- 1Instituto de Tecnologia Química e Biológica António Xavier, Universidade Nova de Lisboa, Oeiras, Portugal
- 2Instituto Nacional de Investigação Agrária e Veterinária, Oeiras, Portugal
Sporomusa ovata is a bacterium that can accept electrons from cathodes to drive microbial electrosynthesis (MES) of acetate from carbon dioxide. It is the biocatalyst with the highest acetate production rate described. Here we review the research on S. ovata across different disciplines, including microbiology, biochemistry, engineering, and materials science, to summarize and assess the state-of-the-art. The improvement of the biocatalytic capacity of S. ovata in the last 10 years, using different optimization strategies is described and discussed. In addition, we propose possible electron uptake routes derived from genetic and experimental data described in the literature and point out the possibilities to understand and improve the performance of S. ovata through genetic engineering. Finally, we identify current knowledge gaps guiding further research efforts to explore this promising organism for the MES field.
Introduction
Electrotrophs such as Sporomusa ovata are organisms capable to use electrons collected from electrodes as an energy source. Together with the autotrophic capacity to produce complex organic compounds from carbon dioxide (CO2), these capabilities enable the production of various chemicals in a process called microbial electrochemical synthesis (MES; Jourdin and Burdyny, 2020). Given that MES allow the production of chemicals from CO2 and renewable energy (Figure 1), it promotes the reduction of CO2 emissions and enables the implementation of a circular economy.
The main products of MES reported so far are acetic acid, ethanol, n-butyric acid, n-butanol, hexanoic acid, and n-hexanol (Jourdin and Burdyny, 2020). Among these, acetate represents the product in 75% of all studies (Flexer and Jourdin, 2020). The worldwide industrial acetate production reached 3.5 million tons (Jourdin et al., 2020) and despite the political efforts for climate change mitigation by decreasing petroleum usage, 90% are still produced from fossil oil and gas (Vidra and Nemeth, 2018). The main importance of acetate is its role as C2 platform chemical used to generate a plethora of different products in the organic chemistry industry. Starting from acetate, e.g., acetic anhydride can be produced by chain elongation which is subsequently converted to cellulose acetate. Cellulose acetate is used to produce textile fibers, cigarette filters, and plastics (Bajracharya, 2016; Falcke et al., 2017).
Koch and Harnisch (2016) identified 45 bacterial strains capable of cathodic electron uptake, with 13 being also autotrophic and thus candidates for MES. Das et al. (2020) rated the acetate production of different pure cultures, showing that S. ovata is by far the most promising biocatalyst. It produces 51.1 g m–2 day–1 of acetate compared to 12.8 g m–2 day–1 by the second best, Acetobacterium woodii, and 0.14 g m–2 day–1 by Clostriudium ljungdahlii. There is already a genetic system and fundamental mechanistic studies of C. ljungdahlii and Acetobacterium ferrooxidans (Sydow et al., 2014), but the production rates of valuable products are not competitive compared to S. ovata. S. ovata is reported to be the best acetate producer when used as a pure culture. The highest reported current density with S. ovata is 22 A m–2 (Aryal et al., 2019).
The main requirements to achieve the needed transition of MES to industrial-scale are the improvement of (i) conversion rates, (ii) electron uptake rates, (iii) chemical production rate, and (iv) product selectivity (Flexer and Jourdin, 2020). Research aiming to close these gaps is intense, highly dynamic, and frequently reviewed. The current state-of-the-art and future perspectives of MES, in general, were recently described (Bian et al., 2020; Jourdin and Burdyny, 2020; Prévoteau et al., 2020; Dessì et al., 2021), its techno-economic future assessed (Jourdin et al., 2020), and a ranking regarding its Technology Readiness Level provided (Fruehauf et al., 2020). These show a solid basis of knowledge about MES. Among others, several non-biological operation parameters such as temperature, salinity, and pressure are elaborated as necessary and easily attainable optimization parameters (Jourdin and Burdyny, 2020). Furthermore, selecting electrode materials and architecture optimized toward mass transport and current distribution inside the structure shows a high potential to increase product yields (Jourdin and Burdyny, 2020). But the core electron uptake process of the microorganisms catalyzing the desired reaction, also recently reviewed in Karthikeyan et al. (2019), is still unknown to a great extent. Exemplary, it is becoming widely accepted that indirect electron transfer is predominant compared to the direct electron uptake from an electrode (Prévoteau et al., 2020). The indirect electron transfer is proposed to occur via hydrogen produced with the help of secreted hydrogenases or catalytically active precipitates on the electrodes (Tremblay et al., 2019), but their nature is not conclusively clarified.
Whether S. ovata uses direct or indirect electron transfer has a decisive impact on tailoring the design of a reactor, electrode materials, and the process parameters. If indirect electron transfer via hydrogen is dominant, it will be essential to understand how S. ovata achieves the necessary hydrogen evolution rates at high electrode potentials. This knowledge will push related fields such as green hydrogen production forward, as presented in Figure 1. Furthermore, it will help to decide on two-stage or one-stage reactors. In the case of indirect electron uptake, two-stage reactors can be used where hydrogen production and the subsequent conversion of hydrogen and CO2 to acetate can be optimized separately. A two-stage reactor in turn will detain the possibility of the supportive effects on hydrogen evolution by the presence of S. ovata seems to have (Tremblay et al., 2019). Thus, the development of the optimal operational conditions relies on elucidating the fundamental mechanism of indirect electron transport. If, by contrast, direct electron transfer is dominant, then the responsible biochemical pathway remains to be identified. From the genome, we expect electron uptake mechanisms to be different from those described for electrotrophs already studied at the biochemical level, such as Rhodopseudomonas palustris TIE-1 or Rhodobacter ferrooxidans SW2 (Saraiva et al., 2012; Bird et al., 2014). Direct electron uptake would enable the use of one-stage reactors, which show a smaller operational complexity but need to meet the requirements of S. ovata and be optimized toward electrochemical parameters at the same time. Thus, fundamental research on the electron transfer mechanisms between the cathode and the biocatalyst is sorely needed.
Within this review, we suggest studying S. ovata as a model organism to enable the detection of bottlenecks and further development of MES. Understanding the molecular mechanisms of electron uptake of S. ovata will lead to the needed knowledge to steer the work in this area.
The Genus Sporomusa
Bacteria of the genus Sporomusa are strictly anaerobic, stain Gram-negative, and form spores. These two latter properties are unusual to be found together, and despite staining Gram-negative, the genus belongs to the Phylum of Gram-positive bacteria (Stackebrandt et al., 1985). A Sporomusa isolate is typically identified by the size between 0.4–0.9 × 2–8 μm, the motility, the banana shape, the formation of endospores, the true Gram-negative character with the presence of two membranes, and the production of acetic acid (Moeller et al., 1984). Substrates that all Sporomusa species can metabolize are N-methyl compounds, hydroxy fatty acids, primary alcohols, and a mixture of H2 and CO2, with the typical fermentation product being acetate (Moeller et al., 1984). Sporomusa species were isolated from various anoxic environments such as soils, sediments, digestive systems, and wastewater (McClane et al., 2006).
Sporomusa species perform homoacetogenic metabolism, which means that they can form acetate from CO2 and H2 via the acetyl-CoA pathway for energy conservation (also known as Wood Ljungdahl, WL-Pathway). This pathway is an ecologically important way to fix CO2, being identified in more than 100 bacteria and is expected to account for a share of approx. 20% of the worldwide carbon fixation (Ljungdahl, 2009).
The Metabolism of S. ovata
The species S. ovata was first described in 1984 by the work of Moeller et al. (1984), where ovata was named due to its egg-shaped endospores. A critical feature of S. ovata enabling the assimilation of CO2 is its homoacetogenic nature that uses the reductive acetyl-CoA pathway for energy conservation and CO2 fixation (Visser et al., 2016). The acetyl-CoA pathway contains two branches that allow the conversion of two molecules of CO2 to acetyl-CoA (Schuchmann and Müller, 2016). In the methyl branch, CO2 is reduced to formate, which is subsequently bound to the cofactor tetrahydrofolate, being reduced step wisely to become a precursor of the methyl group of acetyl-CoA. In the carbonyl branch, a second molecule of CO2 is reduced to CO to become the precursor for the carbonyl group of acetyl CoA, catalyzed by the carbon monoxide dehydrogenase/acetyl-CoA synthase (CODH/Acs; Schuchmann and Müller, 2016). Acetyl-CoA can then be incorporated into biomass or converted to end-products, including acetate. Interestingly, the role of this pathway is to provide an electron sink for redox balancing instead of feeding a respiratory chain. Indeed, no net ATP is gained when acetate is produced, given that one mole of ATP is consumed in the acetate kinase reaction, and one mole of ATP is produced in the formyl-tetrafolate synthetase reaction (Figure 2). The advantage of this pathway is that it can be easily interfaced with a vast variety of electron donors, allowing acetogens to utilize energy from several substrates available in anoxic environments. Indeed, coupling acetogenesis to the conversion of heterotrophic substrates results in improved energy gain compared to classic fermentation (Müller, 2003). S. ovata can use H2 together with one-carbon compounds, including CO2, formate, methanol, and N-methylated compounds, but can also use betaine, sarcosine, fructose, lactate, pyruvate, ethanol, n-propanol, n-butanol, 2,3-butanediol, L-Alanine, and 1,2-propanediol as substrates (Visser et al., 2016).
