- 1Marine Biology Section, University of Copenhagen, Helsingør, Denmark
- 2Israel Oceanographic and Limnological Research, Haifa, Israel
- 3Ocean Science Centre, Memorial University of Newfoundland, St. John’s, NL, Canada
- 4Institute for Biochemistry and Biology, Potsdam University, Potsdam, Germany
- 5Department of Plankton and Microbial Ecology, Leibniz-Institute of Freshwater Ecology and Inland Fisheries (IGB), Stechlin, Germany
- 6Max Planck Institute for Marine Microbiology, Bremen, Germany
- 7Department of Biological Oceanography, Leibniz Institute for Baltic Sea Research, Rostock, Germany
- 8Institute of Microbiology CAS, Centre ALGATECH, Třeboň, Czechia
- 9Aix Marseille Univ, Université de Toulon, CNRS, IRD, MIO, Marseille, France
- 10Turing Center for Living Systems, Aix-Marseille University, Marseille, France
- 11The Jacob Blaustein Institutes for Desert Research, Zuckerberg Institute for Water Research (ZIWR), Ben-Gurion University of the Negev, Be’er Sheva, Israel
Biological dinitrogen (N2) fixation is performed solely by specialized bacteria and archaea termed diazotrophs, introducing new reactive nitrogen into aquatic environments. Conventionally, phototrophic cyanobacteria are considered the major diazotrophs in aquatic environments. However, accumulating evidence indicates that diverse non-cyanobacterial diazotrophs (NCDs) inhabit a wide range of aquatic ecosystems, including temperate and polar latitudes, coastal environments and the deep ocean. NCDs are thus suspected to impact global nitrogen cycling decisively, yet their ecological and quantitative importance remain unknown. Here we review recent molecular and biogeochemical evidence demonstrating that pelagic NCDs inhabit and thrive especially on aggregates in diverse aquatic ecosystems. Aggregates are characterized by reduced-oxygen microzones, high C:N ratio (above Redfield) and high availability of labile carbon as compared to the ambient water. We argue that planktonic aggregates are important loci for energetically-expensive N2 fixation by NCDs and propose a conceptual framework for aggregate-associated N2 fixation. Future studies on aggregate-associated diazotrophy, using novel methodological approaches, are encouraged to address the ecological relevance of NCDs for nitrogen cycling in aquatic environments.
Introduction
Biological dinitrogen (N2) fixation, the conversion of dissolved N2 into ammonia, can represent a critical import of reactive nitrogen to the pelagic environment (Karl et al., 2002). This process is carried out by specialized prokaryotic microorganisms termed diazotrophs (Zehr and Turner, 2001). Aquatic studies have traditionally focused on photoautotrophic cyanobacterial diazotrophs inhabiting oligotrophic and sunlit environments where energy is made available via photosynthetic carbon fixation (Zehr, 2011). However, during the last decade it has become evident that non-cyanobacterial diazotrophs (NCDs; see Box 1) have an almost ubiquitous distribution in pelagic environments (Farnelid et al., 2011; Langlois et al., 2015; Geisler et al., 2020; Hallstrøm et al., 2021; Messer et al., 2021). For instance, the presence and/or expression of the nitrogenase gene (nifH) by NCDs has been reported from low latitude open oceans (Halm et al., 2011; Moisander et al., 2014; Langlois et al., 2015) to environments previously not regarded as suitable for N2 fixation such as eutrophic rivers, estuaries and coastal waters (Mulholland et al., 2012; Bentzon-Tilia et al., 2015; Geisler et al., 2020; Hallstrøm et al., 2021), the aphotic deep sea (Rahav et al., 2013; Benavides et al., 2015), and nutrient-rich arctic waters (Harding et al., 2018). A recent study suggested that some nifH genes are not functional (Mise et al., 2021). Yet, these genes relate to obligate anaerobic bacteria and their prevalence in the marine pelagic environment is likely minor. The activity of NCDs has been indirectly inferred by experimental manipulations that inhibit photoautotrophic activity (Rahav et al., 2016; Benavides et al., 2018b; Geisler et al., 2019, 2020) and from environments putatively void of cyanobacteria such as aphotic waters (Hamersley et al., 2011; Rahav et al., 2013; Benavides et al., 2015). Still, these methods cannot measure NCD-specific N2 fixation rates unambiguously. Thus, despite of the widespread distribution and activity of NCDs, their contribution to aquatic nitrogen cycling remains elusive (see reviews: Riemann et al., 2010; Bombar et al., 2016; Moisander et al., 2017; Benavides et al., 2018a; Marcarelli et al., 2022).
BOX 1 Key term definitions.
Non-cyanobacterial diazotrophs (NCDs): From a phylogenetic, rather than a metabolic, point of view, diazotrophs can be divided into cyanobacteria and non-cyanobacteria. The term NCDs has therefore been widely used in the literature. NCDs are a diverse group of prokaryotes with potentially diverse metabolic pathways (see definition below). In the context of bacterial growth and N2 fixation associated with aggregates, we consider degradation and uptake of organic matter (heterotrophy) the prevailing functionality. However, the reader should be aware that other metabolic strategies such as mixotrophy, photoheterotrophy or chemolithoautotrophy may also be present in NCDs.
Heterotrophic diazotrophs: Archaeal and bacterial N2-fixing microorganisms that require organic matter from external sources.
Metabolism: The combination of energy sources (light, chemical, and organic matter), electron flow and carbon (CO2 or organic matter) used by a microorganism to catalyze catabolic or anabolic processes.
Aggregates: Particles comprising live, dead and/or dormant cells, detritus and minerals that are held together by organic scaffolds. These particles are formed by the aggregation of organic material suspended in seawater. Aggregates are often rich in labile carbon and nutrients, and are therefore hotspots of microbial activity.
The marine water column is generally well oxygenated (except for oxygen minimum zones) and poor in labile organic matter (Arrieta et al., 2015; Santinelli, 2015), whereas the aphotic zone is rich in reactive nitrogen (e.g., Cavender-Bares et al., 2001). Therefore, the wide distribution of NCDs in these habitats with apparent unfavorable conditions for diazotrophy represents a lingering enigma. In this mini-review, we compile recent reports related to NCDs and focus on those associated with aggregates. We argue that the plot thickens [sensu (Azam, 1998)], and that compelling evidence supports the idea of planktonic aggregates as important microenvironments suitable for NCD N2 fixation. We emphasize the need for direct in situ measurements of aggregate-associated, NCD-specific N2 fixation, and provide guidelines for how these can be obtained in future studies. We note that this review paper focuses on marine and estuarine environments, as most data are available from such environments, but acknowledge that NCDs are also found in freshwater ecosystems (Coyne et al., 2020; Fernandez et al., 2020; Geisler et al., 2020).
The Plot Thickens: Previous and New Insights on Aggregate-Associated N2 Fixation
Aggregates are ubiquitous throughout marine and freshwater environments (Alldredge and Gotschalk, 1988; Waite et al., 2000). They are formed by the coagulation of live and dead plankton material (Smith et al., 1992; Grossart and Ploug, 2000; Piontek et al., 2009; Daly et al., 2016). The elevated micronutrient and macronutrient concentration relative to the surrounding waters fosters colonization by dense communities of prokaryotes (del Giorgio and Cole 1998; Simon et al., 2002; Bar-Zeev and Rahav 2015), making aggregates ‘hot spots’ of intense microbial activity (Azam and Long, 2001). More than three decades ago, Hans Paerl and co-workers (Paerl, 1985; Paerl and Prufert, 1987) suggested that NCD N2 fixation may take place in low oxygen microzones within aggregates. This idea was reiterated in several later studies (Riemann et al., 2010; Sohm et al., 2011; Bombar et al., 2016), but has been substantiated only most recently (see below).
