a functional T4SS. The luciferase converts the substrate furimazine into a bioluminescent signal (Figure 2C). We already showed that BepD of B. taylorii is triggering STAT3 phosphorylation in eukaryotic host cells (Supplementary Figure S1D). Therefore, we designed fusion proteins harboring HiBiT and a triple-FLAG epitope tag at the N-terminus of BepDBhe or BepDBta that are expressed under the control of an IPTG-inducible promoter. The fusion proteins were ectopically expressed in the translocation-deficient ΔvirD4 mutants or the Bep-deficient mutant of B. henselae (ΔbepA-G) or B. taylorii (ΔbepA-I). As control, bacteria expressing only the HiBiT-FLAG fragment were created. The expression of pHiBiT-FLAG-bepDBhe and pHiBiT-FLAG-bepDBta after 1 h IPTG-induction was confirmed by immunoblotting using the triple-FLAG epitope tag in bacterial lysates (Supplementary Figures S2C,D). We could not detect the HiBiT-FLAG fragment (estimated mass 4.3 kDa), most likely due to the low molecular mass and possible degradation. We also tested whether the HiBiT-FLAG fragment, HiBiT-FLAG-BepDBhe, and HiBiT-FLAG-BepDBta can complement LgBiT to a functional luciferase. We could detect high luminescent signals of lysed bacteria expressing either HiBiT-FLAG-BepDBhe or HiBiT-FLAG-BepDBta. Expression of the HiBiT-FLAG fragment also lead to detectable albeit lower luminescent signal (Supplementary Figures S2E,F).
To estimate the translocation of the HiBiT fused effectors, we infected RAW LgBiT macrophages for 24 h. We observed increased luminescent signals inside host cells after infection with B. henselae ΔbepA-G pHiBiT-FLAG-bepDBhe (Supplementary Figure S2G) or B. taylorii ΔbepA-I pHiBiT-FLAG-bepDBta (Supplementary Figure S2H), which increased in a MOI-dependent manner. With MOI 50 or higher, significantly increased signals compared to the translocation-deficient mutants were observed for B. henselae and B. taylorii infection. Therefore, the following experiments were performed at MOI 50.
RAW LgBiT macrophages were infected at MOI 50 at 37°C for 24 h. We observed significant higher luminescence compared to the ΔvirD4 mutants if cells were infected with B. henselae ΔbepA-G pHiBiT-bepDBhe (Figure 2D) or B. taylorii ΔbepA-I pHiBiT-bepDBta (Figure 2E). The luminescent signals significantly increased if bacteria were cultured at 28°C prior to infection instead of 35°C for both Bartonella strains (Figures 2D,E).
By adapting the bacterial culture conditions for B. taylorii, changing from CBA to TSA plates and incubating the bacteria in M199 + 10% FCS at 28°C instead of 35°C, the BepD-dependent STAT3 phosphorylation in infected eukaryotic cells was observed after shorter time period. Further, we observed increased luminescent signals in RAW LgBiT macrophages infected with Bartonellae that were cultured at lower temperature prior to infection.
Increased Effector Translocation Correlates With Upregulated Expression of the VirB/VirD4 T4SS
Changing the agar plates from CBA to TSA and incubating the overnight culture in M199 at 28°C instead of 35°C markedly improved the STAT3 activation and the luminescent signal in the translocation assay in infected RAW macrophages. Next, we tested whether the enhanced downregulation of the innate immune response after infection with B. taylorii recovered from TSA correlates with an upregulation of the VirB/VirD4 T4SS. We generated reporter strains expressing GFP under the control of the virB2 promoter (PvirB2) of B. henselae or B. taylorii, driving expression of the virB operon. The expression of the GFP promoter fusions in Bartonella carrying the pCD366-derived reporter plasmids was probed using flow cytometry. As shown previously for B. henselae (Quebatte et al., 2010; Harms et al., 2017), only part of the B. taylorii population grown on CBA expressed GFP. In comparison, B. taylorii cultured on TSA displayed higher GFP-fluorescence, indicating that these bacteria upregulate expression of the VirB/VirD4 T4SS. However, the incubation at lower temperature did not significantly change the GFP expression (Figures 2F,G). The VirB/VirD4 T4SS expression appears to be similar compared to bacteria cultured at 35°C, although we observed increased effector translocation when the bacteria were cultured at lower temperature prior to infection.
Our adapted culture conditions established for B. taylorii facilitate an earlier STAT3 phosphorylation in infected cells. This phenotype correlates with an increased expression of the VirB/VirD4 T4SS. Therefore, we tested whether the improved culture conditions (Figure 3A) also had an impact on the TNF-α secretion after infection by B. taylorii at early time points. Thus, we infected RAW macrophages with B. taylorii wild-type, the Bep-deficient mutant (ΔbepA-I), a strain lacking only BepD (ΔbepD) or the translocation-deficient mutant ΔvirD4. The BepD-dependent STAT3 phosphorylation was observed in cells infected with the wild-type at 6 hpi. The mutants lacking BepD or VirD4 did not trigger increased STAT3 activation (Figures 3B,C). Compared to wild-type infections, cells infected with the ΔvirD4 mutant secreted significantly higher levels of TNF-α secretion. Surprisingly, the TNF-α levels of cells infected with ΔbepD and ΔbepA-I strains were lowered to similar extent as in wild-type infections (Figure 3D). Twenty hours after infection, macrophages infected with the ΔbepD and ΔbepA-I mutants showed elevated TNF-α secretion compared to wild-type infected cells. However, cells infected with the translocation-deficient ΔvirD4 mutant secreted significantly higher levels of TNF-α compared to both Δbep mutants (Figure 3G), although these strains are unable to trigger STAT3 activation to the same extent as the wild-type (Figures 3E,F).