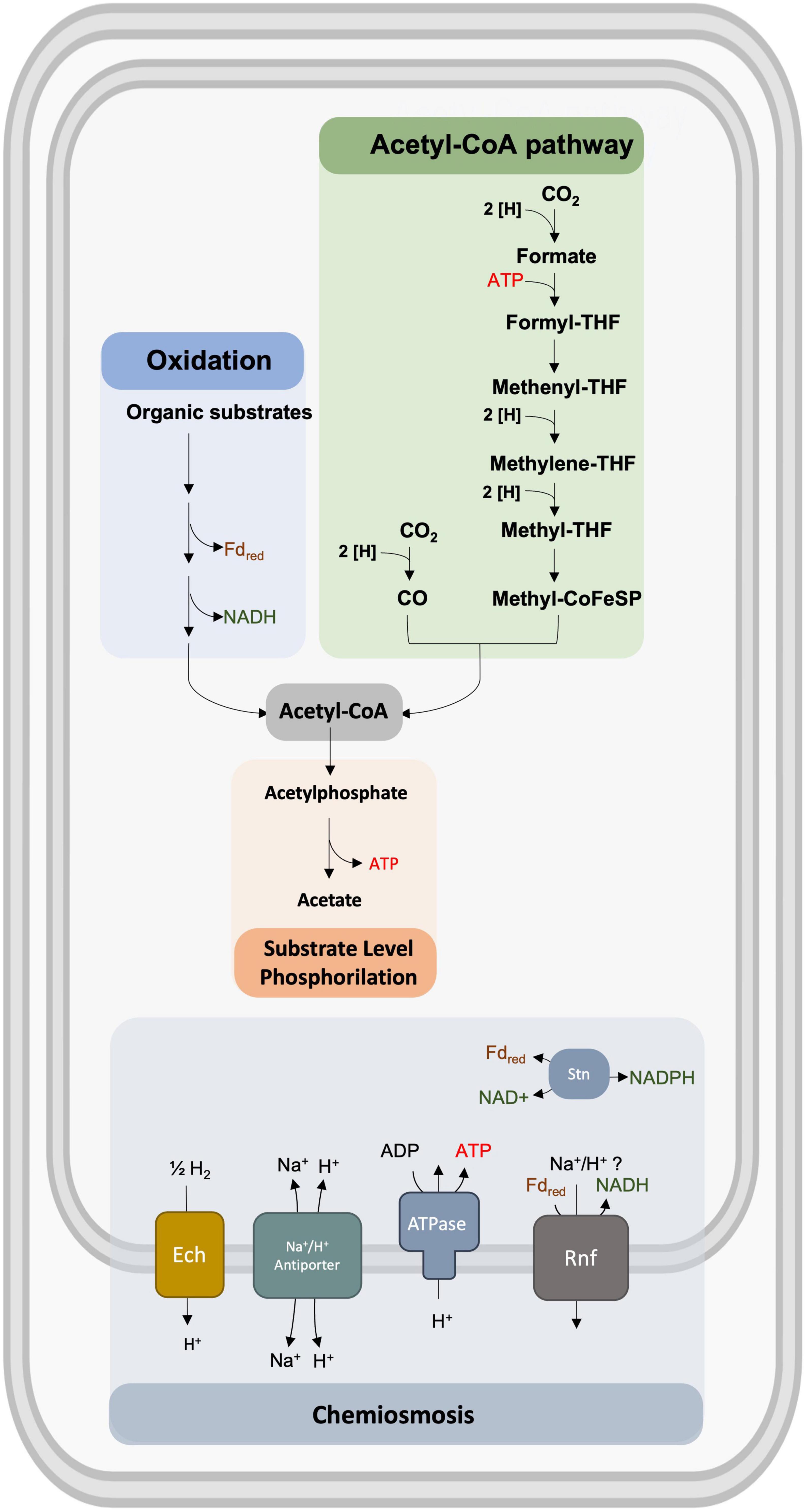
Figure 2. Representation of the bioenergetic metabolism of S. ovata that includes the acetyl-CoA pathway, the oxidation of organic substrates, the generation of ATP by substrate-level phosphorylation (SLP), and chemiosmosis.
All genes coding for enzymes of the acetyl-CoA pathway are present in the genome of S. ovata (Kremp et al., 2020). All these enzymes are predicted to be soluble cytoplasmic proteins, with none of them being involved in chemiosmotic energy conservation (Schuchmann and Müller, 2016). Therefore, the reducing equivalents in these organisms are gained by the oxidation of H2 during autotrophic growth or by NADH and reduced ferredoxin during heterotrophic growth. Membrane-integral protein complexes then oxidize these reduced compounds to generate a chemiosmotic gradient that is used for ATP generation (Figure 2). Acetogens differ in the respiratory enzymes used. While some use a sodium ion-translocating ferredoxin:NAD + oxidoreductase (Rnf) or the ion-translocating ferredoxin:H + oxidoreductase (Ech), others use an NADH-dependent reduced ferredoxin:NADP + oxidoreductase (Nfn; Schuchmann and Müller, 2014, Schuchmann and Müller, 2016; Kremp et al., 2020). Interestingly S. ovata has genes encoding for the Rnf complex and genes encoding the Ech Complex (Müller, 2019). Rnf complexes couple the electron transfer from reduced ferredoxin to NAD + to the translocation of sodium ions or protons across the cytoplasmic membrane, contributing to chemiosmotic ion translocation and thus ATP synthesis (Hess et al., 2013; Tremblay et al., 2013; Kremp et al., 2020; Figure 2). This is only possible by electron bifurcation, which is a mechanism that couples endergonic to exergonic redox reactions, allowing to save cellular ATP (Kremp et al., 2020). However, the ability of S. ovata to use both NADH and NADPH as reductants in the acetyl-CoA pathway and the absence of nfn genes in its genome led to the identification of a novel Sporomusa type Nfn (Stn; Kremp et al., 2020). This complex, composed of existing modules of the soluble Fe–Fe hydrogenase and Nfn, links the cellular NADP(H) pool with the NAD(H) and the ferredoxin pool. It is responsible to catalyze the energetically downhill reaction of NAD + or NADP + reduction by reduced ferredoxin and the reduction of NAD + with NADPH (Kremp et al., 2020).
Laboratory Growth Conditions
Sporomusa ovata can be grown in the DSMZ medium 311 with betaine as substrate but has also been cultivated with H2 and CO2. The optimal temperature to grow the mesophilic S. ovata is between 34 and 39°C (Moeller et al., 1984), and the optimum pH range lies between 5.3 and 7.2 (Moeller et al., 1984). Many acetogens including Sporomusa sp. can grow on iron [Fe(0)] as an electron donor, but not S. ovata (Kato et al., 2015). Similar to the cathodes in MES, Fe(0) is a solid electron donor. It is surprising, that S. ovata cannot use it to take up electrons.
Sporomusa ovata grown on cathodes use the electrode as an electron source and CO2 as the carbon source to sustain their autotrophic metabolism. We found that pre-cultures for bioelectrochemical experiments are also grown autotrophically under H2 and CO2 conditions (Aryal et al., 2016, 2017b,2018, 2019; Chen et al., 2016). We have not found any published study inoculating bioelectrochemical experiments directly from a betaine grown S. ovata culture.
An investigation of the effect of medium salinity on the growth of S. ovata shows, that an increase of the NaCl concentration significantly affects the doubling time: it increases from 10 to approx. 25 ± 5 h when the ionic strength is modified from 100 to 200 mM NaCl (Sakimoto et al., 2014). Interestingly, this study also demonstrated that increased salinity induces filamentous growth and the alignment of the cells on a photoactive silicon nanowire. The hydrostatic pressure in the growth conditions has a smaller impact: the doubling time is around 15 h at 500 kPa, while it is approx. 5 h faster at only 100 kPa (Sakimoto et al., 2014).
Optimization Strategies Aiming to Enhance Bioelectrochemical Acetate Production Using S. ovata
The first published work with S. ovata producing acetate on a cathode was performed by Nevin et al. (2010). Since then, S. ovata has been continuously used and different strains, electrode materials, operating parameters, and process concepts were evaluated with the aim to increase the acetate production rate. The acetate production rate normalized to the electrode surface is a parameter that allows the assessment of the quality of the electrobiocatalyst. Other important parameters such as volumetric production rates, coulombic efficiencies, product concentration, and energetic efficiencies are crucial for the overall assessment of MES concepts and their upscaling potential. However, they also relate to the reactor engineering, operation parameters, and experimental setup, which are not in the main scope of this review. Also beyond the scope of this review is the important work being done to explore how the production of other compounds such as alcohols can be manipulated (Ammam et al., 2016). So, to focus on S. ovata and stick to the aimed acetate production, we chose the normalized production rate as the parameter to establish a common denominator to compare different studies.
Microbiology
The biocatalyst plays a leading role regarding the aimed cathode reactions. Thus, engineering S. ovata, adapting it, or selecting suitable strains, directly influences the acetate production rate.
Strain Selection
There are only a few studies directly comparing different pure cultures for electroacetogenesis. One of them reveals that different Sporomusa species produce different amounts of acetate (Aryal et al., 2017b): S. aerivorans does not produce any detectable acetate, while S. ovata DSM-2663 outcompetes all other tested species or strains (S. malonica DSM-5090 and S. aerivorans DSM-13326, S. acidovorans, S. ovata DSM-2662, S. ovata DSM-3300) with respect to acetate production rate (61.1 ± 18.1 mmol m–2 day–1) (see Figure 3). Regarding coulombic efficiency, S. ovata DSM-2662 showed the best results, with 91.8% ± 5.3 compared to 61.1 ± 12.6% when using S. ovata DSM-2663. Another study shows that S. ovata clearly outcompetes Moorella species: 7.6 ± 0.2 mmol m–2 day–1 for S. ovata vs. 2.1 ± 0.2 mmol m–2 day–1 for M. thermoacetica and 3.5 ± 0.3 mmol m–2 day–1 for M. thermoautotrophica (Faraghiparapari and Zengler, 2017).
