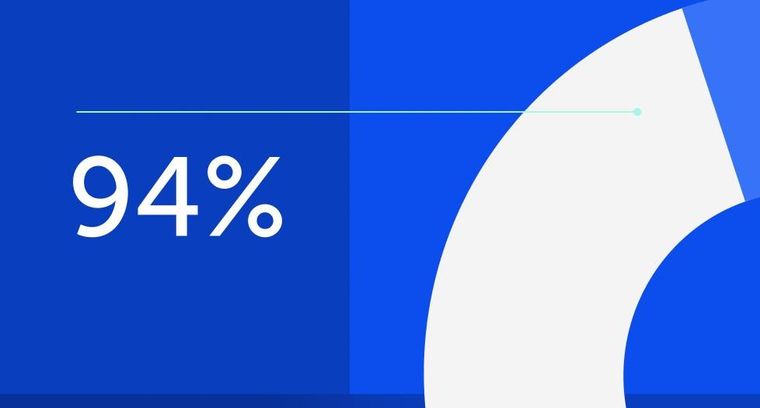
94% of researchers rate our articles as excellent or good
Learn more about the work of our research integrity team to safeguard the quality of each article we publish.
Find out more
ORIGINAL RESEARCH article
Front. Microbiol., 28 April 2022
Sec. Microbe and Virus Interactions with Plants
Volume 13 - 2022 | https://doi.org/10.3389/fmicb.2022.877082
This article is part of the Research TopicMicroorganisms and their Metabolites as Biocontrol Agents for Sustainable AgricultureView all 9 articles
Root-knot nematodes (RKNs) are soil-borne pathogens that severely affect Panax notoginseng growth and productivity. Thus, there is an urgent need for biological control agents or green nematicides to control root-knot nematodes. Rhizosphere bacteria can effectively control RKNs through different mechanisms. In this study, the three rhizosphere Bacillus strains, isolated from the root of P. notoginseng, were evaluated for the nematicidal activity and biological control efficacy against root-knot nematodes. In addition, we also evaluated the colonization ability of the two bacterial strains with significant biocontrol effect and dynamic regulation of genes related to systemic resistance in P. notoginseng. The rhizosphere Bacillus velezensis GJ-7 and Bacillus cereus NS-2 showed high nematicidal activity against Meloidogyne hapla in vitro and significantly reduced the number of root galls in three different control experiments. The results of colonization experiments showed that the strains GJ-7 and NS-2 colonized P. notoginseng root rapidly and stably. Additionally, the colonization of the strains NS-2 and GJ-7 activated the defense-responsive genes in P. notoginseng. These results indicated that the B. cereus strain NS-2 and B. velezensis strain GJ-7 have the potential for successful ecological niche occupation and enhance plant resistance and therefore could be considered as potential biocontrol agents against root-knot nematodes.
Root-knotnematodes (Meloidogyne spp.) are obligate plant parasites that cause severe damage to the host plant, including agricultural crops, vegetables, and even medicinal plants (Anwar and McKenry, 2010; Wang et al., 2021). Plant-parasitic nematodes cause losses of more than $157 billion worldwide every year (Anwar and McKenry, 2012). Due to the short life cycle and high reproduction rates of root-knot nematodes, the control of these has been particularly challenging. Previously, chemical pesticides are effective in controlling RKNs, such as carbofuran, dazomet, fenamiphos, and sebufos (Giannakou and Karpouzas, 2003). However, due to their high virulence, they have been found to be harmful to the eco-environment, animals, and humans and have been banned in many countries (Schneider et al., 2003; Nyczepir and Thomas, 2009). Therefore, researchers are also seeking new and alternative biological control options to solve these negative impacts and the significant economic losses. In the present study, the use of microbial agents to control plant-parasitic nematodes is a potential method, such as bacteria (Stirling et al., 2017; Viljoen et al., 2019), fungi (Hussain et al., 2018, 2020), and actinomycetes (Nimnoi et al., 2017), which are nematophagous or antagonistic for root-knot nematodes. Most notably, the genus Bacillus can significantly reduce root-knot nematode galling and egg production and has been commercially used in many countries for the control of plant-parasitic nematodes (Oka et al., 1993; Terefe et al., 2009; Higaki and Araujo, 2012; Jamal et al., 2017; Xiang et al., 2017).
In addition, the plant rhizosphere contains a large number of beneficial bacteria, which colonize on the root surface to form biofilms so as to protect plants from disease stresses and promote plant growth (Watnick and Kolter, 2000; Nan et al., 2021). Rhizosphere bacteria can effectively control RKNs through different mechanisms. Some bacteria can produce secondary metabolites with nematicidal activity (Kerry, 2000). Other bacteria species can rapidly colonize the feeding sites of RKNs and reduce the infection of root-knot nematodes by competing for niche and nutrition (Hashem and Abo-Elyousr, 2011). Bacteria can also alter plant root exudates and induce plant resistance to reduce RKN damage (Dutta et al., 2012). Therefore, plant rhizosphere bacteria play an important role in the control of nematode disease.