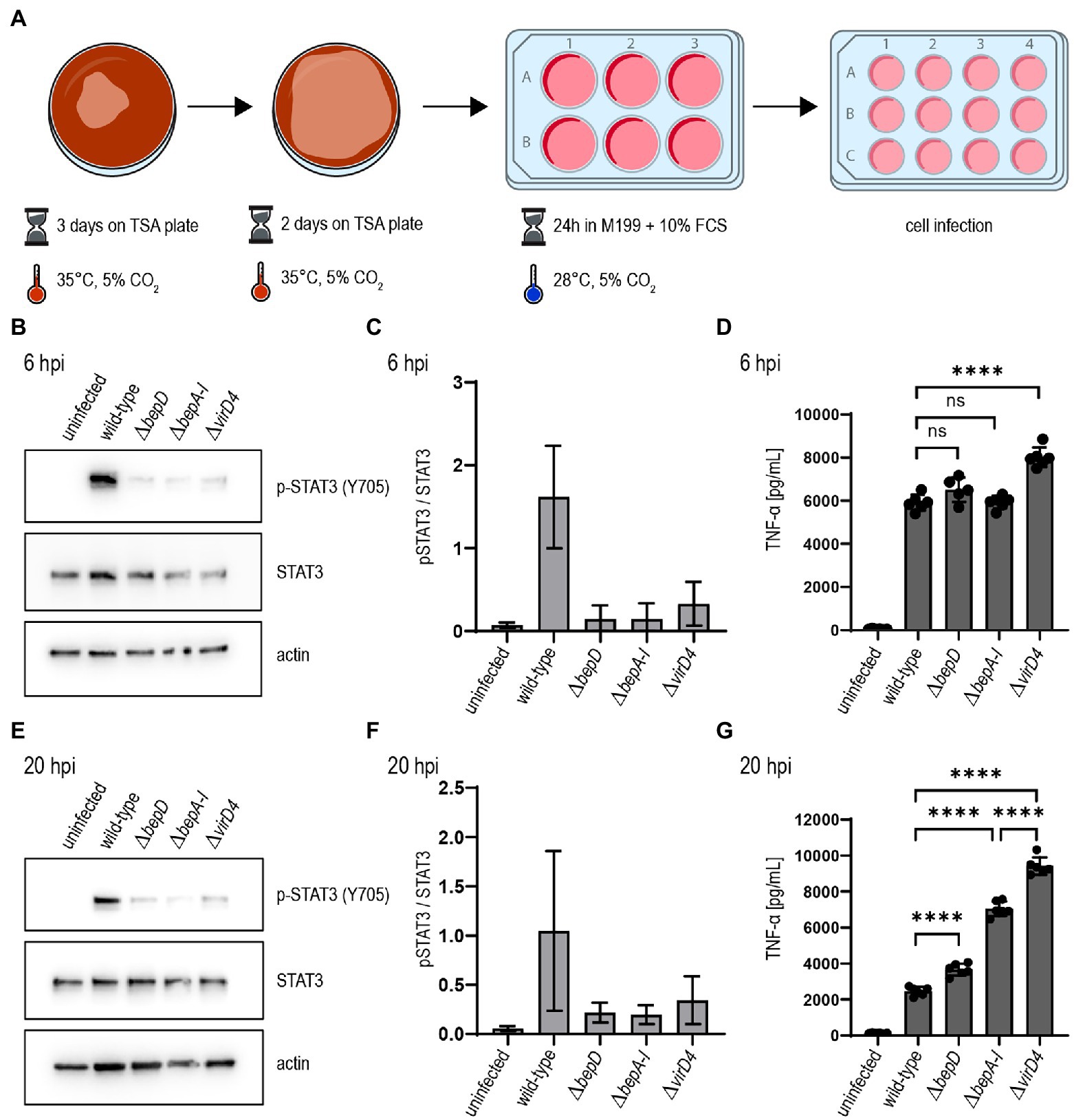
Figure 3. Bartonella taylorii efficiently downregulates the innate immune response using the novel in vitro infection protocol. (A) Scheme of B. taylorii culture conditions used for infection. (B–G) RAW 264.7 macrophages were infected at MOI 50 with B. taylorii wild-type, the ΔbepD or ΔbepA-I mutants or the translocation-deficient mutant ΔvirD4. Secreted TNF-α was quantified by ELISA. Cells were harvested, lysed, and analyzed by immunoblot using specific antibodies against p-STAT3 (Y705), STAT3, and actin. (B) Immunoblot of cellular lysates after 6h infection. (C) Quantification of pSTAT3 signal over STAT3 control of three independent immunoblots as shown in (B). (D) TNF-α secreted by cells in (B) was quantified by ELISA. (E) Immunoblot of RAW macrophages infected for 20 h. (F) Quantification of pSTAT3 signal over STAT3 control of three independent immunoblots as shown in (E). (G) TNF-α secreted by cells in (E) was quantified by ELISA. Data for immunoblots were acquired by pooling three technical replicates. Data representative for three independent biological replicates. Data were analyzed using one-way ANOVA with multiple comparisons (Tukey’s multiple comparison test), ns, not significant, ****p < 0.0001.
