- 1Food Microbiology, Wageningen University, Wageningen, Netherlands
- 2National Institute for Public Health and the Environment, Bilthoven, Netherlands
- 3Faculty of Veterinary Medicine, Department of Infectious Diseases and Immunology, Utrecht University, Utrecht, Netherlands
- 4WHO Collaborating Center for Campylobacter/OIE Reference Laboratory for Campylobacteriosis, Utrecht, Netherlands
Campylobacter jejuni and Campylobacter coli were previously considered asaccharolytic, but are now known to possess specific saccharide metabolization pathways, including L-fucose. To investigate the influence of the L-fucose utilization cluster on Campylobacter growth, survival and metabolism, we performed comparative genotyping and phenotyping of the C. jejuni reference isolate NCTC11168 (human isolate), C. jejuni Ca1352 (chicken meat isolate), C. jejuni Ca2426 (sheep manure isolate), and C. coli Ca0121 (pig manure isolate), that all possess the L-fucose utilization cluster. All isolates showed enhanced survival and prolonged spiral cell morphology in aging cultures up to day seven in L-fucose-enriched MEMα medium (MEMαF) compared to MEMα. HPLC analysis indicated L-fucose utilization linked to acetate, lactate, pyruvate and succinate production, confirming the activation of the L-fucose pathway in these isolates and its impact on general metabolism. Highest consumption of L-fucose by C. coli Ca0121 is conceivably linked to its enhanced growth performance up to day 7, reaching 9.3 log CFU/ml compared to approximately 8.3 log CFU/ml for the C. jejuni isolates. Genetic analysis of the respective L-fucose clusters revealed several differences, including a 1 bp deletion in the Cj0489 gene of C. jejuni NCTC11168, causing a frameshift in this isolate resulting in two separate genes, Cj0489 and Cj0490, while no apparent phenotype could be linked to the presumed frameshift in this isolate. Additionally, we found that the L-fucose cluster of C. coli Ca0121 was most distant from C. jejuni NCTC11168, but confirmation of links to L-fucose metabolism associated phenotypic traits in C. coli versus C. jejuni isolates requires further studies.
Introduction
Campylobacter is a leading cause of gastroenteritis in humans worldwide (Man, 2011; Devleesschauwer et al., 2017). The incidence and prevalence of campylobacteriosis has increased over the past few years in both developed and developing countries (Kaakoush et al., 2015; Hofreuter, 2014; Tack et al., 2019; Špaèková et al., 2019). Most common Campylobacter species causing gastroenteritis are Campylobacter jejuni and Campylobacter coli, causing 83% and 10% of all human cases, respectively (Nachamkin et al., 2008; European Food and Safety Authority, 2019). Studies reported that C. jejuni can infect and cause diarrhea with a relatively low infection dose, generally causing symptoms like gastroenteritis with acute watery or bloody diarrhea, fever and abdominal pain (Black et al., 1988; Galanis, 2007; Hara-Kudo and Takatori, 2011; Kaakoush et al., 2015). Post-infection complications include the severe Guillain-Barré syndrome and Miller-Fisher syndrome (Rees et al., 1995). Most infections are related to incidents that have been reported due to consumption of meat products predominantly poultry, direct contact with animals and via environmental waters (Vellinga and Van Loock, 2002; Clark et al., 2003; Kuusi et al., 2004; Moore et al., 2005; Karagiannis et al., 2010; Kuhn et al., 2017; Montgomery et al., 2018; Pedati et al., 2019; Kenyon et al., 2020).