Sanqi [Panax notoginseng (Burk.) F. H. Chen] is one of the most important Chinese medicinal plants (Yang et al., 2015). In recent years, root-knot nematodes have seriously affected the growth and productivity of P. notoginseng (Wang et al., 2021). In previous studies, we obtained three rhizosphere Bacillus isolates from P. notoginseng, which were resistant to root-knot nematodes. According to the amplification results of 16S rRNA sequence, the three rhizosphere strains were identified as Bacillus cereus NS-2, Lysinibacillus NS-3, and Bacillus velezensis GJ-7, respectively. In this study, we evaluated their nematicidal activity and biological control efficacy against root-knot nematodes. In addition, we also evaluated the colonization ability of the NS-2 and GJ-7 strains with a significant biocontrol effect and dynamic regulation of genes related to systemic resistance in P. notoginseng. The purpose of this study was to screen the efficient bacterial strains for the control of the root-knot nematode to P. notoginseng and to provide a theoretical basis for the development of commercial biocontrol agents.
The strains NS-2, NS-3, and GJ-7 were obtained from the rhizosphere soil of healthy P. notoginseng plants under the forest in Lancang city (Yunnan, China). Egg masses were obtained from P. notoginseng plants infected with the second-stage Meloidogyne hapla juveniles (J2s) grown in the greenhouses of the Yunnan Agricultural University. Eggs were obtained by washing the egg masses with 2% NaOCl solution, and the egg masses were incubated in sterile water at 28°C for 24 h to collect J2s (Hussey and Barker, 1973; Huang et al., 2016). In addition, the seedlings of P. notoginseng were obtained from the Daheqiao farm of Yunnan Agricultural University (103°16′49′′E, 25°31′2′′N) for pot experiments.
First, the rhizosphere bacterial strains, NS-2, NS-3, and GJ-7, were cultured in 100 ml of LB liquid medium. The culture conditions were 30°C and 220 rpm for 48 h (Zhao et al., 2018). Then, the fermentation broth was collected by centrifuging the bacterial culture (8,000 rpm, 5 min) and filtering the supernatant with a 0.22-μm Millipore filter (Aalten and Gowen, 1998). The nematicidal activity of the rhizosphere bacterial strain was determined by a 24-well plate. Approximately 100 fresh J2s were contained in each well of a 24-well plate and treated with 1 ml of 100%, 50%, and 10% fermentation, 10% LB medium, sterilized water, and abamectin (1 μg/ml). The nematode mortality rate was recorded after 24-well plates were cultured at 28°C for 24 h. When the bodies of nematodes were straight and did not move upon stimulation with 0.5 M NaOH, the nematode was considered as dead (Harada and Yoshiga, 2015). The test was independently repeated three times, and each treatment had four replicates. The J2 mortality rate was calculated as follows: mortality rate = (the number of dead J2s/total J2s) × 100%.
Similar to the J2 mortality test described above, the effects of the strains NS-2, NS-3, and GJ-7 on egg hatching were tested in 24-well plates. One milliliter of 100%, 50%, and 10% fermentation broth, 10% LB medium, LB medium, and sterilized water were mixed with 100 eggs in separate wells. The 24-well plates were incubated in a chamber at 28°C for 72 h, and the number of J2s hatched in each well was recorded (Mendoza et al., 2008; Saikia et al., 2013). The egg hatching rate was determined as follows: hatching rate = (the number of hatched J2s/total eggs) × 100%.
Three experiments were designed for testing the potential of the strains NS-2, NS-3, and GJ-7 on biocontrol of the root-knot nematode and P. notoginseng growth promotion under greenhouse at Yunnan Agricultural University, China. P. notoginseng seedlings at the two-leaf stage were transplanted from the Daheqiao farm nursery land to individual pots filled with 200 g of sterilized soil. Then, the P. notoginseng seedlings were maintained at 25–28°C in a greenhouse. Treatments are as follows: (1) sterile water control, (2) LB medium control, (3) 1/1,000 dilution of abamectin control, (4) NS-2 fermentation broth, (5) NS-3 fermentation broth, and (6) GJ-7 fermentation broth. In Experiment 1, P. notoginseng plants were pre-inoculated with 10 ml of each of the three rhizosphere bacterial strains’ fermentation broth. Three days later, 1000 J2s of M. hapla in 5 ml of sterile water were inoculated into 2-cm-deep holes around each P. notoginseng stem. In Experiment 2, P. notoginseng plants were inoculated with 10 ml of rhizosphere bacteria fermentation broth and 1000 J2s at the same time. In Experiment 3, The 1000 J2s were inoculated into the P. notoginseng plants for 3 days, and then, 10 ml of bacterial fermentation broth was inoculated into each plant. Each treatment consisted of 10 pots and was repeated three times independently. The plant biomass values were measured after 30 days post-inoculation (dpi), including root length, stem length, stem fresh weight, and root fresh weight (Munif et al., 2013; Adam et al., 2014a). In addition, the level of nematode control was evaluated by detecting the number of galls on the roots of P. notoginseng.
The strain NS-2 was tagged with the green fluorescent protein (GFP), and the strain GJ-7 was tagged with the red fluorescent protein (RFP) for colonization study. To generate the GFP-tagged strain NS-2 and RFP-tagged strain GJ-7, the pGFP4412 plasmid (Wangqi, Beijing) and the pRFP315 plasmid (stored in our lab) were introduced in the strains NS-2 and GJ-7 by electroporation at 1.8 kV, respectively. In order to ensure that the fluorescently labeled strains can be used for the colonization and migration experiment tracking of the roots of P. notoginseng, the growth curve of fluorescently labeled strains and wild-type (WT) strains was measured.