Our data provides evidence that the cultivation of B. taylorii prior to infection determines its capacity to dampen the innate immune response in vitro. We could correlate this phenotype to an increased expression of the VirB/VirD4 T4SS if bacteria are cultured on TSA plates. The lowered temperature seems to not affect the expression of the T4SS, but we observed a more efficient effector translocation.
Bacterial Culture Conditions Optimized for VirB/VirD4 Expression Correlate With High Infectivity in the Mouse Model
Finally, we tested whether our adapted growth conditions also influence B. taylorii in vivo infections. Previous studies described that mice inoculated with 107 colony forming units (cfu) bacteria remained abacteremic until 5–7 days post-infection (dpi). The infection peaked around day 12–14 with approximately 105–106 bacteria per ml blood. The bacteremia is cleared within 50 days (Siewert et al., 2022), displaying similar kinetics compared to the infection of rats with B. tribocorum (Okujava et al., 2014). We compared the course of bacteremia in wild-type C57BL/6 mice inoculated with different cfus of B. taylorii cultured on CBA or TSA plates. Bacteria cultured on TSA plates caused bacteremia independent of the amount of inoculated bacteria, leading to infection rates of 100% even with an inoculum of 102. Bacteria grown on CBA plates showed reduced infectivity (Figure 4A). Interestingly, if the bacteria were able to invade the blood stream, the bacteremia kinetics were similar independent on the growth conditions and inoculum (Figures 4B–D).
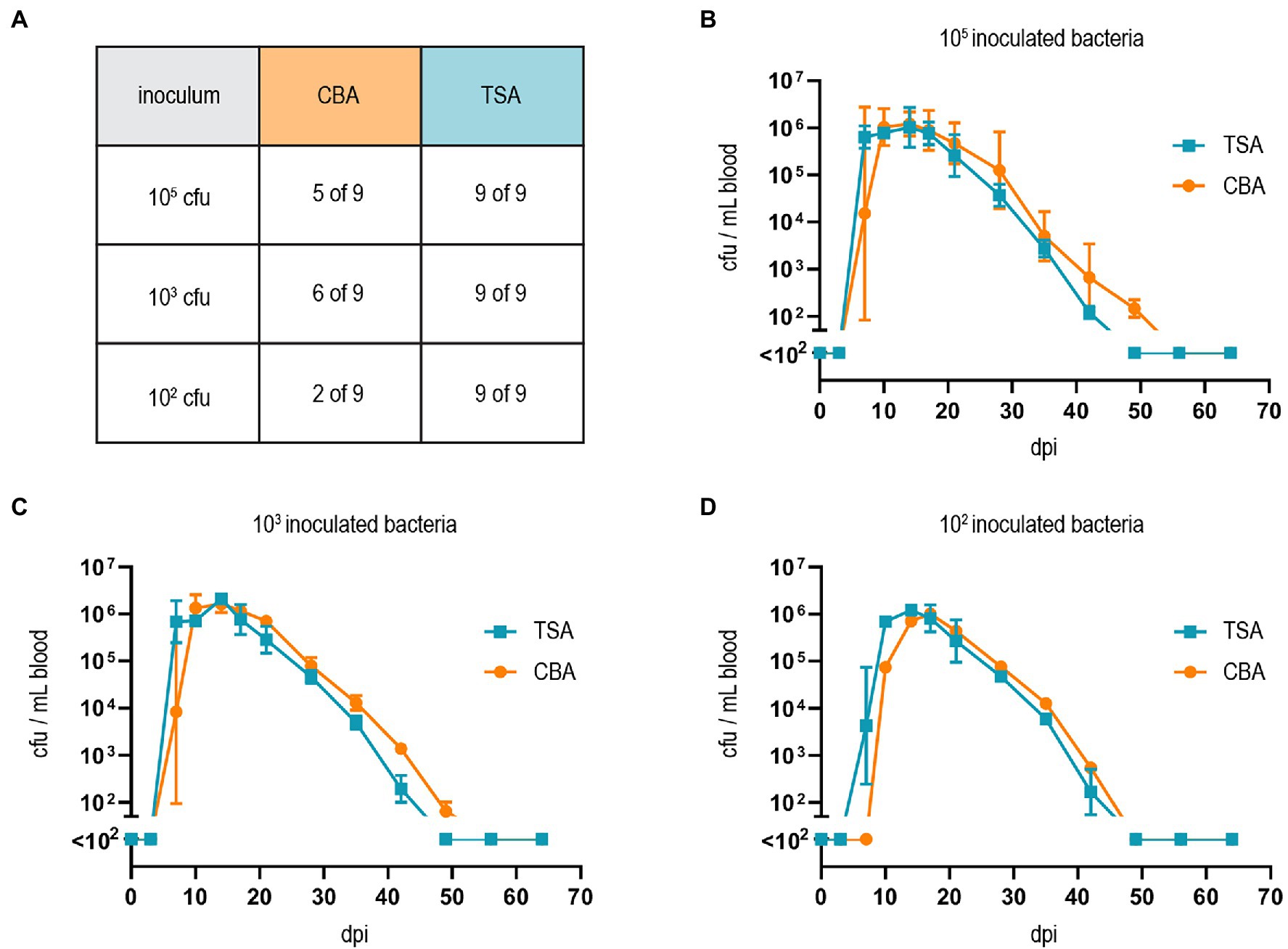
Figure 4. Growth on TSA primes B. taylorii for high infectivity in mice. (A) C57BL/6 mice were infected i.d. with 102, 103, or 105 cfu of B. taylorii wild-type either grown on CBA (orange) or TSA (blue) plates. At several time points after infection, blood was drawn from the tail vein and plated on CBA plates to assess the amount of bacteria inside the blood. Table represents number of mice developing bacteremia vs. mice remaining abacteremic after infection with B. taylorii for nine animals per condition. Bacteremia kinetics (cfu/ml blood) in infected mice shown for (B) 105 (CBA 3 of 3 infected mice bacteremic; TSA 3 of 3 infected mice bacteremic), (C) 103 (CBA 3 of 3 infected mice bacteremic; TSA 3 of 3 infected mice bacteremic) and (D) 102 (CBA 1 of 3 infected mice bacteremic; TSA 3 of 3 infected mice bacteremic). Symbols represent the mean ± SD. (A) Shows pooled data from three independent experiments and (B–D) show representative data from three independent experiments.
