- Institute of Biology, Biotechnology and Environmental Protection, Faculty of Natural Sciences, University of Silesia in Katowice, Katowice, Poland
Plants have co-evolved with diverse microorganisms that have developed different mechanisms of direct and indirect interactions with their host. Recently, greater attention has been paid to a direct “message” delivery pathway from bacteria to plants, mediated by the outer membrane vesicles (OMVs). OMVs produced by Gram-negative bacteria play significant roles in multiple interactions with other bacteria within the same community, the environment, and colonized hosts. The combined forces of innovative technologies and experience in the area of plant–bacterial interactions have put pressure on a detailed examination of the OMVs composition, the routes of their delivery to plant cells, and their significance in pathogenesis, protection, and plant growth promotion. This review synthesizes the available knowledge on OMVs in the context of possible mechanisms of interactions between OMVs, bacteria, and plant cells. OMVs are considered to be potential stimulators of the plant immune system, holding potential for application in plant bioprotection.
Introduction
Interactions between plants and microorganisms constitute an integral element of each terrestrial ecosystem. A plant, being considered to be a meta-organism, is colonized by a distinct microbiome characterized by a diverse range of bacterial species. The coevolution of microorganisms and plants has led to the development of specific mechanisms that enable both partners to recognize each other. Within the plant microbiome, endophytic bacteria, especially those with plant growth-promoting traits and pathogens, enter into the closest interactions with their host plant through various direct and indirect molecular mechanisms. One of the direct tools that Gram-negative bacteria use to interact with other bacteria and to invade or colonize plant cells is outer membrane vesicles (OMVs). Secretion of molecules via OMVs is an essential aspect of the biology of Gram-negative bacteria that offers distinct advantages over other types of secretion. Bacteria release OMVs to facilitate their interactions with the surrounding environment without expending energy to move. These small vesicles can enter the environmental niches and host cells that cannot be accessed by the whole bacterial cell because of their size and growth requirements and the activity of the hosts’ defense mechanisms.
Most research on OMVs has been carried out on animal pathogens (Choi et al., 2017; Zhang et al., 2021; Li et al., 2022); however, the production of OMVs by plant-associated bacteria is predicted to be similarly intrinsic to their biology (Celluzzi and Masotti, 2016). Recent studies on plant–bacteria interactions have shown that OMVs perform many life-support functions for the parental cell and act as an adaptive toolbox in plant pathogenesis and stress (Feitosa-Junior et al., 2019; Bhar et al., 2021; Janda et al., 2021; McMillan et al., 2021).
Composition and Formation of Outer Membrane Vesicles
All the Gram-negative bacteria constitutively release small spherical OMVs, ranging in diameter from 20 to 300 nm, into the environment (Woith et al., 2019). OMV structure is closely related to the architecture of the cell envelope thus, the membrane of these nanovesicles contains the same basic components, such as proteins, lipids, and lipopolysaccharide (LPS) as the parental outer membrane (OM) (Avila-Calderón et al., 2021). However, more detailed structural analyses showed that OMV content can be enriched or depleted compared with the composition of the original bacterial envelope, indicating that OMVs and the OM structure are not identical (Schwechheimer and Kuehn, 2015; Turner et al., 2018). During vesiculation, an array of periplasmic biologically active components, metabolites, and parts of the inner membrane (IM) are trapped in OMV lumen or attached to OMVs as external material. Therefore, OMVs can carry complex cargos, including peptidoglycan (PGN), lipids, proteins, toxins, antibiotics, signaling molecules, and nucleic acids (Dorward and Garon, 1990; Renelli et al., 2004; Kuehn and Kesty, 2005). Being trapped inside OMVs, the soluble compounds are protected from degradation by environmental factors and extracellular enzymes released by other bacteria or plant host cells (Schwechheimer et al., 2014; Volgers et al., 2018; Caruana and Walper, 2020).
It is worth emphasizing that OMV cargo packaging may be a regulated process of sorting specific components into the vesicle lumen. The model of active sorting is based on the observation that the OMV production is not uniformly distributed across the OM but concentrates in distinct areas (Woith et al., 2019). However, the occurrence of targeted OMV cargo sorting requires firm experimental confirmation. Whether the composition of the OMV toolset is precisely selected rather than being just a microcosm of the components synthesized in a bacterial cell needs further elucidation.
Vesiculation is thought to be either a passive or active process that occurs in a well-conserved way; however, various types of OMVs are formed through different mechanisms. In general, nanovesicles are formed by bulging and then detaching fragments of the outer cell membrane. In addition, they can also arise from the lysis of the bacterial cells. It was observed that vesicles are produced when the synthesis of murein is slower than that of the OM, and the excess membrane forms OMVs (Klim and Godlewska, 2017). Although much research has been done on OMVs shedding, the precise mechanism of their release is not fully explained. LPS plays a crucial role in OMV biogenesis. LPS is composed of linearly arranged: lipid A–inner core–outer core–O-polysaccharide chain (LA–IC–OC–OPS). IC, OC, and OPS can vary in the length and composition of their carbohydrate chains (Roier et al., 2016). It was suggested that either the composition of the LPS, the lack of OPS, or mutations in the genes involved in OC or OPS synthesis result in increased OMV secretion and changes in their composition. Further truncations in the IC region can even lead to inhibition of vesiculation (Cahill et al., 2015; Liu et al., 2016). OMVs produced by the mutants with different alterations in LPS biosynthesis showed an increased number of proteins involved in post-translational modification and chaperons, accelerated protein turnover, and a decreased number of OM proteins in their cargo. One of the mechanisms proposed to explain OMV production is based on the repulsive charges of O-antigens in LPS molecules. Since some bacteria have LPS with a neutral charge, this model is characteristic only for certain bacterial species (Haurat et al., 2015).
Another model of OMV release involves the action of signal molecules produced by microorganisms that intercalate between the components of the OM, leading to the disorganization and folding of the membrane (Klim and Godlewska, 2017; Toyofuku et al., 2019). In some plant-colonizing bacteria, OMV production depends on the quorum-sensing (QS) signaling (Wagner-Döbler et al., 2005; Mookherjee et al., 2018). OMV release can be induced by the interaction between LPS and QS molecules such as quinolone and acetyl homoserine lactones (AHLs) (Lin et al., 2018). Another molecule, diffusible signal factor (DSF), is involved in OMV biogenesis and cargo packaging in the plant pathogens Xanthomonas oryzae and Xanthomonas campestris (Bahar et al., 2014).
PGN also plays a key role in OMV formation. Studies performed so far have shown that the disruption of connections between the OM and IM, and PGN accumulation, increase OMV production (Wessel et al., 2013; Ionescu et al., 2014; Paulsson et al., 2021). It is suspected that mutations affecting OM integrity cause accumulation of PGN in order to form more OMVs to release surface stress. Thus, the bulging and detachment of OMVs may occur in regions with relaxed OM–PGN linkage (YashRoy, 2017) that result from the reduced amount of proteins connecting the membrane with the PGN layer (e.g., OmpA, LppAB, TolA, TolB, Pal) (Wlodarczyk and Matlakowska, 2010; Klimentová and Stulík, 2015). Passive vesiculation can arise from the infection with bacteriophages that synthesize endolysins. Endolysins degrade PGN, leading to explosive lysis of the bacterial cells. Shattered membrane fragments formed after cell lysis self-assemble into vesicles that contain endolysin. These vesicles of lytic activity can further attach to neighboring bacterial cells and lead to their death (Toyofuku et al., 2019; Mandal et al., 2021).
Another hypothesis states that the overproduction of some proteins in bacterial cells causes the formation of OMVs (Klim and Godlewska, 2017). OMVs are naturally released during all the stages of bacterial cell growth in a liquid and solid culture; however, their production may increase under stress conditions and in the presence of antimicrobial compounds due to membrane bilayer destabilization and removal of misfolded periplasmic proteins (Manning and Kuehn, 2011; Haurat et al., 2015; Kulkarni et al., 2015). These accumulated proteins increase cell turgor pressure, resulting in local bulging of the OM. An intensive OMV shed has been observed in bacteria exposed to membrane-active antibiotics (i.e., polymyxin B, colistin, melittin), reactive oxygen species (ROSs), or grown under amino acid depletion (Van Peer et al., 1991; Toyofuku et al., 2019). OMVs can act as a shield protecting their host by sequestration of antimicrobial agents (Kulkarni et al., 2015). Bacteria cultured under unfavorable conditions may also produce OMVs with a cargo composition different from the control bacteria. The plant growth-promoting bacterium Pseudomonas chlororaphis O6 (PcO6) was exposed to CuO nanoparticles, and H2O2 released OMVs that contained higher relative concentrations of proteins, lipids, and nucleic acids than PcO6 cells (Potter et al., 2020).