To quantify the amount of strains NS-2 and GJ-7 in P. notoginseng roots, the population of fluorescently labeled strains in P. notoginseng root was determined at 1, 3, 5, 7, 15, and 30 days post-inoculation (dpi). First, 10 ml of the suspension (1.0 × 107 cfu/mL) was watered on P. notoginseng root. Three P. notoginseng plants were carefully taken out from the soil randomly each dpi. For the collection of roots samples, the P. notoginseng seedlings were delicately uprooted from the soil, and all loosely adhering bulk soil was removed, and 1 g of roots was put into 9 ml sterile water and vibrated on the oscillator for 30 min. The root samples were serially diluted and plated onto LB medium with an appropriate antibiotic. After culturing at 30°C for 2 days, the bacterial colonies were examined and counted from the roots.
In order to visually observe the colonization of the antagonistic strain in the root of P. notoginseng, the P. notoginseng seedlings were transferred to containers with 200 ml of 1/2 Hoagland culture medium and quartz sand. Then, they were inoculated with 20 ml of the NS-2-GFP or GJ-7-RFP overnight culture grown at 30°C in liquid LB supplemented with an appropriate antibiotic. Seedlings were incubated at 25°C for 5 days. Afterward, roots were washed with distilled water to eliminate non-adhered bacteria, and the root system was examined with a laser scanning confocal microscopy.
Two-leaf stage P. notoginseng seedlings were transferred to individual pots filled with 200 g of sterilized soil and maintained in a greenhouse as previously described. One week after transplanting, 10 ml of the GJ-7 and NS-2 cultured strains (1.0 × 107 cfu/mL) were watered on P. notoginseng roots as described previously. Three root samples were prepared at 0-, 12-, 24-, 48, and 72-h time points and stored at −80°C after quick freezing with liquid nitrogen. To perform gene expression analysis, the total RNA was extracted from root samples using the Pure Plant Kit (Omega), and cDNA was synthesized using the PrimeScript RT Reagent Kit with gDNA Eraser (Takara, Dalian). The expression of PnACT2 (internal control), PnPR1, and PnCHI1 genes was analyzed by a real-time PCR SYBR Green I reaction system using gene-specific primers, as described previously (Bai et al., 2018; Zhang et al., 2020). The expression level of each gene was normalized and related to that of PnACT2, which was quantified using the ΔΔCt method (Beaubois et al., 2007).
All data were recorded and organized in Microsoft Office Excel (2010). Statistical analysis was carried out using one-way analysis of variance (ANOVA) with Duncan’s multiple range test (P < 0.05) and Tukey’s multiple comparison test (P < 0.001) in DPS.
The mortality rate of J2s in the LB medium, 10% LB medium, and sterilized water was 17%, 13%, and 6.3% at 24 h, respectively (Figure 1). All fermentation broth treatments indicated significantly higher nematicidal activity against M. hapla J2s than negative controls (10% LB medium, LB medium, and sterilized water) at 24 h. Among them, the mortality rate of J2s in 100% fermentation broth of NS-2 was 82.6%, NS-3 was 81.6%, and GJ-7 was 84%. The mortality rate of J2s in 50% fermentation broth of NS-2 was 77%, NS-3 was 72.6%, and GJ-7 was 72.7%. The mortality rate of J2s in 10% fermentation broth of NS-2 was 53.66%, NS-3 was 46.67%, and GJ-7 was 49.33% (Figure 1). Although the mortality rate of J2s in the abamectin (positive control) was 81.66% at 24 h, the 100% and 50% fermentation broth of three rhizosphere bacterial strains had the same effect (Figure 1). The results of the nematicidal activity test in vitro showed that the fermentation broth of three rhizosphere bacteria displayed strong nematicidal abilities (Figure 1).
Figure 1. The mortality rate of the second-stage juveniles (J2s) of Meloidogyne hapla induced by Bacillus cereus strain NS-2, Lysinibacillus strain NS-3, and Bacillus velezensis GJ-7 cell-free supernatant in vitro. Abamectin (1 μg/ml), a strong biological nematicide, was used as a positive control, while LB medium, 10% LB medium, and sterilized water were used as negative controls. Data were analyzed by one-way ANOVA with Duncan’s multiple range test, and error bars represent the standard error of three biological and ten technical replicates (n = 30). Different lowercase letters indicate significant differences among treatments (P < 0.05).
At 72 h, at the highest concentration tested, the egg hatching rate was the lowest when treated with strain NS-2 fermentation (9.01%), followed by the NS-3 fermentation (9.86%) and GJ-7 fermentation (10.25%). At a concentration of 10 times diluted, the egg hatching rate of all fermentation treatments still was significantly lower than the LB (28.73%), 10% LB (38.73%), and sterilized water control (47.47%) (Figure 2). The egg hatching rate in 10% fermentation broth of NS-2 was 16.09%, NS-3 was 17.08%, and GJ-7 was 15.91% (Figure 2). The results in vitro showed that the fermentation broth of three rhizosphere bacterial strains could significantly inhibit the hatching of eggs.