Discussion
Typically, in vitro studies focusing on the effector translocation via the VirB/VirD4 T4SS of Bartonella were conducted with B. henselae. In contrast, in vivo studies were mostly performed with rodent-specific strains, while in vitro infection protocols for those bacteria were missing. Our study characterized the mouse-specific B. taylorii strain IBS296 as a suitable model to study effector translocation in vitro. The main advantage over previously described species is the use of a murine infection model (Siewert et al., 2022), allowing robust and easy to perform in vitro and in vivo studies with the same strain.
Growing bacteria on TSA allowed a drastic reduction of the inoculum in vivo while the infection rate in C57BL/6 mice remained unchanged. Compared to high-dose infections (107 cfu; Siewert et al., 2022), bacteremia kinetics were similar in onset, duration, and clearance if mice were infected with 102 cfu. The lower infection dose might better reflect the natural infection via bacteria-containing feces of the arthropod vector scratched into the skin. In experimentally infected fleas, the amount of bacteria within their feces peaked with an average of 9 × 104 bacteria, which drastically decreased several days after infection (Bouhsira et al., 2013). Thus, we consider an infection with 102–104 bacteria as physiological.
We introduced the split NLuc translocation assay as a tool to study effector translocation into host cells via the VirB/VirD4 T4SS. The split NLuc translocation assay had previously been described for studying protein trafficking in mammalian cells (Rouault et al., 2017) and protein secretion in Gram-positive bacteria (Wang et al., 2018). Protein secretion to the periplasm via the Sec system or into host cells via T3SS was shown by utilizing similar approaches in Gram-negative bacteria (Pereira et al., 2019; Westerhausen et al., 2020). Adopting this translocation assay for studying effector translocation via the VirB/VirD4 T4SS in B. taylorii, we could show that incubating the bacteria at 28°C instead of 35°C prior to infection improved effector translocation. Additionally, we observed increased STAT3 activation in response to effector translocation when cells were infected with bacteria cultured at lower temperatures. Surprisingly, the lowered temperature in the bacterial overnight cultures seems to not affect the expression of the VirB/VirD4 T4SS indicating that there might be other regulation mechanisms involved, e.g., the effector expression or assembly of the translocation machinery. Temperature shift from ambient temperature to 37°C has been shown to regulate virulence factors in several pathogens, such as Salmonella, Shigella, and Yersinia (Cornelis et al., 1987; Prosseda et al., 1998; Shapiro and Cowen, 2012; Lam et al., 2014). In the human-specific pathogen B. quintana the alternative sigma factor RpoE is upregulated at lower temperature and high haemin concentrations (Abromaitis and Koehler, 2013). These conditions recapitulate the environment of the arthropod gut and support a role in the adaptation to the vector. In B. henselae, RpoE was also found to negatively regulate the transcription of badA, which encodes the BadA adhesin (Tu et al., 2016). BadA is an important virulence factor that interacts with endothelial cells in the mammalian host (Riess et al., 2004). In contrast, BadA was described to negatively affect the function of the VirB/VirD4 T4SS (Lu et al., 2013). No evidence was reported that RpoE has direct effects on the virB operon as neither the genomic deletion nor ectopic overexpression did influence virB promoter activity (Quebatte et al., 2013). However, we cannot exclude that RpoE might affect the function of the VirB/VirD4 T4SS via other regulation mechanisms. We observed upregulated expression of the VirB/VirD4 T4SS in B. taylorii cultured on TSA, which might influence the expression of BadA, therefore allowing to monitor effector translocation in vitro.
