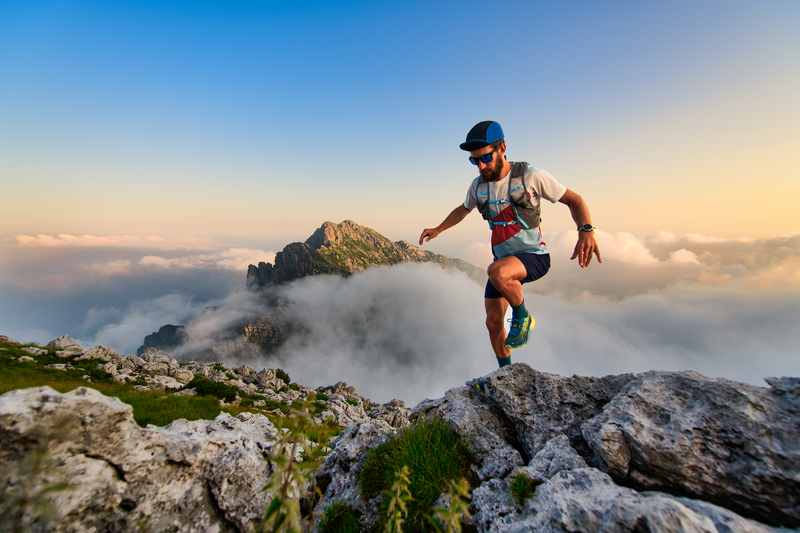
95% of researchers rate our articles as excellent or good
Learn more about the work of our research integrity team to safeguard the quality of each article we publish.
Find out more
REVIEW article
Front. Microbiol. , 20 May 2022
Sec. Infectious Agents and Disease
Volume 13 - 2022 | https://doi.org/10.3389/fmicb.2022.891573
This article is part of the Research Topic Frontiers in Malaria Research View all 7 articles
Malaria remains a vector-borne infectious disease that is still a major public health concern worldwide, especially in tropical regions. Malaria is caused by a protozoan parasite of the genus Plasmodium and transmitted through the bite of infected female Anopheles mosquitoes. The control interventions targeting mosquito vectors have achieved significant success during the last two decades and rely mainly on the use of chemical insecticides through the insecticide-treated nets (ITNs) and indoor residual spraying (IRS). Unfortunately, resistance to conventional insecticides currently being used in public health is spreading in the natural mosquito populations, hampering the long-term success of the current vector control strategies. Thus, to achieve the goal of malaria elimination, it appears necessary to improve vector control approaches through the development of novel environment-friendly tools. Mosquito microbiota has by now given rise to the expansion of innovative control tools, such as the use of endosymbionts to target insect vectors, known as “symbiotic control.” In this review, we will present the viral, fungal and bacterial diversity of Anopheles mosquitoes, including the bacteriophages. This review discusses the likely interactions between the vector microbiota and its fitness and resistance to insecticides.
Malaria remains the most challenging tropical disease caused by parasites of the genus Plasmodium, transmitted through the bite of infected female Anopheles mosquitoes [Müller, 2011; World Health Organization [WHO], 2020]. This disease prompts an intense perilous illness and represents a prominent well-being danger in the most affected regions. In sub-Saharan Africa, where the highest number of malaria cases and mortality rates are recorded, this illness leads to a life-threatening condition, especially among children under 5 years old and pregnant women [World Health Organization [WHO], 2020].
Four components are involved in the human malaria transmission system: (i) the protozoan parasite Plasmodium, (ii) the human host, (iii) the mosquito vector and (iv) a given environment (Castro, 2017). Among the strategies developed to control this disease, targeting the vector has significantly reduced malaria incidence across Africa [Bhatt et al., 2015; World Health Organization [WHO], 2020]. The current vector control programs rely mainly on the use of chemical insecticides through the insecticide-treated nets (ITNs) with pyrethroids and the indoor residual spraying (IRS) with organophosphates and carbamates. The purpose of these conventional tools is to reduce vector density below the threshold required for transmission or to prevent human-vector contact (Karunamoorthi, 2011). Mosquitoes belonging to the genus Anopheles are among the most important malaria vectors in endemic regions (Hay et al., 2010). In African countries, the dominant Anopheles vectors of human malaria include Anopheles gambiae, Anopheles arabiensis, Anopheles coluzzii and Anopheles funestus (Battle et al., 2012; Sinka et al., 2012; Coetzee et al., 2013) as well as the recently confirmed urban environment species Anopheles stephensi (Sinka et al., 2020). Unfortunately, the resistance against conventional insecticides currently used in public health is spreading in the natural mosquito populations. Indeed, there is an increasing number of evidence of insecticide resistance in malaria-transmitting vectors, and this phenomenon is threatening the success of malaria vector control programs (Mekuriaw et al., 2019).
Furthermore, African countries are taking a heavy toll on the Covid-19 pandemic. Indeed, this pandemic emerging from China by the end of December 2019 accounts for 6.3 million cases and 152,927 deaths in the entire African continent by August 12, 2021 [Salyer et al., 2021; World Health Organization [WHO], 2021]. Malaria and Covid-19 can have common clinical manifestations, including fever, tiredness and acute onset headache, leading to a misdiagnosis of malaria for Covid-19 and vice versa (Hussein et al., 2020). In addition, the Covid-19 is hampering the mass distribution of ITNs (Hussein et al., 2020). These situations might hinder the long-term global technical strategy of the World Health Organization (WHO) to reduce malaria case incidence from 222 (in 2021) to 23 per 1,000 populations by 2030, toward malaria elimination [World Health Organization [WHO], 2020]. To reach malaria elimination, it appears necessary to design and implement innovative and environment-friendly approaches to control malaria vectors (Gabrieli et al., 2021).
In such a situation, the mosquito microbiota has by now given rise to the expansion of innovative control tools, such as the use of endosymbionts known as “symbiotic control” (Ricci et al., 2012; Gabrieli et al., 2021). However, before implementing this control measure in natural Anopheles mosquito populations, it will be helpful to understand the bacterial diversity in these vectors and their interactions with their host. The present review is designed to provide an overview of the Anopheles microbiota and discuss the potential implications for the vector fitness, immune response and resistance to insecticides.
Mosquitoes often harbor a diverse and dynamic viral composition. Since mosquito-pathogenic microbes can be used for mosquito control (Huang et al., 2020), a better understanding of the natural and acquired viral communities infecting directly Anopheles mosquitoes cells, is expected to provide a background for developing novel biological tools for malaria control in endemic areas.
Studies have analyzed the virome of different Anopheles mosquitoes and have shown variations in viruses diversity and abundance between the mosquito species (Nanfack Minkeu and Vernick, 2018). Previous studies reported that Anopheles gambiae and Anopheles funestus mosquitoes ensure the biological transmission of the o’nyong-nyong arbovirus (ONNV) (Fauver et al., 2016; Nanfack Minkeu and Vernick, 2018; Belda et al., 2019). ONNV is the only known human pathogenic alphavirus with Anopheles vectors and is responsible for an epidemic febrile polyarthralgia. The symptoms are headaches, pruritic rash, lymphadenopathy, and conjunctivitis (Barrett and Weaver, 2012). Another virus, the densonucleosis virus (AgDNV) belonging to the Parvoviridae family (subfamily Densovirinae) was found to infect and disseminate in An. gambiae mosquitoes (Ren et al., 2008). The newly characterized AgDNV was demonstrated to be favorably transmissible to An. gambiae larvae; to spread to adult tissues and vertically transmitted to the offspring (Ren et al., 2008). Therefore, AgDNV represents a valuable tool for viral paratransgenesis (the genetic manipulation of mosquito symbiotic microorganisms) for malaria vector control. However, within Anopheles hosts, virus infection and replication could have significant side effects on several physiological traits of their bearers.
Overall, more than fifty different virus species belonging to at least thirteen main genera (Almendravirus, Alphavirus, Cripavirus, Cypovirus, Densovirus, Flavivirus, Iridovirus, Mononegavirus, Orbivirus, Orthobunyavirus, Phlebovirus, Poxvirus, and Totivirus) were found infecting diverse Anopheles mosquito species in the tropical countries of the world (virus species and Anopheles species infected were reviewed in Nanfack Minkeu and Vernick, 2018). Different other viruses are capable of infection, dissemination and transmission in Anopheles mosquitoes (see details in Table 1). Recently two novel viruses corresponding to emergent clades of insect-specific negative-strand single RNA viruses, Orthophasmavirus (Bunyavirales) and Anphevirus (Mononegavirales) were found in Anopheles mosquitoes. Orthophasmavirus was discovered in An. triannulatus (Scarpassa et al., 2019) and in Anopheles lutzi (da Silva Neves et al., 2021), while Anphevirus was found in An. marajoara, and An. darlingi (Scarpassa et al., 2019). In addition, another metagenomic sequencing work revealed ten other viruses infecting An. sinensis namely Culex Bunyavirus 1 (Phenuiviridae), Wutai mosquito phasivirus (Phenuiviridae), Wuhan Mosquito Virus 6 (Orthomyxoviridae), Hubei virga-like virus 1 (Tymoviridae), Yongsan picorna-like virus 4 (Iflaviridae), Wuhan Mosquito Virus 9 (Rhabdoviridae), Culex Y virus (Birnaviridae), Hubei virga-like virus 21 (Tymoviridae) Hubei picorna-like virus 58 (Tymoviridae), and Culex mononega-like virus 1 (Virgaviridae) (He et al., 2021). Furthermore, the porcine parvovirus (PPV) belonging to the genus Parvovirus (sub-family Parvovirinae, family Parvoviridae), was also identified in Anopheles mosquitoes including An. sinensis (Shi et al., 2015; Xia et al., 2018; Hameed et al., 2020).
These data show a high diversity of viral communities carried by Anopheles mosquitoes, which may have the potential to infect a wide range of hosts. Further studies are required to characterize these viruses species in terms of prevalence, pathogenicity, and transmission to the vertebrate host during blood-feeding.