Outer Membrane Vesicles as a Versatile Tool in Bacteria–Bacteria and Plant–Bacteria Interactions
Recent investigations of the significance of OMVs in bacterial interactions with the external environment have demonstrated that OMVs constitute pivotal mediators in the cross-talk among bacteria (Cahill et al., 2015). OMV-directed interactions may also occur among endophytes and plant pathogens during plant infection and further colonization. OMVs released by one bacterial species may function as public goods for the entire bacterial community; however, OMV-mediated interaction between bacteria can also be targeted specifically. Tashiro et al. (2009, 2017) have demonstrated that OMVs produced by Pseudomonas aeruginosa could attach to both Gram-negative and Gram-positive bacteria, while OMVs produced by Buttiauxella agrestis isolated from soil could be specifically delivered only to bacteria from the same genus. This specific fusion stemmed from a lower electrostatic repulsion between the OMVs and Buttiauxella spp. than between the OMVs and other Gram-negative bacterial species (Tashiro et al., 2017). Specific adhesion and internalization of OMVs to target bacteria requires particular surface-attached proteins, especially lipoproteins. For example, the small lipoprotein Atu8019 decorating OMVs produced by Agrobacterium tumefaciens is involved in OMV docking to specific bacteria. Homologs of this lipoprotein are constitutively exposed on the surface of the OMVs released by other Gram-negative pathogens, suggesting its conserved function in the targeted exchange of OMV cargo between bacteria (Knoke et al., 2020). The specific attachment and fusion of OMVs with the membranes of mammalian host cells have also been widely confirmed (Kuehn and Kesty, 2005; Bomberger et al., 2009; Vanaja et al., 2016). The occurrence of OMV fusion with a plant cell membrane remains an open question; however, we refer to this possibility in the following section.
One of the most significant functions of OMVs is their involvement in the intercellular signaling between bacteria. OMVs act as specialized carriers of hydrophobic QS signals, including Pseudomonas quinolone signals (PQS), AHLs, and DSFs (Mashburn and Whiteley, 2005; Lynch and Alegado, 2017; Brameyer et al., 2018; Feitosa-Junior et al., 2019). In line with this, biofilm-forming bacterial phenotypes are highly correlated with their intensive OMV production (Yonezawa et al., 2009; Bhar et al., 2021). OMVs produced by the phytopathogen, Xylella fastidiosa are the carriers of DSFs that regulate the switch between a less adhesive, motile phenotype, and more adherent non-motile cells, being modulators of bacterial invasiveness and pathogenicity (Feitosa-Junior et al., 2019). The motile, anti-adherent phenotype is predominant in dispersing bacterial cells throughout the plant. The adhesive phenotype is switched by a high DSF concentration during biofilm formation in xylem vessels (Chatterjee et al., 2008).
The recent studies have shown that OMVs originating from plant pathogens are involved in the secretion of siderophores to acquire iron from plant apoplasts (Feitosa-Junior et al., 2019; Janda et al., 2021). Janda et al. (2021) reported that siderophore transport proteins are upregulated in the OMVs of Pseudomonas syringae pv. tomato DC3000 in planta. OMVs shed by X. fastidiosa and X. campestris are enriched in TonB-dependent receptors that bind and transport siderophores (Feitosa-Junior et al., 2019). OMV-mediated siderophore secretion should also be considered to be an effective strategy of endophytes in competing for iron with pathogens and sharing the scavenged iron with a plant host (Yadav, 2018).
Outer membrane vesicle as carriers of enzymes and transporters can play a double role in plant bacterial colonization and nutrition (Lin et al., 2018). Phytopathogenic P. syringae pv. tomato T1 exports through OMVs chitinase, permeases, galactarate dehydratases, peptidases, and phytase, involved in the antagonism against pathogenic fungi, nitrogen, and phosphorous nutrition, and peptide uptake (Chowdhury and Jagannadham, 2013). Potentially, cell wall degrading enzymes may also be released in OMVs produced by plant endophytes to facilitate the plant cell wall degradation and further colonization (Fan et al., 2016; Afzal et al., 2017). OMV-associated enzymes break down substrates that can be utilized either by an OMV donor or other bacteria present in a community (Elhenawy et al., 2016; Valguarnera et al., 2018). The coordinated action of similar loci has been demonstrated in soil-inhabiting Bacteroidetes and the plant pathogens X. campestris pv. campestris and X. campestris pv. vesicatoria that produce OMVs enriched in xylan−deconstruction enzymes (Déjean et al., 2013; Solé et al., 2015; Larsbrink and McKee, 2020).
Several previous studies have shown that OMVs are involved in the inter- and intra-species DNA dissemination among bacteria (Dorward et al., 1989; Kolling and Matthews, 1999; Yaron et al., 2000; Chiura et al., 2011; Ho et al., 2015). Hypothetically, endophytes and plant pathogens could release nucleic acids associated with OMVs to facilitate horizontal gene transfer and plant colonization. Besides DNA, OMVs contain eRNA and small RNAs whose selective packaging into OMVs and capacity to modulate gene expression have been confirmed in the targeted cells of a mammalian host (Badi et al., 2020). RNA encapsulated in OMVs, protected from mechanical and enzymatic degradation, could be a significant tool for phytopathogens and endophytes in modulating plant response to infection/colonization by blocking mRNA translation or causing genome instability (Badi et al., 2020). Weiberg et al. (2013) and Wang et al. (2017), reported that the fungal pathogen Botrytis cinerea send virulent sRNAs via extracellular vesicles that could silence host-immunity genes. However, the packaging of sRNAs into OMVs of bacterial phytopathogens remains to be elucidated.
Endophytes and plant pathogens may excrete OMVs with enzymes that deactivate antibiotics produced by the competing microbes (Kumari Chikkode Narayana et al., 2017). Antibiotic-resistant bacteria produce OMVs containing a range of enzymes, such as β-lactamases and other hydrolases as well as scavenging proteins that act against membrane-active antibiotics (Kulkarni et al., 2014; Devos et al., 2015). OMV secretion is also considered to be a strategy developed by pathogens and endophytes to protect them from the antimicrobial environment inside a plant (Janda et al., 2021; McMillan et al., 2021). Phytopathogenic P. syringae pv. tomato DC3000 and Stenotrophomonas maltophilia, an opportunistic plant pathogen of soil origin, produce OMVs loaded with β-lactamases and cationic peptides, suggesting their potential role in the deactivation of plant-derived antimicrobial peptides and other metabolites (Devos et al., 2015; Janda et al., 2021). Pseudomonads should be of particular interest because they demonstrate high resistance to alkaloids, saponins, volatile oils, terpenoids, flavonoids, and carbohydrates (Othman et al., 2019). It has been found that soil-inhabiting Pseudomonas putida KT2440 produces OMVs that catabolize lignin-derived aromatic compounds (Salvachúa et al., 2020). Consequently, other strains may secrete OMVs equipped with enzymes and peptides that enable the degradation or deactivation of phenolic and other plant antimicrobial compounds. On the other hand, recent findings emphasize the role of the plant microbiome in producing host bioactive molecules. Since endophytic bacteria may represent an important source of antimicrobial plant secondary metabolites or their precursors (Castronovo et al., 2021) the role of OMVs as a carrier of these compounds should be considered in further research.
OMVs produced by plant pathogens may contain antioxidative enzymes such as catalase (CAT) and superoxide dismutase (SOD), acting as a protective shield against the ROS burst in plant cells. SOD was found in the OMVs shed by P. syringae pv. tomato T1 (Chowdhury and Jagannadham, 2013), whereas CAT was detected in the OMVs of Xanthomonas citri subsp. citri and Ralstonia solanacearum (Tondo et al., 2016, 2020). The presence of oxidative enzymes in the OMVs of other Xanthomonadaceae and Burkholderiaceae causing widespread damage in crop production requires further elucidation (Sidhu et al., 2008; Sousa et al., 2020).