The expression and activation of the VirB/VirD4 T4SS in B. henselae is regulated by the alternative sigma factor RpoH1. Levels of RpoH1 are under the control of the stringent response, an adaptive mechanism that allows pathogens to respond to changes in the microenvironments (Dalebroux et al., 2010; Quebatte et al., 2013). Quebatte et al. showed that the stringent response components SpoT and DksA are key regulators of B. henselae virulence by controlling the levels of RpoH1 (Quebatte et al., 2013). Additionally, induction of the VirB/VirD4 T4SS also requires an active BatR/BatS two-component system (TCS). The BatR/BatS TCS is activated in neutral pH range (pH 7.0–7.8) suggesting that this system is discriminating between the mammalian host (neutral pH in blood and most tissues) and the midgut of the arthropod vector (alkaline pH; Quebatte et al., 2010). Genes of the BatR regulon and the SpoT/DksA/RpoH1 are conserved among Bartonellae (Quebatte et al., 2010, 2013), indicating that the expression of the VirB/VirD4 T4SS relies on the same pathways in various Bartonella species. However, environmental signals and nutritional states triggering these pathways are not fully understood. We showed that the activation of the VirB/VirD4 T4SS in B. taylorii required different culture conditions compared to B. henselae. It is thus tempting to speculate that B. taylorii may have different metabolic capabilities as B. henselae resulting in the observed differences in VirB/VirD4 regulation.
We demonstrated that B. taylorii lacking all Beps impaired the TNF-α secretion to a larger extent than the effector translocation-deficient ΔvirD4 mutant. The TNF-α secretion is likewise impaired following infection with the ΔbepD and the full Bep deletion mutant ΔbepA-I at 6 hpi, but significantly increased in cells infected with the ΔvirD4 mutant. Furthermore, we saw differences in the TNF-α secretion when comparing ΔbepA-I and ΔvirD4 mutant at later time points. This observation suggests that some Bartonella species harbor at least one additional effector that is translocated via the VirB/VirD4 T4SS. All Beps harbor a bipartite secretion signal composed of one or several Bep intracellular delivery (BID) domains followed by a positively charged tail sequence. It was shown that complete or partial deletion of the BID domain strongly impairs the secretion via the VirB/VirD4 T4SS (Schulein et al., 2005; Schmid et al., 2006b). However, other bacteria expressing a homologous T4SS, like Agrobacterium tumefaciens, secrete effector proteins without BID domains. Positively charged amino acids in the C-terminus of recognized effectors serve as translocation signal. For example, the last C-terminal 20 amino acids of VirF of A. tumefaciens are sufficient to serve as translocation signal (Vergunst et al., 2005). Here we provide data suggesting that B. taylorii might harbor other effector proteins probably lacking BID domains, which might be translocated by the VirB/VirD4 T4SS. We suggest to employ the NLuc translocation assay to study the translocation of putative new effectors in Bartonella as this system provides high signal-to-noise ratios and is easy to perform.
Taken together, this study characterized B. taylorii IBS296 as suitable model organism allowing for the first time to directly compare host-pathogen interaction in vitro and in rodent hosts using the same Bartonella species.
Data Availability Statement
The original contributions presented in the study are included in the article/Supplementary Material; further inquiries can be directed to the corresponding author.
Ethics Statement
The animal study was reviewed and approved by the Veterinary Office of the Canton Basel City.
Author Contributions
KF and CD conceptualized the studies and designed all experiments. KF designed the figures. KF performed cell infections, Nano-Luc translocation assay, animal work, flow cytometry, cloning, western blotting, and ELISA. AB performed cell infections, western blotting, and ELISA. MO contributed to Nano-Luc translocation assay and cloning. AW and SM performed cloning of plasmids. EB generated the LgBiT RAW macrophages. SW contributed to the experimental design. KF conducted the experiments and collected and analysed the data. KF and CD wrote the manuscript. All authors contributed to the article and approved the submitted version.
Funding
This work was supported by the Swiss National Science Foundation (SNSF, www.snf.ch) grant 310030B_201273 to CD and a “Fellowship for Excellence” by the Werner Siemens-Foundation to KF.
Conflict of Interest
AW was employed by company Maucher Jenkins, Germany.
The remaining authors declare that the research was conducted in the absence of any commercial or financial relationships that could be construed as a potential conflict of interest.
Publisher’s Note
All claims expressed in this article are solely those of the authors and do not necessarily represent those of their affiliated organizations, or those of the publisher, the editors and the reviewers. Any product that may be evaluated in this article, or claim that may be made by its manufacturer, is not guaranteed or endorsed by the publisher.
Acknowledgments
We specially want to thank Lena Siewert, Jaroslaw Sedzicki, and Maxime Québatte for helpful comments and critical reading of the manuscript.