Interestingly, Campylobacter is generally recognized as being susceptible to a wide variety of environmental stresses (Solomon and Hoover, 1999; Park, 2002; Mihaljevic et al., 2007; Garénaux et al., 2008; Bui et al., 2012). However, outside the animal and human gastro-intestinal tract, Campylobacter is able to survive and has therefore a certain degree of environmental robustness which is needed to endure environmental transmission (Sulaeman et al., 2012; Turonova et al., 2015; Rodrigues et al., 2016). Nutrient availability and acquisition support Campylobacter transmission between different animal hosts and the human host (Stahl et al., 2012; Gao et al., 2017). Several studies dedicated to the characterization of substrate utilization in Campylobacter spp. showed an important role for citric acid cycle intermediates, amino acids and peptides in supporting growth (Parsons, 1984; Hofreuter, 2014; Vorwerk et al., 2014). Preferred amino acids used by C. jejuni include serine, aspartate, asparagine, and glutamate (Hofreuter et al., 2008; Wright et al., 2009; Wagley et al., 2014). Campylobacter was previously thought to be asaccharolytic, lacking most key enzymes to metabolize sugars. However, more recently, evidence was provided that selected C. coli and C. jejuni isolates can metabolize glucose and/or L-fucose (Muraoka and Zhang, 2011; Stahl et al., 2011; Dwivedi et al., 2016; Vegge et al., 2016; van der Hooft et al., 2018; Garber et al., 2020). A systematic search for Entner–Doudoroff (ED) pathway genes encoding glucose utilization enzymes in a wide range of C. coli and C. jejuni isolates from clinical, environmental and animal sources, and in the C. jejuni/coli PubMLST database, revealed that 1.7% of the more than 6,000 available genomes encoded a complete ED pathway involved in glucose metabolism (Vegge et al., 2016). Based on additional phenotyping, it was concluded that some glucose-utilizing C. coli and C. jejuni isolates exhibit specific fitness advantages, including stationary-phase survival and biofilm production, highlighting key physiological benefits of this pathway in addition to energy conservation (Vorwerk et al., 2015; Vegge et al., 2016). Notably, comparative WGS analysis revealed that approximately 65% of the sequenced C. jejuni isolates and 73% of the sequenced C. coli isolates possess the L-fucose utilization cluster (designated Cj0480c – Cj0490 in C. jejuni NCTC11168), so called fuc + isolates. The L-fucose utilization cluster is regulated by Cj0480c and contains 2 predicted transporters encoded by Cj0484 and Cj0486 (FucP). After L-fucose transport into the cell, L-fucose is further metabolized to the end products pyruvic acid and lactic acid via the metabolic enzymes encoded by Cj0488, Cj0485 (FucX), Cj0487, Cj0482/Cj0483, Cj0481 (DapA) and Cj0489/Cj0490 (Stahl et al., 2011; Dwivedi et al., 2016; Garber et al., 2020). Both, pyruvate and lactate can be further metabolized and support growth of C. jejuni and C. coli (Thomas et al., 2011; Stahl et al., 2012).
Putative roles for L-fucose in fuc + C. jejuni isolates in growth, biofilm formation and virulence have been reported, however, no data are available on L-fucose metabolism in C. coli and its impact on growth and survival (Muraoka and Zhang, 2011; Stahl et al., 2011; Dwivedi et al., 2016). The human gastro-intestinal tract is a fucose-rich environment with fucose incorporated in glycan structures found on epithelial cells (Becker and Lowe, 2003; Pickard and Chervonsky, 2015). These fucosylated glycans can be hydrolyzed by fucosidases produced by a range of bacterial inhabitants of the gut such as Bacteroides spp. C. jejuni does not possess any obvious fucosidase homologs, however, C. jejuni can forage on L-fucose released by Bacteroides vulgatus in co-cultures with porcine mucus as a substrate (Garber et al., 2020). Furthermore, fucosylated glycans are not only commonly used as carbon source, but can also serve as adhesion sites or receptors for pathogens like Helicobacter pylori and C. jejuni (Boren et al., 1993). Previous research in Campylobacter has shown that fucose monomers and fucosylated glycans serve as chemoattractant for C. jejuni, supporting adherence to epithelial cell surfaces containing such glycans (Hugdahl et al., 1988; Day et al., 2009).
Despite the potential role of L-fucose in the intestinal ecology and infection efficacy of C. jejuni, relatively little is known about the activation of L-fucose metabolism and the impact on stationary phase survival in fuc + Campylobacter isolates. Interestingly, in human disease C. coli is less prevalent in comparison with C. jejuni, however, the L-fucose utilization cluster is more common among C. coli isolates (Dwivedi et al., 2016). A comparative analysis of the efficacy and metabolite formation following activation of L-fucose utilization clusters in C. jejuni and C. coli isolates, combined with impact on growth and survival, has not been reported up to now.