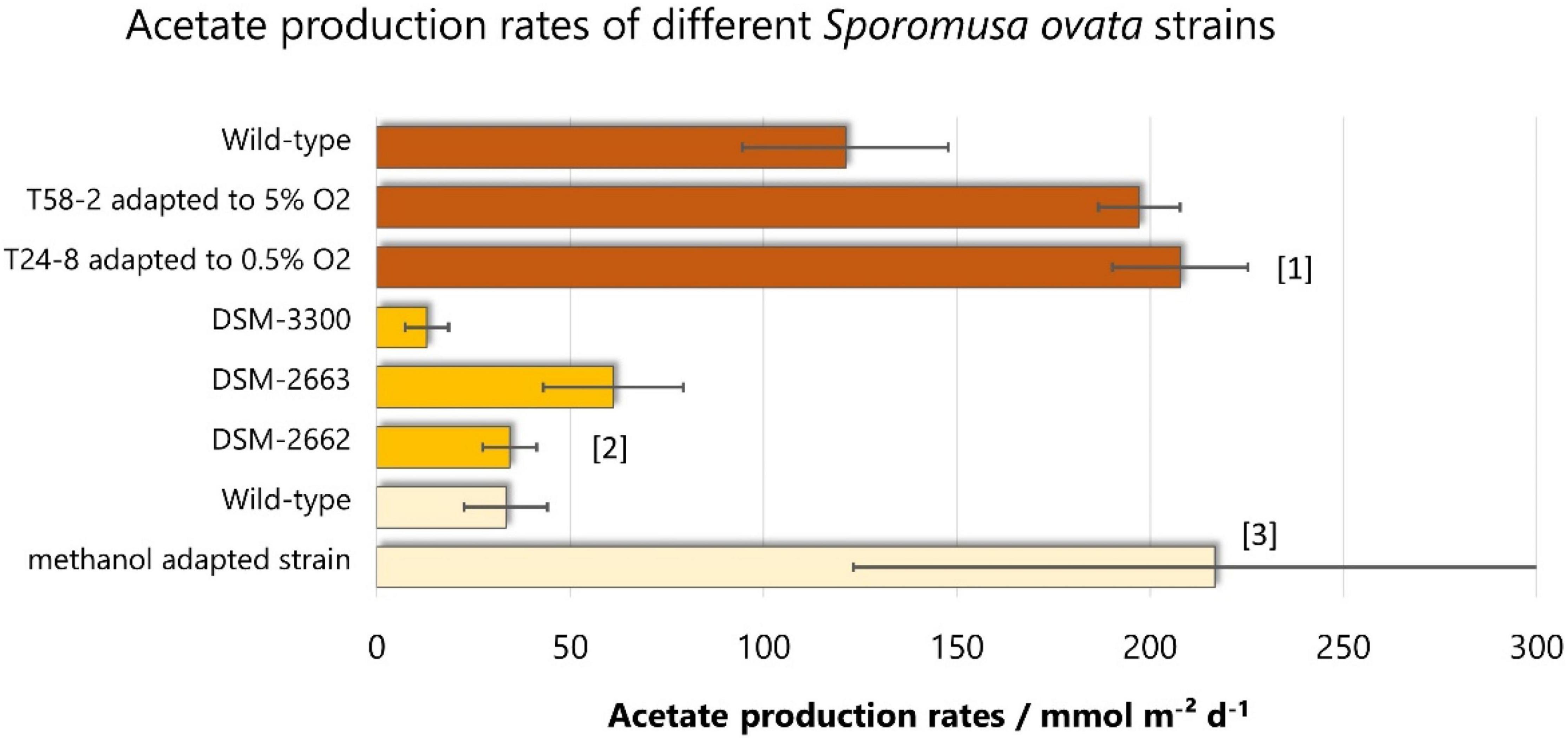
Figure 3. Acetate production rates of different S. ovata strains. Bars of the same color are derived from the same studies: [1]: (Shi et al., 2021), [2]: (Aryal et al., 2017b), [3]: (Tremblay et al., 2015). On that basis, results of the same color are directly comparable, as other parameters (cathode potential, medium, biofilm immobilization, reactor configuration) are not altered.
Adapted Laboratory Evolution
So far, no genetic engineering of S. ovata was reported. But adapted laboratory evolution (ALE) experiments show a great potential to improve the acetate production of S. ovata at the microbiological level. With ALE experiments adapting S. ovata to methanol, Tremblay et al. (2015) significantly increased the acetate production rate by 6.5-fold. The adapted strain produced 866.7 ± 373.5 mM m–2 day–1 at -690 mV vs. SHE, compared to 133.5 ± 43.2 mM m–2 day–1 for the wild-type. Shi et al. (2021) did a similar adaptation experiment but in the presence of oxygen. This study revealed that it is possible to adapt S. ovata to the presence of up to 5% oxygen and that S. ovata adapted to 0.5% oxygen produces 207.8 ± 17.5 mmol acetate m–2 day–1 which is 1.5-fold higher than the wild-type strain. This is of special importance because a slight oxygen tolerance will be advantageous in an industrial application and handling. There is oxygen produced on the anode which can leak to the cathode region and can cause problems for the anaerobic S. ovata. Indeed, even in the studied H-cells, the measured oxygen concentration in the cathode compartment ranged from 0.26 to 0.47 % in the time course of 120 h (Shi et al., 2021).
Sporomusa ovata does not seem to be a good biofilm builder on electrode surfaces. Nevertheless, different studies improved the attachment of the cells to the cathode and reported enhanced acetate production rates (Chen et al., 2016; Krige et al., 2021).
Electrode Materials
So far, one of the biggest advances in terms of acetate production rates was achieved through the optimization of electrode materials (Figure 4). They have a decisive impact on the cell-electrode interface and are crucial for a functioning biocatalyst. Generally, the most important parameters regarding electrode materials relate to distinct hierarchical levels: the electrode surface material itself, the electrode microstructure, and the electrode topology. While the surface is important regarding its chemistry, the electrode microstructure influences the electrochemically accessible surface area and the mass transport. The electrode topology on the other hand is the physical macrostructure and affects the electronic and ionic conductivity, the surface area per volume, and the mass transport (Kerzenmacher, 2017). Studies reporting different electrode materials often do not distinguish between these levels, making the interpretation difficult. For example, the coating of electrodes can increase accessible surface area and surface chemistry at the same time. This makes it difficult to distinguish if a bigger area or the surface chemistry leads to a different result.
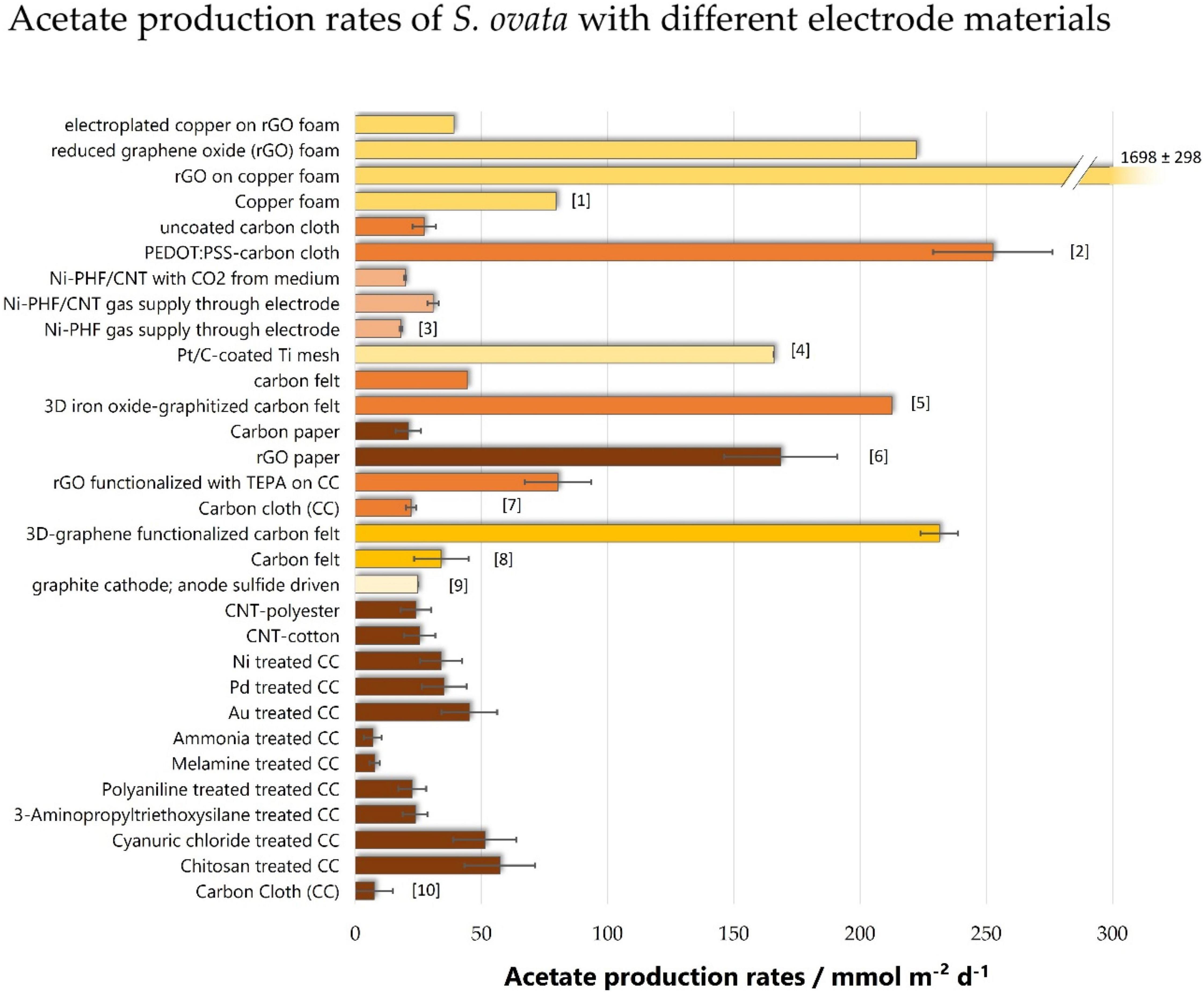
Figure 4. Acetate production rates of S. ovata with different electrode materials. Bars of the same color are derived from the same studies: [1]: (Aryal et al., 2019), [2]: (Aryal et al., 2016), [3]: (Bian et al., 2018), [4]: (Li et al., 2018), [5]: (Cui et al., 2017), [6]: (Aryal et al., 2017a), [7]: (Aryal et al., 2018), [8]: (Aryal et al., 2016), [9]: (Gong et al., 2013), [10]: (Zhang et al., 2013). On that basis, the same color results are directly comparable, as other parameters (cathode potential, medium, temperature, reference for normalization) are equal. rGO, reduced graphene oxide; PEDOT:PSS, poly(3,4-ethylenedioxythiophene:polystyrene sulfonate); Ni-PHF, porous nickel hollow fiber; TEPA, tetraethylene pentaamine; CNT, carbon nanotubes; CC, carbon cloth.