In the past, and especially during the last decade, evidence has accumulated for the association of NCDs with aquatic organisms and aggregates. NCDs have been isolated from copepods (Proctor, 1997) and nifH genes have been amplified and sequenced from copepods and euphausiids (Braun et al., 1999; Scavotto et al., 2015), and dinoflagellates (Farnelid et al., 2010). Moreover, individual and bulk aggregates collected with sediment traps deployed at 150 m depth in the open ocean contained nifH gene sequences of diverse NCDs (Farnelid et al., 2018). The prevalence of NCDs on aggregates has also been reported using metagenomics sequencing. In the Tara oceans dataset, representing 197 globally distributed pelagic oceanic metagenomes, the putative heterotrophic Planctomyces and Proteobacteria accounted for ~25% of the nifH reads obtained from the 180 to 2,000 μm size-fraction (Karlusich et al., 2021). Moreover, metagenome assembled genomes representing NCDs occurred in the 5–2,000 μm planktonic size-fractions (Delmont et al., 2021). Finally, one of the most widely distributed NCDs, Gamma-A, showed a ubiquitous presence in nifH genes across the North Atlantic Ocean quantified by qPCR in the >3 μm fraction (Benavides et al., 2016). This Gamma-A was also found in metatranscriptomes from the 3 to 2,000 μm size-fraction in the Tara oceans dataset, suggesting a filamentous or aggregate-attached lifestyle for this putative heterotrophic bacterium (Cornejo-Castillo and Zehr, 2020). Hence, both PCR-dependent and -independent approaches suggest the presence and/or activity of NCDs on aggregates.
Experimental data also suggest aggregate-associated N2 fixation by NCDs. In an early study from the Chesapeake Bay, United States, experiments by Guerinot and Colwell (1985) suggested that isolated strains of NCDs could fix N2 in the presence of plankton and particulate matter. In an experiment with aggregates from the Southern Indian Ocean, nifH genes related to Deltaproteobacteria were enriched in metatranscriptomes from experimental incubations with aggregates relative to controls without aggregates (Debeljak et al., 2021). Similarly, N2 fixation was stimulated in seawater from a Danish nutrient rich estuary and the Mediterranean Sea by amendment with natural aggregates (Pedersen et al., 2018) or a transparent exopolymer aggregate analog (gum-xanthan; Rahav et al., 2016), respectively. Hence, the presence of aggregates appears to stimulate N2 fixation by NCDs. Finally, presence of NCDs was recently documented on aggregates by immunolabeling of the nitrogenase enzyme while at the same time superimposing the aggregate matrix, total bacteria and cyanobacteria (Figure 1A). Collectively, the above-mentioned findings suggest that NCDs benefit from colonizing aggregates. Yet, our mechanistic understanding of how aggregates support N2 fixation by NCDs is still rather limited.
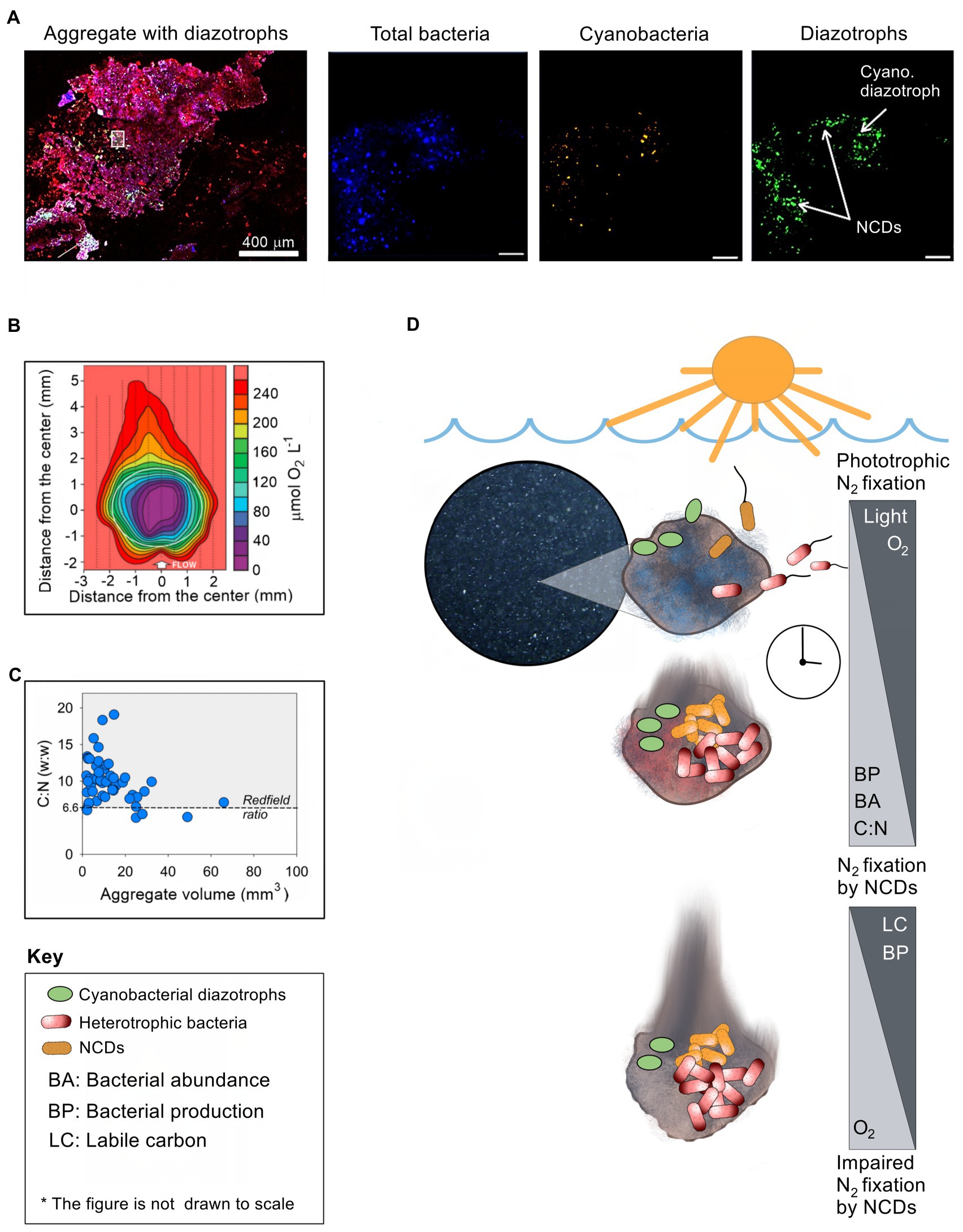
Figure 1. The association of NCDs with planktonic aggregates. (A) Enlarged confocal images of diazotrophs associated with aggregates after staining (red) the polysaccharide matrix by concanavalin A (Geisler et al., 2022). Enlarged images within the aggregate were captured to differentiate between three distinct channels (from left to right): total bacteria (stained by DAPI, blue); total cyanobacteria by autofluorescence of the phycoerythrin pigment (orange/white), and diazotrophs by immunolabeling nitrogenase enzyme. The white square on the aggregate shows the magnified location (scale bar of 10 μm). (B) Micro-sensor image showing the oxygen levels within a large (> 0.5 mm) planktonic aggregate (Klawonn et al., 2015). (C) Carbon to nitrogen ratio relative to aggregate size (Alldredge and Silver, 1988; Grossart and Ploug, 2001). (D) Conceptual figure illustrating time-course changes in conditions on an aggregate as it sinks in the water column, of key relevance for N2 fixation by associated NCDs. See text for explanation.