In this study we quantified L-fucose utilization and impact on metabolism of amino acids and short chain (di)carboxylic acids including acetate, lactate and succinate, and long-term culturability of three fuc + C. jejuni isolates and one fuc + C. coli isolate, and correlated this to genetic features of respective L-fucose utilization clusters of the tested Campylobacter isolates.
Materials and Methods
Bacterial Isolates and Culture Preparation
The following Campylobacter isolates were used during this study: C. jejuni NCTC11168 (reference isolate) isolated from human feces, C. jejuni Ca1352 isolated from chicken meat, C. jejuni Ca2426 isolated from sheep manure and C. coli Ca0121 isolated from pig manure. Campylobacter stock cultures were prepared using BactoTM Heart Infusion broth (Becton, Dickinson and Company, Vianen, the Netherlands) and were grown for 24 h at 41.5°C in microaerobic conditions (5% O2, 10% CO2, 85% N2) which was created using an Anoxomat WS9000 (Mart Microbiology, Drachten, the Netherlands). Glycerol stocks were prepared using 30% glycerol and 70% overnight culture and they were stored at −80°C.
Routinely, prior to growth experiments, the Campylobacter freezer stocks were streaked on Columbia Agar Base [CAB (Oxoid, Landsmeer, Netherlands)] plates supplemented with 5% (v/v) lysed horse blood (BioTrading Benelux B.V. Mijdrecht, Netherlands) and 0.5% bacteriological agar No.1 (Oxoid) for optimal recovery. The plates were incubated in anaerobic jars for 24 h at 41.5°C in microaerobic conditions. Colonies were routinely selected and grown overnight in 10 mL GibcoTM MEMα medium (Thermo Fisher Scientific, Bleiswijk, the Netherlands) (see Supplementary Table 1 for medium details) supplemented with 20 μM FeSO4 (Merck Schiphol-Rijk, the Netherlands), 10 mL MH2 broth (Merck) or 10 mL MH3 broth (Oxoid). A second overnight culture was made by diluting the Campylobacter suspension 1:100 in 10 mL fresh MEMα, MH2 or MH3 medium. The suspension was incubated at 41.5°C for 24 h in microaerobic conditions to obtain standardized working cultures for use in further experiments.
L-Fucose Growth Experiments
Prior to growth experiments, MEMα medium was supplemented with 21.0 mM L-fucose (MEMαF medium) and filter-sterilized using 0.2-μm pore sized filters. Infusion bottles were closed using a rubber stopper and aluminum cap and next they were sterilized. Sterilized 100 mL infusion bottles were filled with 45 mL filter-sterilized MEMα medium or MEMαF medium by using a syringe. Filled infusion bottles were stored at 4°C until further use.
Working cultures were decimally diluted in MEMα medium to a cell concentration of approximately 105 CFU/mL. A final dilution step was done by adding 5 mL into the infusion bottles filled with 45 mL MEMα medium or MEMαF medium, resulting in a starting cell concentration of 104 CFU/mL. Incubation of the inoculated infusion bottles was done at 37°C. Daily, approximately 4 mL sample was taken from each infusion bottle, starting at day 0. After each sampling point, the head space of infusion bottles was flushed for 2 min with microaerobic gas (5% O2, 10% CO2, 85% N2) using a home-made gas flushing device using syringes to puncture the rubber stopper.
The samples were used to determine the bacterial concentration and for microscopic analysis. The remainder of each sample was stored at −20°C for high pressure liquid chromatography (HPLC) analyses. Bacterial concentrations were determined by decimally diluting 1 mL of sample in peptone physiological salt solution (PPS, Tritium Microbiologie, Eindhoven, Netherlands), followed by surface plating on CAB plates. CAB plates were incubated in jars for 48 h at 41.5°C in microaerobic conditions. Colonies were counted and expressed in log10 CFU/mL. Each sample was microscopically analyzed using an Olympus BX 41 microscope (lens Ach 100x/1.25, Olympus Nederland, Leiderdorp, Netherlands) and pictures were captured using CellSens Imaging software (Olympus Corporation). Three biologically independent reproductions were performed per condition, i.e., MEMα medium, and MEMαF medium, on different days.