To date, to our knowledge, no study has provided the specific function of viruses in Anopheles vectors. Since Anopheles mosquitoes are the primary vectors of malaria parasites in endemic countries, coinfection of viruses and Plasmodium could occur in the same mosquito vector. It is then possible that a virus infection could suppress other viruses’ replication or block the Plasmodium transmission. Thus, a better understanding of the virus-mosquito interactions requires detailed and specific investigations.
Among the wide range of pathogens capable of infecting Anopheles mosquitoes, there are poor reports on fungi species in the natural core microbiota of mosquito populations. Unlike bacteria, viruses, and Plasmodium parasites that need to be ingested by the mosquitoes before being transmitted to the vertebrate hosts, fungi infect mosquito hosts through the cuticle and afterward proliferate in the hemolymph (Mannino et al., 2019).
The interactions between entomopathogenic or non-entomopathogenic fungi and mosquitoes remain not well understood. Previous studies have found an Ascomycete fungus, Penicillium chrysogenum, a non-entomopathogenic species in the midgut of field-caught An. gambiae mosquitoes (Angleró-Rodríguez et al., 2016). In An. gambiae maintained in semi-field conditions, several other taxa were found at different developmental stages (larvae, pupae and adult) and in different tissues (ovary, midgut, body carcass). These fungi isolates included Hyphopichia burtonii, Hyphopichia sp., Penicillium georgiense, Periconia sp. Leptosphaerulina chartarum, Cladosporium cladosporioid, Hasegawazyma lactos, Epicoccum sp., Alternaria alternate, and Lichtheimia hyalospora (Nattoh et al., 2021). Some yeast isolates, including Meyerozyma guilliermondii, Rhodotorula glutinis, were also identified in the guts of laboratory strains of An. gambiae with M. guilliermondii also found in An. stephensi gut (Bozic et al., 2017). Most importantly, the opportunistic pathogen Candida parapsilosis, was also found in the gut of both An. gambiae and An. stephensi strains. In addition, C. parapsilosis was found at all developmental stages and in adult male and female guts and reproductive tissues (Bozic et al., 2017). Another yeast species Wickerhamomyces anomalus, was found to colonize immature stages (larvae and pupae) and adults of An. stephensi at different ages (Ricci et al., 2011). Like the yeasts reported by Bozic et al. (2017), W. anomalus was also shown to be localized in the midgut of both male and female An. stephensi reproductive systems (Ricci et al., 2011). However, fungi of the genus Aspergillus have been observed in the midgut of field-collected An. stephensi larvae but not in the adult mosquitoes (Tajedin et al., 2009), suggesting that Aspergillus might play a specific role at the larval stage in this mosquito species.
Some fungi species infecting mosquito vectors and their transmission to the subsequent developmental stages suggest a transstadial transmission, which could shape adult mosquito’s physiology and likely female insects’ susceptibility to the malaria parasite infection. Therefore, further works are needed to thoroughly understand the mosquito-mycobiota interactions.
Anopheles mosquitoes are colonized by a huge range of fungal microorganisms that may affect mosquito biology and vectorial capacity. According to the mosquito-mycobiota relationships, most fungi species are considered non-pathogenic (in commensal or symbiotic relationships) (Steyn et al., 2016) while some are pathogenic (developing parasitic interaction with mosquitoes) (Tawidian et al., 2019). Broad knowledge of the functions of fungi with the fitness of their mosquito host, as well as interactions with transmitted pathogens (especially Plasmodium parasites), is henceforth an important factor in the development and implementation of novel malaria vector control tools.
Although there are fewer reports on fungal species isolated from the natural microbiome community of mosquito populations, entomopathogenic fungi capable of experimentally infecting Anopheles vectors have been extensively studied (Bukhari et al., 2010; Howard et al., 2011; Valero-Jiménez et al., 2014). Indeed, it has been demonstrated that after topical infection, the entomopathogenic fungus Beauveria bassiana can interact with the gut bacteria in An. stephensi to accelerate the death of the mosquitoes (Wei et al., 2017). Both laboratory and wild-caught An. arabiensis were found susceptible to Beauveria bassiana infection regardless of their insecticide susceptibility (Kikankie et al., 2010). Using fungal suspensors, the authors found that exposure to B. bassiana spores reduced significantly the longevity of all mosquito colonies (Kikankie et al., 2010). Wild An. arabiensis, An. gambiae and An. funestus treated with mineral oil formulations of B. bassiana also resulted in a reduced lifespan of mosquitoes (Mnyone et al., 2012). Significant reduction in An. gambiae survival was also observed when mosquitoes were exposed to co-formulations of both M. anisopliae and B. bassiana fungus (Mnyone et al., 2009). The same trend was reported in An. gambiae exposed to dried conidia of M. anisopliae (Scholte et al., 2003) and in both An. gambiae and An. funestus exposed to low doses of conidia by direct contact (Farenhorst et al., 2008; Rhodes et al., 2018). M. anisopliae were able to infect wild An. gambiae s.l. and reduce mosquito longevity upon contact on M. anisopliae-impregnated black cotton sheets (Scholte et al., 2005). In laboratory conditions, it was shown that following mosquitoes infection, these fungi species can autodisseminate between the vectors through mating (Scholte et al., 2004). In addition, it was shown that age and blood-feeding status did not affect mosquito susceptibility to fungal infection (Mnyone et al., 2011). These findings are interesting as they could enhance the propagation of the fungal infection in mosquito populations when the biological malaria vector control based on these pathogens is implemented. However, further investigations are required to evaluate the autodissemination potential of the fungal community in the field populations of Anopheles vectors. The presence and the dissemination of some fungi into the reproductive organs of both male and female mosquitoes may suggest their potential involvement in mosquito reproduction and their likely vertical transmission (Ricci et al., 2011). Other mosquito life-history traits such as blood-feeding, flight ability, fecundity (Scholte et al., 2006; Blanford et al., 2011; Ondiaka et al., 2015); and host-seeking behavior (George et al., 2011) were also reported to be negatively affected following B. bassiana and M. anisopliae exposure. All these findings highlight the potential of fungi as biological control agents for malaria vector control.
Other fungi have been shown to contribute to the pathogen’s development in mosquitoes. However, it was reported that the presence of Pe. chrysogenum in the An. gambiae midgut does not affect mosquito survival, and it increases the mosquito susceptibility to Plasmodium infection by suppressing the host immune system (Angleró-Rodríguez et al., 2016). Conversely, Leptosphaerulina sp. was shown to activate the host immune system to induce melanization (production of melanin deposits) on the fat body in An. gambiae (Nattoh et al., 2021). Further knowledge regarding the interactions between host and pathogens is required to better understand how these fungi affect longevity and other mosquito life-history traits.
During the aquatic developmental period, bacteria constitute one of the major sources of nutrition for mosquito larvae (Walker et al., 1988). Numerous studies have demonstrated that mosquitoes host huge bacterial communities that vary according to the mosquito sex, developmental stage, and living environment (Minard et al., 2013). Endosymbionts have been proposed as a promising candidate to develop paratransgenesis approaches (Coutinho-Abreu et al., 2010). This prompts the urgent need to deeply understand the bacterial spectrum in malaria vectors.
As Anopheles mosquitoes are the definitive hosts responsible for malaria parasite transmission, the composition and diversity of the bacterial communities they host apart from Plasmodium species need to be taken into account. Several studies revealed the hugeness of bacterial diversity in Anopheles vectors (Table 2). It was reported the large presence of the uncultured Paenibacillaceae in male An. stephensis and Serratia marcescens in females and larvae individuals. The authors also reported that, unlike field-collected mosquitoes, Serratia marcescens and Cryseobacterium meninqosepticum bacteria were abundant in the laboratory-reared An. stephensis strain (Rani et al., 2009).
Studies have characterized the bacterial communities in larvae, pupae and adults of An. gambiae reared in semi-natural habitats. It was shown that photosynthetic Cyanobacteria were predominant in the larval and pupal guts while Proteobacteria and Bacteroidetes were abundant in adults guts with core taxa of Enterobacteriaceae and Flavobacteriaceae (Wang et al., 2011). Enterobacter cloacae and Serratia marcescens were two predominant species among the bacteria isolated from An. gambiae s.l. (Ezemuoka et al., 2020). Fourteen bacteria species from eight different genera (Staphylococcus, Burkholderia, Cedecea, Enterobacter, Klebsiella, Pantoea, Serratia, and Acinetobacter) were identified in the feces of wild An. darlingi (Arruda et al., 2021). The most frequent species were members of the Serratia genus with Serratia liquefaciens and Serratia marcescens the major representative bacterial species (Arruda et al., 2021). It was reported in the malaria vectors An. funestus and An. arabiensis, the presence of a number of bacteria even weeks after mosquitoes’ preservation on silica or in RNAlater® solution (E Silva et al., 2021). The authors found that the midgut of An. arabiensis was mainly colonized by Bacteroidetes and Proteobacteria, with the latter being the predominant one colonizing An. funestus midgut (E Silva et al., 2021). Elizabethkingia and Serratia were found in both An. arabiensis males and females, while Serratia, Elizabethkingia and Aeromonas were the dominant genera in males An. arabiensis (E Silva et al., 2021). The same authors discovered that at the species level, Elizabethkingia anophelis, Serratia oryzae and Aeromonas hydrophila were common bacteria between female and male An. arabiensis. In the preserved field-collected An. arabiensis, Staphylococcus was the dominant genus, with Staphylococcus epidermidis and Staphylococcus hominis present after 8 and 12 weeks of preservation (E Silva et al., 2021). In both An. funestus and An. arabiensis, Enterobacter cloacae was specific to females and S. epidermidis was specific to male mosquitoes (E Silva et al., 2021). It appears that the bacterial communities can be well preserved in Anopheles mosquitoes. However, it was reported that the bacterial composition and diversity in An. gambiae s.l. rely upon several factors such as season, geography and environmental variations (Akorli et al., 2016; Krajacich et al., 2018). In field-caught An. arabiensis from Zambia, seven bacterial species including Comamonas sp., Acinetobacter sp., Pseudomonas putida, Pantoea sp., Pseudomonas rhodesiae, Serratia marcescens and Elizabethkingia anophelis were isolated from their midgut (Cirimotich et al., 2011; Bahia et al., 2014).