How Can Aggregates Support Heterotrophic Diazotrophy?
Theoretical considerations as well as experimental and field observations indicate that aggregates provide several conditions, which at least ephemerally, can support N2 fixation by NCDs: (1) Low oxygen conditions: Nitrogenase, a central enzyme for N2 fixation, is irreversibly damaged by molecular oxygen (Goldberg et al., 1987); however, aerobic respiration by bacteria that colonize the aggregate combined with slow diffusion rates (depending on the size and density of the particle) leads to local reduction in oxygen concentrations (Alldredge and Cohen, 1987; Paerl and Prufert, 1987; Ploug et al., 1997; Simon et al., 2002; Klawonn et al., 2015). The low oxygen levels in some parts of the aggregate vary from 50% to 90% air-saturation to anaerobic conditions on some occasions inside compact and large (few mm) aggregates (Ploug et al., 1997; Ploug, 2001; Klawonn et al., 2015). Consequently, low-oxygen microzones within aggregates likely provide loci where the nitrogenase enzyme is protected from oxygen (Figure 1B). (2) Metabolic energy: Aggregates are characterized by elevated levels of labile organic carbon relative to the surrounding waters and rapidly become colonized by diverse bacteria. Enzymatic hydrolysis of the aggregate matrix allows ample carbon and nutrient supply and extensive microbial growth (Ploug and Grossart, 2000). This organic-rich microenvironment can, therefore, support the high energy requirements associated with diazotrophy by NCDs. (3) Reactive nitrogen availability: The high C:N ratio of aggregates (Figure 1C), and the consequent reduction in nitrogen availability due to microbial growth, may provide NCDs a competitive edge over other bacteria unable to fix N2. (4) Trace metal and phosphorus availability: Diazotrophy requires trace metals such as iron (Berman-Frank et al., 2001) and molybdenum (Marino et al., 2003). Since aggregates usually contain higher concentrations of trace metals than ambient water (Jackson and Burd, 1998; Engel et al., 2004), inhabiting diazotrophs may gain efficient access to these nutrients, in particular in the presence of increased microbial activity. Based on these observations, it may be surmised that aggregates can provide conditions that are beneficial for N2 fixation by NCDs.
NCDs Associated With Aggregates: Towards a Conceptual Framework
Aggregates may provide favorable conditions for NCDs under various conditions in marine and freshwater environments. Yet, how these conceivably ephemeral conditions develop on aggregates and how NCDs exploit them is currently unclear. Based on the overall emerging picture outlined above, and recent experimental (Martínez-Pérez et al., 2018; Paerl et al., 2018) and modeling work (Chakraborty et al., 2021), we suggest a conceptual framework for N2 fixation by NCDs associated with aggregates (Figure 1D).
In the photic, well-oxidized zone, newly formed aggregates are sparsely colonized by microorganisms, thus limited respiration is expected. At that time, diffusion of oxygen from the surrounding water, and potential photosynthesis by associated phototrophs, will keep the aggregates well oxidized. If N2 fixation takes place, it is likely carried out mostly by associated cyanobacterial diazotrophs (Farnelid et al., 2018; Klawonn et al., 2019). It may be speculated that aggregate associated cyanobacterial diazotrophs can switch to mixotrophic metabolism to sustain N2 fixation as they sink to aphotic layers and photosynthesis is impaired (e.g., Rahav et al., 2013). Over time, aggregate-associated heterotrophic bacteria will proliferate, while preferentially exploiting labile nitrogen-rich organic compounds (Smith et al., 1992; Schneider et al., 2003), growing to cell concentrations commonly several orders of magnitude higher than in the surrounding water (Grossart and Simon, 1993; Turley and Mackie, 1994). This raises the C:N values of the aggregate over the Redfield ratio, gradually generating local nitrogen limitation (Figure 1C). At the same time extensive bacterial respiration exceeds the influx of oxygen diffusing from the surrounding water and causes formation of low oxygen microzones within the aggregate (Figure 1B). There is now a window of opportunity for N2 fixation by NCDs fueled by aerobic respiration. The low oxygen microzones may become anoxic if extensive bacterial respiration continues and exceeds the diffusive oxygen flux into the particle from the surrounding environment. NCDs may then switch to anaerobic respiration using nitrate or sulfate as alternative electron acceptors to meet their energetic requirements, as has been described for other aggregate-associated microbial processes (Wright et al., 2012; Bianchi et al., 2018) and recently modeled for NCDs (Chakraborty et al., 2021). The usual inhibition of N2 fixation by nitrate can be outweighed by enhanced diazotroph growth under low N:P ratio conditions (i.e., phosphate in excess; Knapp, 2012). However, it is unknown whether the high nitrate levels in deep waters may affect aggregate-associated NCD activity. It has been suggested that due to the high energetic costs associated with nitrate reduction, bacteria designed for diazotrophy may have few ecological reasons to use nitrate as a nitrogen source (Sprent and Sprent, 1990). In addition that high cell concentration near the surface of the aggregate may exhaust the supply of nitrate to the aggregate interior, supporting prevalence of sulfate over nitrate respiration within the aggregate (Chakraborty et al., 2021). Eventually, most of the labile carbon is consumed and heterotrophic respiration decreases. At that time, oxygen levels in the aggregate increase as oxygen consumption is exceeded by its diffusion from the surrounding water leading to significant reduction in N2 fixation rates by NCDs.
This conceptual framework for the interaction between NCDs and the dynamic environment on aggregates was recently modeled and yielded N2 fixation rates comparable to bulk rates measured in aphotic waters (Chakraborty et al., 2021), and agrees with field observations (Rahav et al., 2013, 2015; Benavides et al., 2016). Factors such as the level and type of substrate within the aggregate, the size of the aggregate, and its sinking speed may regulate the extent of aggregate associated N2 fixation both directly or indirectly, as they modulate the placement of the aggregate within the vertical gradients of nitrate, oxygen and carbon in the water column (Klawonn et al., 2015; Bianchi et al., 2018; Chakraborty et al., 2021).
New Approaches and Methods
How Much N2 Do NCDs Fix on Aggregates?
N2 fixation rates in aquatic environments are most commonly measured by 15N2 stable isotope labeling. Methodological challenges such as incomplete gas dissolution during incubations (Mohr et al., 2010) or contaminated gas stocks (Dabundo et al., 2014) causing under- or over-estimates of N2 fixation appear resolved (White et al., 2020). NCD-specific N2 fixation rates measurements have, however, remained elusive due to the coexistence of NCDs with cyanobacterial diazotrophs (Moisander et al., 2017). Approaches to distinguish the NCD N2 fixation signal from bulk rates have included dark incubations (Singh et al., 2021) and the addition of photosynthesis blocking agents (Rahav et al., 2016; Benavides et al., 2018b; Geisler et al., 2020). Unfortunately, these approaches cannot unambiguously measure NCD-specific N2 fixation rates since NCDs may be photoheterotrophic (Riemann et al., 2010). Moreover, blocking photosynthesis may not halt cyanobacterial N2 fixation at the expense of carbon storage, and alter the natural oxygen concentrations in incubation bottles (Table 1). Sample enrichment with 15N2 followed by nanoscale secondary ion mass spectrometry (nanoSIMS) yields cell-specific N2 fixation rates (Angel et al., 2018; Martínez-Pérez et al., 2018). The combination of nanoSIMS with phylogenetic or functional identity methods provides phylogenetic-specific N2 fixation rates (Musat et al., 2012), but hybridization preparations can dilute isotope signals impacting detectability when N2 fixation rates are low (Musat et al., 2014; Meyer et al., 2020; Table 1). Alternatives to circumvent this issue include correlation microscopy approaches and non-halogenated probes (gold-ISH; Kubota et al., 2014; Jiang et al., 2016; Table 1). In addition to the above, tagging the aggregate itself, while maintaining its structure during sample preparation for NanoSIMS or any other electron-based microscopy is highly challenging and calls for the development of dedicated sample preparation and imaging approaches.