High Pressure Liquid Chromatography for Organic Acids
Samples obtained from the growth experiments were centrifugated at 13,000 g at 4°C for 5 min. Pellets were removed and the supernatant was treated for protein decontamination with Carrez A (K4FeCN)6.3H20, Merck) and B (ZnO4⋅7H20, Merck). After centrifugation, the supernatant was added to HPLC vials. Quantitative analyses were done using standards with pre-made concentrations for L-fucose, acetate, alpha-ketoglutarate, succinate, pyruvate and lactate. The HPLC was performed on an Ultimate 3000 HPLC (Dionex, Sunnyvile, United States) equipped with an RI-101 refractive index detector (Shodex, Kawasaki, Japan), an autosampler and an ion-exclusion Aminex HPX – 87H column (7.8 × 300 mm) with a guard column (Bio-Rad, Hercules, CA). As mobile phase, 5 mM H2SO4 (Merck) was used at a flow rate of 0.6mL/min. Column temperature was kept at 40°C. For each run, the injection volume was 10 μL and the run time 30 min. Chromeleon software (Thermo Fisher Scientific, Waltham, United States) was used for quantification of compound concentrations.
High Pressure Liquid Chromatography for Amino Acids
Samples obtained from the growth experimented were used in aliquots of 40 μL. These aliquots were kept on ice and were diluted with 50 μL of 0.1 M HCl (containing 250 μM Norvalin as internal standard, Merck). The samples were deproteinized by addition of 10 μL of cold 5-sulphosalicylic acid (SSA, Merck) (300 mg/ml) and centrifuged at 13,000 g at 4°C for 10 min. In order to obtain an optimal pH for derivatization (pH between 8.2 to 10.0), approximately 60 to 150 μL of 4N NaOH was added to 5 mL of the AccQTag Ultra borate buffer (Borate/NaOH buffer, Waters, Milford, United States). For derivatization 60 μL of Borate/NaOH was added to a total recovery vial. Twenty μL of the supernatant obtained after deproteinization of the plasma was added and mixed. To each of the vials 20 μL of AccQ Tag Ultra derivatization reagent (Waters) dissolved in acetonitrile was added and mixed for 10 s. Each vial was immediately capped. The vials were then heated for 10 min at 55°C. The vials were stored at −20°C prior to HPLC analysis. Quantitative analyses were done using standards with pre-made concentrations for, histidine, asparagine, serine, glutamine, arginine, glycine, aspartic acid, glutamic acid, threonine, alanine, proline, cysteine, lysine, tyrosine, methionine, and valine. HPLC was performed on an Ultimate 3000 HPLC (Dionex) equipped with an RI-101 refractive index detector (Shodex), an autosampler and an ion-exclusion Aminex HPX – 87H column (7.8 × 300 mm) with a guard column (Bio-Rad). As mobile phase, eluants A and B (Waters) was used at a flow rate of 0.7mL/min. Column temperature was kept at 55°C. For each run, the injection volume was 1 μL and the run time 17min. Chromeleon software (Thermo Fisher Scientific) was used for the determination of compound concentrations. Baseline separation was obtained for all amino acids except glutamine and arginine.
Genomic Analyses
The sequence of NCTC11168 was obtained from the public collection of genbank with accession number AL111168. The sequences of the assembled genomes of C. coli Ca0121, C. jejuni Ca1352 and C. jejuni Ca2426 were obtained via the Netherlands Food and Consumer Product Safety Authority (NVWA) and are described in (Mughini-Gras et al., 2020).
Genome alignments were performed using the online Benchling software1. Each gene was translated into an amino acid sequence which was used for further alignments. Protein interactions were analyzed with STRING2. Alignments were visualized by using the T-Coffee (Di Tommaso et al., 2011) or clinker3. Box shade figures were generated using BOX-SHADE 3.214 using the RTF_old output format.
Statistical Analyses
Differences in log10-counts observed for growth in MEMα medium and growth in MEMαF medium were statistically tested using a two-tailed Student’s t-test. P values ≤ 0.05 were considered as significant difference.