In Anopheles mosquitoes from china, Bifidobacterium, Faecalibacterium, Escherichia-Shigella, and Blautia were significantly enriched in An. lesteri, wheras Pseudomonas and Ruminococcaceae were predominant in An. dirus and Asaia in An. sinensis (Feng et al., 2021). The genus Asaia, previously reported as the dominant bacterium in An. stephensi microbiota (Favia et al., 2007), was also found in all aquatic stages (eggs, L1–L4 larvae, pupae) as well as in the midgut, salivary glands and reproductive tissues of both laboratory-reared and filed-collected adult An. gambiae mosquitoes (Damiani et al., 2010).
Actinobacteria including Arthrobacter sp., Brevibacterium sp., Leucobacter sp., and Microbacterium sp., were found in adults, larvae, pupae and eggs of An. darlingi (Rocha et al., 2021). The common genera including Acinetobacter, Enterobacter, Klebsiella, Serratia, Bacillus, Elizabethkingia, Stenotrophomonas and Pantoea were predominantly identified in this mosquito species (Rocha et al., 2021). The natural endosymbiont bacterium Wolbachia was found in diverse field-caught Anopheles species including An. maculatus, An. sinensis and other Anopheles species collected in Malaysia (Wong et al., 2020). The presence of Wolbachia in field populations of An. gambiae has also been reported in Burkina-Faso (Baldini et al., 2014); in Mali (Gomes et al., 2017); in a large number of Anopheles species from Gabon (Ayala et al., 2019); and in An. funestus populations in Senegal (Niang et al., 2018).
Anopheles species harbor huge microbial communities. However, the existence of a main bacterial community is still not clear (Romoli and Gendrin, 2018). The Gram-negative aerobic or facultative aerobic bacteria, mostly belonging to the families Enterobacteriaceae (Serratia, Ewingella, Enterobacter and Klebsiella), Acetobacteraceae (Acetobacter and Asaia) and Flavobacteriaceae (Elizabethkingia and Chryseobacterium) represent the bacterial genera commonly found in Anopheles mosquitoes (Gendrin and Christophides, 2013). An individual mosquito host variable and dynamic microbiota (Table 2). Such diversity in the bacterial composition could depend on environmental factors and individual history. Indeed, the implication of the environment in shaping mosquitoes was reported in previous studies (Boissière et al., 2012; Osei-Poku et al., 2012). Furthermore, it was shown that larval breeding water and adult mosquito sugar food contribute to midgut microbiota composition in mosquitoes (Saab et al., 2020). However, some authors suggest that genetic factors might be more important than environmental factors in influencing the divergence of mosquito microbiota across the different species (Feng et al., 2021). Some bacteria genera were suggested to be transmitted from females to offspring by likely vertical symbiont transmission mechanism via eggs (Damiani et al., 2010). Indeed, effective horizontal and vertical transmission routes of bacteria were already described in An. stephensi (Favia et al., 2007; Damiani et al., 2008).
Although further studies are needed to investigate the role that bacteria play in Anopheles mosquitoes, previous works have attempted to provide more insights. Bacterial communities have been shown to impede Plasmodium development in An. gambiae (Beier et al., 1994). An association of distinct bacteria with the pyrethroid resistance in An. gambiae has been reported (Omoke et al., 2021). Bacteria of Anopheles vectors interfere with both the physiology and vector competence of their bearers. Several studies have revealed an overall inhibitory effect of the bacterial communities on Plasmodium parasites in Anopheles species. It was found that the intestinal bacterial communities can regulate the expression of the thioester-containing protein (TEP1) via an RNA interference (RNAi) mechanism to inhibit P. yoelii development in An. dirus (Wang et al., 2013). Other bacterial species, including Escherichia coli (strains H243, HS5); Pseudomonas aeruginosa; Pseudomonas sutzeria; Ewingella americana, Serratia marcescens; Xanthomonas malthophila; Cedecea lapageia; Enterobacter cloacae; Enterobacter amnigenus, S. aureus; Comamonas spp.; Bacillus pumilus; Chromobacterium sp. Csp_P, and Methylobacterium were reported to affect the development of P. falciparum, P. vivax, and P. berghei in An. gambiae (Dong et al., 2009; Meister et al., 2009; Cirimotich et al., 2011; Bahia et al., 2014; Ramirez et al., 2014; Tchioffo et al., 2016), An. stephensi (Pumpuni et al., 1993, 1996; Cirimotich et al., 2011; Bando et al., 2013), An. albimanus (Gonzalez-Ceron et al., 2003) and An. coluzzii (Meister et al., 2009; Tchioffo et al., 2013). Another bacterial species of the genus Serratia, S. ureilytica, isolated from the midguts of wild An. sinensis mosquitoes have been shown to inhibit the development of P. falciparum or the rodent parasite P. berghei by producing a lipase that is lethal to the parasites at different developmental stages (Gao et al., 2021). Contrary, in An. stephensi, the bacterium Asaia bogorensis, was demonstrated to increase the midgut pH and subsequently promote the Plasmodium berghei gametogenesis by alkalizing the mosquito midgut (Wang et al., 2021). The genome of the Asaia strain isolated from An. stephensi was analyzed and it was found that this bacterial strain had the most predicted regulatory proteins, suggesting its ability to adapt to frequent environmental changes in the mosquito gut (Chen et al., 2021).
Furthermore, Wolbachia infection was shown to significantly reduce the prevalence and intensity of sporozoite infection in An. gambiae s.l. (Gomes et al., 2017). Besides the negative correlation with Plasmodium development, Wolbachia infections in natural Anopheles populations also affect egg-laying (Shaw et al., 2016). Reintroduction of Pseudomonas putida, Pantoea sp., and Serratia marcescens into An. arabiensis through sugar feeding, resulted also in significant inhibition of P. falciparum infection, with S. marcescens and P. putida exhibiting the strongest parasite-blocking activity (Bahia et al., 2014). Experimental infection of Chromobacterium violaceum in insecticide-resistant An. coluzzii females was shown to significantly reduce mosquito survival, blood-feeding and to affect fecundity and hatching rate (Gnambani et al., 2020).
The biology of bacterial communities is an essential determinant for Anopheles mosquitoes’ fitness and resistance to insecticide molecules. During mosquito development, Anopheles mosquito larvae feed on microorganisms, organic matter and biofilm in aquatic habitats. Biofilm on the breeding site water surface is enriched with bacteria and provides nutrients to the larvae (Cansado-Utrilla et al., 2021). Some ingested bacteria in aquatic habitats colonize the mosquito’s gut and are transmitted to the adult stage (Wotton et al., 1997). The colonization of the host organism by the bacteria is mutually beneficial to both. Indeed, the host provides a stable, nutrient-rich environment, and the bacteria assist in digestion, protection from opportunistic pathogens, and immune system maturation (Kamada et al., 2013).
Modulating mosquito’s bacterial composition could shape the vector lifespan or the susceptibility to the existing classes of insecticides. Indeed, it was reported that bacteria depletion by using bactericidal antibiotics increased the longevity of susceptible An. arabiensis while bacterial supplementation increased insecticide tolerance in the resistant individuals (Barnard et al., 2019). On the contrary, other authors have shown that antibiotic mediated disorder of gut homeostasis leads to a decreased longevity in An. arabiensis (Debalke et al., 2019). Furthermore, dysbiosis in An. gambiae s.l. through antibiotics treatment resulted in reduced lifespan while the reintroduction of Enterobacter cloacae and Serratia marcescens in female mosquitoes did not affect the average fecundity but they positively affected hatching rates (Ezemuoka et al., 2020). However, microbiome dysbiosis using oxytetracycline has been shown to reduce the fecundity of An. gambiae bearing kdrR (L1014F) allele (Medjigbodo et al., 2021).
Overall, the molecular mechanisms involved in the interactions between bacteria and mosquito hosts, as well as between bacterial species of the mosquito microbiome remain not well understood. Further investigations are needed to investigate the mechanisms by which the vector longevity and fecundity are influenced by the gut bacterial community. Adult male mosquitoes obtain their nutrients from sugar, while both sugar and blood constitute the food sources for females. Consequently, the microbiome is involved in food digestion. Even though it was found in other mosquito species that Enterobacteriaceae is the main family of the gut microbiota at assimilating monosaccharides (Guégan et al., 2020), this is not yet characterized in Anopheles species. Therefore, the influence of the gut-associated microbiota on Anopheles vectors nutrition remains a field of interest not well explored and open for subsequent studies.
Bacteriophages were discovered in 1915 and are viruses infecting bacteria and archaebacteria (Clokie et al., 2011). They are found in all biomes (Herridge et al., 2020), including mosquito vectors. Although some of these viruses are latent (the infection does not immediately result in cell death), there are virulent bacteriophages that only replicate through a lytic cycle to release new virions and kill their bacterial host (Chevallereau et al., 2022). There are also obligate lytic bacteriophages that are lethal to a specific bacteria genus of a particular species (de Jonge et al., 2019). Besides, lytic bacteriophages were already isolated from bacterial species, which can degrade insecticides. Indeed, three bacteriophages were isolated from bacteria belonging to the Klebsiella genus, bacteria associated with organophosphate insecticide resistance in Anopheles albimanus (Dada et al., 2018). These isolated bacteriophages belong to the Podoviridae family and have demonstrated strong lytic potential in vitro against Klebsiella pneumoniae strain ZS15 (Kupritz et al., 2021). Moreover, it was demonstrated that phage G, initially considered a bacteriophage of Bacillus megaterium, is a Lysinibacillus bacteriophage (González et al., 2020). Lysinibacillus is a pyrethroid-degrading taxon and bacteria genus associated with permethrin resistance in western Kenyan An. gambiae (Omoke et al., 2021).
The presence of bacteriophages in insecticide degrading bacteria of malaria vectors suggests that bacteriophages infection could be involved in mosquito bacterial composition regulation as they attack and kill bacteria. As a result, the isolation and the characterization of mosquito bacteriophages communities may lead to the identification of virulent bacteriophages that could be used to design biological alternative vector control strategies. However, investigations on the diversity and the composition of Anopheles mosquito’s bacteriophages communities are still lacking.