Supplementary Material
The Supplementary Material for this article can be found online at: https://www.frontiersin.org/articles/10.3389/fmicb.2022.913434/full#supplementary-material
References
Abromaitis, S., and Koehler, J. E. (2013). The Bartonella quintana extracytoplasmic function sigma factor RpoE has a role in bacterial adaptation to the arthropod vector environment. J. Bacteriol. 195, 2662–2674. doi: 10.1128/JB.01972-12
Berge, C., Waksman, G., and Terradot, L. (2017). Structural and molecular biology of type IV secretion systems. Curr. Top. Microbiol. Immunol. 413, 31–60. doi: 10.1007/978-3-319-75241-9_2
Bouhsira, E., Franc, M., Boulouis, H. J., Jacquiet, P., Raymond-Letron, I., and Lienard, E. (2013). Assessment of persistence of Bartonella henselae in Ctenocephalides felis. Appl. Environ. Microbiol. 79, 7439–7444. doi: 10.1128/AEM.02598-13
Boulouis, H. J., Barrat, F., Bermond, D., Bernex, F., Thibault, D., Heller, R., et al. (2001). Kinetics of Bartonella birtlesii infection in experimentally infected mice and pathogenic effect on reproductive functions. Infect. Immun. 69, 5313–5317. doi: 10.1128/iai.69.9.5313-5317.2001
Chomel, B. B., Boulouis, H. J., Breitschwerdt, E. B., Kasten, R. W., Vayssier-Taussat, M., Birtles, R. J., et al. (2009). Ecological fitness and strategies of adaptation of Bartonella species to their hosts and vectors. Vet. Res. 40:29. doi: 10.1051/vetres/2009011
Chomel, B. B., and Kasten, R. W. (2010). Bartonellosis, an increasingly recognized zoonosis. J. Appl. Microbiol. 109, 743–750. doi: 10.1111/j.1365-2672.2010.04679.x
Chomel, B. B., Kasten, R. W., Floyd-Hawkins, K., Chi, B., Yamamoto, K., Roberts-Wilson, J., et al. (1996). Experimental transmission of Bartonella henselae by the cat flea. J. Clin. Microbiol. 34, 1952–1956. doi: 10.1128/JCM.34.8.1952-1956.1996
Cornelis, G., Vanootegem, J. C., and Sluiters, C. (1987). Transcription of the yop regulon from Y. enterocolitica requires trans acting pYV and chromosomal genes. Microb. Pathog. 2, 367–379. doi: 10.1016/0882-4010(87)90078-7
Dalebroux, Z. D., Svensson, S. L., Gaynor, E. C., and Swanson, M. S. (2010). ppGpp conjures bacterial virulence. Microbiol. Mol. Biol. Rev. 74, 171–199. doi: 10.1128/MMBR.00046-09
Deng, H., Pang, Q., Xia, H., Le Rhun, D., Le Naour, E., Yang, C., et al. (2016). Identification and functional analysis of invasion associated locus B (IalB) in Bartonella species. Microb. Pathog. 98, 171–177. doi: 10.1016/j.micpath.2016.05.007
Foil, L., Andress, E., Freeland, R. L., Roy, A. F., Rutledge, R., Triche, P. C., et al. (1998). Experimental infection of domestic cats with Bartonella henselae by inoculation of Ctenocephalides felis (Siphonaptera: Pulicidae) feces. J. Med. Entomol. 35, 625–628. doi: 10.1093/jmedent/35.5.625
Fromm, K., and Dehio, C. (2021). The impact of Bartonella VirB/VirD4 type IV secretion system effectors on eukaryotic host cells. Front. Microbiol. 12:762582. doi: 10.3389/fmicb.2021.762582
Harms, A., and Dehio, C. (2012). Intruders below the radar: molecular pathogenesis of Bartonella spp. Clin. Microbiol. Rev. 25, 42–78. doi: 10.1128/CMR.05009-11
Harms, A., Segers, F. H., Quebatte, M., Mistl, C., Manfredi, P., Korner, J., et al. (2017). Evolutionary dynamics of pathoadaptation revealed by three independent acquisitions of the VirB/D4 type IV secretion system in Bartonella. Genome Biol. Evol. 9, 761–776. doi: 10.1093/gbe/evx042
Huarcaya, E., Maguina, C., Merello, J., Cok, J., Birtles, R., Infante, B., et al. (2002). A prospective study of cat-scratch disease in Lima-Peru. Rev. Inst. Med. Trop. Sao Paulo 44, 325–330. doi: 10.1590/s0036-46652002000600006
Jiang, X., Shen, C., Rey-Ladino, J., Yu, H., and Brunham, R. C. (2008). Characterization of murine dendritic cell line JAWS II and primary bone marrow-derived dendritic cells in chlamydia muridarum antigen presentation and induction of protective immunity. Infect. Immun. 76, 2392–2401. doi: 10.1128/IAI.01584-07
Khalfe, N., and Lin, D. (2022). Diagnosis and interpretation of testing for cat scratch disease. Proc. (Baylor Univ. Med. Cent.) 35, 68–69. doi: 10.1080/08998280.2021.1984791
Koesling, J., Aebischer, T., Falch, C., Schulein, R., and Dehio, C. (2001). Cutting edge: antibody-mediated cessation of hemotropic infection by the intraerythrocytic mouse pathogen Bartonella grahamii. J. Immunol. 167, 11–14. doi: 10.4049/jimmunol.167.1.11
Kunz, S., Oberle, K., Sander, A., Bogdan, C., and Schleicher, U. (2008). Lymphadenopathy in a novel mouse model of Bartonella-induced cat scratch disease results from lymphocyte immigration and proliferation and is regulated by interferon-alpha/beta. Am. J. Pathol. 172, 1005–1018. doi: 10.2353/ajpath.2008.070591
Lam, O., Wheeler, J., and Tang, C. M. (2014). Thermal control of virulence factors in bacteria: a hot topic. Virulence 5, 852–862. doi: 10.4161/21505594.2014.970949
Li, D.-M., Hou, Y., Song, X.-P., Fu, Y.-Q., Li, G.-C., Li, M., et al. (2015). High prevalence and genetic heterogeneity of rodent-borne Bartonella species on Heixiazi Island, China. Appl. Environ. Microbiol. 81, 7981–7992. doi: 10.1128/AEM.02041-15
Lu, Y. Y., Franz, B., Truttmann, M. C., Riess, T., Gay-Fraret, J., Faustmann, M., et al. (2013). Bartonella henselae trimeric autotransporter adhesin BadA expression interferes with effector translocation by the VirB/D4 type IV secretion system. Cell. Microbiol. 15, 759–778. doi: 10.1111/cmi.12070
Mada, P. K., Zulfiqar, H., and Joel Chandranesan, A. S. (2022). “Bartonellosis,” in StatPearls. ed. A. S. Joel Chandranesan (Treasure Island (FL): StatPearls Publishing LLC).