Figure 2. The egg hatching rate of Meloidogyne hapla exposed to Bacillus cereus strain NS-2, Lysinibacillus strain NS-3, and Bacillus velezensis GJ-7 cell-free supernatant in vitro. LB medium, 10% LB medium, and sterilized water were used as negative controls. Data were analyzed by one-way ANOVA with Duncan’s multiple range test, and error bars represent standard error of three biological and ten technical replicates (n = 30). Different lowercase letters indicate significant differences of 72 h among treatments (P < 0.05).
The effect of rhizosphere bacteria fermentation broth in biocontrol of M. hapla and plant growth promotion was verified in a pot experiment. The results indicated that inoculation of the NS-2, NS-3, and GJ-7 strains in P. notoginseng roots in different experiments significantly reduced the number of galls compared with the negative control (sterile water and LB medium) (Figure 3). In the pre-inoculation of rhizosphere bacteria or rhizosphere bacteria and M. hapla that were inoculated at the same time treatments, abamectin (positive control) showed higher efficacies in reducing the number of galls, but there was no significant difference with NS-2 and GJ-7 treatments (Figures 3A,B). Compared with the water control, abamectin treatment reduced the number of galls by 85%, and pre-inoculation of NS-2, NS-3, and GJ-7 treatments reduced the number of galls by 74.3%, 63.3%, and 72.5%, respectively. When rhizosphere bacteria and M. hapla were inoculated at the same time treatment, the number of galls reduced by 77.6%, 74%, and 77.6%, respectively. Although, the pre-inoculation with M. hapla did not have a solid effect on the three bacteria isolates on the reduction of the galls compared with abamectin control (Figure 3C). However, the number of galls still decreased by 64%, 54.5%, and 64.4%, respectively. In addition, all treatments with NS-2 and GJ-7 exhibited higher efficacies in reducing the number of galls, followed by NS-3. These results suggest that rhizosphere bacteria have protective and therapeutic effects. In contrast, the protective effect is stronger, which may be related to the predetermined colonization of rhizosphere bacteria. The growth parameters presented in Supplementary Table 1 revealed that the three rhizosphere bacterial isolates have different effects on plant growth. In all treatments, NS-2 and GJ-7 enhanced root length and stem length, but compared with the control, the root fresh weight and stem fresh weight are not significant (Supplementary Table 1).
Figure 3. The rhizosphere bacterial strains NS-2, NS-3, and GJ-7 in biological control potential of Panax notoginseng plants infected with Meloidogyne hapla in three treatments of pot experiment. Means in each column followed by the same letter do not differ significantly according to Duncan’s multiple range test at P ≤ 0.05.
We successfully transferred the green fluorescent protein plasmid pGFP4412 and the red fluorescent protein plasmid pRFP315 to the strains NS-2 and GJ-7, respectively. The transformed strains were observed under a fluorescence microscope, and the results showed that the plasmid has been successfully transformed into the NS-2 and GJ-7 strains and could express the fluorescent normally (Figure 4). In addition, the growth curve of the GFP-tagged strain NS-2, RFP-tagged strain GJ-7, and WT strains was measured under the same inoculation and culture conditions. The results showed that the growth of GFP-NS-2 and RFP-GJ-7 was consistent with WT strains (Figure 5), indicating that the plasmids, pGFP4412 and pRFP315, had no significant effect on the growth of NS-2 and GJ-7 strains, and the tagged strains can be used for experiment tracing colonization and migration in plants.
Figure 4. Fluorescence observation of NS-2 and GJ-7 under microscopy. DIC, differential interference contrast field; RFP, red fluorescent field; and GFP, green fluorescent field. Representative fluorescent micrograph of (A) NS-2 and (B) GJ-7 under a fluorescent microscope.
Figure 5. The growth rate of the tagged strain compared with the wild-type strain. OD600 measures were used to determine bacterial growth rate. (A) Growth rate of the strain NS-2. (B) Growth rate of the strain GJ-7. Three replicates were included.
The population of GFP-tagged strain NS-2 and RFP-tagged strain GJ-7 on P. notoginseng roots was monitored at 1, 3, 5, 7, 15, and 30 days after inoculation, as described in Materials and Methods. GFP-NS-2 and RFP-GJ-7 can rapidly proliferate in large quantities within 5 dpi on the P. notoginseng roots. The population of GFP-NS-2 and RFP-GJ-7 in the roots was stable, with ≥ 103 cfu/g of fresh weight until 30 dpi (Figure 6). In addition, RFP-GJ-7 was found to have the strongest colonization ability, and the maximum density of cells was 5.63 × 105 cfu/g of fresh weight at 5 dpi on the P. notoginseng roots; overall colonization was significantly higher than GFP-NS-2 (P ≤ 0.05).
Figure 6. Population of GFP-NS-2 and RFP-GJ-7 on Panax notoginseng roots. Population is expressed in log (cfu/g) of roots (y-axis) and days (x-axis).
To explore the colonization patterns of GFP-NS-2 and RFP-GJ-7 in P. notoginseng roots, we used the laser confocal microscope (LCM) to observe the roots of P. notoginseng inoculated with the GFP-NS-2 and RFP-GJ-7 strains for 5 days, respectively.
The results indicate that P. notoginseng roots were colonized by GFP-NS-2 and RFP-GJ-7, and GFP-NS-2 and RFP-GJ-7 were observed on root epidermal cells at 5 days after inoculation (DAI) (Figure 7).