Outer Membrane Vesicle-Associated Virulence Factors of Plant Pathogens
Outer membrane vesicles has been recognized as one of the key carriers of virulence factors (VFs) produced by plant pathogens that are involved in callose deposition, increased transcription of plant cell surface-localized receptors (PRRs), and enhanced ROS release in plants (Solé et al., 2015; Bahar et al., 2016; Tayi et al., 2016). Proteomic analysis of the OMVs secreted by X. campestris pv. campestris demonstrated that 42% of the proteins associated with OMVs are T3SS-associated effectors, including the translocon HrpF, the adhesin XvdA1, xylosidase, and avirulence proteins AvrBs1 and AvrBs2 that stunt the hypersensitive response (HR) in plants. Besides the enriched T3SS-dependent fraction, the OMVs of X. campestris pv. campestris consist of selected proteins associated with OM, cellulase, and β-glucosidase (Sidhu et al., 2008). On the other hand, using immunoelectron microscopy, Solé et al. (2015) observed that, instead of T3SS elements, the OMVs of X. campestris pv. vesicatoria assist in releasing T2SS effectors such as lipase, protease, and xylanase to the plant intracellular milieu. OMVs of another closely related pathogen, X. oryzae pv. oryzae are enriched in the OMP Ax21, processed by the general secretion system, which is necessary for bacterial motility and biofilm formation (Bahar et al., 2014). X. fastidiosa, a pathogen that does not possess T3SS, releases OMVs that act as nanovehicles loaded with a complete suite of VFs such as lipases/esterases, adhesins (XadH1, XadH2 XdA3), hemagglutinins, proteases, porins, and pectin-lyase such as protein (Ionescu et al., 2014; Feitosa-Junior et al., 2019). OMV-associated adhesins and hemagglutinins enable the attachment of OMVs to the plant cell wall to facilitate its degradation by lipases/esterases (LesA and LesB) and pectin-lyases at long distances from the bacterial cells (Ionescu et al., 2014; Nascimento et al., 2016; Feitosa-Junior et al., 2019). Moreover, the OMVs of X. fastidiosa were enriched in six types of porins, suggesting their capacity to secrete and internalize different cargo compounds (Feitosa-Junior et al., 2019). Proteomic analyses of the OMVs secreted by P. syringae pv. tomato T1 and DC3000 showed that they also release multiple T3SS effectors defining their invasiveness in plants, including the proteins VrA1 and HopI1, which cause a hypersensitive response in plants, chlorosis-inducing toxin, coronatine, serine protease MucD, and glycoside hydrolases HopAJ2 and HopAH2-2 (Chowdhury and Jagannadham, 2013; Janda et al., 2021).
Analysis of the OMV cargo of these few phytopathogens indicates that despite close relatedness between some of them, the pathogens may use OMVs to customize their virulence strategies, involving effectors associated with different secretion systems. Plant endophytic bacteria could also abundantly release OMVs loaded with a wide diversity of tolerogenic and immunomodulatory molecules facilitating their plant colonization strategies (Bhar et al., 2021). Enterobacter cloacae, as an exceptionally ubiquitous bacterium, is a human intestine commensal, plant growth-promoting endophytic bacterium and opportunistic pathogen of humans and plants (Macedo-Raygoza et al., 2019; Bhar et al., 2021). Proteomic analysis has shown that in vitro cultured E. cloacae secretes OMVs holding a toolset customized to the colonization of a particular niche, which enables the conversion of this bacterium from the commensal to the pathogen. Cargo components of these OMVs include OmpX, adhesins, glycolytic, pectinolytic, and proteolytic enzymes, genetic material and extracellular polysaccharides that promote invasiveness and build the scaffold of biofilm matrix (Bhar et al., 2021).
Hypothetical Pathways of OMV Interactions With a Plant Cell
It is well-known that the plant cell wall is a passive barrier to plant–microbe interactions; however, bacterial OMVs could pass it during its relaxation associated with plant cell growth (Katsir and Bahar, 2017). We consider three hypothetic pathways of OMV interactions with plant cells, pointing out that plant cell wall relaxation is accompanied by a decrease in pH (Cosgrove, 2014) and that the cell wall forms a continuum with the plasma membrane and cytoskeleton (McKenna et al., 2014). The first model assumes the recognition of OMV antigens by plant cell surface-localized receptors, the second relies on the lysis and release of the OMV enzymes into the apoplast followed by the loss of plant cell wall integrity, and the third one predicts the direct internalization of OMVs in a clathrin-dependent or independent manner. Our hypotheses are partially consistent with the ‘spatial invasion model’, assuming that plant immunity is divided into apoplast-initiated and cytosolic immune responses (Kanyuka and Rudd, 2019).
The first possible way of the OMV interactions with plant cells could be associated with the recognition of microbe-associated molecular patterns (MAMPs) localized on the surface of OMVs by the PRRs followed by the activation of the basal resistance termed MAMP-triggered immunity (Bahar et al., 2016; Katsir and Bahar, 2017; McMillan et al., 2021) (Figure 1.1). MAMPs associated with OMVs are widely represented by LPS and PGN. Other MAMPs found in purified OMVs are elongation factor thermo unstable (EF–Tu), flagella (Kuehn and Kesty, 2005; Sidhu et al., 2008; Harvey et al., 2019), and putatively, RNA (Lee et al., 2015). OMV-associated MAMPs could be recognized by PRRs such as receptor-like kinases (RLKs) and receptor-like proteins (RLPs). OMV ligand recognition, followed by binding, and dimerization of PRRs, activates the cascade of intracellular immune signaling. Signal transduction is triggered by a strictly controlled process of phosphorylation of respiratory burst oxidase homolog protein D (RBOHD) by the intracellular domain of PRR dimers (Couto and Zipfel, 2016). RBOHD is an NADPH oxidase pivotal for plant defense responses, responsible for ROS production outside plant cells (Lee et al., 2020). The signaling cascade is initialized quasi-instantaneously to promote local and systemic responses in the plant. These processes can last up to several days and can be divided into early and late phases. The early phase includes ROS bursts, rapid ion flux, and increased cytoplasmic Ca2+ concentration followed by the stimulation of kinases (Demidchik et al., 2018; Zhang et al., 2020). The activation of mitogen-activated (MAPKs) and calcium-dependent protein kinases (CDPKs), which are responsible for propagating immune signals, triggers phosphorylation-induced signaling cascades resulting in the activation of WRKY transcription factors (WRKY TFs) in the nucleus. The effect of the activation of WRKY TFs occurring in the late phase of the immune signaling cascade is the reprogramming of gene expression leading to the final induction of the plant defense mechanism including callose deposition, nutrient relocation, the release of antimicrobial metabolites (phytoalexin), and initiation of plant defense hormone signaling (ethylene and jasmonic acid) (Meng and Zhang, 2013; Rodriguez et al., 2019; Zhang et al., 2020).