Mosquitoes develop robust innate immune responses against invading pathogens such as viruses, bacteria (Levashina et al., 2001) and malaria parasites (Richman et al., 1997). The mosquito immune system is divided into two responses: humoral and cellular defense mechanisms.
In the humoral defense, the recognition of pathogens is done through the activation of effector molecules such as specific proteases that trigger processes such as melanization. Among these effectors, antimicrobial peptides of An. gambiae are known to be produced against different types of pathogens. These include defensin (active against Gram-positive bacteria), cecropin-1 (against Gram-positive and Gram-negative bacteria and fungi) and gambicin (against Gram-positive and Gram-negative bacteria) (Vizioli et al., 2000, 2001; Bartholomay and Michel, 2018).
The cellular immune responses are mediated by mosquito blood cells (hemocytes) and include phagocytosis of pathogens. Previous studies have shown that humoral and cellular immune responses involved three signaling pathways: the toll pathway (Luo and Zheng, 2000; Goto et al., 2003), the immunodeficiency pathway (IMD) (Meister et al., 2005) and janus kinase/signal transducer and activator of transcription (JAK/STAT) (Gupta et al., 2009). The An. gambiae JAK/STAT pathway contributes to anti-Plasmodium immune responses against the development of early Plasmodium spp. oocysts through the activation of nitric oxide synthase (NOS) (Gupta et al., 2009).
In general, the ability of mosquitoes to inhibit the growth of pathogens occurs through three mechanisms. Firstly, bacterial growth after a blood meal triggers an immune response via the immunodeficiency pathway (IMD), which causes the synthesis of antimicrobial peptides and other immune effectors (Meister et al., 2009). These effectors target bacterial populations in the mosquito midgut and exert antiparasitic effects (Meister et al., 2009). Secondly, symbiont bacteria of the Anopheles mosquito have been shown naturally to have the ability to inhibit Plasmodium spp. development. Specifically, the genus Enterobacter (Esp_Z) has been shown to inhibit P. falciparum ookinete, oocyst, and sporozoite formation in An. gambiae by up to 99% via elevated reactive oxygen species (ROS) synthesis (Cirimotich et al., 2011). Some mosquito gut bacteria, including Serratia marcescens and Acinetobacter spp. inhibit malaria parasite infection in mosquitoes (Wang et al., 2017). Thirdly, a microbiota-dependent immune priming system is reported during Plasmodium spp. infection. This effect protects mosquitoes from subsequent Plasmodium spp. infections and is likely mediated by hemocytes differentiation (Smith et al., 2015). Thus, insects’ gut microbiota appears as one of the important factors in host resistance to pathogen development. In particular, it has been shown that mosquito bacteria negatively impact oocysts density through colonization mechanisms involving either direct interactions between Plasmodium spp. and the microbiota or through induction of the mosquito’s immune response by the bacteria (Dong et al., 2009; Meister et al., 2009).
The presence of bacteria that naturally prevent Plasmodium infection in Anopheles mosquitoes provides a solid background for further genetic modification of predominant bacteria species found in these vectors to prevent parasite transmission. Indeed, it was already demonstrated that modified bacteria of the genus Asaia could express anti-malaria effectors, and the engineered strains obtained inhibit the development of malaria parasites (Shane et al., 2018).
Recently, several research reports showed associations between the mosquito microbiota and resistance to the current insecticides used for vector control. Recent work has identified 21 bacterial genera specific to the resistant An. gambiae harboring the kdr-East allele (L1014S, conferring resistance to permethrin) and 16 genera unique to the susceptible individuals (Omoke et al., 2021). Indeed, the well-known pyrethroid-degrading taxa bacteria of Sphingobacterium, Lysinibacillus and Streptococcus genera, in addition to the radiotolerant Rubrobacter were reported in resistant An. gambiae mosquitoes which were resistant at fivefold the diagnostic dose of permethrin (Omoke et al., 2021). Interestingly, the genus Myxococcus, was abundant in the susceptible An. gambiae and was not detected in their resistant counterparts (Omoke et al., 2021). Ochrobactrum, Lysinibacillus, and Stenotrophomonas genera (each of which comprised insecticide-degrading species) were significantly enriched in deltamethrin-resistant An. coluzzii, while susceptible mosquitoes had a significant reduction in bacterial diversity, with Asaia and Serratia as dominating microbial genera (Pelloquin et al., 2021). In the Lysinibacillus genus, Lysinibacillus sphaericus was demonstrated to be able to degrade up to 83% of cyfluthrin (a pyrethroid insecticide) by using the insecticide as a source of carbon or nitrogen (Hu et al., 2014).
An indirect effect of gut-associated microbiota on mosquito longevity by mediating insecticides resistance was also reported. Klebsiella, Enterobacter, Staphylococcus and Aeromonas were primarily found in the guts of the fourth instar larvae and non-blood fed adult females of the multiple-resistant An. arabiensis (Barnard et al., 2019). The organophosphate insecticides degrading bacterial species were found in fenitrothion resistant An. albimanus, including the predominant genera Klebsiella, Enterobacter, Acinetobacter, Escherichia, and Salmonella (Dada et al., 2018). Furthermore, pyrethroid insecticides exposure was found to induce changes in the cuticle, and the internal microbiota of both larvae and adults exposed An. albimanus (Dada et al., 2019). Indeed, Pseudomonas fragi and Pseudomonas agglomerans were more abundant in the internal microbiota of both alphacypermethrin- and permethrin-exposed An. albimanus adults, while unique taxa annotated as Acinetobacter and Asaia were more abundant in the internal and cuticle surface microbiota of non-exposed mosquitoes (Dada et al., 2019). In another vector species An. stephensi, four dominant genera, including Pseudomonas, Aeromonas, Exiguobacterium, and Microbacterium, were found in the midguts of temephos-resistant individuals (Soltani et al., 2017).
All these observations suggest that there is an additional microbe-mediated insecticide resistance mechanism in addition to the known target site modification mechanisms in these malaria vectors. The insecticide degrading properties could be due to the high expression of xenobiotic degrading genes and enzymes in bacteria when they are in contact with insecticidal molecules. Apart from larvicides that inter larvae through breeding water, insecticide molecules enter the adult mosquito body through the respiratory system (by inhalation) or the cuticle. Once the insecticides go through the cuticle, they enter the hemolymph where they could migrate to any organ of the insect. Thus, once in the gut, for example, they will be in contact with gut bacteria degrading insecticide. Then, the insecticide metabolism will lead to a decrease in the quantity of insecticidal molecules available to reach their target site. As a result, mosquitoes will be insensitive to that insecticide. However, further investigations are needed to understand the causality and pathways underlying such interactions. Yet, since microbes are likely to produce effector molecules that degrade insecticides, it is thought that the microbe-mediated mechanism of insecticide resistance would be likely of a metabolic nature (Dada et al., 2018; Omoke et al., 2021). This hypothesis is supported by other research findings. Indeed, high relative abundances of hydrolases, isomerases, and lyases were reported in xenobiotic-degrading bacteria colonizing fenitrothion resistant An. albimanus with two significantly enriched carboxylesterases (carboxymethylenebutenolidase and gluconolactonase), and two significantly enriched phosphomonoesterases (alkaline phosphatase, and acid phosphatase) (Dada et al., 2018).
A more thorough comprehension of the role of the microbiome in insecticide resistance will empower the improvement of techniques toward curbing the widespread resistance and sustaining the effectiveness of the current vector control interventions (Cansado-Utrilla et al., 2021). Several studies have reported significant differences in microbiota composition between susceptible and resistant mosquitoes, with the highest bacterial diversity found in susceptible mosquitoes (Dada et al., 2018; Barnard et al., 2019; Omoke et al., 2021). Thus, modulating mosquito bacterial diversity could lead to a change in insecticide resistance phenotype that would be beneficial for resistant vector populations’ management. In the actual context of the search for eco-friendly alternative vector control strategies, the success of malaria vector control requires a combined action of all existing control strategies. As a result, strategies targeting the microbiota can be considered to prevent insecticide degradation by mosquitoes’ bacteria.
Mosquitoes have exceptionally diverse microbial taxa, which implies the significant effect of the microenvironment in modeling microbial organization. Several studies were carried out to characterize the wild mosquito microbiota. However, further research works are needed to better understand the full microbiome spectrum and its potential implications for malaria-transmitting vectors. More information on these vectors’ microbiota is fundamental for implementing paratransgenic strategies and symbiotic control approaches. In the current context of widespread insensitivity of malaria mosquitoes to the existing chemical insecticides, the role of bacteria in the mosquito immune system and resistance to insecticides open perspectives on the research of alternative biological control strategies based on endosymbiosis.
OD, AG, DN, and LSD contributed to the conception and design of the study. OD organized the sessions and wrote the first draft of the manuscript. OD, AM, AG, HS, HL, DN, LD, RB, PS, M-JF, RAg, RAk, and WM wrote sections of the manuscript. All authors contributed to manuscript revision, read, and approved the submitted version.
The authors declare that the research was conducted in the absence of any commercial or financial relationships that could be construed as a potential conflict of interest.
All claims expressed in this article are solely those of the authors and do not necessarily represent those of their affiliated organizations, or those of the publisher, the editors and the reviewers. Any product that may be evaluated in this article, or claim that may be made by its manufacturer, is not guaranteed or endorsed by the publisher.