Results
Diversity in Growth Performance of Campylobacter in the Absence and Presence of L-Fucose
We investigated growth and survival of different C. jejuni host isolates and one C. coli host isolate; namely C. jejuni NCTC11168 (human stool isolate), C. jejuni Ca1352 (chicken meat isolate), C. jejuni Ca2426 (sheep manure isolate) and C. coli Ca0121 (pig manure isolate), in MEMα medium and MEMαF medium up to 7 days. At day 1 and 2, no difference was observed between growth in MEMαF medium and MEMα medium for the tested isolates and at day 2 cell concentrations reached were 8.4, 8.2, 8.3 and 8.6 log10 CFU/mL, for C. jejuni NCTC11168, Ca1352, Ca2426 and C. coli Ca0121, respectively (Figures 1A–D). However, from day 3 onwards, cell viability decreased in the absence of L-fucose and significantly lower cell counts were observed from day 3 until day 7, at which final cell counts reached 1.0, 4.4, 5.0, and 5.7 log10 CFU/mL in MEMα medium for isolates C. jejuni NCTC11168, Ca2426, Ca1352 and C. coli Ca0121, respectively. In MEMαF, cell concentrations did not decrease up to day 4 or 5, after which lower or stable cell concentrations were observed for isolate C. jejuni NCTC11168, Ca2426 and Ca1352 (Figures 1A-C). Notably, C. coli Ca0121 showed continuation of growth after day 2, reaching 9.3 log10 CFU/ml at day 7 (Figure 1D). Linking cell counts to morphology using microscopic images revealed that in MEMα medium, coccoid cells were commonly observed, increasingly over time after day 2, while in MEMαF medium, higher quantities of spiral-shaped cells were observed for all tested isolates (Supplementary Figure 1). In line with the observed increase in plate counts, isolate C. coli Ca0121 showed almost no increase in the amount of coccoid cells overtime when grown in MEMαF medium (Supplementary Figure 1).
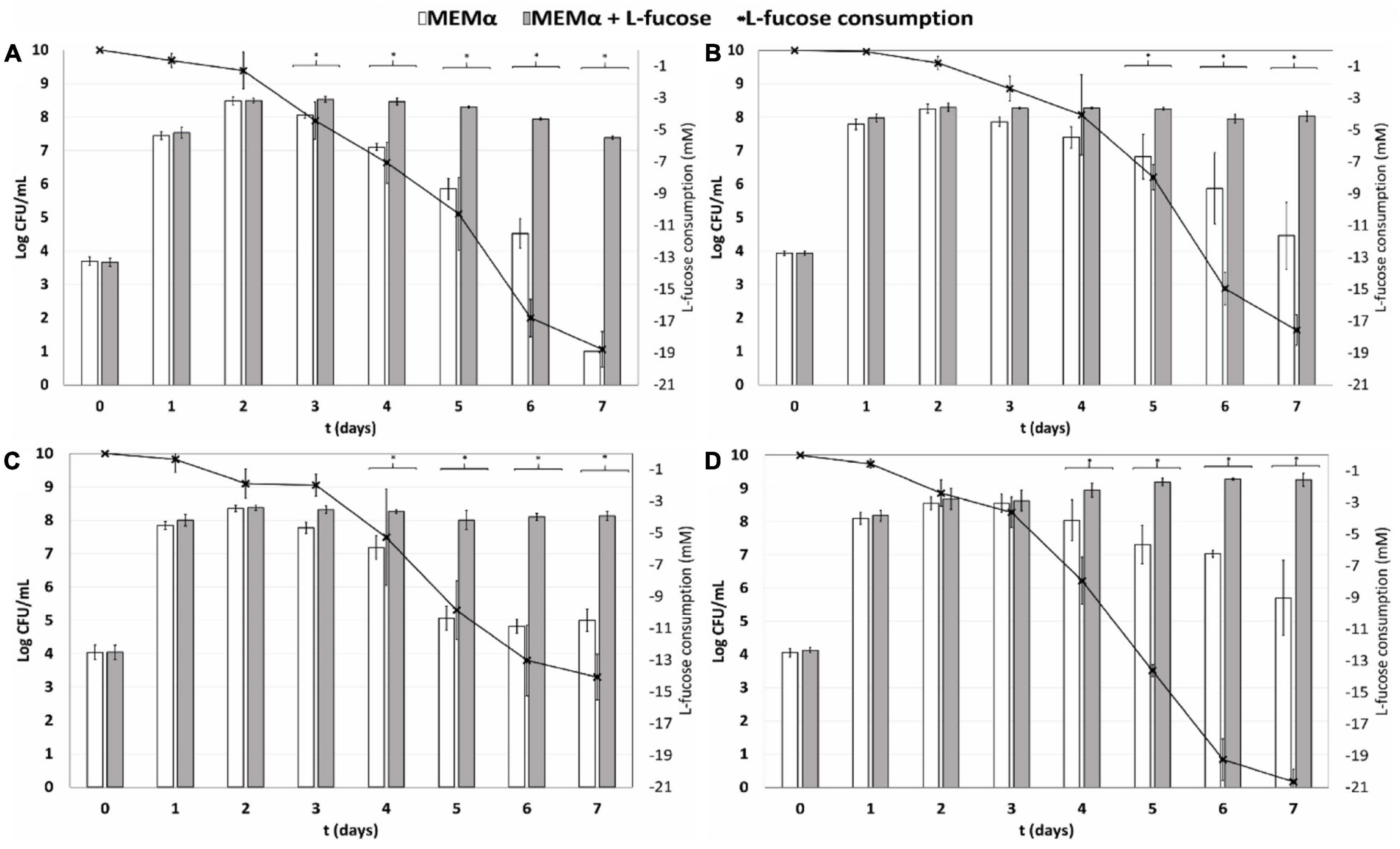