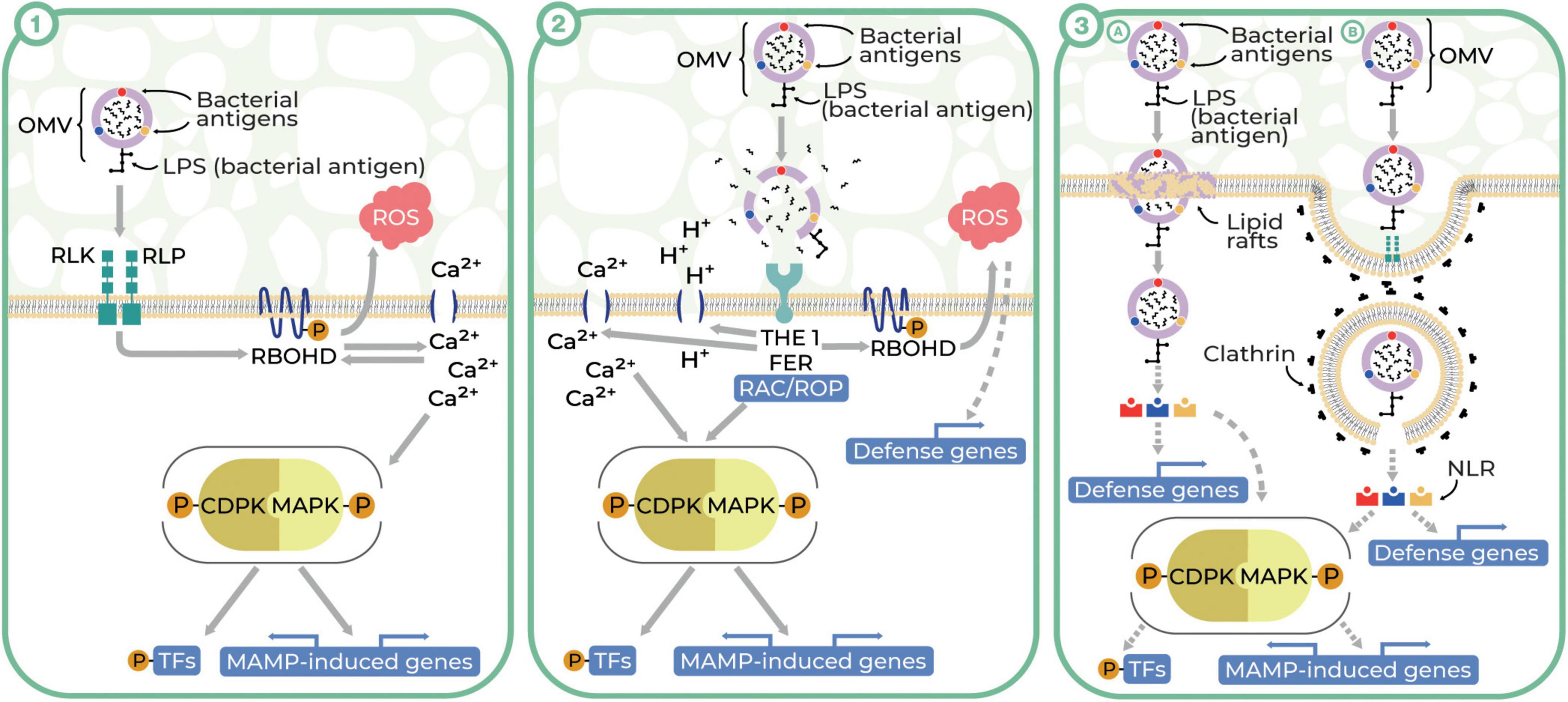
Figure 1. Three theoretical pathways of plant–OMV interactions (Illustrator: Maraszewska Małgorzata). (1) OMVs enter plant cells after recognition of OMV-associated MAMPs by RLKs and RLPs (PRRs) and activate PTI. The binding of MAMPs to PRRs results in their dimerization. The intracellular domain of PRR dimers phosphorylates RBOHD, leading to ROS bursts and rapid Ca2+ ion-flux. An increase in Ca2+ concentration activates CDPK and MAPK and co-regulates the activity of RBOHD. Activation of MAPKs and CDPKs induces cascades of immune signaling by phosphorylation, resulting the activation of transcription factors (TFs) in the nucleus and regulating gene expression. (2) OMVs lyse and release the cargo due to the acidic environment of the plant apoplast. Hydrolases released from OMV disturb CWI. CWI is controlled by plasma membrane-localized receptor-like kinases THE1 and FER. FER acts as a cell surface regulator for ROP/RAC GTPases responsible for activating RBOHD, ROS burst, changes in cytosolic pH and Ca2+ concentration, and also CDPK and MAPK activity. (3) OMVs may enter into the plant cells by the clathrin-independent pathway through lipid rafts (A) or clathrin-dependent endocytosis (B). The latter route begins with invagination of clathrin-coated pits (CCPs) with simultaneous recruitment of conserved adaptor protein complex 2. Mature clathrin-coated vesicles (CCVs) detach from the plasma membrane and move into the cytosol with the involvement of the cytoskeleton. The last stage of endocytosis is the uncoating and release of CCV cargo to the cytoplasm or transporting the CCV to the early endosome, where the fate of CCV is determined. In both pathways, the OMV cargo, being recognized by cytoplasmic receptors NLR (nucleotide-binding, leucine-rich repeat receptor), may activate MAMP-induced defense components (RBOHD, ROS burst, MAPKs, and CDPKs) or changes in defense genes’ expression.
The second potential way of OMV interactions with plant cells predicts that unfavorable acidic conditions in the plant apoplast may cause the lysis of OMVs followed by the release of their cargo (Rosa et al., 2019). As was shown in recent studies (Solé et al., 2015; Nascimento et al., 2016), OMV cargo may contain xylanases, cellulases, proteases, and lipases, which may contribute to the disruption of cell wall integrity (CWI), facilitating bacterial plant colonization (Ionescu et al., 2014). In CWI-controlled systems, plasma membrane-localized receptor-like kinases–THESEUS1 (THE1) and FERONIA (FER)–may be involved (Cheung and Wu, 2011; Feng et al., 2018; Engelsdorf et al., 2019; Ge et al., 2019; Ji et al., 2020). THE1 and FER can activate the components involved in various processes associated with plant defense such as other kinases, production of ROS, changes in cytosolic pH and Ca2+ concentration, and cell wall strengthening (Bacete et al., 2018; Rui and Dinneny, 2020) (Figure 1.2).
The third possible scenario involves OMV entry into plant cells through their direct internalization in plasmalemma in a clathrin-dependent or clathrin-independent manner. This scenario is in agreement with the ability of OMVs to enter mammalian cells by fusion in the course of macropinocytosis, caveolin-dependent, or clathrin-dependent endocytosis (O’Donoghue and Krachler, 2016; Rosa et al., 2019). We hypothesize that in the clathrin-independent pathway OMVs may enter plant cells through lipid rafts (plasma membrane domains composed of sterols and sphingolipids). In this model, plant-specific proteins, especially remorins, that play a role in intercellular signaling and plant defense, may be involved (Jacobson et al., 2007; Jarsch and Ott, 2011) [Figure 1.3(A)]. Notwithstanding, the implication of lipid rafts in endocytic processes in plant defense is not yet well understood (Leborgne-Castel et al., 2010; Iswanto and Kim, 2017) but was confirmed partially by Tran et al. (2022), who observed the interactions of OMVs with plant lipid nanodomains.
Clathrin-dependent endocytosis plays a crucial role in main physiological processes such as growth, development, nutrient uptake, and defense against pathogens (Barberon et al., 2011; Fan et al., 2015; Mbengue et al., 2016). Being a major mechanism of regulating plant responses to external stimuli and involved in local cell signaling, this type of endocytosis could be an important way of OMVs’ entry into plant cells. The first step in this process is the initiation of clathrin-coated pit (CCP) invasion. Mature clathrin-coated vesicles (CCVs) detach from the plasma membrane and move into the cytosol with the involvement of the cytoskeleton (Fujimoto et al., 2010; Yoshinari et al., 2016). The final step is the detachment and release of the OMVs into the cytoplasm (McMahon and Boucrot, 2011), where by binding with cytoplasmic receptors—nucleotide-binding, leucine-rich repeat receptors (NLRs) —OMVs may activate MAMP-induced defense components such as RBOHD, ROS burst, MAPKs, and CDPKs or changes in expression of defense genes (Yuan et al., 2021) [Figure 1.3(B)].
The selection of the proper pathway of OMV interactions with plant cells among these three putative models require further experimental elucidation. We suspect that the type of these interactions could be determined by the specificity of the structural and biochemical profiles of particular OMVs.
Future Research Directions
Understanding the mechanisms of plant–bacteria interactions is not complete without accounting for the significance of OMVs as mediators facilitating these interactions. The multitude of roles played by these extracellular organelles, from immune modulation to regulation of biofilms, nutrient acquisition, protein secretion, and detoxification (Chatterjee et al., 2008; Chowdhury and Jagannadham, 2013; McMillan et al., 2021), make them a multifunctional tool capable of responding to various bacterial requirements and environmental situations. The huge species diversity of plant-associated bacteria offers many possibilities for investigating the way in which bacteria utilize OMVs in specific interactions between bacteria and a host.
In this review, we summarized the research revealing that the OMV secretory pathway, universal for Gram-negative bacteria, maybe a smart and efficient way of delivering various compounds to a plant host. We suggested potential mechanisms of OMV interactions with a plant cell and described the diverse roles of OMVs in plant–bacterial interactions. Experimental verification of these mechanisms and many issues concerning the composition and fate of the OMVs released by plant-colonizing bacteria should be subjected to further research. It is essential to elucidate in detail: (1) whether the selection of OMV cargo components can be regulated by a bacterium; (2) how OMVs interact with the plant cell and how they pass the cell wall and enter the cytoplasm; (2) how OMV cargo is released inside the host cell; (3) the fate of functional OMV components inside the plant cell; (4) how these components influence plant immunity. Resolving these questions would open new opportunities to consider OMVs as potential boosters of plant immunity that could support plant defense against pathogens.
Author Contributions
MR, MN, MM, KK, and MP: conceptualization and writing—original draft. MR, MN, MM, and ZP-S: writing—review and editing. ZP-S: supervision and funding acquisition. All authors contributed to the article and approved the submitted version.
Funding
The conceptualization and writing of this article were supported by the National Science Centre in Poland [Grant number 2020/37/B/NZ8/00855].