Akorli, J., Gendrin, M., Pels, N. A. P., Yeboah-Manu, D., Christophides, G. K., and Wilson, M. D. (2016). Seasonality and locality affect the diversity of Anopheles gambiae and Anopheles coluzzii midgut microbiota from Ghana. PLoS One 11:e0157529. doi: 10.1371/journal.pone.0157529
Angleró-Rodríguez, Y. I., Blumberg, B. J., Dong, Y., Sandiford, S. I., Pike, A., Clayton, A. M., et al. (2016). A natural Anopheles-associated Penicillium chrysogenum enhances mosquito susceptibility to Plasmodium infection. Sci. Rep. 6:34084. doi: 10.1038/srep34084
Arruda, A., Ferreira, G. E. M., Santos Júnior, A., Matos, N. B., Carvalho, T. S., Ozaki, L. S., et al. (2021). Diversity of culturable bacteria isolated from the feces of wild Anopheles darlingi (Diptera: Culicidae) mosquitoes from the Brazilian Amazon. J. Med. Entomol. 58, 1900–1907. doi: 10.1093/jme/tjab028
Ayala, D., Akone-Ella, O., Rahola, N., Kengne, P., Ngangue, M. F., Mezeme, F., et al. (2019). Natural Wolbachia infections are common in the major malaria vectors in central Africa. Evol. Appl. 12, 1583–1594. doi: 10.1111/eva.12804
Bahia, A. C., Dong, Y., Blumberg, B. J., Mlambo, G., Tripathi, A., BenMarzouk-Hidalgo, O. J., et al. (2014). Exploring Anopheles gut bacteria for Plasmodium blocking activity. Environ. Microbiol. 16, 2980–2994. doi: 10.1111/1462-2920.12381
Baldini, F., Segata, N., Pompon, J., Marcenac, P., Shaw, W. R., Dabiré, R. K., et al. (2014). Evidence of natural Wolbachia infections in field populations of Anopheles gambiae. Nat. Commun. 5:3985. doi: 10.1038/ncomms4985
Bando, H., Okado, K., Guelbeogo, W. M., Badolo, A., Aonuma, H., Nelson, B., et al. (2013). Intra-specific diversity of Serratia marcescens in Anopheles mosquito midgut defines Plasmodium transmission capacity. Sci. Rep. 3:1641. doi: 10.1038/srep01641
Barnard, K., Jeanrenaud, A. C. S. N., Brooke, B. D., and Oliver, S. V. (2019). The contribution of gut bacteria to insecticide resistance and the life histories of the major malaria vector Anopheles arabiensis (Diptera: Culicidae). Sci. Rep. 9:9117. doi: 10.1038/s41598-019-45499-z
Barrett, A. D. T., and Weaver, S. C. (2012). “51 - Arboviruses: alphaviruses, flaviviruses and bunyaviruses: encephalitis; yellow fever; dengue; haemorrhagic fever; miscellaneous tropical fevers; undifferentiated fever,” in Medical Microbiology, 18th Edn, eds D. Greenwood, M. Barer, R. Slack, and W. Irving (Edinburgh: Churchill Livingstone), 520–536. doi: 10.1016/B978-0-7020-4089-4.00066-4
Bartholomay, L. C., and Michel, K. (2018). Mosquito immunobiology: the intersection of vector health and vector competence. Annu. Rev. Entomol. 63, 145–167. doi: 10.1146/annurev-ento-010715-023530
Battle, K. E., Gething, P. W., Elyazar, I. R. F., Moyes, C. L., Sinka, M. E., Howes, R. E., et al. (2012). The global public health significance of Plasmodium vivax. Adv. Parasitol. 80, 1–111. doi: 10.1016/B978-0-12-397900-1.00001-3
Beier, M. S., Pumpuni, C. B., Beier, J. C., and Davis, J. R. (1994). Effects of para-aminobenzoic acid, insulin, and gentamicin on Plasmodium falciparum development in Anopheline mosquitoes (Diptera: Culicidae). J. Med. Entomol. 31, 561–565. doi: 10.1093/jmedent/31.4.561
Belda, E., Nanfack-Minkeu, F., Eiglmeier, K., Carissimo, G., Holm, I., Diallo, M., et al. (2019). De novo profiling of RNA viruses in Anopheles malaria vector mosquitoes from forest ecological zones in Senegal and Cambodia. BMC Genomics 20:664. doi: 10.1186/s12864-019-6034-1
Bhatt, S., Weiss, D. J., Cameron, E., Bisanzio, D., Mappin, B., Dalrymple, U., et al. (2015). The effect of malaria control on Plasmodium falciparum in Africa between 2000 and 2015. Nature 526, 207–211. doi: 10.1038/nature15535
Blanford, S., Shi, W., Christian, R., Marden, J. H., Koekemoer, L. L., Brooke, B. D., et al. (2011). Lethal and pre-lethal effects of a fungal biopesticide contribute to substantial and rapid control of malaria vectors. PLoS One 6:e23591. doi: 10.1371/journal.pone.0023591
Boissière, A., Tchioffo, M. T., Bachar, D., Abate, L., Marie, A., Nsango, S. E., et al. (2012). Midgut microbiota of the malaria mosquito vector Anopheles gambiae and interactions with Plasmodium falciparum infection. PLoS Pathog. 8:e1002742. doi: 10.1371/journal.ppat.1002742
Bozic, J., Capone, A., Pediconi, D., Mensah, P., Cappelli, A., Valzano, M., et al. (2017). Mosquitoes can harbour yeasts of clinical significance and contribute to their environmental dissemination. Environ. Microbiol. Rep. 9, 642–648. doi: 10.1111/1758-2229.12569
Bukhari, T., Middelman, A., Koenraadt, C. J. M., Takken, W., and Knols, B. G. J. (2010). Factors affecting fungus-induced larval mortality in Anopheles gambiae and Anopheles stephensi. Malar. J. 9:22. doi: 10.1186/1475-2875-9-22
Cansado-Utrilla, C., Zhao, S. Y., McCall, P. J., Coon, K. L., and Hughes, G. L. (2021). The microbiome and mosquito vectorial capacity: rich potential for discovery and translation. Microbiome 9:111. doi: 10.1186/s40168-021-01073-2
Castro, M. C. (2017). Malaria transmission and prospects for malaria eradication: the role of the environment. Cold Spring Harb. Perspect. Med. 7:a025601. doi: 10.1101/cshperspect.a025601
Chen, S., Yu, T., Terrapon, N., Henrissat, B., and Walker, E. D. (2021). Genome features of Asaia sp. W12 isolated from the mosquito Anopheles stephensi reveal symbiotic traits. Genes 12:752. doi: 10.3390/genes12050752
Chevallereau, A., Pons, B. J., van Houte, S., and Westra, E. R. (2022). Interactions between bacterial and phage communities in natural environments. Nat. Rev. Microbiol. 20, 49–62. doi: 10.1038/s41579-021-00602-y
Cirimotich, C. M., Dong, Y., Clayton, A. M., Sandiford, S. L., Souza-Neto, J. A., Mulenga, M., et al. (2011). Natural microbe-mediated refractoriness to Plasmodium infection in Anopheles gambiae. Science 332, 855–858. doi: 10.1126/science.1201618
Clokie, M. R., Millard, A. D., Letarov, A. V., and Heaphy, S. (2011). Phages in nature. Bacteriophage 1, 31–45. doi: 10.4161/bact.1.1.14942
Coetzee, M., Hunt, R. H., Wilkerson, R., Della Torre, A., Coulibaly, M. B., and Besansky, N. J. (2013). Anopheles coluzzii and Anopheles amharicus, new members of the Anopheles gambiae complex. Zootaxa 3619, 246–274. doi: 10.11646/zootaxa.3619.3.2
Coutinho-Abreu, I. V., Zhu, K. Y., and Ramalho-Ortigao, M. (2010). Transgenesis and paratransgenesis to control insect-borne diseases: current status and future challenges. Parasitol. Int. 59, 1–8. doi: 10.1016/j.parint.2009.10.002
da Silva Neves, N. A., Pinto, A. Z. L., Melo, F. L., Maia, L. M. S., da Silva Ferreira, R., de Carvalho, M. S., et al. (2021). Sialovirome of Brazilian tropical anophelines. Virus Res. 302:198494. doi: 10.1016/j.virusres.2021.198494
Dada, N., Lol, J. C., Benedict, A. C., López, F., Sheth, M., Dzuris, N., et al. (2019). Pyrethroid exposure alters internal and cuticle surface bacterial communities in Anopheles albimanus. ISME J. 13, 2447–2464. doi: 10.1038/s41396-019-0445-5
Dada, N., Sheth, M., Liebman, K., Pinto, J., and Lenhart, A. (2018). Whole metagenome sequencing reveals links between mosquito microbiota and insecticide resistance in malaria vectors. Sci. Rep. 8:2084. doi: 10.1038/s41598-018-20367-4
Damiani, C., Ricci, I., Crotti, E., Rossi, P., Rizzi, A., Scuppa, P., et al. (2008). Paternal transmission of symbiotic bacteria in malaria vectors. Curr. Biol. 18, R1087–R1088. doi: 10.1016/j.cub.2008.10.040
Damiani, C., Ricci, I., Crotti, E., Rossi, P., Rizzi, A., Scuppa, P., et al. (2010). Mosquito-bacteria symbiosis: the case of Anopheles gambiae and Asaia. Microb. Ecol. 60, 644–654. doi: 10.1007/s00248-010-9704-8
de Jonge, P. A., Nobrega, F. L., Brouns, S. J. J., and Dutilh, B. E. (2019). Molecular and evolutionary determinants of bacteriophage host range. Trends Microbiol. 27, 51–63. doi: 10.1016/j.tim.2018.08.006
Debalke, S., Habtewold, T., Duchateau, L., and Christophides, G. K. (2019). The effect of silencing immunity related genes on longevity in a naturally occurring Anopheles arabiensis mosquito population from southwest Ethiopia. Parasit. Vectors 12:174. doi: 10.1186/s13071-019-3414-y
Dong, Y., Manfredini, F., and Dimopoulos, G. (2009). Implication of the mosquito midgut microbiota in the defense against malaria parasites. PLoS Pathog. 5:e1000423. doi: 10.1371/journal.ppat.1000423
E Silva, B., Matsena Zingoni, Z., Koekemoer, L. L., and Dahan-Moss, Y. L. (2021). Microbiota identified from preserved Anopheles. Malar. J. 20:230. doi: 10.1186/s12936-021-03754-7
Ezemuoka, L. C., Akorli, E. A., Aboagye-Antwi, F., and Akorli, J. (2020). Mosquito midgut Enterobacter cloacae and Serratia marcescens affect the fitness of adult female Anopheles gambiae s.l. PLoS One 15:e0238931. doi: 10.1371/journal.pone.0238931
Farenhorst, M., Farina, D., Scholte, E.-J., Takken, W., Hunt, R. H., Coetzee, M., et al. (2008). African water storage pots for the delivery of the entomopathogenic fungus Metarhizium anisopliae to the malaria vectors Anopheles gambiae s.s. and Anopheles funestus. Am. J. Trop. Med. Hyg. 78, 910–916. doi: 10.4269/ajtmh.2008.78.910
Fauver, J. R., Grubaugh, N. D., Krajacich, B. J., Weger-Lucarelli, J., Lakin, S. M., Fakoli, L. S., et al. (2016). West African Anopheles gambiae mosquitoes harbor a taxonomically diverse virome including new insect-specific flaviviruses, mononegaviruses, and totiviruses. Virology 498, 288–299. doi: 10.1016/j.virol.2016.07.031
Favia, G., Ricci, I., Damiani, C., Raddadi, N., Crotti, E., Marzorati, M., et al. (2007). Bacteria of the genus Asaia stably associate with Anopheles stephensi, an Asian malarial mosquito vector. Proc. Natl. Acad. Sci. U.S.A. 104, 9047–9051. doi: 10.1073/pnas.0610451104