Surface Chemistry
One unambiguous finding regarding the material chemistry is that providing a positive surface charge can effectively enhance MES (Zhang et al., 2013). The surface charge of S. ovata has a Zeta potential of about -12.8 ± 1.6 mV (Chen et al., 2016), which explains why the positive charge helps the bacteria to grow on the electrode. Coating a thin layer of chitosan on top of graphite felt provides a positive charge and Zhang et al. (2013) showed that this significantly enhances acetate production rates by 7.6-fold compared to uncoated graphite felt. This improvement comes along with 9× higher cell densities of S. ovata on the electrode surface and it was the highest improvement compared to other electrode modifications tested in this study. As the specific surfaces are not reported and the acetate production rate is normalized to the geometric area, it is not always clear to which extent the chemical modification, or the better microstructure is the reason for improvement.
3D Electrodes
3D electrodes are described as a promising concept to increase current densities in all bioelectrochemical systems and target the improvement of the macrostructure. They specifically increase the area for bacteria to interact with the electrode which makes them extremely promising. Unfortunately, most studies with 3D electrodes are normalized to the geometric 2D surface, making comparison difficult.
Aryal et al. (2019) developed a copper foam coated with reduced graphene oxide (rGO), that allowed the production of 1,697.6 ± 298.1 mmol m–2 day–1 of acetate at -990 mV vs. SHE. This is by far the highest production rate with S. ovata and also any other pure culture reported in the literature. The copper core, which is highly conductive and inexpensive, provides an optimal carrier for the biocompatible rGO. Compared to the uncoated copper foam, the surface was increased 161-fold, while current density and acetate production rate were increased 3-fold and 21-fold, respectively. This condenses in very different coulombic efficiencies: While the uncoated copper displays a very low coulombic efficiency of 10 ± 6.5%, coating it with rGO results in 70.2 ± 14.1%.
Cui et al. (2017) investigated the acetate production of S. ovata with a three-dimensional hierarchical metal oxide–carbon electrode. This modification of a carbon felt electrode results in a 4.8-fold increase of acetate production rate. This study is the only one cited here that reports the acetate production normalized to the volume of the electrode instead of the geometric area.
To compare these volumetric production rates with other studies (Figure 4), the acetate production reported in Cui et al. (2017) was normalized to the reported size of the electrode’s geometric surface. However, we want to emphasize and encourage the shift of paradigm in future work to report production rates and current densities normalized to the volume of the electrode whenever it is not possible to completely rule out that the inner part of the electrode is accessible. Unfortunately, the volumetric current density or the accessible surface area is often difficult to assess from the limited data reported in the literature making it impossible to recalculate the values normalized to the volume or specific surface for all studies.
Gas Supply Through Electrodes
Another promising and exciting approach to increasing MES’s efficiency is the use of electrically conductive gas permeable materials as electrodes (Bian et al., 2018) or gas diffusion cathodes (Bajracharya et al., 2016). In this setup, the electrode itself acts as a three-phase boundary bringing together the four necessary components for acetate production: the substrate CO2, the bacteria as the catalyst, the medium providing H+, and the cathode delivering electrons. The direct supply of CO2 through the porous electrode showed a 1.6-fold increase in acetate production (Bian et al., 2018).
Operation Parameters
Temperature, medium composition, and different cell voltages were also tested with S. ovata and are discussed in the following sections. But the pressure inside the reactor which influences gas solubility has not been investigated with S. ovata. The hypothesis that increasing pressure might be an easy way of optimizing MES (Jourdin and Burdyny, 2020) needs to be tested (Jourdin and Burdyny, 2020). Exemplary, pressurized reactors are known to improve the activated sludge process treating wastewater by increasing the dissolved oxygen levels (Jin et al., 2010). But we did not find MES studies with S. ovata exploring this possibility. In contrast, it was shown, that higher pressure generally slows down S. ovata growth (Sakimoto et al., 2014). Still, pressurizing the reactors would enhance the CO2 availability (Jourdin and Burdyny, 2020) and can therefore be an easy way of improving acetate production. The investigation of the balance between the two effects may reveal conditions that enhance the acetate production rate.
Another topic that has not been systematically investigated is the method of CO2 supply into the system. The effect of parameters like the volumetric flow of the gas, the gas composition, and reactor mixing on the acetate production rates of S. ovata is not known yet. In mixed community biofilms, it was shown that the supply of CO2 is favorable compared to HCO3 (Mohanakrishna et al., 2016; Izadi et al., 2020). Still, the chemical equilibrium between CO2 and HCO3 is determined by the pH. Thus, the form of carbon supply itself might be secondary compared to the pH in the system.
Temperature
The temperature is an essential parameter when trying to optimize MES. On the one hand, the gas solubility is higher at lower temperatures. On the other hand, the cells have an optimal temperature for growth. A study that compares different temperatures (Figure 5) showed that S. ovata achieves the optimal acetate production rate at 25°C, but no temperatures lower than this were investigated (Faraghiparapari and Zengler, 2017). This temperature is surprising, as 37°C, also tested in this study, is the temperature closer to the optimal growth temperature for S. ovata (between 34 and 39°C; Moeller et al., 1984). The higher acetate production rate at lower temperatures is likely to be related to the better gas solubility at lower temperatures. More fundamental research would be necessary to find out if the gas solubility of the possibly occurring H2 or of the substrate CO2 is decisive.
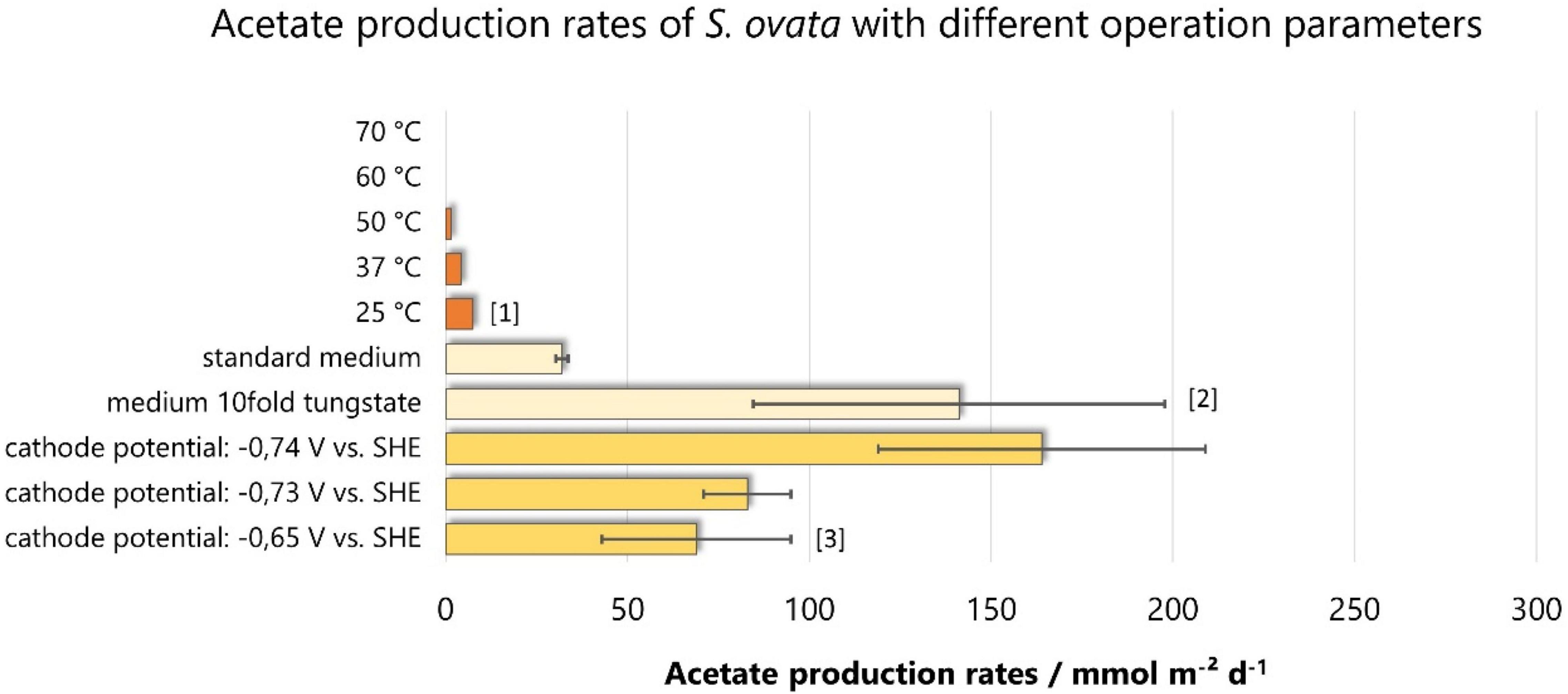
Figure 5. Acetate production rates among different operation parameters with S. ovata. Bars of the same color are derived from the same studies: [1]: (Faraghiparapari and Zengler, 2017), [2]: (Ammam et al., 2016), [3]: (Giddings et al., 2015). On that basis, the same color results are directly comparable, as other parameters (electrode material, reactor setup, Sporomusa strain, etc.) are equal.