Maguina, C., Guerra, H., and Ventosilla, P. (2009). Bartonellosis. Clin. Dermatol. 27, 271–280. doi: 10.1016/j.clindermatol.2008.10.006
Marlaire, S., and Dehio, C. (2021). Bartonella effector protein C mediates actin stress fiber formation via recruitment of GEF-H1 to the plasma membrane. PLoS Pathog. 17:e1008548. doi: 10.1371/journal.ppat.1008548
McCord, A. M., Burgess, A. W., Whaley, M. J., and Anderson, B. E. (2005). Interaction of Bartonella henselae with endothelial cells promotes monocyte/macrophage chemoattractant protein 1 gene expression and protein production and triggers monocyte migration. Infect. Immun. 73, 5735–5742. doi: 10.1128/IAI.73.9.5735-5742.2005
Musso, T., Badolato, R., Ravarino, D., Stornello, S., Panzanelli, P., Merlino, C., et al. (2001). Interaction of Bartonella henselae with the murine macrophage cell line J774: infection and proinflammatory response. Infect. Immun. 69, 5974–5980. doi: 10.1128/IAI.69.10.5974-5980.2001
Okujava, R., Guye, P., Lu, Y. Y., Mistl, C., Polus, F., Vayssier-Taussat, M., et al. (2014). A translocated effector required for Bartonella dissemination from derma to blood safeguards migratory host cells from damage by co-translocated effectors. PLoS Pathog. 10:e1004187. doi: 10.1371/journal.ppat.1004187
Pereira, G. C., Allen, W. J., Watkins, D. W., Buddrus, L., Noone, D., Liu, X., et al. (2019). A high-resolution luminescent assay for rapid and continuous monitoring of protein translocation across biological membranes. J. Mol. Biol. 431, 1689–1699. doi: 10.1016/j.jmb.2019.03.007
Prosseda, G., Fradiani, P. A., Di Lorenzo, M., Falconi, M., Micheli, G., Casalino, M., et al. (1998). A role for H-NS in the regulation of the virF gene of Shigella and enteroinvasive Escherichia coli. Res. Microbiol. 149, 15–25. doi: 10.1016/s0923-2508(97)83619-4
Quebatte, M., Dehio, M., Tropel, D., Basler, A., Toller, I., Raddatz, G., et al. (2010). The BatR/BatS two-component regulatory system controls the adaptive response of Bartonella henselae during human endothelial cell infection. J. Bacteriol. 192, 3352–3367. doi: 10.1128/JB.01676-09
Quebatte, M., Dick, M. S., Kaever, V., Schmidt, A., and Dehio, C. (2013). Dual input control: activation of the Bartonella henselae VirB/D4 type IV secretion system by the stringent sigma factor RpoH1 and the BatR/BatS two-component system. Mol. Microbiol. 90, 756–775. doi: 10.1111/mmi.12396
Raschke, W. C., Baird, S., Ralph, P., and Nakoinz, I. (1978). Functional macrophage cell lines transformed by Abelson leukemia virus. Cell 15, 261–267. doi: 10.1016/0092-8674(78)90101-0
Regnath, T., Mielke, M. E., Arvand, M., and Hahn, H. (1998). Murine model of Bartonella henselae infection in the immunocompetent host. Infect. Immun. 66, 5534–5536. doi: 10.1128/IAI.66.11.5534-5536.1998
Riess, T., Andersson, S. G., Lupas, A., Schaller, M., Schafer, A., Kyme, P., et al. (2004). Bartonella adhesin a mediates a proangiogenic host cell response. J. Exp. Med. 200, 1267–1278. doi: 10.1084/jem.20040500
Rouault, A. A. J., Lee, A. A., and Sebag, J. A. (2017). Regions of MRAP2 required for the inhibition of orexin and prokineticin receptor signaling. Biochim. Biophys. Acta, Mol. Cell Res. 1864, 2322–2329. doi: 10.1016/j.bbamcr.2017.09.008
Schmid, D., Dengjel, J., Schoor, O., Stevanovic, S., and Munz, C. (2006a). Autophagy in innate and adaptive immunity against intracellular pathogens. J. Mol. Med. 84, 194–202. doi: 10.1007/s00109-005-0014-4
Schmid, M. C., Scheidegger, F., Dehio, M., Balmelle-Devaux, N., Schulein, R., Guye, P., et al. (2006b). A translocated bacterial protein protects vascular endothelial cells from apoptosis. PLoS Pathog. 2:e115. doi: 10.1371/journal.ppat.0020115
Schulein, R., and Dehio, C. (2002). The VirB/VirD4 type IV secretion system of Bartonella is essential for establishing intraerythrocytic infection. Mol. Microbiol. 46, 1053–1067. doi: 10.1046/j.1365-2958.2002.03208.x
Schulein, R., Guye, P., Rhomberg, T. A., Schmid, M. C., Schroder, G., Vergunst, A. C., et al. (2005). A bipartite signal mediates the transfer of type IV secretion substrates of Bartonella henselae into human cells. Proc. Natl. Acad. Sci. U. S. A. 102, 856–861. doi: 10.1073/pnas.0406796102