Feng, Y., Tang, J., Zhu, D., Zhang, Y., Zhu, G., and Wang, J. (2021). The microbiota of three Anopheles species in China. J. Am. Mosq. Control Assoc. 37, 38–40. doi: 10.2987/20-6940
Gabrieli, P., Caccia, S., Varotto-Boccazzi, I., Arnoldi, I., Barbieri, G., Comandatore, F., et al. (2021). Mosquito trilogy: microbiota, immunity and pathogens, and their implications for the control of disease transmission. Front. Microbiol. 12:630438. doi: 10.3389/fmicb.2021.630438
Gao, H., Bai, L., Jiang, Y., Huang, W., Wang, L., Li, S., et al. (2021). A natural symbiotic bacterium drives mosquito refractoriness to Plasmodium infection via secretion of an antimalarial lipase. Nat. Microbiol. 6, 806–817. doi: 10.1038/s41564-021-00899-8
Gendrin, M., and Christophides, G. K. (2013). “The Anopheles mosquito microbiota and their impact on pathogen transmission,” in Anopheles mosquitoes - New Insights into Malaria Vectors, ed. S. Manguin (London: InTech). doi: 10.5772/55107
George, J., Blanford, S., Domingue, M. J., Thomas, M. B., Read, A. F., and Baker, T. C. (2011). Reduction in host-finding behaviour in fungus-infected mosquitoes is correlated with reduction in olfactory receptor neuron responsiveness. Malar. J. 10:219. doi: 10.1186/1475-2875-10-219
Gnambani, E. J., Bilgo, E., Sanou, A., Dabiré, R. K., and Diabaté, A. (2020). Infection of highly insecticide-resistant malaria vector Anopheles coluzzii with entomopathogenic bacteria Chromobacterium violaceum reduces its survival, blood feeding propensity and fecundity. Malar. J. 19:352. doi: 10.1186/s12936-020-03420-4
Gomes, F. M., Hixson, B. L., Tyner, M. D. W., Ramirez, J. L., Canepa, G. E., Alves e Silva, T. L., et al. (2017). Effect of naturally occurring Wolbachia in Anopheles gambiae s.l. mosquitoes from Mali on Plasmodium falciparum malaria transmission. Proc. Natl. Acad. Sci. U.S.A. 114, 12566–12571. doi: 10.1073/pnas.1716181114
González, B., Monroe, L., Li, K., Yan, R., Wright, E., Walter, T., et al. (2020). Phage G structure at 6.1 Å resolution, condensed DNA, and host identity revision to a Lysinibacillus. J. Mol. Biol. 432, 4139–4153. doi: 10.1016/j.jmb.2020.05.016
Gonzalez-Ceron, L., Santillan, F., Rodriguez, M. H., Mendez, D., and Hernandez-Avila, J. E. (2003). Bacteria in midguts of field-collected Anopheles albimanus block Plasmodium vivax sporogonic development. J. Med. Entomol. 40, 371–374. doi: 10.1603/0022-2585-40.3.371
Goto, A., Blandin, S., Royet, J., Reichhart, J.-M., and Levashina, E. A. (2003). Silencing of toll pathway components by direct injection of double-stranded RNA into Drosophila adult flies. Nucleic Acids Res. 31, 6619–6623. doi: 10.1093/nar/gkg852
Guégan, M., Tran Van, V., Martin, E., Minard, G., Tran, F.-H., Fel, B., et al. (2020). Who is eating fructose within the Aedes albopictus gut microbiota? Environ. Microbiol. 22, 1193–1206. doi: 10.1111/1462-2920.14915
Gupta, L., Molina-Cruz, A., Kumar, S., Rodrigues, J., Dixit, R., Zamora, R. E., et al. (2009). The STAT pathway mediates late-phase immunity against Plasmodium in the mosquito Anopheles gambiae. Cell Host Microbe 5, 498–507. doi: 10.1016/j.chom.2009.04.003
Hameed, M., Liu, K., Anwar, M. N., Wahaab, A., Li, C., Di, D., et al. (2020). A viral metagenomic analysis reveals rich viral abundance and diversity in mosquitoes from pig farms. Transbound. Emerg. Dis. 67, 328–343. doi: 10.1111/tbed.13355
Hay, S. I., Sinka, M. E., Okara, R. M., Kabaria, C. W., Mbithi, P. M., Tago, C. C., et al. (2010). Developing global maps of the dominant Anopheles vectors of human malaria. PLoS Med. 7:e1000209. doi: 10.1371/journal.pmed.1000209
He, X., Yin, Q., Zhou, L., Meng, L., Hu, W., Li, F., et al. (2021). Metagenomic sequencing reveals viral abundance and diversity in mosquitoes from the Shaanxi-Gansu-Ningxia region, China. PLoS Negl. Trop. Dis. 15:e0009381. doi: 10.1371/journal.pntd.0009381
Herridge, W. P., Shibu, P., O’Shea, J., Brook, T. C., and Hoyles, L. (2020). Bacteriophages of Klebsiella spp., their diversity and potential therapeutic uses. J. Med. Microbiol. 69, 176–194. doi: 10.1099/jmm.0.001141
Howard, A. F. V., N’Guessan, R., Koenraadt, C. J. M., Asidi, A., Farenhorst, M., Akogbéto, M., et al. (2011). First report of the infection of insecticide-resistant malaria vector mosquitoes with an entomopathogenic fungus under field conditions. Malar. J. 10:24. doi: 10.1186/1475-2875-10-24
Hu, G. P., Zhao, Y., Song, F. Q., Liu, B., Vasseur, L., Douglas, C., et al. (2014). Isolation, identification and cyfluthrin-degrading potential of a novel Lysinibacillus sphaericus strain, FLQ-11-1. Res. Microbiol. 165, 110–118. doi: 10.1016/j.resmic.2013.11.003
Huang, W., Wang, S., and Jacobs-Lorena, M. (2020). Use of microbiota to fight mosquito-borne disease. Front. Genet. 11:196. doi: 10.3389/fgene.2020.00196
Huhtamo, E., Lambert, A. J., Costantino, S., Servino, L., Krizmancic, L., Boldorini, R., et al. (2013). Isolation and full genomic characterization of Batai virus from mosquitoes, Italy 2009. J. Gen. Virol. 94, 1242–1248. doi: 10.1099/vir.0.051359-0
Hussein, M. I. H., Albashir, A. A. D., Elawad, O. A. M. A., and Homeida, A. (2020). Malaria and COVID-19: unmasking their ties. Malar. J. 19:457. doi: 10.1186/s12936-020-03541-w
Jöst, H., Bialonski, A., Schmetz, C., Günther, S., Becker, N., and Schmidt-Chanasit, J. (2011). Isolation and phylogenetic analysis of Batai Virus, Germany. Am. J. Trop. Med. Hyg. 84, 241–243. doi: 10.4269/ajtmh.2011.10-0483
Kamada, N., Seo, S.-U., Chen, G. Y., and Núñez, G. (2013). Role of the gut microbiota in immunity and inflammatory disease. Nat. Rev. Immunol. 13, 321–335. doi: 10.1038/nri3430
Karunamoorthi, K. (2011). Vector control: a cornerstone in the malaria elimination campaign. Clin. Microbiol. Infect. 17, 1608–1616. doi: 10.1111/j.1469-0691.2011.03664.x
Kikankie, C. K., Brooke, B. D., Knols, B. G., Koekemoer, L. L., Farenhorst, M., Hunt, R. H., et al. (2010). The infectivity of the entomopathogenic fungus Beauveria bassiana to insecticide-resistant and susceptible Anopheles arabiensis mosquitoes at two different temperatures. Malar. J. 9:71. doi: 10.1186/1475-2875-9-71
Krajacich, B. J., Huestis, D. L., Dao, A., Yaro, A. S., Diallo, M., Krishna, A., et al. (2018). Investigation of the seasonal microbiome of Anopheles coluzzii mosquitoes in Mali. PLoS One 13:e0194899. doi: 10.1371/journal.pone.0194899
Kupritz, J., Martin, J., Fischer, K., Curtis, K. C., Fauver, J. R., Huang, Y., et al. (2021). Isolation and characterization of a novel bacteriophage WO from Allonemobius socius crickets in Missouri. PLoS One 16:e0250051. doi: 10.1371/journal.pone.0250051
Levashina, E. A., Moita, L. F., Blandin, S., Vriend, G., Lagueux, M., and Kafatos, F. C. (2001). Conserved role of a complement-like protein in phagocytosis revealed by dsRNA knockout in cultured cells of the mosquito, Anopheles gambiae. Cell 104, 709–718. doi: 10.1016/s0092-8674(01)00267-7
Luo, C., and Zheng, L. (2000). Independent evolution of toll and related genes in insects and mammals. Immunogenetics 51, 92–98. doi: 10.1007/s002510050017
Mannino, M. C., Huarte-Bonnet, C., Davyt-Colo, B., and Pedrini, N. (2019). Is the insect cuticle the only entry gate for fungal infection? Insights into alternative modes of action of entomopathogenic fungi. J. Fungi 5:33. doi: 10.3390/jof5020033
Medjigbodo, A. A., Sonounameto, E. G., Djihinto, O. Y., Abbey, E., Salavi, E. B., Djossou, L., et al. (2021). Interplay between oxytetracycline and the homozygote kdr (L1014F) resistance genotype on fecundity in Anopheles gambiae (Diptera: Culicidae) mosquitoes. J. Insect Sci. 21:13. doi: 10.1093/jisesa/ieab056
Meister, S., Agianian, B., Turlure, F., Relógio, A., Morlais, I., Kafatos, F. C., et al. (2009). Anopheles gambiae PGRPLC-mediated defense against bacteria modulates infections with malaria parasites. PLoS Pathog. 5:e1000542. doi: 10.1371/journal.ppat.1000542
Meister, S., Kanzok, S. M., Zheng, X.-L., Luna, C., Li, T.-R., Hoa, N. T., et al. (2005). Immune signaling pathways regulating bacterial and malaria parasite infection of the mosquito Anopheles gambiae. Proc. Natl. Acad. Sci. U.S.A. 102, 11420–11425. doi: 10.1073/pnas.0504950102
Mekuriaw, W., Balkew, M., Messenger, L. A., Yewhalaw, D., Woyessa, A., and Massebo, F. (2019). The effect of ivermectin® on fertility, fecundity and mortality of Anopheles arabiensis fed on treated men in Ethiopia. Malar. J. 18:357. doi: 10.1186/s12936-019-2988-3
Minard, G., Mavingui, P., and Moro, C. V. (2013). Diversity and function of bacterial microbiota in the mosquito holobiont. Parasit. Vectors 6:146. doi: 10.1186/1756-3305-6-146
Mnyone, L. L., Kirby, M. J., Lwetoijera, D. W., Mpingwa, M. W., Knols, B. G. J., Takken, W., et al. (2009). Infection of the malaria mosquito, Anopheles gambiae, with two species of entomopathogenic fungi: effects of concentration, co-formulation, exposure time and persistence. Malar. J. 8:309. doi: 10.1186/1475-2875-8-309
Mnyone, L. L., Kirby, M. J., Mpingwa, M. W., Lwetoijera, D. W., Knols, B. G. J., Takken, W., et al. (2011). Infection of Anopheles gambiae mosquitoes with entomopathogenic fungi: effect of host age and blood-feeding status. Parasitol. Res. 108, 317–322. doi: 10.1007/s00436-010-2064-y
Mnyone, L. L., Lyimo, I. N., Lwetoijera, D. W., Mpingwa, M. W., Nchimbi, N., Hancock, P. A., et al. (2012). Exploiting the behaviour of wild malaria vectors to achieve high infection with fungal biocontrol agents. Malar. J. 11:87. doi: 10.1186/1475-2875-11-87
Müller, O. (2011). Malaria in Africa: Challenges for Control and Elimination in the 21 st Century. Frankfurt: Peter Lang Publisher.