Medium Composition
The medium composition is also an essential factor influencing acetate production with S. ovata. Indeed, tungstate concentration in the medium influences the acetate production rates (Ammam et al., 2016). A 10-fold increase of tungstate concentration in the medium resulted in a 1.2-fold lower doubling time. The effect on acetate production is even higher: it increases the rate 4.4-fold (from 32.0 ± 1.7 to 141.2 ± 56.6 mmol m–2 day–1; Ammam et al., 2016). Analyzing the gene expression at different tungstate levels showed that enhanced biosynthesis of acetate and other compounds come along with higher gene expression of tungsten-containing aldehyde ferredoxin oxidoreductases and a tungsten-containing formate dehydrogenase. An increase in tungstate concentration also steers the process to ethanol production in S. ovata (Ammam et al., 2016).
Cathode Potential
Another decisive parameter affecting acetate production is the cathode potential. The more negative it is the higher are acetate production rates (Giddings et al., 2015; Tremblay et al., 2019). Unfortunately, it is not yet clear to what extent S. ovata performs direct electron uptake on the cathode or relies on the intermediate H2. In both cases, the level of knowledge hypothesizes that a low cathode potential is favorable, providing a higher driving force. But from the microbial perspective, the optimal electrode potential can depend on the used metabolic pathway and must be investigated. From a techno-economic assessment, the optimal cathode potential will be a trade-off between high acetate production rates and the needed energy to power low cathode potentials.
Giddings et al. (2015) report acetate production rates of S. ovata at different cell voltages resulting in cathode potentials of -0.74, -0.73, and -0.65 V vs. SHE. These results allow a first impression of the effect of cathode potential on the acetate production rates but do not draw a comprehensive picture. This study aims to implement a simple reactor setup without potentiostatic control and works with a DC power source between working and counter electrodes (cathode and anode, respectively). Thus, the resulting cathode potentials are not fixed and regulated but result from the imposed cell voltages. The difference of 10 mV in the resulting cathode potential between the reactors running at 3.5 and 5 V cell voltage can hardly explain the significantly higher acetate production rate of 164 ± 45 mmol m–2 day–1 at -0.73 V vs. SHE compared to 83 ± 12 mmol m–2 day–1 at -0.74 V vs. SHE. We assume that this correlation is not caused by the slight potential difference and want to point out that the influence of the cathode potential on MES performance with S. ovata is a critical knowledge gap to be studied.
Microbial Electrosynthesis Process Engineering
Sporomusa ovata is not only used for acetate production directly on electrodes but was also already investigated in a two-stage reactor, where hydrogen is produced electrochemically on the electrode, which is then subsequently used by S. ovata to reduce CO2 to acetate. Kracke et al. (2019) report a maximum acetate production rate of 4.84 × 10–3 mmol h–1 with S. ovata and a 1 cm2 flat MoS2 electrode at ca. -1.0 V vs. SHE. The acetate production corresponds to 1,162 mmol m–2 day–1, which exceeds all reviewed acetate production rates of S. ovata with 2D electrodes (see Figure 4). Additionally, it has a very high coulombic efficiency reported at 103.7%. This value >100% indicates that a two-stage approach aiming and optimizing for intermediate hydrogen production is favorable. But a one-stage process circumventing hydrogen production with direct electron uptake of the biocatalyst will be more competitive in terms of safety and effectiveness (Rabaey and Rozendal, 2010). Another successfully tested concept is the introduction of hydrogen carriers into a system where hydrogen is produced abiotically (Rodrigues et al., 2019). With the help of the carrier, which in this case was a perfluorocarbon nanoemulsion, the hydrogen availability is improved in the entire reactor and acetate production rates increased by 190% could be shown (Rodrigues et al., 2019).
However, to conclusively assess this core question and decide which way to go, the biocatalyst’s underlying electron transfer mechanisms and energetic metabolism need to be investigated.
Independently of whether S. ovata uses direct or indirect electron transfer, the produced acetate will need to be extracted from the MES reactor. For this, Gildemyn et al. (2015) propose a three-compartment reactor system in which an anion exchange membrane and a cation exchange membrane separate the cathode and the anode compartments, respectively, (Gupta et al., 2019). In the resulting extraction compartment in the middle, the acetate spontaneously accumulates, driven by the electric field of the MES reactor.
And while acetate can be the target product, it can also be directly upgraded to long-chain alkyl esters in MES, aiming at the production of biofuels. Lehtinen et al. (2017) use S. ovata to produce acetate, and Acinetobacter baylyi subsequently converts it into long alkyl esters in a second aerobic step (Lehtinen et al., 2017). S. ovata is also capable of producing ethanol (Blanchet et al., 2015; Ammam et al., 2016), and 2-oxobutyrate (Nevin et al., 2010). These products are not in the scope of this review but demonstrate the versatility of S. ovata for different processes.
Electron Uptake Mechanisms of S. ovata
In the case of mixed communities in MES, there is evidence that biofilms have paramount importance for electron uptake and acetate production (Jourdin et al., 2016; Izadi et al., 2020). This biofilm role also seems true for pure S. ovata cultures: Aryal et al. (2016) showed that a dense immobilized biofilm in a bioinorganic matrix significantly improves current densities and acetate production rates. But a systematic study of the biofilms‘ contribution to current uptake compared to the planktonic fraction is not yet available. This open research question is interdependent with the direct or indirect nature of electron uptake by S. ovata. While Nevin et al. (2010) stated that S. ovata consumes electrons directly from an electrode, newer publications suggest a hydrogen-dependent electron uptake process (Tremblay et al., 2019). To assess possible electron uptake mechanisms, multiheme cytochromes and hydrogenases were searched in the genome of S. ovata DSM 2662 (GCF_000445445.1) using Interproscan 5.39–77.0. Only matches that were simultaneously present in the RefSeq (NCBI) and UniProtKB databases were considered.
Indirect Electron Uptake via Hydrogen
If the electron uptake occurs indirectly through hydrogen, hydrogen must be formed by reducing water. Hydrogen can then be used as the electron donor in S. ovata to form acetate with CO2. Different ways of hydrogen evolution are possible: (i) abiotic production at electrode potentials ≤-500 mV vs. NHE (Karthikeyan et al., 2019); (ii) hydrogen evolution at higher electrode potentials ≤-300 mV vs. NHE through the catalytically active and secreted compounds like hydrogenases but of unknown nature; (iii) abiotic hydrogen production at higher electrode potentials as expected due to very low partial pressures of hydrogen (Philips, 2020).
The difficulty in exploring the role of hydrogen as an intermediate is that hydrogen is consumed by the bacteria at a fast rate, making the detection difficult. Tremblay et al. (2019) placed a microsensor close to the cathode, which could not detect hydrogen evolution at -501 mV vs. NHE with the abiotic fresh medium. Interestingly, in cell-free but already used medium by S. ovata, hydrogen production was observed at more positive potentials (-301 mV vs. NHE; Tremblay et al., 2019). This observation suggests the presence of secreted metabolites, cell components, or chemicals produced by S. ovata which enhance the catalytic hydrogen production. Indeed, Co and Ni deposition on the cathodes was determined, but no intact hydrogenases were found. Theoretically, it is possible that S. ovata secretes hydrogenases or uses hydrogenases on the cell membrane to form hydrogen which will subsequently be used as the electron donor. A search of the genome and annotated proteins reveals at least four different hydrogenase complexes coded. Among them are [NiFe] and [FeFe] hydrogenases, whose contribution to MES would need to be investigated. Table 1 lists all found hydrogenases. Still, there is no evidence to prove if hydrogenases in S. ovata are secreted or acting intracellularly.
Visser et al. (2016) introduce an S. ovata strain called An4, for which the authors report a [NiFe] hydrogenase complex for which the small subunit contains a tat signal motif. The presence of such a signal motif opens up the possibility that this hydrogenase (WP_021167303.1) is transported across the cytoplasmic membrane. It is also present in S. ovata 2662. Still, there is no evidence for hydrogenases, which are also transported across the outer cell membrane to the surrounding medium. This capability was previously reported for Methanococcus maripaludis (Deutzmann et al., 2015; Tsurumaru et al., 2018), and a mixed biofilm (Marshall et al., 2017) but never for S. ovata.
It has also been hypothesized that the hydrogen consumption by acetogenic bacteria induces a low partial pressure, which again favors hydrogen evolution by reducing the activation energy without additional catalysts (Philips, 2020).