What is the Distribution and Spatial Organization of NCDs on and Within Aggregates?
NCDs may be localized on single aggregates using various tagging methods. Immunolabeling of the nitrogenase enzyme is a universal method to detect active nitrogenases (Geisler et al., 2019). The localization of diazotrophs on the particle could be achieved by tagging the aggregate matrix and immunolabeling the diazotrophs (Geisler et al., 2019). Moreover, NCDs can be differentiated from cyanobacteria by superimposing nitrogenase immunolabeling and phycoerythrin fluorescence images (Geisler et al., 2019). This approach does, however, not provide phylogenetic information. Yet, the biochemical heterogeneity and chemical gradients within aggregates (Ploug, 2001; Klawonn et al., 2015) likely regulate the distribution of phylogenetically and functionally distinct microbes (Wright et al., 2012). This implies that the colonizing architecture of NCDs on aggregates needs to be considered from a 3D perspective. This may be partially approached by laser scanning confocal microscopy (Geisler et al., 2019, 2020) and/or other approaches such as resin embedding followed by microtome slicing and 3D image reconstruction to investigate the internal aggregate structure (Flintrop et al., 2018; Rogge et al., 2018).
Who Are the NCDs That Colonize Aggregates?
Barcoding, genomic and transcriptomic analyses have been applied on concentrated aggregate samples such as sediment trap material (Farnelid et al., 2018; Boeuf et al., 2019; Baumas et al., 2021). Such bulk approaches do, however, not allow visualizing the distribution of individual taxa at the single aggregate level. This would require specific methods such as rRNA oligonucleotide probes (catalyzed reporter deposition fluorescent in situ hybridization or CARD-FISH, (e.g., Thompson et al., 2012) and/or in combination with polynucleotide probes targeting specific gene fragments (geneFISH), which allows identifying individual phylogenetic groups expressing a gene of interest (Moraru et al., 2010). Recently, geneFISH was successfully used to quantify Gamma-A heterotrophic diazotrophs on marine aggregates (Harding, 2021).
How Important Is Aggregate-Associated N2 Fixation by NCDs for Aquatic Nitrogen Cycling?
Traditional approaches to sample aggregate-associated microbes include hand-picking by SCUBA diving (Alldredge and Gotschalk, 1988) and size-fractionation (Mestre et al., 2017). Given the heterogeneous distribution of aggregates in water columns, small volume sampling devices such as Niskin bottles underestimate aggregate abundance causing a bias towards free-living microbes and dissolved materials (Planquette and Sherrell, 2012; Puigcorbé et al., 2020). A plethora of devices that integrate larger water volumes such as in situ pumps, marine snow catchers and sediment traps exist today (McDonnell et al., 2015). While these provide a better representation of aggregate abundances and distributions in the water column, the downstream analyses proposed above to yield NCD-specific metabolic and phylogenetic information are mostly low throughput (Table 1). Extrapolating low throughput discrete measurements to large spatial and temporal scales would require, on top of a sufficiently representative sampling, knowledge on aggregate size spectra and spatiotemporal distribution (Boyd et al., 2019; Giering et al., 2020). The advent of automated aggregate counting and imaging methods (Stemmann et al., 2012; Giering et al., 2020; Karlusich et al., 2021) will likely improve the accuracy of spatiotemporal scale extrapolations in the future.
Epilog: Heterotrophic Diazotrophs Associated With Aggregates
We argue that aggregates act as dynamic loci suitable for N2 fixation by NCDs in aquatic ecosystems. Molecular analyses and microscopical identification have shown that material collected in large size fractions and sediment trap material harbor clusters of NCDs. However, the phylogeny, the specific N2 fixation rates of NCDs on aggregates and their contribution to nitrogen cycling remain largely unquantified. It is, therefore, important to develop dedicated methods and approaches capable of isolating NCD-specific N2 fixation rates and to identify their phylogeny. Our recommendation to the scientific community is to (1) develop cell-specific staining methods combined with 15N2 labeling, (2) consider the 3D architecture of single aggregates, and (3) account for their heterogeneous spatiotemporal distribution in aquatic ecosystems. Advances in automated particle characterization and counting should increase the throughput of these methods in the future. These recommendations will inspire future research to unveil the ecology and quantitative relevance of aggregate-associated NCDs in marine as well as freshwater environments.
Author Contributions
LR, ER, MB, and EB-Z wrote the manuscript. UP, H-PG, DB, IK, and ME commented on the final version of the manuscript. All authors contributed to the article and approved the submitted version.
Funding
MB and LR were supported by the BNP Paribas Foundation for Climate and Diversity grant “NOTION.” LR was supported by the Danish Council for Independent Research (6108-00013B). EB-Z was supported by the Israeli Science Foundation (grant number 944\21). H-PG was supported by the German Science Foundation (GR1540/28-1 and 37-1).
Conflict of Interest
The authors declare that the research was conducted in the absence of any commercial or financial relationships that could be construed as a potential conflict of interest.
Publisher’s Note
All claims expressed in this article are solely those of the authors and do not necessarily represent those of their affiliated organizations, or those of the publisher, the editors and the reviewers. Any product that may be evaluated in this article, or claim that may be made by its manufacturer, is not guaranteed or endorsed by the publisher.