Nanfack Minkeu, F., and Vernick, K. D. (2018). A systematic review of the natural virome of Anopheles mosquitoes. Viruses 10:E222. doi: 10.3390/v10050222
Nattoh, G., Bargul, J. L., Magoma, G., Mbaisi, L., Butungi, H., Mararo, E., et al. (2021). The fungus Leptosphaerulina persists in Anopheles gambiae and induces melanization. PLoS One 16:e0246452. doi: 10.1371/journal.pone.0246452
Niang, E. H. A., Bassene, H., Makoundou, P., Fenollar, F., Weill, M., and Mediannikov, O. (2018). First report of natural Wolbachia infection in wild Anopheles funestus population in Senegal. Malar. J. 17:408. doi: 10.1186/s12936-018-2559-z
Omoke, D., Kipsum, M., Otieno, S., Esalimba, E., Sheth, M., Lenhart, A., et al. (2021). Western Kenyan Anopheles gambiae showing intense permethrin resistance harbour distinct microbiota. Malar. J. 20:77. doi: 10.1186/s12936-021-03606-4
Ondiaka, S. N., Masinde, E. W., Koenraadt, C. J., Takken, W., and Mukabana, W. R. (2015). Effects of fungal infection on feeding and survival of Anopheles gambiae (Diptera: Culicidae) on plant sugars. Parasit. Vectors 8:35. doi: 10.1186/s13071-015-0654-3
Osei-Poku, J., Mbogo, C. M., Palmer, W. J., and Jiggins, F. M. (2012). Deep sequencing reveals extensive variation in the gut microbiota of wild mosquitoes from Kenya. Mol. Ecol. 21, 5138–5150. doi: 10.1111/j.1365-294X.2012.05759.x
Pelloquin, B., Kristan, M., Edi, C., Meiwald, A., Clark, E., Jeffries, C. L., et al. (2021). Overabundance of Asaia and Serratia bacteria is associated with deltamethrin insecticide susceptibility in Anopheles coluzzii from Agboville, Côte d’Ivoire. Microbiol. Spectr. 9:e00157-21. doi: 10.1128/Spectrum.00157-21
Pumpuni, C. B., Beier, M. S., Nataro, J. P., Guers, L. D., and Davis, J. R. (1993). Plasmodium falciparum: inhibition of sporogonic development in Anopheles stephensi by gram-negative bacteria. Exp. Parasitol. 77, 195–199. doi: 10.1006/expr.1993.1076
Pumpuni, C. B., Demaio, J., Kent, M., Davis, J. R., and Beier, J. C. (1996). Bacterial population dynamics in three anopheline species: the impact on Plasmodium sporogonic development. Am. J. Trop. Med. Hyg. 54, 214–218. doi: 10.4269/ajtmh.1996.54.214
Ramirez, J. L., Short, S. M., Bahia, A. C., Saraiva, R. G., Dong, Y., Kang, S., et al. (2014). Chromobacterium Csp_P reduces malaria and dengue infection in vector mosquitoes and has entomopathogenic and in vitro anti-pathogen activities. PLoS Pathog. 10:e1004398. doi: 10.1371/journal.ppat.1004398
Rani, A., Sharma, A., Rajagopal, R., Adak, T., and Bhatnagar, R. K. (2009). Bacterial diversity analysis of larvae and adult midgut microflora using culture-dependent and culture-independent methods in lab-reared and field-collected Anopheles stephensi-an Asian malarial vector. BMC Microbiol. 9:96. doi: 10.1186/1471-2180-9-96
Ren, X., Hoiczyk, E., and Rasgon, J. L. (2008). Viral paratransgenesis in the malaria vector Anopheles gambiae. PLoS Pathog. 4:e1000135. doi: 10.1371/journal.ppat.1000135
Rhodes, V. L., Thomas, M. B., and Michel, K. (2018). The interplay between dose and immune system activation determines fungal infection outcome in the African malaria mosquito, Anopheles gambiae. Dev. Comp. Immunol. 85, 125–133. doi: 10.1016/j.dci.2018.04.008
Ricci, I., Damiani, C., Scuppa, P., Mosca, M., Crotti, E., Rossi, P., et al. (2011). The yeast Wickerhamomyces anomalus (Pichia anomala) inhabits the midgut and reproductive system of the Asian malaria vector Anopheles stephensi. Environ. Microbiol. 13, 911–921. doi: 10.1111/j.1462-2920.2010.02395.x
Ricci, I., Valzano, M., Ulissi, U., Epis, S., Cappelli, A., and Favia, G. (2012). Symbiotic control of mosquito borne disease. Pathog. Glob. Health 106, 380–385. doi: 10.1179/2047773212Y.0000000051
Richman, A. M., Dimopoulos, G., Seeley, D., and Kafatos, F. C. (1997). Plasmodium activates the innate immune response of Anopheles gambiae mosquitoes. EMBO J. 16, 6114–6119. doi: 10.1093/emboj/16.20.6114
Rocha, E. M., Marinotti, O., Serrão, D. M., Correa, L. V., Katak, R., de, M., et al. (2021). Culturable bacteria associated with Anopheles darlingi and their paratransgenesis potential. Malar. J. 20:40. doi: 10.1186/s12936-020-03574-1
Romoli, O., and Gendrin, M. (2018). The tripartite interactions between the mosquito, its microbiota and Plasmodium. Parasit. Vectors 11:200. doi: 10.1186/s13071-018-2784-x
Saab, S. A., Dohna, H. Z., Nilsson, L. K. J., Onorati, P., Nakhleh, J., Terenius, O., et al. (2020). The environment and species affect gut bacteria composition in laboratory co-cultured Anopheles gambiae and Aedes albopictus mosquitoes. Sci. Rep. 10:3352. doi: 10.1038/s41598-020-60075-6
Sadeghi, M., Popov, V., Guzman, H., Phan, T. G., Vasilakis, N., Tesh, R., et al. (2017). Genomes of viral isolates derived from different mosquitos species. Virus Res. 242, 49–57. doi: 10.1016/j.virusres.2017.08.012
Salyer, S. J., Maeda, J., Sembuche, S., Kebede, Y., Tshangela, A., Moussif, M., et al. (2021). The first and second waves of the COVID-19 pandemic in Africa: a cross-sectional study. Lancet 397, 1265–1275. doi: 10.1016/S0140-6736(21)00632-2
Scarpassa, V. M., Debat, H. J., Alencar, R. B., Saraiva, J. F., Calvo, E., Arcà, B., et al. (2019). An insight into the sialotranscriptome and virome of Amazonian anophelines. BMC Genomics 20:166. doi: 10.1186/s12864-019-5545-0
Scholte, E.-J., Knols, B. G. J., and Takken, W. (2004). Autodissemination of the entomopathogenic fungus Metarhizium anisopliae amongst adults of the malaria vector Anopheles gambiae s.s. Malar. J. 3:45. doi: 10.1186/1475-2875-3-45
Scholte, E.-J., Knols, B. G. J., and Takken, W. (2006). Infection of the malaria mosquito Anopheles gambiae with the entomopathogenic fungus Metarhizium anisopliae reduces blood feeding and fecundity. J. Invertebr. Pathol. 91, 43–49. doi: 10.1016/j.jip.2005.10.006
Scholte, E.-J., Ng’habi, K., Kihonda, J., Takken, W., Paaijmans, K., Abdulla, S., et al. (2005). An entomopathogenic fungus for control of adult African malaria mosquitoes. Science 308, 1641–1642. doi: 10.1126/science.1108639
Scholte, E.-J., Njiru, B. N., Smallegange, R. C., Takken, W., and Knols, B. G. J. (2003). Infection of malaria (Anopheles gambiae s.s.) and filariasis (Culex quinquefasciatus) vectors with the entomopathogenic fungus Metarhizium anisopliae. Malar. J. 2:29. doi: 10.1186/1475-2875-2-29
Shane, J. L., Grogan, C. L., Cwalina, C., and Lampe, D. J. (2018). Blood meal-induced inhibition of vector-borne disease by transgenic microbiota. Nat. Commun. 9:4127. doi: 10.1038/s41467-018-06580-9
Shaw, W. R., Marcenac, P., Childs, L. M., Buckee, C. O., Baldini, F., Sawadogo, S. P., et al. (2016). Wolbachia infections in natural Anopheles populations affect egg laying and negatively correlate with Plasmodium development. Nat. Commun. 7:11772. doi: 10.1038/ncomms11772
Shi, C., Liu, Y., Hu, X., Xiong, J., Zhang, B., and Yuan, Z. (2015). A metagenomic survey of viral abundance and diversity in mosquitoes from Hubei province. PLoS One 10:e0129845. doi: 10.1371/journal.pone.0129845
Sinka, M. E., Bangs, M. J., Manguin, S., Rubio-Palis, Y., Chareonviriyaphap, T., Coetzee, M., et al. (2012). A global map of dominant malaria vectors. Parasit. Vectors 5:69. doi: 10.1186/1756-3305-5-69