Figure 7. Colonization observation of the strains NS-2 and GJ-7 on Panax notoginseng roots. (A) Images of GFP-tagged strain NS-2-treated roots in the dark field (GFP), the dark field (RFP), and the superposition field (merged). (B) Images of RFP-tagged strain GJ-7-treated roots in the dark field (GFP), the dark field (RFP), and the superposition field (merged).
The inoculation treatment with the NS-2 and GJ-7 strains upregulated the expression in P. notoginseng root of PnPR1 and PnCHI1 genes. The PR1 gene-encoding proteins are involved in the SA signaling pathway. In addition, chitinase (CHI) is also an important part of the disease resistance and defense response system. In the inoculation treatment with NS-2 strain, the expression of PnPR1 and PnCHI1 gene was significantly upregulated compared with the control (0 h) after 24 h (Figures 8A,B). In the inoculation treatment with GJ-7 strain, the expression of PnPR1 gene was lower than that of the control within 24 h of inoculation, but its expression has a trend of continuous growth. After 48 h, the PnPR1 gene expression level exceeds that of the control. The expression level of PnCHI1 gene was higher than the control in all time periods, reaching a peak at 48 h (Figures 8C,D). These results indicated that the strains NS-2 and GJ-7 activate the defense signaling pathways in P. notoginseng roots.
Figure 8. Analysis of defense response in Panax notoginseng roots treated with the strains NS-2 and GJ-7. (A,B) Relative expression level of PnPR1 (A) and PnCHI1 (B) in P. notoginseng roots treated with strain NS-2. (C,D) Relative expression level of PnPR1 (C) and PnCHI1 (D) in P. notoginseng treated with the strain GJ-7. Expression levels were normalized relative to the control at the 0 h time point. Data were analyzed by one-way ANOVA with Duncan’s multiple range test, and error bars represent standard error of three biological and three technical replicates (n = 9). Different lowercase letters indicated significant differences among treatments (P < 0.05).
Antagonistic bacteria are generally considered as effective microorganisms for the biocontrol of root-knot nematode (Adam et al., 2014b; Xiong et al., 2015) as well as plant growth-promoting bacteria (Vetrivelkalai et al., 2010). However, few studies have reported the possibility of B. cereus and B. velezensis as rhizosphere bacteria to serve as a biocontrol of M. hapla. In this study, the B. cereus NS-2, Lysinibacillus NS-3, and B. velezensis GJ-7 from the rhizosphere of P. notoginseng plants showed strong nematicidal activity to M. hapla J2s and suppressed the hatching of eggs in vitro. These results indicated that three rhizosphere bacteria may kill M. hapla J2s and its eggs before infecting P. notoginseng plants. In addition, the results of the pot experiment showed that B. cereus NS-2 and B. velezensis GJ-7 could effectively control M. hapla and promote plant growth. These data suggest that the strains NS-2 and GJ-7 can be developed as biological nematicides to control M. hapla.
In addition, comparing the efficiency biocontrol of M. hapla under three experiments, infection by M. hapla was significantly reduced by rhizosphere bacteria pre-colonization. These results indicate that root colonization by rhizosphere bacteria is a prerequisite for the biological control of pathogens. As previously reported, some beneficial microorganisms can colonize the root surface to form biofilm so as to protect plants against pathogen infection (Davey and O’toole, 2000; O’Toole et al., 2000). Root colonization by bacteria could prevent nematode infection by inhibiting J2 hatching, motility, and viability (Mendoza et al., 2008; Terefe et al., 2009; Xiong et al., 2015). In this study, to confirm the colonization of P. notoginseng roots by the strains NS-2 and GJ-7, we generated the GFP-tagged strain NS-2 and RFP-tagged strain GJ-7. In our experiment, the strains NS-2 and GJ-7 occupied the ecological niche, with a rapidly growing population of up to 3.46 × 104 and 3.53 × 105 cfu/g of roots for 5 dpi, respectively. The population of two bacterial colonization was stabilized in P. notoginseng roots and maintained at 103 cfu/g of roots in 30 days. The colonization of NS-2 and GJ-7 on the root surface of P. notoginseng was also observed under the CLSM. These data indicate that the strains NS-2 and GJ-7 have a large ability of establishment and persistence either on the P. notoginseng roots.
Previously, some studies reported that bacterial colonization of the root surface can enhance the host defense mechanism and reduce the invasion of RKNs (Xiang et al., 2017). Vos et al. (2013) suggested that bacteria might enhance the activity of biomolecules and enzymes related to plant defense against root-knot nematodes. The major defense mechanisms of plants were regulated through SA and JA signaling pathways, and the PR1 gene was the marker of the signaling pathway (Shoresh et al., 2005; Islam et al., 2008; Pulla et al., 2010). The PnPR1 gene is the only gene cloned in the PR1 family of P. notoginseng (Zhang et al., 2020). In this study, the expression of PnPR1 gene was increased, indicating that the defense response of P. notoginseng to M. hapla mediated by the SA signaling pathway was activated. In addition, chitinase (CHI) is also an important part of the disease resistance and defense response system. Studies have shown that chitinase has the activity of inhibiting the growth of fungal hyphae (Bai et al., 2018). The increase in the expression of the PnCHI1 gene (the chitinase gene in P. notoginseng) also indicates the activation of the defense response of P. notoginseng. Due to the limitation of reports on the defense-related genes of P. notoginseng, this study only proved the expression of two related genes. However, these data confirmed that the strains NS-2 and GJ-7 activate defense responses in P. notoginseng roots against M. hapla.