Conflict of Interest
The authors declare that the research was conducted in the absence of any commercial or financial relationships that could be construed as a potential conflict of interest.
Publisher’s Note
All claims expressed in this article are solely those of the authors and do not necessarily represent those of their affiliated organizations, or those of the publisher, the editors and the reviewers. Any product that may be evaluated in this article, or claim that may be made by its manufacturer, is not guaranteed or endorsed by the publisher.
Acknowledgments
We would like to express our gratitude to Małgorzata Maraszewska from the Academy of Fine Arts in Katowice, Poland, who designed the graphical background for Figure 1 in this article.
References
Afzal, I., Iqrar, I., Shinwari, Z. K., and Yasmin, A. (2017). Plant growth-promoting potential of endophytic bacteria isolated from roots of wild Dodonaea viscosa L. Plant Growth Regul. 81, 399–408. doi: 10.1007/s10725-016-0216-5
Avila-Calderón, E. D., Ruiz-Palma, M. S., Aguilera-Arreola, M. G., Velázquez-Guadarrama, N., Ruiz, E. A., Gomez-Lunar, Z., et al. (2021). Outer membrane vesicles of Gram-negative bacteria: an outlook on biogenesis. Front. Microbiol. 12:557902. doi: 10.3389/fmicb.2021.557902
Bacete, L., Mélida, H., Miedes, E., and Molina, A. (2018). Plant cell wall-mediated immunity: cell wall changes trigger disease resistance responses. Plant J. 93, 614–636. doi: 10.1111/tpj.13807
Badi, S., Bruno, S. P., Moshiri, A., Tarashi, S., Siadat, S. D., and Masotti, A. (2020). Small RNAs in outer membrane vesicles and their function in host-microbe interactions. Front. Microbiol. 11:1209. doi: 10.3389/fmicb.2020.01209
Bahar, O., Mordukhovich, G., Luu, D. D., Schwessinger, B., Daudi, A., Jehle, A. K., et al. (2016). Bacterial outer membrane vesicles induce plant immune responses. Mol. Plant-Microbe Interact. 29, 374–384. doi: 10.1094/MPMI-12-15-0270-R
Bahar, O., Pruitt, R., Luu, D. D., Schwessinger, B., Daudi, A., Liu, F., et al. (2014). The Xanthomonas Ax21 protein is processed by the general secretory system and is secreted in association with outer membrane vesicles. PeerJ 2014, 1–14. doi: 10.7717/peerj.242
Barberon, M., Zelazny, E., Robert, S., Conejìeào, G., Curie, C., Friml, J., et al. (2011). Monoubiquitin-dependent endocytosis of the Iron-Regulated Transporter 1 (IRT1) transporter controls iron uptake in plants. Proc. Natl. Acad. Sci. U. S. A. 108, 1–9. doi: 10.1073/pnas.1100659108
Bhar, S., Edelmann, M. J., and Jones, M. K. (2021). Characterization and proteomic analysis of outer membrane vesicles from a commensal microbe, Enterobacter cloacae. J. Proteomics 231:103994. doi: 10.1016/j.jprot.2020.103994
Bomberger, J. M., MacEachran, D. P., Coutermarsh, B. A., Ye, S., O’Toole, G. A., and Stanton, B. A. (2009). Long-distance delivery of bacterial virulence factors by Pseudomonas aeruginosa outer membrane vesicles. PLoS Pathog. 5:1000382. doi: 10.1371/journal.ppat.1000382
Brameyer, S., Plener, L., Müller, A., Klingl, A., Wanner, G., and Jung, K. (2018). Outer membrane vesicles facilitate trafficking of the hydrophobic signaling molecule CAI-1 between Vibrio harveyi cells. J. Bacteriol. 200, e00740–17. doi: 10.1128/JB.00740-17
Cahill, B. K., Seeley, K. W., Gutel, D., and Ellis, T. N. (2015). Klebsiella pneumoniae O antigen loss alters the outer membrane protein composition and the selective packaging of proteins into secreted outer membrane vesicles. Microbiol. Res. 180, 1–10. doi: 10.1016/j.micres.2015.06.012
Caruana, J. C., and Walper, S. A. (2020). Bacterial membrane vesicles as mediators of microbe – microbe and microbe – host community interactions. Front. Microbiol. 11:432. doi: 10.3389/fmicb.2020.00432
Castronovo, L. M., Vassallo, A., Mengoni, A., Miceli, E., Bogani, P., Firenzuoli, F., et al. (2021). Medicinal plants and their bacterial microbiota: a review on antimicrobial compounds production for plant and human health. Pathogens 10:106. doi: 10.3390/pathogens10020106
Celluzzi, A., and Masotti, A. (2016). How our other genome controls our epi-genome. Trends Microbiol. 24, 777–787. doi: 10.1016/j.tim.2016.05.005
Chatterjee, S., Almeida, R. P. P., and Lindow, S. (2008). Living in two worlds: the plant and insect lifestyles of xylella fastidiosa. Annu. Rev. Phytopathol. 46, 243–271. doi: 10.1146/annurev.phyto.45.062806.094342
Cheung, A. Y., and Wu, H. M. (2011). THESEUS 1, FERONIA and relatives: a family of cell wall-sensing receptor kinases? Curr. Opin. Plant Biol. 14, 632–641. doi: 10.1016/j.pbi.2011.09.001
Chiura, H. X., Kogure, K., Hagemann, S., Ellinger, A., and Velimirov, B. (2011). Evidence for particle-induced horizontal gene transfer and serial transduction between bacteria. FEMS Microbiol. Ecol. 76, 576–591. doi: 10.1111/j.1574-6941.2011.01077.x
Choi, J. W., Kim, S. C., Hong, S. H., and Lee, H. J. (2017). Secretable small RNAs via outer membrane vesicles in periodontal pathogens. J. Dent. Res. 96, 458–466. doi: 10.1177/0022034516685071
Chowdhury, C., and Jagannadham, M. V. (2013). Virulence factors are released in association with outer membrane vesicles of Pseudomonas syringae pv. tomato T1 during normal growth. Biochim. Biophys. Acta Proteins Proteom. 1834, 231–239. doi: 10.1016/j.bbapap.2012.09.015
Cosgrove, D. J. (2014). “Plant Cell Growth and Elongatio,” in eLS, (Hoboken: John Wiley & Sons), 1–12. doi: 10.1002/9780470015902.a0001688.pub2
Couto, D., and Zipfel, C. (2016). Regulation of pattern recognition receptor signalling in plants. Nat. Rev. Immunol. 16, 537–552. doi: 10.1038/nri.2016.77
Déjean, G., Blanvillain-Baufumé, S., Boulanger, A., Darrasse, A., de Bernonville, T. D., Girard, A.-L., et al. (2013). The xylan utilization system of the plant pathogen Xanthomonas campestris pv campestris controls epiphytic life and reveals common features with oligotrophic bacteria and animal gut symbionts. New Phytol. 198, 899–915. doi: 10.1111/nph.12187
Demidchik, V., Shabala, S., Isayenkov, S., Cuin, T. A., and Pottosin, I. (2018). Calcium transport across plant membranes: mechanisms and functions. New Phytol. 220, 49–69. doi: 10.1111/nph.15266
Devos, S., Van Oudenhove, L., Stremersch, S., Van Putte, W., De Rycke, R., Van Driessche, G., et al. (2015). The effect of imipenem and diffusible signaling factors on the secretion of outer membrane vesicles and associated Ax21 proteins in Stenotrophomonas maltophilia. Front. Microbiol. 6:298. doi: 10.3389/fmicb.2015.00298
Dorward, D. W., and Garon, C. F. (1990). DNA is packaged within membrane-derived vesicles of Gram-negative but not Gram-positive bacteria. Appl. Environ. Microbiol. 56, 1960–1962. doi: 10.1128/aem.56.6.1960-1962.1990
Dorward, D. W., Garon, C. F., and Judd, R. C. (1989). Export and intercellular transfer of DNA via membrane blebs of Neisseria gonorrhoeae. J. Bacteriol. 171, 2499–2505. doi: 10.1128/jb.171.5.2499-2505.1989
Elhenawy, W., Bording-Jorgensen, M., Valguarnera, E., Haurat, M. F., Wine, E., and Feldman, M. F. (2016). LPS remodeling triggers formation of outer membrane vesicles in Salmonella. mBio 7:4. doi: 10.1128/mBio.00940-16