Sinka, M. E., Pironon, S., Massey, N. C., Longbottom, J., Hemingway, J., Moyes, C. L., et al. (2020). A new malaria vector in Africa: predicting the expansion range of Anopheles stephensi and identifying the urban populations at risk. Proc. Natl. Acad. Sci. U.S.A. 117, 24900–24908. doi: 10.1073/pnas.2003976117
Smith, R. C., Barillas-Mury, C., and Jacobs-Lorena, M. (2015). Hemocyte differentiation mediates the mosquito late-phase immune response against Plasmodium in Anopheles gambiae. Proc. Natl. Acad. Sci. U.S.A. 112, E3412–E3420. doi: 10.1073/pnas.1420078112
Soltani, A., Vatandoost, H., Oshaghi, M. A., Enayati, A. A., and Chavshin, A. R. (2017). The role of midgut symbiotic bacteria in resistance of Anopheles stephensi (Diptera: Culicidae) to organophosphate insecticides. Pathog. Glob. Health 111, 289–296. doi: 10.1080/20477724.2017.1356052
Steyn, A., Roets, F., and Botha, A. (2016). Yeasts associated with Culex pipiens and Culex theileri mosquito larvae and the effect of selected yeast strains on the ontogeny of Culex pipiens. Microb. Ecol. 71, 747–760. doi: 10.1007/s00248-015-0709-1
Tajedin, L., Hashemi, J., Abaei, M., Hosseinpour, L., Rafei, F., and Basseri, H. (2009). Study on fungal flora in the midgut of the larva and adult of the different populations of the malaria vector Anopheles stephensi. Iran. J. Arthropod Borne Dis. 3, 36–40.
Tawidian, P., Rhodes, V. L., and Michel, K. (2019). Mosquito-fungus interactions and antifungal immunity. Insect Biochem. Mol. Biol. 111:103182. doi: 10.1016/j.ibmb.2019.103182
Tchioffo, M. T., Boissière, A., Abate, L., Nsango, S. E., Bayibéki, A. N., Awono-Ambéné, P. H., et al. (2016). Dynamics of bacterial community composition in the malaria mosquito’s epithelia. Front. Microbiol. 6:1500. doi: 10.3389/fmicb.2015.01500
Tchioffo, M. T., Boissière, A., Churcher, T. S., Abate, L., Gimonneau, G., Nsango, S. E., et al. (2013). Modulation of malaria infection in Anopheles gambiae mosquitoes exposed to natural midgut bacteria. PLoS One 8:e81663. doi: 10.1371/journal.pone.0081663
Valero-Jiménez, C. A., Debets, A. J. M., van Kan, J. A. L., Schoustra, S. E., Takken, W., Zwaan, B. J., et al. (2014). Natural variation in virulence of the entomopathogenic fungus Beauveria bassiana against malaria mosquitoes. Malar. J. 13:479. doi: 10.1186/1475-2875-13-479
Vizioli, J., Bulet, P., Charlet, M., Lowenberger, C., Blass, C., Müller, H. M., et al. (2000). Cloning and analysis of a cecropin gene from the malaria vector mosquito, Anopheles gambiae. Insect Mol. Biol. 9, 75–84. doi: 10.1046/j.1365-2583.2000.00164.x
Vizioli, J., Bulet, P., Hoffmann, J. A., Kafatos, F. C., Müller, H.-M., and Dimopoulos, G. (2001). Gambicin: a novel immune responsive antimicrobial peptide from the malaria vector Anopheles gambiae. Proc. Natl. Acad. Sci. U.S.A. 98, 12630–12635. doi: 10.1073/pnas.221466798
Walker, E. D., Olds, E. J., and Merritt, R. W. (1988). Gut content analysis of mosquito larvae (Diptera: Culicidae) using DAPI stain and epifluorescence microscopy. J. Med. Entomol. 25, 551–554. doi: 10.1093/jmedent/25.6.551
Wang, M., An, Y., Gao, L., Dong, S., Zhou, X., Feng, Y., et al. (2021). Glucose-mediated proliferation of a gut commensal bacterium promotes Plasmodium infection by increasing mosquito midgut pH. Cell Rep. 35:108992. doi: 10.1016/j.celrep.2021.108992
Wang, S., Dos-Santos, A. L. A., Huang, W., Liu, K. C., Oshaghi, M. A., Wei, G., et al. (2017). Driving mosquito refractoriness to Plasmodium falciparum with engineered symbiotic bacteria. Science 357, 1399–1402. doi: 10.1126/science.aan5478
Wang, Y., Gilbreath, T. M. III, Kukutla, P., Yan, G., and Xu, J. (2011). Dynamic gut microbiome across life history of the malaria mosquito Anopheles gambiae in Kenya. PLoS One 6:e24767. doi: 10.1371/journal.pone.0024767
Wang, Y., Wang, Y., Zhang, J., Xu, W., Zhang, J., and Huang, F. S. (2013). Ability of TEP1 in intestinal flora to modulate natural resistance of Anopheles dirus. Exp. Parasitol. 134, 460–465. doi: 10.1016/j.exppara.2013.04.003
Wei, G., Lai, Y., Wang, G., Chen, H., Li, F., and Wang, S. (2017). Insect pathogenic fungus interacts with the gut microbiota to accelerate mosquito mortality. Proc. Natl. Acad. Sci. U.S.A. 114, 5994–5999. doi: 10.1073/pnas.1703546114
Wong, M. L., Liew, J. W. K., Wong, W. K., Pramasivan, S., Mohamed Hassan, N., Wan Sulaiman, W. Y., et al. (2020). Natural Wolbachia infection in field-collected Anopheles and other mosquito species from Malaysia. Parasit. Vectors 13:414. doi: 10.1186/s13071-020-04277-x
World Health Organization [WHO] (2020). World Malaria Report 2020: 20 Years of Global Progress and Challenges. Available Online at: https://www.who.int/publications-detail-redirect/9789240015791 (accessed February 3, 2021).
World Health Organization [WHO] (2021). Coronavirus (COVID-19). WHO | Regional Office for Africa. Available online at: https://www.afro.who.int/health-topics/coronavirus-covid-19 [accessed December 9, 2021].
Wotton, R. S., Chaloner, D. T., Yardley, C. A., and Merritt, R. W. (1997). Growth of Anopheles mosquito larvae on dietary microbiota in aquatic surface microlayers. Med. Vet. Entomol. 11, 65–70. doi: 10.1111/j.1365-2915.1997.tb00291.x
Keywords: Anopheles, mosquito, microbiota, malaria, insecticide resistance
Citation: Djihinto OY, Medjigbodo AA, Gangbadja ARA, Saizonou HM, Lagnika HO, Nanmede D, Djossou L, Bohounton R, Sovegnon PM, Fanou M-J, Agonhossou R, Akoton R, Mousse W and Djogbénou LS (2022) Malaria-Transmitting Vectors Microbiota: Overview and Interactions With Anopheles Mosquito Biology. Front. Microbiol. 13:891573. doi: 10.3389/fmicb.2022.891573
Received: 07 March 2022; Accepted: 04 May 2022;
Published: 20 May 2022.
Edited by:
George Christophides, Imperial College London, United KingdomReviewed by:
Lizette Koekemoer, University of the Witwatersrand, South AfricaCopyright © 2022 Djihinto, Medjigbodo, Gangbadja, Saizonou, Lagnika, Nanmede, Djossou, Bohounton, Sovegnon, Fanou, Agonhossou, Akoton, Mousse and Djogbénou. This is an open-access article distributed under the terms of the Creative Commons Attribution License (CC BY). The use, distribution or reproduction in other forums is permitted, provided the original author(s) and the copyright owner(s) are credited and that the original publication in this journal is cited, in accordance with accepted academic practice. No use, distribution or reproduction is permitted which does not comply with these terms.
*Correspondence: Luc S. Djogbénou, bGRqb2diZW5vdTIyMDAyQHlhaG9vLmZy
Disclaimer: All claims expressed in this article are solely those of the authors and do not necessarily represent those of their affiliated organizations, or those of the publisher, the editors and the reviewers. Any product that may be evaluated in this article or claim that may be made by its manufacturer is not guaranteed or endorsed by the publisher.
Research integrity at Frontiers
Learn more about the work of our research integrity team to safeguard the quality of each article we publish.