In summary, the B. cereus NS-2 and B. velezensis GJ-7 from the rhizosphere of P. notoginseng exhibited direct nematicidal and egg inhibition against M. hapla and formed a biofilm on the surface of P. notoginseng roots, which effectively prevented nematode invasion. In addition, the strains NS-2 and GJ-7 also stimulated defensive responses to enhance plant resistance against M. hapla. Taken together, our results revealed a possible anti-nematode mechanism of rhizosphere bacteria from P. notoginseng. B. cereus NS-2 and B. velezensis GJ-7 with nematicidal activity help Panax notoginseng against root-knot nematodes through rapid colonization and mediated resistance.
The raw data supporting the conclusions of this article will be made available by the authors, without undue reservation.
WW conceived, designed, and performed the experiment, analyzed the data, and wrote the manuscript. YW conceived and designed the experiment and prepared the manuscript. JW and ZW analyzed the data. LG and SZ participated in the revision of the manuscript. XH and YZ supervised the research and provided funding support. All authors contributed to the article and approved the submitted version.
This work was supported by the China Agriculture Research System (CARS-21) and the Major Science and Technology Project of Yunnan and Kunming (202102AE090042 and 2021JH002).
The authors declare that the research was conducted in the absence of any commercial or financial relationships that could be construed as a potential conflict of interest.
All claims expressed in this article are solely those of the authors and do not necessarily represent those of their affiliated organizations, or those of the publisher, the editors and the reviewers. Any product that may be evaluated in this article, or claim that may be made by its manufacturer, is not guaranteed or endorsed by the publisher.
The Supplementary Material for this article can be found online at: https://www.frontiersin.org/articles/10.3389/fmicb.2022.877082/full#supplementary-material
Aalten, P., and Gowen, S. (1998). Entomopathogenic nematodes and fluorescent Pseudomonas rhizosphere bacteria inhibiting Radopholus simlis invasion in banana roots. Brighton Crop Prot. Conf. Pests Dis. 2, 675–680.
Adam, M., Heuer, H., and Hallmann, J. (2014a). Bacterial antagonists of fungal pathogens also control root-knot nematodes by induced systemic resistance of tomato plants. PLoS One 9:e90402. doi: 10.1371/journal.pone.0090402
Adam, M., Westphal, A., Hallmann, J., and Heuer, H. (2014b). Specific microbial attachment to root-knot nematodes in suppressive soil. Appl. Environ. Microbiol. 80, 2679–2686. doi: 10.1128/AEM.03905-13
Anwar, S. A., and McKenry, M. V. (2010). Incidence and reproduction of Meloidogyne incognita on vegetable crop genotypes. Pak. J. Zool. 42, 135–141.
Anwar, S. A., and McKenry, M. V. (2012). Incidence and population density of plant-parasitic nematodes infecting vegetable crops and associated yield losses. Pak. J. Zool. 44, 327–333. doi: 10.1670/11-013
Bai, Z., Pu, L., Tang, B., Wang, Q., Cui, X., and Liu, D. (2018). Functional analysis of a chitinase gene PnCHI1 from Panax notoginseng. Zhongguo Zhong Yao Za Zhi 43, 1832–1837. doi: 10.19540/j.cnki.cjcmm.20180201.012
Beaubois, E., Girard, S., Lalléchère, S., Davies, E., Paladian, F., Bonnet, P., et al. (2007). Intercellular communication in plants: evidence for two rapidly transmitted systemic signals generated in response to electromagnetic field stimulation in tomato. Plant Cell Environ. 30, 834–844. doi: 10.1111/j.1365-3040.2007.01669.x
Davey, M. E., and O’toole, G. A. (2000). Microbial biofilms: from ecology to molecular genetics. Microbiol. Mol. Biol. R. 64, 847–867. doi: 10.1128/MMBR.64.4.847-867.2000
Dutta, T. K., Powers, S. J., Gaur, H. S., Birkett, M., and Curtis, R. H. C. (2012). Effect of small lipophilic molecules in tomato and rice root exudates on the behaviour of Meloidogyne incognita and M. graminicola. Nematology 14, 309–320. doi: 10.1163/156854111X612306
Giannakou, I. O., and Karpouzas, D. G. (2003). Evaluation of chemical and integrated strategies as alternatives to methyl bromide for the control of root-knot nematodes in Greece. Pest Manag. Sci. 59, 883–892. doi: 10.1002/ps.692
Harada, Y., and Yoshiga, T. (2015). Distinguishing between inactivated and dead second stage juveniles of Meloidogyne incognita using the NaOH method. Nematol. Res. 45, 51–55. doi: 10.3725/jjn.45.51
Hashem, M., and Abo-Elyousr, K. A. M. (2011). Management of the root-knot nematode Meloidogyne incognita on tomato with combinations of different biocontrol organisms. Crop Prot. 30, 285–292. doi: 10.1016/j.cropro.2010.12.009
Higaki, W. A., and Araujo, F. F. D. (2012). Bacillus subtilis and abamectin for nematode control and physiological changes in cotton grown in soil naturally infested. Nematropica 42, 295–303. doi: 10.1186/1742-9994-9-34