References
Agawin, N. S. R., Benavides, M., Busquets, A., Ferriol, P., Stal, L. J., and Arístegui, J. (2014). Dominance of unicellular cyanobacteria in the diazotrophic community in the Atlantic Ocean. Limnol. Oceanogr. 59, 623–637. doi: 10.4319/lo.2014.59.2.0623
Alldredge, A. L., and Cohen, Y. (1987). Can microscale chemical patches persist in the sea? Microelectrode study of marine snow, fecal pellets. Science 235, 689–691. doi: 10.1126/science.235.4789.689
Alldredge, A. L., and Gotschalk, C. (1988). In situ settling behavior of marine snow. Limnol. Oceanogr. 33, 339–351. doi: 10.4319/lo.1988.33.3.0339
Alldredge, A. L., and Silver, M. W. (1988). Characteristics, dynamics and significance of marine snow. Prog. Oceanogr. 20, 41–82. doi: 10.1016/0079-6611(88)90053-5
Angel, R., Panhölzl, C., Gabriel, R., Herbold, C., Wanek, W., Richter, A., et al. (2018). Application of stable-isotope labelling techniques for the detection of active diazotrophs. Environ. Microbiol. 20, 44–61. doi: 10.1111/1462-2920.13954
Arrieta, J. M., Mayol, E., Hansman, R. L., Herndl, G., Dittmar, T., and Duarte, C. M. (2015). Dilution limits dissolved organic carbon utilization in the deep ocean. Sciencexpress 348:331. doi: 10.1126/science.1258955
Azam, F. (1998). Microbial control of ocean carbon flux: the plot thickens. Science 280, 694–696. doi: 10.1126/science.280.5364.694
Azam, F., and Long, R. A. (2001). Sea snow microcosms. Nature 414(6863), 495–498. doi: 10.1038/35107174
Bar-Zeev, E., and Eyal, R. (2015). Microbial metabolism of transparent exopolymer particles during the summer months along a eutrophic estuary system. Front. Microbiol. 6:403. doi: 10.3389/fmicb.2015.00403
Baumas, C. M. J., Le Moigne, F. A. C., Garel, M., Bhairy, N., Guasco, S., Riou, V., et al. (2021). Mesopelagic microbial carbon production correlates with diversity across different marine particle fractions. Front. Microbiol. 6:403. doi: 10.3389/fmicb.2015.00403
Benavides, M., Bonnet, S., Berman-frank, I., and Riemann, L. (2018a). Deep into oceanic N2 fixation. Front. Mar. Sci. 5:108. doi: 10.3389/fmars.2018.00108
Benavides, M., Martias, C., Elifantz, H., Berman-Frank, I., Dupouy, C., and Bonnet, S. (2018b). Dissolved organic matter influences N2 fixation in the new Caledonian lagoon (Western tropical South Pacific). Front. Mar. Sci. 5:89. doi: 10.3389/fmars.2018.00089
Benavides, M., Moisander, P. H., Berthelot, H., Dittmar, T., Grosso, O., and Bonnet, S. (2015). Mesopelagic N2 fixation related to organic matter composition in the Solomon and Bismarck seas (Southwest Pacific). PLoS One 10:e0143775, 26659074. doi: 10.1371/journal.pone.0143775
Benavides, M., Moisander, P. H., Daley, M. C., Bode, A., and Arístegui, J. (2016). Longitudinal variability of diazotroph abundances in the subtropical North Atlantic Ocean. J. Plankton Res. 38, 662–672. doi: 10.1093/plankt/fbv121
Bentzon-Tilia, M., Traving, S. J., Mantikci, M., Knudsen-Leerbeck, H., Hansen, J. L. S., Markager, S., et al. (2015). Significant N2 fixation by heterotrophs, photoheterotrophs and heterocystous cyanobacteria in two temperate estuaries. ISME J. 9, 273–285. doi: 10.1038/ismej.2014.119
Berman-Frank, I., Cullen, J. T., Shaked, Y., Sherrell, R. M., and Falkowski, P. G. (2001). Iron availability, cellular iron quotas, and nitrogen fixation in Trichodesmium. Limnol. Oceanogr. 46, 1249–1260. doi: 10.4319/lo.2001.46.6.1249
Bianchi, D., Weber, T. S., Kiko, R., and Deutsch, C. (2018). Global niche of marine anaerobic metabolisms expanded by particle microenvironments. Nat. Geosci. 11, 263–268. doi: 10.1038/s41561-018-0081-0
Biegala, I. C., and Raimbault, P. (2008). High abundance of diazotrophic picocyanobacteria (<3 μm) in a Southwest Pacific coral lagoon. Aquat. Microb. Ecol. 51, 45–53. doi: 10.3354/ame01185
Boeuf, D., Edwards, B. R., Eppley, J. M., Hu, S. K., Poff, K. E., Romano, A. E., et al. (2019). Biological composition and microbial dynamics of sinking particulate organic matter at abyssal depths in the oligotrophic open ocean. Proc. Natl. Acad. Sci. U. S. A. 116, 11824–11832. doi: 10.1073/pnas.1903080116
Bombar, D., Paerl, R. W., and Riemann, L. (2016). Marine non-Cyanobacterial diazotrophs: moving beyond molecular detection. Trends Microbiol. 24, 916–927. doi: 10.1016/j.tim.2016.07.002
Boyd, P. W., Claustre, H., Levy, M., Siegel, D. A., and Weber, T. (2019). Multi-faceted particle pumps drive carbon sequestration in the ocean. Nature 568, 327–335. doi: 10.1038/s41586-019-1098-2
Braun, S. T., Proctor, L. M., Zani, S., Mellon, M. T., and Zehr, J. P. (1999). Molecular evidence for zooplankton-associated nitrogen-fixing anaerobes based on amplification of the nifH gene. FEMS Microbiol. Ecol. 28, 273–279. doi: 10.1111/j.1574-6941.1999.tb00582.x
Briggs, N., Dall’Olmo, G., and Claustre, H. (2020). Major role of particle fragmentation in regulating biological sequestration of CO2 by the oceans. Science 367, 791–793. doi: 10.1126/science.aay1790
Cavender-Bares, K. K., Karl, D. M., and Chisholm, S. W. (2001). Nutrient gradients in the western North Atlantic Ocean: relationship to microbial community structure and comparison to patterns in the Pacific Ocean. Deep-Sea Res. I Oceanogr. Res. Pap. 48, 2373–2395. doi: 10.1016/S0967-0637(01)00027-9
Chakraborty, S., Andersen, K. H., Visser, A. W., Inomura, K., Follows, M. J., and Riemann, L. (2021). Quantifying nitrogen fixation by heterotrophic bacteria in sinking marine particles. Nat. Commun. 12:4085. doi: 10.1038/s41467-021-23875-6
Cornejo-Castillo, F. M., and Zehr, J. P. (2020). Intriguing size distribution of the uncultured and globally widespread marine non-cyanobacterial diazotroph gamma-A. ISME J. 15, 124–128. doi: 10.1038/s41396-020-00765-1
Coyne, K. J., Parker, A. E., Lee, C. K., Sohm, J. A., Kalmbach, A., Gunderson, T., et al. (2020). The distribution and relative ecological roles of autotrophic and heterotrophic diazotrophs in the McMurdo dry valleys, Antarctica. FEMS Microbiol. Ecol. 96:fiaa010. doi: 10.1093/femsec/fiaa010
Dabundo, R., Lehmann, M. F., Treibergs, L., Tobias, C. R., Altabet, M. A., Moisander, P. H., et al. (2014). The contamination of commercial 15N2 gas stocks with 15N-labeled nitrate and ammonium and consequences for nitrogen fixation measurements. PLoS One 9:e110335. doi: 10.1371/journal.pone.0110335
Daly, K. L., Passow, U., Chanton, J., and Hollander, D. (2016). Assessing the impacts of oil-associated marine snow formation and sedimentation during and after the Deepwater horizon oil spill. Anthropocene 13, 18–33. doi: 10.1016/j.ancene.2016.01.006
Debeljak, P., Blain, S., Bowie, A., Merwe, P., Bayer, B., and Obernosterer, I. (2021). Homeostasis drives intense microbial trace metal processing on marine particles. Limnol. Oceanogr. 66, 3842–3855. doi: 10.1002/lno.11923
Dekas, A. E., Connon, S. A., Chadwick, G. L., Trembath-Reichert, E., and Orphan, V. J. (2016). Activity and interactions of methane seep microorganisms assessed by parallel transcription and FISH-NanoSIMS analyses. ISME J. 10, 678–692. doi: 10.1038/ismej.2015.145
del Giorgio, P. A., and Cole, J. J. (1998). Bacterial growth efficiency in natural aquatic systems. Annu. Rev. Ecol. Evol. Syst. 29, 503–541. doi: 10.1146/annurev.ecolsys.29.1.503