Engelsdorf, T., Kjaer, L., Gigli-Bisceglia, N., Vaahtera, L., Bauer, S., Miedes, E., et al. (2019). Functional characterization of genes mediating cell wall metabolism and responses to plant cell wall integrity impairment. BMC Plant Biol. 19:320. doi: 10.1186/s12870-019-1934-4
Fan, L., Li, R., Pan, J., Ding, Z., and Lin, J. (2015). Endocytosis and its regulation in plants. Trends Plant Sci. 20, 388–397. doi: 10.1016/j.tplants.2015.03.014
Fan, X., Yang, R., Qiu, S., Cai, X., Zou, H., and Hu, F. (2016). The endo-β-1,4-glucanase of Bacillus amyloliquefaciens is required for optimum endophytic colonization of plants. J. Microbiol. Biotechnol. 26, 946–952. doi: 10.4014/jmb.1512.12055
Feitosa-Junior, O. R., Stefanello, E., Zaini, P. A., Nascimento, R., Pierry, P. M., Dandekar, A. M., et al. (2019). Proteomic and metabolomic analyses of Xylella fastidiosa OMV-enriched fractions reveal association with virulence factors and signaling molecules of the DSF family. Phytopathology 109, 1344–1353. doi: 10.1094/PHYTO-03-19-0083-R
Feng, W., Kita, D., Peaucelle, A., Cartwright, H. N., Doan, V., Duan, Q., et al. (2018). The FERONIA receptor kinase maintains cell-wall integrity during salt stress through Ca2+ signaling. Curr. Biol. 28, 666–675.e5. doi: 10.1016/j.cub.2018.01.023
Fujimoto, M., Arimura, S. I., Ueda, T., Takanashi, H., Hayashi, Y., Nakano, A., et al. (2010). Arabidopsis dynamin-related proteins DRP2B and DRP1A participate together in clathrin-coated vesicle formation during endocytosis. Proc. Natl. Acad. Sci. U. S. A. 107, 6094–6099. doi: 10.1073/pnas.0913562107
Ge, Z., Dresselhaus, T., and Qu, L.-J. (2019). How CrRLK1L receptor complexes perceive RALF signals. Trends Plant Sci. 24, 978–981. doi: 10.1016/j.tplants.2019.09.002
Harvey, K. L., Jarocki, V. M., Charles, I. G., and Djordjevic, S. P. (2019). The diverse functional roles of elongation factor Tu (EF-Tu) in microbial pathogenesis. Front. Microbiol. 10:2351. doi: 10.3389/fmicb.2019.02351
Haurat, M. F., Elhenawy, W., and Feldman, M. F. (2015). Prokaryotic membrane vesicles: new insights on biogenesis and biological roles. Biol. Chem. 396, 95–109. doi: 10.1515/hsz-2014-0183
Ho, M.-H., Chen, C.-H., Goodwin, J. S., Wang, B.-Y., and Xie, H. (2015). Functional Advantages of Porphyromonas gingivalis Vesicles. PLoS One 10:0123448. doi: 10.1371/journal.pone.0123448
Ionescu, M., Zaini, P. A., Baccari, C., Tran, S., Da Silva, A. M., and Lindow, S. E. (2014). Xylella fastidiosa outer membrane vesicles modulate plant colonization by blocking attachment to surfaces. Proc. Natl. Acad. Sci. U. S. A. 111, E3910–E3918. doi: 10.1073/pnas.1414944111
Iswanto, A. B. B., and Kim, J. Y. (2017). Lipid raft, regulator of plasmodesmal callose homeostasis. Plants 6, 257–278. doi: 10.3390/plants6020015
Jacobson, K., Mouritsen, O. G., and Anderson, R. G. W. (2007). Lipid rafts: at a crossroad between cell biology and physics. Nat. Cell Biol. 9, 7–14. doi: 10.1038/ncb0107-7
Janda, M., Ludwig, C., Rybak, K., Meng, C., Stigliano, E., Botzenhardt, L., et al. (2021). Biophysical and proteomic analyses suggest functions of Pseudomonas syringae pv tomato DC3000 extracellular vesicles in bacterial growth during plant infection. biorxiv [Preprint]. doi: 10.1101/2021.02.08.430144
Jarsch, I. K., and Ott, T. (2011). Perspectives on remorin proteins, membrane rafts, and their role during plant-microbe interactions. Mol. Plant-Microbe Interact. 24, 7–12. doi: 10.1094/MPMI-07-10-0166
Ji, D., Chen, T., Zhang, Z., Li, B., and Tian, S. (2020). Versatile roles of the receptor-like kinase FERONIA in plant growth, development and host-pathogen interaction. Int. J. Mol. Sci. 21:7881. doi: 10.3390/ijms21217881
Kanyuka, K., and Rudd, J. J. (2019). Cell surface immune receptors: the guardians of the plant’s extracellular spaces. Curr. Opin. Plant Biol. 50, 1–8. doi: 10.1016/j.pbi.2019.02.005
Katsir, L., and Bahar, O. (2017). Bacterial outer membrane vesicles at the plant–pathogen interface. PLoS Pathog. 13:1006306. doi: 10.1371/journal.ppat.1006306
Klim, J. J., and Godlewska, R. (2017). Zastosowanie bakteryjnych pêcherzyków zewnątrzbłonowych w konstrukcji szczepionek. Postêpy Mikrobiol. 56, 43–55.
Klimentová, J., and Stulík, J. (2015). Methods of isolation and purification of outer membrane vesicles from gram-negative bacteria. Microbiol. Res. 170, 1–9. doi: 10.1016/j.micres.2014.09.006
Knoke, L. R., Abad Herrera, S., Götz, K., Justesen, B. H., Günther Pomorski, T., Fritz, C., et al. (2020). Agrobacterium tumefaciens small lipoprotein Atu8019 is involved in selective outer membrane vesicle (OMV) docking to bacterial cells. Front. Microbiol. 11:1228. doi: 10.3389/fmicb.2020.01228
Kolling, G. L., and Matthews, K. R. (1999). Export of virulence genes and Shiga toxin by membrane vesicles of Escherichia coli O157:H7. Appl. Environ. Microbiol. 65, 1843–1848. doi: 10.1128/aem.65.5.1843-1848.1999
Kuehn, M. J., and Kesty, N. C. (2005). Bacterial outer membrane vesicles and the host-pathogen interaction. Genes Dev. 19, 2645–2655. doi: 10.1101/gad.1299905
Kulkarni, H. M., Nagaraj, R., and Jagannadham, M. V. (2015). Protective role of E. coli outer membrane vesicles against antibiotics. Microbiol. Res. 181, 1–7. doi: 10.1016/j.micres.2015.07.008
Kulkarni, H. M., Swamy, C. V. B., and Jagannadham, M. V. (2014). Molecular characterization and functional analysis of outer membrane vesicles from the Antarctic bacterium Pseudomonas syringae suggest a possible response to environmental conditions. J. Proteome Res. 13, 1345–1358. doi: 10.1021/pr4009223
Kumari Chikkode Narayana, K., Bai Aswathanarayan, J., and Rai Vittal, R. (2017). Endophytic peptides-a source of therapeutic agents. Curr. Protein Pept. Sci. 18, 284–290. doi: 10.2174/1389203717666161025112121
Larsbrink, J., and McKee, L. S. (2020). “Bacteroidetes bacteria in the soil: Glycan acquisition, enzyme secretion, and gliding motility,” in Advances in Applied Microbiology, eds G. M. Gadd and S. Sariaslani (Cambridge, Massachusetts: Academic Press), 63–98. doi: 10.1016/bs.aambs.2019.11.001
Leborgne-Castel, N., Adam, T., and Bouhidel, K. (2010). Endocytosis in plant-microbe interactions. Protoplasma 247, 177–193. doi: 10.1007/s00709-010-0195-8
Lee, D., Lal, N. K., Lin, Z.-J. D., Ma, S., Liu, J., Castro, B., et al. (2020). Regulation of reactive oxygen species during plant immunity through phosphorylation and ubiquitination of RBOHD. Nat. Commun. 11:1838. doi: 10.1038/s41467-020-15601-5
Lee, J., Eschen-Lippold, L., Lassowskat, I., Böttcher, C., and Scheel, D. (2015). Cellular reprogramming through mitogen-activated protein kinases. Front. Plant Sci. 6:940. doi: 10.3389/fpls.2015.00940
Li, C., Wen, R., Mu, R., Chen, X., Ma, P., Gu, K., et al. (2022). Outer membrane vesicles of avian pathogenic Escherichia coli mediate the horizontal transmission of blaCTX-M-55. Pathogens 11:481. doi: 10.3390/pathogens11040481