Intracellular hydrogen formation, low hydrogen partial pressures, and immediate consumption make hydrogen detection challenging. To better understand the role of hydrogen, it is necessary to reveal the electron uptake mechanism on a biomolecular level.
Hypothetical Mechanisms for Direct Electron Uptake
Multiheme c-type cytochromes play a decisive role in known electron transfer mechanisms, especially in extracellular electron transfer pathways of exoelectrogens that transfer electrons to an anode, including Geobacter and Shewanella. Similar pathways can function in reverse on the cathode in some electroactive organisms, such as R. palustris TIE-1 (Gupta et al., 2019). Although, for S. ovata, the existence of multiheme c-type cytochromes is reported (Kamlage et al., 1993; Visser et al., 2016), it is not yet known if they are involved in electron uptake processes (Table 2). Should such a mechanism be operative, it could feed electrons directly in the bioenergetics electron transport chain or promote intracellular hydrogen production via hydrogenases or nitrogenases (Marshall et al., 2017; Izadi et al., 2020) at unknown electrode potentials.
Nitrogenases fix dinitrogen to ammonia and co-produce H2, but are also capable of reducing other substances such as CO2 to formate. In the absence of these, nitrogenase will lead the electron flux toward hydrogen (Milton and Minteer, 2019), meaning that electrons are accepted by the cell and transported to the nitrogenases. If the electrons are received directly from the electrode or through a carrier needs to be discussed. In any case, hydrogen is produced inside the cells. If the hydrogen gets released into the medium, it would then contribute to indirect electron transport and electrons stored in the hydrogen could be reused. Depending on the presence of nitrogen species in the medium, different amount of hydrogen gets produced. According to the reaction stoichiometry of N2 reacting to NH3 catalyzed by nitrogenases, the produced hydrogen contains 25 % of the originally up taken electrons (Hoffman et al., 2009).
Marshall et al. (2017) showed that the nitrogenase encoding NifH is one of the most abundant genes in a mixed community biofilm with high acetate production rates, suggesting an essential role in acetate production on cathodes. An analysis of the S. ovata 2662 genome in the PATRIC resource center1 and UniProt databank reveals the presence of NifH, NifB, NifE, NifN, and NifDK encoding for FeMo nitrogenases. The presence of these genes is an indication that this route of transient hydrogen production might also play a role in acetate production with S. ovata. Interestingly, but in opposite direction, Tremblay et al. (2015) show that a highly efficient methanol adapted strain of S. ovata producing acetate, had downregulated the gene SOV_1c07070 which is annotated as a dinitrogenase FeMo cofactor biosynthesis protein. But this might happen since the medium used in this study contains ammonium chloride resulting in transcriptional downregulation of the redundant nitrogenase as a strategy to save energy.
A close look into the proteome of S. ovata reveals that 6 out of 7 c-type cytochromes belong to NrfHA complexes attributed to the nitrite reductase family (determined by UniProt; Igarashi and Kato, 2021). These are membrane-attached proteins that agree with the reported presence of c-type cytochromes in the membrane fractions of S. ovata (Moeller et al., 1984). Indeed, the BLAST survey on NCBI reveals homology with the well-characterized NrfHA complex of Desulfovibrio vulgaris, which is bound to the periplasmic membrane (Rodrigues et al., 2006; Luisa Rodrigues et al., 2008). All three encoded NrfAH complexes are paralogs. Another c-type cytochrome is the WP_021170960.1 which does not show homology to any known protein and thus remains hypothetical. The sequence reveals that it is a soluble dihemic protein, which can be involved in electron transfer in the periplasmic space of S. ovata.
Concluding Remarks
From the previous sections, we can conclude that S. ovata is a very promising biocatalyst for the generation of acetate in MES. And considerable work has already been done for optimizing cathode materials, operation parameters, and microbiological adaptation. The achieved acetate production rates with S. ovata are far beyond other species, and ALE experiments showed the flexibility of S. ovata to increase acetate production and oxygen tolerance by adaptation. But still, the net acetate production rates obtained need to be improved to drive MES into application scenarios. Fruehauf et al. (2020) rate the technological readiness level (TRL) among different products and different MES configurations and conclude that it is TRL 2-3 for biofilm-based MES and TRL 4-5 in two-stage reactors with hydrogen as intermediate. TRL 6 is considered to be the transition point from research to industrial implementation. Among others, one significant knowledge gap and research needed to push these technologies to the next development levels is the understanding of the underlying electron uptake mechanisms. To further optimize systems running with S. ovata and aiming for acetate production, it is of utmost importance to reveal if current uptake happens directly on the electrode or if S. ovata can steer MES indirectly through enzymes improving the intermediate hydrogen production. The latter is expected to be driven through hydrogenases or nitrogenases but has not been elucidated. The study of cathode potentials and their effect on the acetate production of S. ovata is also lacking and would already point out the underlying nature of direct or indirect electron transfer.
Another area to be studied is the role of nitrogenases in MES. Practically it is crucial to assess if the presence of nitrogen in gaseous or ammonia form influences the outcome of MES. To our best knowledge, this parameter has not yet been investigated.
On the protein level, it is essential to understand if c-type cytochromes are involved in the current uptake and, in that case, to reveal their function. To address these open issues, it is necessary to develop strategies to manipulate S. ovata genetically. Until this is possible, the production and characterization of the individual proteins provide an alternative to characterize their function (Louro et al., 2018). There are good prospects that S. ovata can be made genetically tractable by adapting tools developed for C. ljungdahlii described in Molitor et al. (2016) as S. ovata shows considerable genetic similarities to Clostridia. In general, genetic engineering will be of utmost importance and of high value to answer these fundamental questions about the electron uptake mechanisms on cathodes.
Making a back-of-the-envelope calculation summing up all achieved improvements in the discussed biological, operational and engineering parameters could improve acetate production rates more than 80 times. Summing up the enhancements which rely on different parameters in different studies is not a rigorous way to assess the great potential of S. ovata. Still, it provides an estimate of the magnitude of the challenge ahead. A better understanding of the nature of the underlying electron transfer will allow assessing the bottlenecks of MES and will be the next step on the way to application.
Author Contributions
JM: conceptualization, formal analysis, investigation, writing – original draft, writing – review and editing, visualization, project administration, and funding aquisition. CP: investigation, writing – original draft, writing – review and editing, visualization, and supervision. RS: investigarion, data curation, and visualization. RL: conceptualization, validation, resources, writing – review and editing, and supervision. All authors contributed to the article and approved the submitted version.
Funding
This project has received funding from the European Union’s Horizon 2020 Research and Innovation Program under the grant agreement number BioCat 867360.
Conflict of Interest
The authors declare that the research was conducted in the absence of any commercial or financial relationships that could be construed as a potential conflict of interest.
Publisher’s Note
All claims expressed in this article are solely those of the authors and do not necessarily represent those of their affiliated organizations, or those of the publisher, the editors and the reviewers. Any product that may be evaluated in this article, or claim that may be made by its manufacturer, is not guaranteed or endorsed by the publisher.
Footnotes
References
Ammam, F., Tremblay, P.-L., Lizak, D. M., and Zhang, T. (2016). Effect of tungstate on acetate and ethanol production by the electrosynthetic bacterium Sporomusa ovata. Biotechnol. Biofuels 9:163. doi: 10.1186/s13068-016-0576-0
Aryal, N., Halder, A., Tremblay, P. L., Chi, Q., and Zhang, T. (2016). Enhanced microbial electrosynthesis with three-dimensional graphene functionalized cathodes fabricated via solvothermal synthesis. Electrochim. Acta 217, 117–122. doi: 10.1016/j.electacta.2016.09.063
Aryal, N., Halder, A., Zhang, M., Whelan, P. R., Tremblay, P.-L., Chi, Q., et al. (2017a). Freestanding and flexible graphene papers as bioelectrochemical cathode for selective and efficient CO2 conversion. Sci. Rep. 7:9107.
Aryal, N., Tremblay, P. L., Lizak, D. M., and Zhang, T. (2017b). Performance of different Sporomusa species for the microbial electrosynthesis of acetate from carbon dioxide. Bioresour. Technol. 233, 184–190. doi: 10.1016/j.biortech.2017.02.128
Aryal, N., Tremblay, P.-L., Xu, M., Daugaard, A. E., and Zhang, T. (2018). Highly conductive poly(3,4-ethylenedioxythiophene) polystyrene sulfonate polymer coated cathode for the microbial electrosynthesis of acetate from carbon dioxide. Front. Energy Res. 6:72. doi: 10.3389/fenrg.2018.00072
Aryal, N., Wan, L., Overgaard, M. H., Stoot, A. C., Chen, Y., Tremblay, P. L., et al. (2019). Increased carbon dioxide reduction to acetate in a microbial electrosynthesis reactor with a reduced graphene oxide-coated copper foam composite cathode. Bioelectrochemistry 128, 83–93. doi: 10.1016/j.bioelechem.2019.03.011
Bajracharya, S. (2016). Microbial Electrosynthesis of Biochemicals. Wageningen: Wageningen University.