Seubert, A., Schulein, R., and Dehio, C. (2002). Bacterial persistence within erythrocytes: a unique pathogenic strategy of Bartonella spp. Int. J. Med. Microbiol. 291, 555–560. doi: 10.1078/1438-4221-00167
Shapiro, R. S., and Cowen, L. E. (2012). Thermal control of microbial development and virulence: molecular mechanisms of microbial temperature sensing. mBio 3:e00238. doi: 10.1128/mBio.00238-12
Siewert, L. K., Korotaev, A., Sedzicki, J., Fromm, K., Pinschewer, D. D., and Dehio, C. (2022). Identification of the Bartonella autotransporter CFA as a protective antigen and hypervariable target of neutralizing antibodies in mice. PNAS 119:e2202059119. doi: 10.1073/pnas.2202059119
Sorg, I., Schmutz, C., Lu, Y. Y., Fromm, K., Siewert, L. K., Bogli, A., et al. (2020). A Bartonella effector acts as signaling hub for intrinsic STAT3 activation to trigger anti-inflammatory responses. Cell Host Microbe 27, 476.e7–485.e7. doi: 10.1016/j.chom.2020.01.015
Stepanic, M., Duvnjak, S., Reil, I., Spicic, S., Kompes, G., and Beck, R. (2019). First isolation and genotyping of Bartonella henselae from a cat living with a patient with cat scratch disease in Southeast Europe. BMC Infect. Dis. 19:299. doi: 10.1186/s12879-019-3929-z
Tu, N., Lima, A., Bandeali, Z., and Anderson, B. (2016). Characterization of the general stress response in Bartonella henselae. Microb. Pathog. 92, 1–10. doi: 10.1016/j.micpath.2015.12.010
Vayssier-Taussat, M., Le Rhun, D., Deng, H. K., Biville, F., Cescau, S., Danchin, A., et al. (2010). The Trw type IV secretion system of Bartonella mediates host-specific adhesion to erythrocytes. PLoS Pathog. 6:e1000946. doi: 10.1371/journal.ppat.1000946
Vergunst, A. C., van Lier, M. C., den Dulk-Ras, A., Stuve, T. A., Ouwehand, A., and Hooykaas, P. J. (2005). Positive charge is an important feature of the C-terminal transport signal of the VirB/D4-translocated proteins of agrobacterium. Proc. Natl. Acad. Sci. U. S. A. 102, 832–837. doi: 10.1073/pnas.0406241102
Wagner, A., and Dehio, C. (2019). Role of distinct type-IV-secretion systems and secreted effector sets in host adaptation by pathogenic Bartonella species. Cell. Microbiol. 21:e13004. doi: 10.1111/cmi.13004
Wagner, A., Tittes, C., and Dehio, C. (2019). Versatility of the BID domain: conserved function as type-IV-secretion-signal and secondarily evolved effector functions within Bartonella-infected host cells. Front. Microbiol. 10:921. doi: 10.3389/fmicb.2019.00921
Waksman, G. (2019). From conjugation to T4S systems in gram-negative bacteria: a mechanistic biology perspective. EMBO Rep. 20:e47012. doi: 10.15252/embr.201847012
Wang, C., Fu, J., Wang, M., Cai, Y., Hua, X., Du, Y., et al. (2019). Bartonella quintana type IV secretion effector BepE-induced selective autophagy by conjugation with K63 polyubiquitin chain. Cell. Microbiol. 21:e12984. doi: 10.1111/cmi.12984
Wang, C. Y., Patel, N., Wholey, W. Y., and Dawid, S. (2018). ABC transporter content diversity in Streptococcus pneumoniae impacts competence regulation and bacteriocin production. Proc. Natl. Acad. Sci. U. S. A. 115, E5776–E5785. doi: 10.1073/pnas.1804668115
Keywords: Bartonella, effector proteins, type IV secretion system, luciferase, NanoLuc, STAT3
Citation: Fromm K, Boegli A, Ortelli M, Wagner A, Bohn E, Malmsheimer S, Wagner S and Dehio C (2022) Bartonella taylorii: A Model Organism for Studying Bartonella Infection in vitro and in vivo. Front. Microbiol. 13:913434. doi: 10.3389/fmicb.2022.913434
Edited by:
Axel Cloeckaert, Institut National de Recherche pour l’agriculture, l’alimentation et l’environnement (INRAE), FranceReviewed by:
Volkhard A. J. Kempf, Goethe University Frankfurt, GermanyMatthias Truttmann, University of Michigan, United States
Algimantas Paulauskas, Vytautas Magnus University, Lithuania
Copyright © 2022 Fromm, Boegli, Ortelli, Wagner, Bohn, Malmsheimer, Wagner and Dehio. This is an open-access article distributed under the terms of the Creative Commons Attribution License (CC BY). The use, distribution or reproduction in other forums is permitted, provided the original author(s) and the copyright owner(s) are credited and that the original publication in this journal is cited, in accordance with accepted academic practice. No use, distribution or reproduction is permitted which does not comply with these terms.
*Correspondence: Christoph Dehio, Y2hyaXN0b3BoLmRlaGlvQHVuaWJhcy5jaA==