Lin, J., Cheng, J., Wang, Y., and Shen, X. (2018). The Pseudomonas quinolone signal (PQS): not just for quorum sensing anymore. Front. Cell. Infect. Microbiol. 8:230. doi: 10.3389/fcimb.2018.00230
Liu, Q., Liu, Q., Yi, J., Liang, K., Liu, T., Roland, K. L., et al. (2016). Outer membrane vesicles derived from Salmonella typhimurium mutants with truncated LPS induce cross-protective immune responses against infection of Salmonella enterica serovars in the mouse model. Int. J. Med. Microbiol. 306, 697–706. doi: 10.1016/j.ijmm.2016.08.004
Lynch, J. B., and Alegado, R. A. (2017). Spheres of hope, packets of doom: the good and bad of outer membrane vesicles in interspecies and ecological dynamics. J. Bacteriol. 199, e00012–17. doi: 10.1128/JB.00012-17
Macedo-Raygoza, G. M., Valdez-Salas, B., Prado, F. M., Prieto, K. R., Yamaguchi, L. F., Kato, M. J., et al. (2019). Enterobacter cloacae, an endophyte that establishes a nutrient-transfer symbiosis with banana plants and protects against the black sigatoka pathogen. Front. Microbiol. 10:804. doi: 10.3389/fmicb.2019.00804
Mandal, P. K., Ballerin, G., Nolan, L. M., Petty, N. K., and Whitchurch, C. B. (2021). Bacteriophage infection of Escherichia coli leads to the formation of membrane vesicles via both explosive cell lysis and membrane blebbing. Microbiology 167:001021. doi: 10.1099/mic.0.001021
Manning, A. J., and Kuehn, M. J. (2011). Contribution of bacterial outer membrane vesicles to innate bacterial defense. BMC Microbiol. 11:258. doi: 10.1186/1471-2180-11-258
Mashburn, L. M., and Whiteley, M. (2005). Membrane vesicles traffic signals and facilitate group activities in a prokaryote. Nature 437, 422–425. doi: 10.1038/nature03925
Mbengue, M., Bourdais, G., Gervasi, F., Beck, M., Zhou, J., Spallek, T., et al. (2016). Clathrin-dependent endocytosis is required for immunity mediated by pattern recognition receptor kinases. Proc. Natl. Acad. Sci. U. S. A. 113, 11034–11039. doi: 10.1073/pnas.1606004113
McKenna, J. F., Tolmie, A. F., and Runions, J. (2014). Across the great divide: the plant cell surface continuum. Curr. Opin. Plant Biol. 22, 132–140. doi: 10.1016/j.pbi.2014.11.004
McMahon, H. T., and Boucrot, E. (2011). Molecular mechanism and physiological functions of clathrin-mediated endocytosis. Nat. Rev. Mol. Cell Biol. 12, 517–533. doi: 10.1038/nrm3151
McMillan, H. M., Zebell, S. G., Ristaino, J. B., Dong, X., and Kuehn, M. J. (2021). Protective plant immune responses are elicited by bacterial outer membrane vesicles. Cell Rep. 34:108645. doi: 10.1016/j.celrep.2020.108645
Meng, X., and Zhang, S. (2013). MAPK cascades in plant disease resistance signaling. Annu. Rev. Phytopathol. 51, 245–266. doi: 10.1146/annurev-phyto-082712-102314
Mookherjee, A., Singh, S., and Maiti, M. K. (2018). Quorum sensing inhibitors: can endophytes be prospective sources? Arch. Microbiol. 200, 355–369. doi: 10.1007/s00203-017-1437-3
Nascimento, R., Gouran, H., Chakraborty, S., Gillespie, H. W., Almeida-Souza, H. O., Tu, A., et al. (2016). The Type II secreted lipase/esterase LesA is a key virulence factor required for Xylella fastidiosa pathogenesis in grapevines. Sci. Rep. 6:18598. doi: 10.1038/srep18598
O’Donoghue, E. J., and Krachler, A. M. (2016). Mechanisms of outer membrane vesicle entry into host cells. Cell. Microbiol. 18, 1508–1517. doi: 10.1111/cmi.12655
Othman, L., Sleiman, A., and Abdel-Massih, R. M. (2019). Antimicrobial activity of polyphenols and alkaloids in middle eastern plants. Front. Microbiol. 10:911. doi: 10.3389/fmicb.2019.00911
Paulsson, M., Kragh, K. N., Su, Y. C., Sandblad, L., Singh, B., Bjarnsholt, T., et al. (2021). Peptidoglycan-binding anchor is a Pseudomonas aeruginosa OmpA family lipoprotein with importance for outer membrane vesicles, biofilms, and the periplasmic shape. Front. Microbiol. 12:639582. doi: 10.3389/fmicb.2021.639582
Potter, M., Hanson, C., Anderson, A. J., Vargis, E., and Britt, D. W. (2020). Abiotic stressors impact outer membrane vesicle composition in a beneficial rhizobacterium: raman spectroscopy characterization. Sci. Rep. 10:21289. doi: 10.1038/s41598-020-78357-4
Renelli, M., Matias, V., Lo, R. Y., and Beveridge, T. J. (2004). DNA-containing membrane vesicles of Pseudomonas aeruginosa PAO1 and their genetic transformation potential. Microbiology 150, 2161–2169. doi: 10.1099/mic.0.26841-0
Rodriguez, P. A., Rothballer, M., Chowdhury, S. P., Nussbaumer, T., Gutjahr, C., and Falter-Braun, P. (2019). Systems biology of plant-microbiome interactions. Mol. Plant 12, 804–821. doi: 10.1016/j.molp.2019.05.006
Roier, S., Zingl, F. G., Cakar, F., Durakovic, S., Kohl, P., Eichmann, T. O., et al. (2016). A novel mechanism for the biogenesis of outer membrane vesicles in Gram-negative bacteria. Nat. Commun. 7:10515. doi: 10.1038/ncomms10515
Rosa, P., Stigliano, E., and Poltronieri, P. (2019). “Foresight on nanovesicles in plant-pathogen interactions,” in Applied Plant Biotechnology for Improving Resistance to Biotic Stress, eds P. Poltronieri and Y. Hong (Cambridge, Massachusetts: Academic Press), 307–319. doi: 10.1016/B978-0-12-816030-5.00016-1
Rui, Y., and Dinneny, J. R. (2020). A wall with integrity: surveillance and maintenance of the plant cell wall under stress. New Phytol. 225, 1428–1439. doi: 10.1111/nph.16166
Salvachúa, D., Werner, A. Z., Pardo, I., Michalska, M., Black, B. A., Donohoe, B. S., et al. (2020). Outer membrane vesicles catabolize lignin-derived aromatic compounds in Pseudomonas putida KT2440. Proc. Natl. Acad. Sci. U. S. A. 117, 9302–9310. doi: 10.1073/pnas.1921073117
Schwechheimer, C., and Kuehn, M. J. (2015). Outer-membrane vesicles from Gram-negative bacteria: biogenesis and functions. Nat. Rev. Microbiol. 13, 605–619. doi: 10.1038/nrmicro3525
Schwechheimer, C., Kulp, A., and Kuehn, M. J. (2014). Modulation of bacterial outer membrane vesicle production by envelope structure and content. BMC Microbiol. 14:324. doi: 10.1186/s12866-014-0324-1
Sidhu, V. K., Vorhölter, F. J., Niehaus, K., and Watt, S. A. (2008). Analysis of outer membrane vesicle associated proteins isolated from the plant pathogenic bacterium Xanthomonas campestris pv. campestris. BMC Microbiol. 8:87. doi: 10.1186/1471-2180-8-87
Solé, M., Scheibner, F., Hoffmeister, A. K., Hartmann, N., Hause, G., Rother, A., et al. (2015). Xanthomonas campestris pv. vesicatoria secretes proteases and xylanases via the Xps type II secretion system and outer membrane vesicles. J. Bacteriol. 197, 2879–2893. doi: 10.1128/JB.00322-15
Sousa, S. A., Soares-Castro, P., Seixas, A. M. M., Feliciano, J. R., Balugas, B., Barreto, C., et al. (2020). New insights into the immunoproteome of B. cenocepacia J2315 using serum samples from cystic fibrosis patients. N. Biotechnol. 54, 62–70. doi: 10.1016/j.nbt.2019.08.006
Tashiro, Y., Hasegawa, Y., Shintani, M., Takaki, K., Ohkuma, M., Kimbara, K., et al. (2017). Interaction of bacterial membrane vesicles with specific species and their potential for delivery to target cells. Front. Microbiol. 8:571. doi: 10.3389/fmicb.2017.00571