Bajracharya, S., Vanbroekhoven, K., and Buisman, C. J. N. (2016). Application of gas diffusion biocathode in microbial electrosynthesis from carbon dioxide. Environ. Sci. Pollut. Res. 23, 22292–22308. doi: 10.1007/s11356-016-7196-x
Bian, B., Alqahtani, M. F., Katuri, K. P., Liu, D., Bajracharya, S., Lai, Z., et al. (2018). Porous nickel hollow fiber cathodes coated with CNTs for efficient microbial electrosynthesis of acetate from CO2 using: Sporomusa ovata. J. Mater. Chem. A 6, 17201–17211. doi: 10.1039/c8ta05322g
Bian, B., Bajracharya, S., Xu, J., Pant, D., and Saikaly, P. E. (2020). Microbial electrosynthesis from CO2: challenges, opportunities and perspectives in the context of circular bioeconomy. Bioresour. Technol. 302:122863. doi: 10.1016/j.biortech.2020.122863
Bird, L. J., Saraiva, I. H., Park, S., Calçada, E. O., Salgueiro, C. A., Nitschke, W., et al. (2014). Nonredundant roles for cytochrome c2 and two high-potential iron-sulfur proteins in the photoferrotroph rhodopseudomonas palustris TIE-1. J. Bacteriol. 196, 850–858. doi: 10.1128/JB.00843-13
Blanchet, E., Duquenne, F., Rafrafi, Y., Etcheverry, L., Erable, B., and Bergel, A. (2015). Importance of the hydrogen route in up-scaling electrosynthesis for microbial CO 2 reduction. Energy Environ. Sci. Energy Environ. Sci. 8, 3731–3744. doi: 10.1039/c5ee03088a
Chen, L., Tremblay, P. L., Mohanty, S., Xu, K., and Zhang, T. (2016). Electrosynthesis of acetate from CO2 by a highly structured biofilm assembled with reduced graphene oxide-tetraethylene pentamine. J. Mater. Chem. A 4, 8395–8401. doi: 10.1039/c6ta02036d
Cui, M., Nie, H., Zhang, T., Lovley, D., and Russell, T. P. (2017). Three-dimensional hierarchical metal oxide-carbon electrode materials for highly efficient microbial electrosynthesis. Sustain. Energy Fuels 1, 1171–1176. doi: 10.1039/c7se00073a
Das, S., Diels, L., Pant, D., Patil, S. A., and Ghangrekar, M. M. (2020). Review—microbial electrosynthesis: a way towards the production of electro-commodities through carbon sequestration with microbes as biocatalysts. J. Electrochem. Soc. 167:155510. doi: 10.1149/1945-7111/abb836
Dessì, P., Rovira-Alsina, L., Sánchez, C., Dinesh, G. K., Tong, W., Chatterjee, P., et al. (2021). Microbial electrosynthesis: towards sustainable biorefineries for production of green chemicals from CO2 emissions. Biotechnol. Adv. 46:107675. doi: 10.1016/j.biotechadv.2020.107675
Deutzmann, J. S., Sahin, M., and Spormann, A. M. (2015). Extracellular enzymes facilitate electron uptake in biocorrosion. mBio 6:e00496-15. doi: 10.1128/mBio.00496-15
Falcke, H., Holbrook, S., Clenahan, I., Carretero, A. L., and Sanalan, T. (2017). Best Available Techniques (BAT) Reference Document for the Production of Large Volume Organic Chemicals. Brussels: European Comission.
Faraghiparapari, N., and Zengler, K. (2017). Production of organics from CO2 by microbial electrosynthesis (MES) at high temperature. J. Chem. Technol. Biotechnol. 92, 375–381. doi: 10.1002/jctb.5015
Flexer, V., and Jourdin, L. (2020). Purposely designed hierarchical porous electrodes for high rate microbial electrosynthesis of acetate from carbon dioxide. Accounts Chem. Res. 53, 311–321. doi: 10.1021/acs.accounts.9b00523
Fruehauf, H. M., Enzmann, F., Harnisch, F., Ulber, R., and Holtmann, D. (2020). Microbial electrosynthesis—an inventory on technology readiness level and performance of different process variants. Biotechnol. J. 15, 1–11. doi: 10.1002/biot.202000066
Giddings, C. G. S., Nevin, K. P., Woodward, T., Lovley, D. R., and Butler, C. S. (2015). Simplifying microbial electrosynthesis reactor design. Front. Microbiol. 6:468. doi: 10.3389/fmicb.2015.00468
Gildemyn, S., Verbeeck, K., Slabbinck, R., Andersen, S. J., Prévoteau, A., and Rabaey, K. (2015). Integrated production, extraction, and concentration of acetic acid from CO2 through microbial electrosynthesis. Environ. Sci. Technol. Lett. 2, 325–328. doi: 10.1021/acs.estlett.5b00212
Gong, Y., Ebrahim, A., Feist, A. M., Embree, M., Zhang, T., Lovley, D., et al. (2013). Sulfide-driven microbial electrosynthesis. Environ. Sci. Technol. 47, 568–573. doi: 10.1021/es303837j
Gupta, D., Sutherland, M. C., Rengasamy, K., Mark Meacham, J., Kranz, R. G., and Bose, A. (2019). Photoferrotrophs produce a PioAB electron conduit for extracellular electron uptake. mBio 10:e02668-19. doi: 10.1128/mBio.02668-19
Hess, V., Schuchmann, K., and Müller, V. (2013). The ferredoxin: NAD+ Oxidoreductase (Rnf) from the acetogen acetobacterium woodii requires na+ and is reversibly coupled to the membrane potential. J. Biol. Chem. 288, 31496–31502. doi: 10.1074/jbc.M113.510255
Hoffman, B. M., Dean, D. R., and Seefeldt, L. C. (2009). Climbing nitrogenase: toward a mechanism of enzymatic nitrogen fixation. Accounts Chem. Res. 42, 609–619. doi: 10.1021/ar8002128
Igarashi, K., and Kato, S. (2021). Reductive transformation of Fe(III) (oxyhydr)oxides by mesophilic homoacetogens in the genus Sporomusa. Front. Microbiol. 12:600808. doi: 10.3389/fmicb.2021.600808
Izadi, P., Fontmorin, J., and Godain, A. (2020). Parameters influencing the development of highly conductive and effi cient biofilm during microbial electrosynthesis: the importance of applied potential and inorganic carbon source. NPJ Biofilms Microbiomes 6:40. doi: 10.1038/s41522-020-00151-x
Jin, Z., Pan, Z., Yu, S., and Lin, C. (2010). Experimental study on pressurized activated sludge process for high concentration pesticide wastewater. J. Environ. Sci. 22, 1342–1347. doi: 10.1016/S1001-0742(09)60260-6
Jourdin, L., and Burdyny, T. (2020). Microbial electrosynthesis: where do we go from here? Trends Biotechnol. 39, 359–369. doi: 10.1016/j.tibtech.2020.10.014
Jourdin, L., Lu, Y., Flexer, V., Keller, J., and Freguia, S. (2016). Biologically induced hydrogen production drives high rate/high efficiency microbial electrosynthesis of acetate from carbon dioxide. ChemElectroChem 3, 581–591. doi: 10.1002/celc.201500530
Jourdin, L., Sousa, J., van Stralen, N., and Strik, D. P. B. T. B. (2020). Techno-economic assessment of microbial electrosynthesis from CO 2 and/or organics: an interdisciplinary roadmap towards future research and application. Appl. Energy 279:115775. doi: 10.1016/j.apenergy.2020.115775
Kamlage, B., Boelter, A., and Blaut, M. (1993). Spectroscopic and potentiometric characterization of cytochromes in two Sporomusa species and their expression during growth on selected substrates. Arch. Microbiol. 159, 189–196. doi: 10.1007/BF00250281
Karthikeyan, R., Singh, R., and Bose, A. (2019). Microbial electron uptake in microbial electrosynthesis: a mini-review. J. Ind. Microbiol. Biotechnol. 46, 1419–1426. doi: 10.1007/s10295-019-02166-6
Kato, S., Yumoto, I., and Kamagata, Y. (2015). Isolation of acetogenic bacteria that induce biocorrosion by utilizing metallic iron as the sole electron donor. Appl. Environ. Microbiol. 81, 67–73. doi: 10.1128/AEM.02767-14
Kerzenmacher, S. (2017). “Engineering of microbial electrodes,” in Advances in Biochemical Engineering/Biotechnology, eds T. Scheper and R. Ulber (Berlin: Springer), 1–46.
Koch, C., and Harnisch, F. (2016). Is there a specific ecological niche for electroactive microorganisms? ChemElectroChem 3, 1282–1295. doi: 10.1002/celc.201600079
Kracke, F., Wong, A. B., Maegaard, K., Deutzmann, J. S., Hubert, M. A., Hahn, C., et al. (2019). Robust and biocompatible catalysts for efficient hydrogen-driven microbial electrosynthesis. Commun. Chem. 2:45. doi: 10.1038/s42004-019-0145-0
Kremp, F., Roth, J., and Müller, V. (2020). The Sporomusa type Nfn is a novel type of electron - bifurcating transhydrogenase that links the redox pools in acetogenic bacteria. Sci. Rep. 10:14872. doi: 10.1038/s41598-020-71038-2
Krige, A., Rova, U., and Christakopoulos, P. (2021). 3D bioprinting on cathodes in microbial electrosynthesis for increased acetate production rate using Sporomusa ovata. J. Environ. Chem. Eng. 9:106189. doi: 10.1016/j.jece.2021.106189
Lehtinen, T., Efimova, E., Tremblay, P.-L., Santala, S., Zhang, T., and Santala, V. (2017). Production of long chain alkyl esters from carbon dioxide and electricity by a two-stage bacterial process. Bioresour. Technol. 243, 30–36.
Li, X., Angelidaki, I., and Zhang, Y. (2018). Salinity-gradient energy driven microbial electrosynthesis of value-added chemicals from CO2 reduction. Water Res. 142, 396–404. doi: 10.1016/j.watres.2018.06.013
Ljungdahl, L. G. (2009). A life with acetogens, thermophiles, and cellulolytic anaerobes. Annu. Rev. Microbiol. 63, 1–25.