Delmont, T. O., Pierella Karlusich, J. J., Veseli, I., Fuessel, J., Eren, A. M., Foster, R. A., et al. (2021). Heterotrophic bacterial diazotrophs are more abundant than their cyanobacterial counterparts in metagenomes covering most of the sunlit ocean. ISME J. doi: 10.1038/s41396-021-01135-1 [Epub ahead of print]
Engel, A., Thoms, S., Riebesell, U., Rochelle-Newall, E., and Zondervan, I. (2004). Polysaccharide aggregation as a potential sink of marine dissolved organic carbon. Nature 428, 929–932. doi: 10.1038/nature02453
Farnelid, H., Andersson, A. F., Bertilsson, S., Al-Soud, W. A., Hansen, L. H., Sørensen, S., et al. (2011). Nitrogenase gene amplicons from global marine surface waters are dominated by genes of non-cyanobacteria. PLoS One 6:e19223. doi: 10.1371/journal.pone.0019223
Farnelid, H., Tarangkoon, W., Hansen, G., Hansen, P. J., and Riemann, L. (2010). Putative N2-fixing heterotrophic bacteria associated with dinoflagellate-cyanobacteria consortia in the low-nitrogen Indian Ocean. Aquat. Microb. Ecol. 61, 105–117. doi: 10.3354/ame01440
Farnelid, H., Turk-Kubo, K., Ploug, H., Ossolinski, J. E., Collins, J. R., Van Mooy, B. A. S., et al. (2018). Diverse diazotrophs are present on sinking particles in the North Pacific subtropical gyre. ISME J. 13, 170–182. doi: 10.1038/s41396-018-0259-x
Fernandez, L., Bertilsson, S., and Peura, S. (2020). Non-cyanobacterial diazotrophs dominate nitrogen-fixing communities in permafrost thaw ponds. Limnol. Oceanogr. 65, S180–S193. doi: 10.1002/lno.11243
Flintrop, C. M., Rogge, A., Miksch, S., Thiele, S., Waite, A. M., and Iversen, M. H. (2018). Embedding and slicing of intact in situ collected marine snow. Limnol. Oceanogr. Methods 16, 339–355. doi: 10.1002/lom3.10251
Geisler, E., Bogler, A., Bar-Zeev, E., and Rahav, E. (2020). Heterotrophic nitrogen fixation at the hyper-eutrophic Qishon River and estuary system. Front. Microbiol. 11:1370. doi: 10.3389/fmicb.2020.01370
Geisler, E., Bogler, A., Rahav, E., and Bar-Zeev, E. (2019). Direct detection of heterotrophic Diazotrophs associated with planktonic aggregates. Sci. Rep. 9, 1–9. doi: 10.1038/s41598-019-45505-4
Geisler, E., Rahav, E., and Bar Zeev, E. (2022). Contribution of heterotrophic Diazotrophs to N2 fixation in a Eutrophic River: free-living vs Antarctica. Front. Microbiol. 13:779820. doi: 10.3389/fmicb.2022.779820
Giering, S. L. C., Cavan, E. L., Basedow, S. L., Briggs, N., Burd, A. B., Darroch, L. J., et al. (2020). Sinking organic particles in the ocean—flux estimates from in situ optical devices. Front. Mar. Sci. 6:834. doi: 10.3389/fmars.2019.00834
Goldberg, I., Nadler, V., and Hochman, A. (1987). Mechanism of nitrogenase switch-off by oxygen. J. Bacteriol. 169, 874–879. doi: 10.1128/jb.169.2.874-879.1987
Grossart, H.-P., and Ploug, H. (2000). Bacterial production and growth efficiencies: direct measurements on riverine aggregates. Limnol. Oceanogr. 45, 436–445. doi: 10.4319/lo.2000.45.2.0436
Grossart, H. P., and Ploug, H. (2001). Microbial degradation of organic carbon and nitrogen on diatom aggregates. Limnol. Oceanogr. 46, 267–277. doi: 10.4319/lo.2001.46.2.0267
Grossart, H.-P., and Simon, M. (1993). Limnetic macroscopic organic aggregates (lake snow): occurrence, characteristics, and microbial dynamics in Lake Constance. Limnol. Oceanogr. 38, 532–546. doi: 10.4319/lo.1993.38.3.0532
Guerinot, M. L., and Colwell, R. R. (1985). Enumeration, isolation, and characterization of N2-fixing bacteria from seawater. Appl. Environ. Microbiol. 50, 350–355. doi: 10.1128/aem.50.2.350-355.1985
Hallstrøm, S., Benavides, M., Salamon, E. R., Evans, C. W., Potts, L. J., Granger, J., et al. (2021). Pelagic N 2 fixation dominated by sediment diazotrophic communities in a shallow temperate estuary. Limnol. Oceanogr. 67, 364–378. doi: 10.1002/lno.11997
Halm, H., Lam, P., Ferdelman, T. G., Lavik, G., Dittmar, T., Laroche, J., et al. (2011). Heterotrophic organisms dominate nitrogen fixation in the South Pacific gyre. ISME J. 6, 1238–1249. doi: 10.1038/ismej.2011.182
Hamersley, M. R., Turk, K. A., Leinweber, A., Gruber, N., Zehr, J. P., Gunderson, T., et al. (2011). Nitrogen fixation within the water column associated with two hypoxic basins in the Southern California bight. Aquat. Microb. Ecol. 63, 193–205. doi: 10.3354/ame01494
Harding, K. J. (2021). Insights Into marine unicellular Cyanobacterial and non-Cyanobacterial diazotrophs through single-cell analyses. Available at: https://escholarship.org/content/qt9886g5gm/qt9886g5gm.pdf (Accessed March 21, 2022).
Harding, K., Turk-Kubo, K. A., Sipler, R. E., Mills, M. M., Bronk, D. A., and Zehr, J. P. (2018). Symbiotic unicellular cyanobacteria fix nitrogen in the Arctic Ocean. Proc. Natl. Acad. Sci. 115, 13371–13375. doi: 10.1073/pnas.1813658115
Jackson, G. A., and Burd, A. B. (1998). Aggregation in the marine environment. Environ. Sci. Technol. 32, 2805–2814. doi: 10.1021/es980251w
Jiang, H., Kilburn, M. R., Decelle, J., and Musat, N. (2016). NanoSIMS chemical imaging combined with correlative microscopy for biological sample analysis. Curr. Opin. Biotechnol. 41, 130–135. doi: 10.1016/j.copbio.2016.06.006
Karl, D., Michaels, A., Bergman, B., Capone, D., Carpenter, E., Letelier, R., et al. (2002). Dinitrogen fixation in the World’s oceans. Biogeochemistry 57-58, 47–98. doi: 10.1023/A:1015798105851
Karlusich, J. J. P., Pelletier, E., Lombard, F., Carsique, M., Dvorak, E., Colin, S., et al. (2021). Global distribution patterns of marine nitrogen-fixers by imaging and molecular methods. Nat. Commun. 12, 1–18. doi: 10.1038/s41467-021-24299-y
Klawonn, I., Bonaglia, S., Brüchert, V., and Ploug, H. (2015). Aerobic and anaerobic nitrogen transformation processes in N2-fixing cyanobacterial aggregates. ISME J. 9, 1456–1466. doi: 10.1038/ismej.2014.232
Klawonn, I., Eichner, M. J., Wilson, S. T., Moradi, N., Thamdrup, B., Kümmel, S., et al. (2019). Distinct nitrogen cycling and steep chemical gradients in Trichodesmium colonies. ISME J. 14, 399–412. doi: 10.1038/s41396-019-0514-9
Knapp, A. N. (2012). The sensitivity of marine N2 fixation to dissolved inorganic nitrogen. Front. Microbiol. 3:374. doi: 10.3389/fmicb.2012.00374
Kubota, K., Morono, Y., Ito, M., Terada, T., Itezono, S., Harada, H., et al. (2014). Gold-ISH: A nano-size gold particle-based phylogenetic identification compatible with NanoSIMS. Syst. Appl. Microbiol. 37, 261–266. doi: 10.1016/j.syapm.2014.02.003
Langlois, R., Großkopf, T., Mills, M., Takeda, S., and LaRoche, J. (2015). Widespread distribution and expression of gamma A (UMB), an uncultured, diazotrophic, γ-proteobacterial nifH phylotype. PLoS One 10:e0128912. doi: 10.1371/journal.pone.0128912
Loussert-Fonta, C., Toullec, G., Paraecattil, A. A., Jeangros, Q., Krueger, T., Escrig, S., et al. (2020). Correlation of fluorescence microscopy, electron microscopy, and NanoSIMS stable isotope imaging on a single tissue section. Commun. Biol. 3:362. doi: 10.1038/s42003-020-1095-x
Marcarelli, A. M., Fulweiler, R. W., and Scott, J. T. (2022). Nitrogen fixation: a poorly understood process along the freshwater-marine continuum. Limnol. Oceanogr. Lett. 7, 1–10. doi: 10.1002/lol2.10220
Marino, R., Howarth, R. W., Chan, F., Cole, J. J., and Likens, G. E. (2003). “Sulfate inhibition of molybdenum-dependent nitrogen fixation by planktonic cyanobacteria under sea water conditions: a non-reversible effect,” in Aquatic Biodiversity: A Celebratory Volume in Honour of Henri J. Dumont. ed. K. Martens (Dordrecht: Springer Netherlands), 277–293.