Tashiro, Y., Ichikawa, S., Nakajima-Kambe, T., Uchiyama, H., and Nomura, N. (2009). Pseudomonas quinolone signal affects membrane vesicle production in not only Gram-negative but also Gram-positive bacteria. Microbes Environ. 25, 120–125. doi: 10.1264/jsme2.me09182
Tayi, L., Maku, R., Patel, H. K., and Sonti, R. V. (2016). Action of multiple cell wall-degrading enzymes is required for elicitation of innate immune responses during Xanthomonas oryzae pv. oryzae infection in rice. Mol. Plant-Microbe Interact. 29, 599–608. doi: 10.1094/MPMI-02-16-0039-R
Tondo, M. L., de Pedro-Jové, R., Vandecaveye, A., Piskulic, L., Orellano, E. G., and Valls, M. (2020). KatE from the bacterial plant pathogen Ralstonia solanacearum is a monofunctional catalase controlled by HrpG that plays a major role in bacterial survival to hydrogen peroxide. Front. Plant Sci. 11:1156. doi: 10.3389/fpls.2020.01156
Tondo, M. L., Delprato, M. L., Kraiselburd, I., Fernández Zenoff, M. V., Farías, M. E., and Orellano, E. G. (2016). KatG, the bifunctional catalase of Xanthomonas citri subsp. citri, responds to hydrogen peroxide and contributes to epiphytic survival on citrus leaves. PLoS One 11:0151657. doi: 10.1371/journal.pone.0151657
Toyofuku, M., Nomura, N., and Eberl, L. (2019). Types and origins of bacterial membrane vesicles. Nat. Rev. Microbiol. 17, 13–24. doi: 10.1038/s41579-018-0112-2
Tran, T. M., Chng, C.-P., Pu, X., Ma, Z., Han, X., Liu, X., et al. (2022). Potentiation of plant defense by bacterial outer membrane vesicles is mediated by membrane nanodomains. Plant Cell 34, 395–417. doi: 10.1093/plcell/koab276
Turner, L., Bitto, N. J., Steer, D. L., Lo, C., D’Costa, K., Ramm, G., et al. (2018). Helicobacter pylori outer membrane vesicle size determines their mechanisms of host cell entry and protein content. Front. Immunol. 9:1466. doi: 10.3389/fimmu.2018.01466
Valguarnera, E., Scott, N. E., Azimzadeh, P., and Feldman, M. F. (2018). Surface exposure and packing of lipoproteins into outer membrane vesicles are coupled processes in Bacteroides. mSphere 3, e00559–18. doi: 10.1128/mSphere.00559-18
Van Peer, R., Niemann, G. J., and Schippers, B. (1991). Induced resistance and phytoalexin accumulation in biological control of Fusarium wilt of carnation by Pseudomonas sp. strain WCS 417r. Phytopathology 81, 728–734.
Vanaja, S. K., Russo, A. J., Behl, B., Banerjee, I., Yankova, M., Deshmukh, S. D., et al. (2016). Bacterial outer membrane vesicles mediate cytosolic localization of LPS and caspase-11 activation. Cell 165, 1106–1119. doi: 10.1016/j.cell.2016.04.015
Volgers, C., Savelkoul, P. H. M., and Stassen, F. R. M. (2018). Gram-negative bacterial membrane vesicle release in response to the host-environment: different threats, same trick? Crit. Rev. Microbiol. 44, 258–273. doi: 10.1080/1040841X.2017.1353949
Wagner-Döbler, I., Thiel, V., Eberl, L., Allgaier, M., Bodor, A., Meyer, S., et al. (2005). Discovery of complex mixtures of novel long-chain quorum sensing signals in free-living and host-associated marine alphaproteobacteria. ChemBioChem 6, 2195–2206. doi: 10.1002/cbic.200500189
Wang, M., Weiberg, A., Dellota, E. Jr., Yamane, D., and Jin, H. (2017). Botrytis small RNA Bc-siR37 suppresses plant defense genes by cross-kingdom RNAi. RNA Biol. 14, 421–428. doi: 10.1080/15476286.2017.1291112
Weiberg, A., Wang, M., Lin, F.-M., Zhao, H., Zhang, Z., Kaloshian, I., et al. (2013). Fungal small RNAs suppress plant immunity by hijacking host RNA interference pathways. Science 342, 118–123. doi: 10.1126/science.1239705
Wessel, A. K., Liew, J., Kwon, T., Marcotte, E. M., and Whiteley, M. (2013). Role of Pseudomonas aeruginosa peptidoglycan-associated outer membrane proteins in vesicle formation. J. Bacteriol. 195, 213–219. doi: 10.1128/JB.01253-12
Wlodarczyk, A., and Matlakowska, R. (2010). Membrane vesicles-secretion and transport system in Grain-negative bacteria. Postep. Mikrobiol. 49, 305–315.
Woith, E., Fuhrmann, G., and Melzig, M. F. (2019). Extracellular vesicles—connecting kingdoms. Int. J. Mol. Sci. 20:5695. doi: 10.3390/ijms20225695
Yadav, A. N. (2018). Biodiversity and biotechnological applications of host-specific endophytic fungi for sustainable agriculture and allied sectors. Acta Sci. Microbiol. 1, 1–5.
Yaron, S., Kolling, G. L., Simon, L., and Matthews, K. R. (2000). Vesicle-mediated transfer of virulence genes from Escherichia coli O157: H7 to other enteric bacteria. Appl. Environ. Microbiol. 66, 4414–4420. doi: 10.1128/AEM.66.10.4414-4420.2000
YashRoy, R. C. (2017). “Outer membrane vesicles of gram-negative bacteria: nanoware for combat against microbes and macrobes,” in Nanostructures for Antimicrobial Therapy, eds A. Ficai and A. H. Grumezescu (Amsterdam: Elsevier Inc), 341–367. doi: 10.1016/B978-0-323-46152-8.00015-9
Yonezawa, H., Osaki, T., Kurata, S., Fukuda, M., Kawakami, H., Ochiai, K., et al. (2009). Outer membrane vesicles of Helicobacter pylori TK1402 are involved in biofilm formation. BMC Microbiol. 9:197. doi: 10.1186/1471-2180-9-197
Yoshinari, A., Fujimoto, M., Ueda, T., Inada, N., Naito, S., and Takano, J. (2016). DRP1-dependent endocytosis is essential for polar localization and boron-induced degradation of the borate transporter BOR1 in Arabidopsis thaliana. Plant Cell Physiol. 57, 1985–2000. doi: 10.1093/pcp/pcw121
Yuan, M., Jiang, Z., Bi, G., Nomura, K., Liu, M., Wang, Y., et al. (2021). Pattern-recognition receptors are required for NLR-mediated plant immunity. Nature 592, 105–109. doi: 10.1038/s41586-021-03316-6
Zhang, J., Coaker, G., Zhou, J. M., and Dong, X. (2020). Plant immune mechanisms: from reductionistic to holistic points of view. Mol. Plant 13, 1358–1378. doi: 10.1016/j.molp.2020.09.007
Keywords: endophytes, Gram-negative bacteria, induced systemic resistance, outer membrane vesicles, phytopathogens, plant-bacterial interactions, plant growth-promoting bacteria
Citation: Rudnicka M, Noszczyńska M, Malicka M, Kasperkiewicz K, Pawlik M and Piotrowska-Seget Z (2022) Outer Membrane Vesicles as Mediators of Plant–Bacterial Interactions. Front. Microbiol. 13:902181. doi: 10.3389/fmicb.2022.902181
Received: 22 March 2022; Accepted: 02 May 2022;
Published: 01 June 2022.
Edited by:
Anna Maria Pirttilä, University of Oulu, FinlandReviewed by:
Andrea Masotti, Bambino Gesù Children’s Hospital (IRCCS), ItalyM. Florencia Haurat, United States Food and Drug Administration, United States
Copyright © 2022 Rudnicka, Noszczyńska, Malicka, Kasperkiewicz, Pawlik and Piotrowska-Seget. This is an open-access article distributed under the terms of the Creative Commons Attribution License (CC BY). The use, distribution or reproduction in other forums is permitted, provided the original author(s) and the copyright owner(s) are credited and that the original publication in this journal is cited, in accordance with accepted academic practice. No use, distribution or reproduction is permitted which does not comply with these terms.
*Correspondence: Monika Malicka, bW9uaWthLm1hbGlja2FAdXMuZWR1LnBs