Huang, Y.-H., Mao, Z.-C., and Xie, B.-Y. (2016). Chinese leek (Allium tuberosum Rottler ex Sprengel) reduced disease symptom caused by root-knot nematode. J. Integr. Agr. 15, 364–372. doi: 10.1016/S2095-3119(15)61032-2
Hussain, M., Maòasová, M., Zouhar, M., and Rysanek, P. (2020). Comparative virulence assessment of different nematophagous fungi and chemicals against northern root-knot nematodes, Meloidogyne hapla, on carrots. Pak. J. Zool. 52, 199–206. doi: 10.17582/journal.pjz/2020.52.1.199.206
Hussain, M., Zouhar, M., and Rysanek, P. (2018). Suppression of Meloidogyne incognita by the entomopathogenic fungus Lecanicillium muscarium. Plant Dis. 102, 977–982. doi: 10.1094/PDIS-09-17-1392-RE
Hussey, R. S., and Barker, K. R. (1973). Comparison of methods of collecting inocula of Meloidogyne spp., including a new technique. Plant Dis. Rep. 57, 1025–1028. doi: 10.1007/BF00010711
Islam, S. Z., Babadoost, M., Bekal, S., and Lambert, K (2008). Red light-induced systemic disease resistance against root-knot nematode Meloidogyne javanica and Pseudomonas syringae pv. tomato DC 3000. J. Phytopathol. 156, 708–714. doi: 10.1111/j.1439-0434.2008.01435.x
Jamal, Q., Cho, J.-Y., Moon, J.-H., Munir, S., Anees, M., and Kim, K. Y. (2017). Identification for the first time of cyclo(d-Pro-l-Leu) produced by Bacillus amyloliquefaciens Y1 as a nematocide for control of Meloidogyne incognita. Molecules 22:1839. doi: 10.3390/molecules22111839
Kerry, B. R. (2000). Rhizosphere interactions and the exploitation of microbial agents for the biological control of plant-parasitic nematodes. Annu. Rev. Phytopathol. 38, 423–441. doi: 10.1146/annurev.phyto.38.1.423
Mendoza, A. R., Kiewnick, S., and Sikora, R. A. (2008). In vitro activity of Bacillus firmus against the burrowing nematode Radopholus similis, the root-knot nematode Meloidogyne incognita and the stem nematode Ditylenchus dipsaci. Biocontrol Sci. Technol. 18, 377–389. doi: 10.1080/09583150801952143
Munif, A., Hallmann, J., and Sikora, R. (2013). The influence of endophytic bacteria on Meloidogyne incognita infection and tomato plant growth. J. ISSAAS 19, 68–74.
Nan, Y., JianLong, Z., Rui, L., Yan, L., Jian, L., YuHong, Y., et al. (2021). Biocontrol efficacy of Bacillus cereus strain Bc-cm103 against Meloidogyne incognita. Plant Dis. 105, 2061–2070. doi: 10.1094/PDIS-03-20-0648-RE
Nimnoi, P., Pongsilp, N., and Ruanpanun, P. (2017). Monitoring the efficiency of Streptomyces galilaeus strain KPS-C004 against root knot disease and the promotion of plant growth in the plant-parasitic nematode infested soils. Biol. Control 114, 158–166. doi: 10.1016/j.biocontrol.2017.08.016
Nyczepir, A. P., and Thomas, S. H. (2009). “Current and future management strategies in intensive crop production systems,” in Root-knot Nematodes, eds R. N. Perry, M. Moens, and J. L. Starr (Wallingford: CAB International), doi: 10.1016/0011-2275(86)90009-3
Oka, Y., Chet, I., and Spiegel, Y. (1993). Control of the root-knot nematode Meloidogyne javanica by Bacillus cereus. Biocontrol Sci. Technol. 3, 115–126. doi: 10.1080/09583159309355267
O’Toole, G., Kaplan, H. B., and Kolter, R. (2000). Biofilm formation as microbial development. Annu. Rev. Microbiol. 54, 49–79. doi: 10.1146/annurev.micro.54.1.49
Pulla, R. K., Lee, O. R., In, J. G., Kim, Y. J., Senthil, K., and Yang, D. C. (2010). Expression and functional characterization of pathogenesis-related protein family 10 gene, PgPR10-2, from Panax ginseng C.A. Meyer. Physiol. Mol. Plant Pathol. 74, 323–329. doi: 10.1016/j.pmpp.2010.05.001
Saikia, S. K., Tiwari, S., and Pandey, R. (2013). Rhizospheric biological weapons for growth enhancement and Meloidogyne incognita management in Withania somnifera cv. Poshita. Biol. Control 65, 225–234. doi: 10.1016/j.biocontrol.2013.01.014
Schneider, S. M., Rosskopf, E. N., Leesch, J. G., Chellemi, D. O., Bull, C. T., and Mazzola, M. (2003). United States Department of Agriculture-Agricultural Research Service research on alternatives to methyl bromide: pre-plant and post-harvest. Pest Manage. Sci. 59, 814–826. doi: 10.1002/ps.728
Shoresh, M., Yedidia, I., and Chet, I. (2005). Involvement of jasmonic acid/ethylene signaling pathway in the systemic resistance induced in cucumber by Trichoderma asperellum T203. Phytopathology 95, 76–84. doi: 10.1094/PHYTO-95-0076
Stirling, G. R., Wong, E., and Bhuiyan, S. (2017). Pasteuria, a bacterial parasite of plant-parasitic nematodes: Its occurrence in Australian sugarcane soils and its role as a biological control agent in naturally-infested soil. Australas. Plant Pathol. 46, 563–569. doi: 10.1007/s13313-017-0522-z
Terefe, M., Tefera, T., and Sakhuja, P. K. (2009). Effect of a formulation of Bacillus firmus on root-knot nematode Meloidogyne incognita infestation and the growth of tomato plants in the greenhouse and nursery. J. Invertebr. Pathol. 100, 94–99. doi: 10.1016/j.jip.2008.11.004
Vetrivelkalai, P., Sivakumar, M., and Jonathan, E. (2010). Biocontrol potential of endophytic bacteria on Meloidogyne incognita and its effect on plant growth in bhendi. J. Biopest. 3, 452–457.