Louro, R. O., Costa, N. L., Fernandes, A. P., Silva, A. V., Trindade, I. B., Fonseca, B. M., et al. (2018). “Exploring the molecular mechanisms of extracellular electron transfer for harnessing reducing power in METs: methodologies and approaches,” in Biomass, Biofuels, Biochemicals: Microbial Electrochemical Technology: Sustainable Platform for Fuels, Chemicals and Remediation, eds S. V. Mohan, A. Pandey, and S. Varjani (Amsterdam: Elsevier), 261–293.
Luisa Rodrigues, M., Scott, K. A., Sansom, M. S. P., Pereira, I. A. C., and Archer, M. (2008). Quinol oxidation by c-type cytochromes: structural characterization of the menaquinol binding site of NrfHA. J. Mol. Biol. 381, 341–350. doi: 10.1016/j.jmb.2008.05.066
Marshall, C. W., Ross, D. E., Handley, K. M., Weisenhorn, P. B., Edirisinghe, J. N., Henry, C. S., et al. (2017). Metabolic reconstruction and modeling microbial electrosynthesis. Sci. Rep. 7:8391. doi: 10.1038/s41598-017-08877-z
McClane, B. A., Uzal, F. A., Fernandez Miyakawa, M. E., Lyerly, D., and Wilkins, T. (2006). “The enterotoxic clostridia,” in The Prokaryotes, eds M. Dworkin, S. Falkow, E. Rosenberg, K. H. Schleifer, and E. Stackebrandt (New York, NY: Springer). doi: 10.3390/toxins8050153
Milton, R. D., and Minteer, S. D. (2019). Nitrogenase bioelectrochemistry for synthesis applications. Accounts Chem. Res. 52, 3351–3360. doi: 10.1021/acs.accounts.9b00494
Moeller, B., Oßmer, R., Howard, B., Gottschalk, G., and Hippe, H. (1984). Sporomusa, a new genus of gram-negative anaerobic bacteria including Sporomusa sphaeroides spec. nov. and Sporomusa ovata spec. nov. * Bernhard. Arch. Microbiol. 139, 388–396. doi: 10.2166/wst.2010.137
Mohanakrishna, G., Vanbroekhoven, K., and Pant, D. (2016). Imperative role of applied potential and inorganic carbon source on acetate production through microbial electrosynthesis. J. CO2 Utiliz. 15, 57–64. doi: 10.1016/j.jcou.2016.03.003
Molitor, B., Kirchner, K., Henrich, A. W., Schmitz, S., and Rosenbaum, M. A. (2016). Expanding the molecular toolkit for the homoacetogen Clostridium ljungdahlii. Sci. Rep. 6:31518. doi: 10.1038/srep31518
Müller, V. (2003). Energy conservation in acetogenic bacteria. Appl. Environ. Microbiol. 69, 6345–6353. doi: 10.1128/AEM.69.11.6345-6353.2003
Müller, V. (2019). New horizons in acetogenic conversion of one-carbon substrates and biological hydrogen storage. Trends Biotechnol. 37, 1344–1354. doi: 10.1016/j.tibtech.2019.05.008
Nevin, K. P., Woodard, T. L., and Franks, A. E. (2010). Microbial electrosynthesis: feeding microbes electricity to convert carbon dioxide and water to multicarbon extracellular organic compounds. mBio 1:e00103-10. doi: 10.1128/mBio.00103-10.Editor
Philips, J. (2020). Extracellular electron uptake by acetogenic bacteria: does H2 consumption favor the H2 evolution reaction on a cathode or metallic iron? Front. Microbiol. 10:2997. doi: 10.3389/fmicb.2019.02997
Prévoteau, A., Carvajal-Arroyo, J. M., Ganigué, R., and Rabaey, K. (2020). Microbial electrosynthesis from CO2: forever a promise? Curr. Opin. Biotechnol. 62, 48–57. doi: 10.1016/j.copbio.2019.08.014
Rabaey, K., and Rozendal, R. A. (2010). Microbial electrosynthesis - revisiting the electrical route for microbial production. Nat. Rev. Microbiol. 8, 706–716. doi: 10.1038/nrmicro2422
Rodrigues, M. L., Oliveira, T. F., Pereira, I. A. C., and Archer, M. (2006). X-ray structure of the membrane-bound cytochrome c quinol dehydrogenase NrfH reveals novel haem coordination. EMBO J. 25, 5951–5960. doi: 10.1038/sj.emboj.7601439
Rodrigues, R. M., Guan, X., Iñiguez, J. A., Estabrook, D. A., Chapman, J. O., Huang, S., et al. (2019). Perfluorocarbon nanoemulsion promotes the delivery of reducing equivalents for electricity-driven microbial CO2 reduction. Nat. Catalysis 2, 407–414. doi: 10.1038/s41929-019-0264-0
Sakimoto, K. K., Liu, C., Lim, J., and Yang, P. (2014). Salt-induced self-assembly of bacteria on nanowire arrays. Nano Lett. 14, 5471–5476. doi: 10.1021/nl502946j
Saraiva, I. H., Newman, D. K., and Louro, R. O. (2012). Functional characterization of the FoxE iron oxidoreductase from the photoferrotroph rhodobacter ferrooxidans SW2. J. Biol. Chem. 287, 25541–25548. doi: 10.1074/jbc.M112.360636
Schuchmann, K., and Müller, V. (2014). Autotrophy at the thermodynamic limit of life: a model for energy conservation in acetogenic bacteria. Nat. Rev. Microbiol. 12, 809–821. doi: 10.1038/nrmicro3365
Schuchmann, K., and Müller, V. (2016). Energetics and application of heterotrophy in acetogenic bacteria. Appl. Environ. Microbiol. 82, 4056–4069. doi: 10.1128/AEM.00882-16
Shi, X. C., Tremblay, P. L., Wan, L., and Zhang, T. (2021). Improved robustness of microbial electrosynthesis by adaptation of a strict anaerobic microbial catalyst to molecular oxygen. Sci. Total Environ. 754:142440. doi: 10.1016/j.scitotenv.2020.142440
Stackebrandt, E., Pohla, H., Kroppenstedt, R., Hippe, H., and Woese, C. R. (1985). 16S rRNA analysis of Sporomusa, selenomonas, and Megasphaera: on the phylogenetic origin of gram-positive Eubacteria. Arch. Microbiol. 143, 270–276. doi: 10.1007/BF00411249
Sydow, A., Krieg, T., Mayer, F., Schrader, J., and Holtmann, D. (2014). Electroactive bacteria—molecular mechanisms and genetic tools. Appl. Microbiol. Biotechnol. 98, 8481–8495. doi: 10.1007/s00253-014-6005-z
Tremblay, P., Faraghiparapari, N., and Zhang, T. (2019). Accelerated H 2 evolution during electrosynthesis with Sporomusa ovata. Catalysts 9:166. doi: 10.3390/catal9020166
Tremblay, P. L., Höglund, D., Koza, A., Bonde, I., and Zhang, T. (2015). Adaptation of the autotrophic acetogen Sporomusa ovata to methanol accelerates the conversion of CO2 to organic products. Sci. Rep. 5:16168. doi: 10.1038/srep16168
Tremblay, P. L., Zhang, T., Dar, S. A., Leang, C., and Lovley, D. R. (2013). The Rnf complex of Clostridium ljungdahlii is a proton-translocating ferredoxin: NAD+ oxidoreductase essential for autotrophic growth. mBio 4:e00406-12. doi: 10.1128/mBio.00406-12
Tsurumaru, H., Ito, N., Mori, K., Wakai, S., Uchiyama, T., Iino, T., et al. (2018). An extracellular [NiFe] hydrogenase mediating iron corrosion is encoded in a genetically unstable genomic island in Methanococcus maripaludis. Sci. Rep. 8:15149. doi: 10.1038/s41598-018-33541-5
Vidra, A., and Nemeth, A. (2018). Bio-produced acetic acid: a review. Period. Polytechn. Chem. Eng. 62, 245–256. doi: 10.3311/PPch.11004
Visser, M., Pieterse, M. M., Pinkse, M. W., Nijsse, B., Verhaert, P. D., de Vos, W. M., et al. (2016). Unravelling the one-carbon metabolism of the acetogen Sporomusa strain An4 by genome and proteome analysis. Environ. Microbiol. 18, 2843–2855. doi: 10.1111/1462-2920.12973
Keywords: microbial electrosynthesis, Sporomusa ovata, electron uptake mechanisms, microbial cathodes, c-type cytochromes
Citation: Madjarov J, Soares R, Paquete CM and Louro RO (2022) Sporomusa ovata as Catalyst for Bioelectrochemical Carbon Dioxide Reduction: A Review Across Disciplines From Microbiology to Process Engineering. Front. Microbiol. 13:913311. doi: 10.3389/fmicb.2022.913311
Received: 05 April 2022; Accepted: 23 May 2022;
Published: 20 June 2022.
Edited by:
Sunil A. Patil, Indian Institute of Science Education and Research Mohali, IndiaReviewed by:
Tian Zhang, Wuhan University of Technology, ChinaFederico Aulenta, Water Research Institute, National Research Council, Italy
Miriam A. Rosenbaum, Leibniz Institute for Natural Product Research and Infection Biology, Germany
Copyright © 2022 Madjarov, Soares, Paquete and Louro. This is an open-access article distributed under the terms of the Creative Commons Attribution License (CC BY). The use, distribution or reproduction in other forums is permitted, provided the original author(s) and the copyright owner(s) are credited and that the original publication in this journal is cited, in accordance with accepted academic practice. No use, distribution or reproduction is permitted which does not comply with these terms.
*Correspondence: Joana Madjarov, am9hbmEubWFkamFyb3ZAaXRxYi51bmwucHQ=