Martínez-Pérez, C., Mohr, W., Schwedt, A., Dürschlag, J., Callbeck, C. M., Schunck, H., et al. (2018). Metabolic versatility of a novel N2-fixing Alphaproteobacterium isolated from a marine oxygen minimum zone. Environ. Microbiol. 20, 755–768. doi: 10.1111/1462-2920.14008
McDonnell, A. M. P., Lam, P. J., Lamborg, C. H., Buesseler, K. O., Sanders, R., Riley, J. S., et al. (2015). The oceanographic toolbox for the collection of sinking and suspended marine particles. Prog. Oceanogr. 133, 17–31. doi: 10.1016/j.pocean.2015.01.007
Messer, L. F., Brown, M. V., Van Ruth, P. D., Doubell, M., and Seymour, J. R. (2021). Temperate southern Australian coastal waters are characterised by surprisingly high rates of nitrogen fixation and diversity of diazotrophs. PeerJ 9:e10809. doi: 10.7717/peerj.10809
Mestre, M., Borrull, E., Sala, M., and Gasol, J. M. (2017). Patterns of bacterial diversity in the marine planktonic particulate matter continuum. ISME J. 11, 999–1010. doi: 10.1038/ismej.2016.166
Meyer, N. R., Fortney, J. L., and Dekas, A. E. (2020). NanoSIMS sample preparation decreases isotope enrichment: magnitude, variability and implications for single-cell rates of microbial activity. Environ. Microbiol. 23, 81–98. doi: 10.1111/1462-2920.15264
Mise, K., Masuda, Y., Senoo, K., and Itoh, H. (2021). Undervalued pseudo-nifHSequences in public databases distort metagenomic insights into biological nitrogen fixers. Comput. Biol. 6, e00785–e00721. doi: 10.1128/msphere.00785-21
Mohr, W., Grosskopf, T., Wallace, D. W. R., and LaRoche, J. (2010). Methodological underestimation of oceanic nitrogen fixation rates. PLoS One 5:e12583. doi: 10.1371/journal.pone.0012583
Moisander, P. H., Benavides, M., Bonnet, S., Berman-Frank, I., White, A. E., and Riemann, L. (2017). Chasing after non-cyanobacterial nitrogen fixation in marine pelagic environments. Front. Microbiol. 8:1736. doi: 10.3389/fmicb.2017.01736
Moisander, P. H., Serros, T., Paerl, R. W., Beinart, R. A., and Zehr, J. P. (2014). Gammaproteobacterial diazotrophs and nifH gene expression in surface waters of the South Pacific Ocean. ISME J. 8, 1962–1973. doi: 10.1038/ismej.2014.49
Moraru, C., Lam, P., Fuchs, B. M., Kuypers, M. M. M., and Amann, R. (2010). GeneFISH - an in situ technique for linking gene presence and cell identity in environmental microorganisms. Environ. Microbiol. 12, 3057–3073. doi: 10.1111/j.1462-2920.2010.02281.x
Mulholland, M. R., Bernhardt, P. W., Blanco-Garcia, J. L., Mannino, A., Hyde, K., Mondragon, E., et al. (2012). Rates of dinitrogen fixation and the abundance of diazotrophs in north American coastal waters between Cape Hatteras and Georges Bank. Limnol. Oceanogr. 57, 1067–1083. doi: 10.4319/lo.2012.57.4.1067
Musat, N., Foster, R., Vagner, T., Adam, B., and Kuypers, M. M. M. (2012). Detecting metabolic activities in single cells, with emphasis on nanoSIMS. FEMS Microbiol. Rev. 36, 486–511. doi: 10.1111/j.1574-6976.2011.00303.x
Musat, N., Stryhanyuk, H., Bombach, P., Adrian, L., Audinot, J.-N., and Richnow, H. H. (2014). The effect of FISH and CARD-FISH on the isotopic composition of 13 C- and 15 N-labeled pseudomonas putida cells measured by nanoSIMS. Syst. Appl. Microbiol. 37, 267–276. doi: 10.1016/j.syapm.2014.02.002
Paerl, H. W. (1985). Microzone formation: its role in the enhancement of aquatic N2 fixation. Limnol. Oceanogr. 30, 1246–1252. doi: 10.4319/lo.1985.30.6.1246
Paerl, R. W., Hansen, T. N. G., Henriksen, N. N. S. E., Olesen, A. K., and Riemann, L. (2018). N-fixation and related O2 constraints on model marine diazotroph pseudomonas stutzeri BAL361. Aquat. Microb. Ecol. 81, 125–136. doi: 10.3354/ame01867
Paerl, H. W., and Prufert, L. E. (1987). Oxygen-poor microzones as potential sites of microbial N2 fixation in nitrogen-depleted aerobic marine waters. Appl. Environ. Microbiol. 53, 1078–1087. doi: 10.1128/aem.53.5.1078-1087.1987
Pedersen, J. N., Bombar, D., Paerl, R. W., and Riemann, L. (2018). Diazotrophs and N2-fixation associated with particles in coastal estuarine waters. Front. Microbiol. 9:2759. doi: 10.3389/fmicb.2018.02759
Piontek, J., Händel, N., Langer, G., Wohlers, J., Riebesell, U., and Engel, A. (2009). Effects of rising temperature on the formation and microbial degradation of marine diatom aggregates. Aquat. Microb. Ecol. 54, 305–318. doi: 10.3354/ame01273
Planquette, H., and Sherrell, R. M. (2012). Sampling for particulate trace element determination using water sampling bottles: methodology and comparison to in situ pumps. Limnol. Oceanogr. Methods 10, 367–388. doi: 10.4319/lom.2012.10.367
Ploug, H. (2001). Small-scale oxygen fluxes and remineralization in sinking aggregates. Limnol. Oceanogr. 46, 1624–1631. doi: 10.4319/lo.2001.46.7.1624
Ploug, H., and Grossart, H.-P. (2000). Bacterial growth and grazing on diatom aggregates: respiratory carbon turnover as a function of aggregate size and sinking velocity. Limnol. Oceanogr. 45, 1467–1475. doi: 10.4319/lo.2000.45.7.1467
Ploug, H., Kuhl, M., Buchholz-Cleven, B., and Jorgensen, B. B. (1997). Anoxic aggregates-an ephermeral phenomenon in the pelagic environment? Aquat. Microb. Ecol. 13, 285–294. doi: 10.3354/ame013285
Proctor, L. M. (1997). Nitrogen-fixing, photosynthetic, anaerobic bacteria associated with pelagic copepods. Aquat. Microb. Ecol. 12, 105–113. doi: 10.3354/ame012105
Puigcorbé, V., Ruiz-González, C., Masqué, P., and Gasol, J. M. (2020). Sampling device-dependence of prokaryotic community structure on marine particles: higher diversity recovered by in situ pumps Than by oceanographic bottles. Front. Microbiol. 11:1645. doi: 10.3389/fmicb.2020.01645
Rahav, E., Bar-Zeev, E., Ohayon, S., Elifantz, H., Belkin, N., Herut, B., et al. (2013). Dinitrogen fixation in aphotic oxygenated marine environments. Front. Microbiol. 4:227. doi: 10.3389/fmicb.2013.00227
Rahav, E., Giannetto, M. J., and Bar-Zeev, E. (2016). Contribution of mono and polysaccharides to heterotrophic N2 fixation at the eastern Mediterranean coastline. Sci. Rep. 6:27858. doi: 10.1038/srep27858
Rahav, E., Herut, B., Mulholland, M. R., Belkin, N., Elifantz, H., and Berman-Frank, I. (2015). Heterotrophic and autotrophic contribution to dinitrogen fixation in the Gulf of Aqaba. Mar. Ecol. Prog. Ser. 522, 67–77. doi: 10.3354/meps11143
Riemann, L., Farnelid, H., and Steward, G. F. (2010). Nitrogenase genes in non-cyanobacterial plankton: prevalence, diversity and regulation in marine waters. Aquat. Microb. Ecol. 61, 235–247. doi: 10.3354/ame01431
Rogge, A., Flintrop, C. M., Iversen, M. H., Salter, I., Fong, A. A., Vogts, A., et al. (2018). Hard and soft plastic resin embedding for single-cell element uptake investigations of marine-snow-associated microorganisms using nano-scale secondary ion mass spectrometry. Limnol. Oceanogr. Methods 16, 484–503. doi: 10.1002/lom3.10261
Santinelli, C. (2015). “Chapter 13 – DOC in the Mediterranean Sea,” in Biogeochemistry of Marine Dissolved Organic Matter. eds. D. A. Hansell and C. A. Carlson 2nd Edn. (Boston: Academic Press), 579–608.