Viljoen, J. J. F., Labuschagne, N., Fourie, H., and Sikora, R. A. (2019). Biological control of the root-knot nematode Meloidogyne incognita on tomatoes and carrots by plant growth-promoting rhizobacteria. Trop. Plant Pathol. 44, 284–291. doi: 10.1007/s40858-019-00283-2
Vos, C., Schouteden, N., van Tuinen, D., Chatagnier, O., Elsen, A., De Waele, D., et al. (2013). Mycorrhiza-induced resistance against the root-knot nematode Meloidogyne incognita involves priming of defense gene responses in tomato. Soil Biol. Biochem. 60, 45–54. doi: 10.1016/j.soilbio.2013.01.013
Wang, Z., Wang, W., Liu, Y., Jiang, C., Yang, K., Zhu, Y., et al. (2021). Investigation and infection source analysis of root knot nematode disease of Panax notoginseng in Lancang County, Yunnan Province. J. Yunnan Agric. Univ. 36, 60–68.
Watnick, P., and Kolter, R. (2000). Biofilm, city of microbes. J. Bacteriol. 10, 2675–2679. doi: 10.1128/JB.182.10.2675-2679.2000
Xiang, N., Lawrence, K. S., Kloepper, J. W., Donald, P. A., McInroy, J. A., and Lawrence, G. W. (2017). Biological control of Meloidogyne incognita by spore-forming plant growth-promoting rhizobacteria on cotton. Plant Dis. 101, 774–784. doi: 10.1094/PDIS-09-16-1369-RE
Xiong, J., Zhou, Q., Luo, H., Xia, L., Li, L., Sun, M., et al. (2015). Systemic nematicidal activity and biocontrol efficacy of Bacillus firmus against the root-knot nematode Meloidogyne incognita. World J. Microb. Biotechnol. 31, 661–667. doi: 10.1007/s11274-015-1820-7
Yang, M., Zhang, X., Xu, Y., Mei, X., Jiang, B., Liao, J., et al. (2015). Autotoxic ginsenosides in therhizosphere contribute to the replant failure of Panax notoginseng. PLoS One 10:e0118555. doi: 10.1371/journal.pone.0118555
Zhang, Y., Chen, H., Zheng, L., Wang, Z., Cui, X., Ge, F., et al. (2020). Expression Patterns and Functional Analysis of Pathogensis-related Protein PnPR1 Gene of Panax notoginseng. Acta Bot. Boreal. 40, 2000–2007. doi: 10.7606/j.issn.1000-4025.2020.12.2000
Keywords: rhizosphere bacteria, biological control, Meloidogyne hapla, rapid colonization, resistance induction
Citation: Wu W, Wang J, Wang Z, Guo L, Zhu S, Zhu Y, Wang Y and He X (2022) Rhizosphere Bacteria From Panax notoginseng Against Meloidogyne hapla by Rapid Colonization and Mediated Resistance. Front. Microbiol. 13:877082. doi: 10.3389/fmicb.2022.877082
Received: 16 February 2022; Accepted: 28 March 2022;
Published: 28 April 2022.
Edited by:
Ileana Vigentini, University of Milan, ItalyReviewed by:
Olivera Topalovic, Aarhus University, DenmarkCopyright © 2022 Wu, Wang, Wang, Guo, Zhu, Zhu, Wang and He. This is an open-access article distributed under the terms of the Creative Commons Attribution License (CC BY). The use, distribution or reproduction in other forums is permitted, provided the original author(s) and the copyright owner(s) are credited and that the original publication in this journal is cited, in accordance with accepted academic practice. No use, distribution or reproduction is permitted which does not comply with these terms.
*Correspondence: Yang Wang, d2FuZ3lhbmc2MjZAc2luYS5jb20=; Xiahong He, aGV4aWFob25nQGhvdG1haWwuY29t
Disclaimer: All claims expressed in this article are solely those of the authors and do not necessarily represent those of their affiliated organizations, or those of the publisher, the editors and the reviewers. Any product that may be evaluated in this article or claim that may be made by its manufacturer is not guaranteed or endorsed by the publisher.
Research integrity at Frontiers
Learn more about the work of our research integrity team to safeguard the quality of each article we publish.