Scavotto, R. E., Dziallas, C., Bentzon-Tilia, M., Riemann, L., and Moisander, P. H. (2015). Nitrogen-fixing bacteria associated with copepods in coastal waters of the North Atlantic Ocean. Environ. Microbiol. 17, 3754–3765. doi: 10.1111/1462-2920.12777
Schneider, B., Schlitzer, R., Fischer, G., and Nöthig, E.-M. (2003). Depth-dependent elemental compositions of particulate organic matter (POM) in the ocean. Global Biogeochem. Cycles 17:1032 doi: 10.1029/2002GB001871
Simon, M., Grossart, H., Schweitzer, B., and Ploug, H. (2002). Microbial ecology of organic aggregates in aquatic ecosystems. Aquat. Microb. Ecol. 28, 175–211. doi: 10.3354/ame028175
Singh, A., Bach, L. T., Löscher, C. R., Paul, A. J., Ojha, N., and Riebesell, U. (2021). Impact of increasing carbon dioxide on dinitrogen and carbon fixation rates under oligotrophic conditions and simulated upwelling. Limnol. Oceanogr. 66, 2855–2867. doi: 10.1002/lno.11795
Smith, D. C., Simon, M., Alldredge, A. L., and Azam, F. (1992). Intense hydrolytic enzyme activity on marine aggregates and implications for rapid particle dissolution. Nature 359, 139–142. doi: 10.1038/359139a0
Sohm, J. A., Webb, E. A., and Capone, D. G. (2011). Emerging patterns of marine nitrogen fixation. Nat. Rev. Microbiol. 9, 499–508. doi: 10.1038/nrmicro2594
Sprent, J. I., and Sprent, P. (eds.) (1990). Nitrogen Fixing Organisms Pure and Applied Aspects. Chapman and Hall: Springer Dordrecht.
Stemmann, L., Picheral, M., Guidi, L., Lombard, F., Prejger, F., Claustre, H., et al. (2012). “Assessing the Spatial and Temporal Distributions of Zooplankton and Marine Particles using the Underwater Vision Profiler,” in Sensors for Ecology: Towards Integrated Knowledge of Ecosystems. eds. J.-F. Le Gailliard, J.-M. Guarini, and F. Gaill Plouzane (France: Centre National de la recherche scientifique (CNRS) Institut Écologie et Environnement (INEE)), 119–137.
Thompson, A. W., Foster, R. A., Krupke, A., Carter, B. J., Musat, N., Vaulot, D., et al. (2012). Unicellular cyanobacterium symbiotic with a single-celled eukaryotic alga. Science 337, 1546–1550. doi: 10.1126/science.1222700
Turley, C. M., and Mackie, P. J. (1994). Biogeochemical significance of attached and free-living bacteria and the flux of particles in the NE Atlantic Ocean. Mar. Ecol. Prog. Ser. 115, 191–203. doi: 10.3354/meps115191
Waite, A. M., Safi, K. A., Hall, J. A., and Nodder, S. D. (2000). Mass sedimentation of picoplankton embedded in organic aggregates. Limnol. Oceanogr. 45, 87–97. doi: 10.4319/lo.2000.45.1.0087
Walcutt, N. L., Knörlein, B., Cetinić, I., Ljubesic, Z., Bosak, S., Sgouros, T., et al. (2020). Assessment of holographic microscopy for quantifying marine particle size and concentration. Limnol. Oceanogr. Methods 18, 516–530. doi: 10.1002/lom3.10379
White, A. E., Granger, J., Selden, C., Gradoville, M. R., Potts, L., Bourbonnais, A., et al. (2020). A critical review of the 15N2 tracer method to measure diazotrophic production in pelagic ecosystems. Limnol. Oceanogr. Methods 18, 129–147. doi: 10.1002/lom3.10353
Wright, J. J., Konwar, K. M., and Hallam, S. J. (2012). Microbial ecology of expanding oxygen minimum zones. Nat. Rev. Microbiol. 10, 381–394. doi: 10.1038/nrmicro2778
Zehr, J. P. (2011). Nitrogen fixation by marine cyanobacteria. Trends Microbiol. 19, 162–173. doi: 10.1016/j.tim.2010.12.004
Keywords: aggregates, nitrogen fixation, heterotrophic bacteria, marine, aquatic, NCDs
Citation: Riemann L, Rahav E, Passow U, Grossart H-P, de Beer D, Klawonn I, Eichner M, Benavides M and Bar-Zeev E (2022) Planktonic Aggregates as Hotspots for Heterotrophic Diazotrophy: The Plot Thickens. Front. Microbiol. 13:875050. doi: 10.3389/fmicb.2022.875050
Edited by:
Francesco Pomati, Swiss Federal Institute of Aquatic Science and Technology, SwitzerlandReviewed by:
Barbara Bayer, University of California, Santa Barbara, United StatesCopyright © 2022 Riemann, Rahav, Passow, Grossart, de Beer, Klawonn, Eichner, Benavides and Bar-Zeev. This is an open-access article distributed under the terms of the Creative Commons Attribution License (CC BY). The use, distribution or reproduction in other forums is permitted, provided the original author(s) and the copyright owner(s) are credited and that the original publication in this journal is cited, in accordance with accepted academic practice. No use, distribution or reproduction is permitted which does not comply with these terms.
*Correspondence: Lasse Riemann, bHJpZW1hbm5AYmlvLmt1LmRr; Eyal Rahav, ZXlhbC5yYWhhdkBvY2Vhbi5vcmcuaWw=; Mar Benavides, bWFyLmJlbmF2aWRlc0BpcmQuZnI=; Edo Bar-Zeev, YmFyemVldmVAYmd1LmFjLmls
†ORCID: Hans-Peter Grossart, orcid.org/0000-0002-9141-0325