Figure 1. Quantification of planktonic growth for (A) isolate C. jejuni NCTC11168 (human stool isolate), (B) C. jejuni Ca1352 (chicken meat isolate) (C) C. jejuni Ca2426 (sheep manure isolate) and (D) C. coli Ca0121 (pig manure isolate), in MEMα medium (white bars) and MEMαF medium (gray bars). The black line shows consumption of L-fucose over time. Each value represents the average of 3 biologically independent replicates, and error bars show the standard deviation. The asterisks indicate significant (P < 0.05) differences in cell counts after incubation in MEMα medium and MEMαF medium.
HPLC analyses confirmed absence of L-fucose in MEMα medium and enabled quantitative analysis of L-fucose consumption by the tested isolates in MEMαF medium. The L-fucose concentration decreased after day 1 for all four isolates. Interestingly, the C. coli isolate showed the highest and fasted L-fucose consumption over time, which was in line with the robust growth performance of this isolate.
Metabolite Concentrations in Campylobacter jejuni and Campylobacter coli Isolates When Grown in MEMα or MEMαF Medium
We determined the impact of L-fucose on concentrations of metabolites and amino acids during growth in MEMα. Lactate production was not detected in MEMα medium after growth (Figure 2). In MEMαF medium, up to 1.1 to 1.75 mM lactate was produced by all tested isolates up to day 5 and remained rather stable afterward (Figure 2). Starting concentrations of 0.25 mM of pyruvate were found to be depleted at day 2 in MEMα medium, whereas in MEMαF medium pyruvate concentrations remained at these low levels in C. jejuni NCTC11168 and C. coli Ca0121, and increased up to 0.6 mM and 1.3 mM in C. jejuni Ca1352 and Ca2426, respectively (Figure 2). In MEMα medium low concentrations of acetate (< 1 mM) were initially produced up to day 2 by all tested isolates and consumed later on (Figure 2), indicative of the acetate switch (Wright et al., 2009). In MEMαF medium, higher amounts of acetate were produced and concentrations increased until day 7 in isolates C. jejuni NCTC11168, Ca1352 and Ca2426 acetate reaching 4.4 mM, 4.1 mM and 2.9 mM, respectively. Notably, C. coli Ca0121 produced highest levels of acetate at day 5 (6.3 mM), and the lower level at day 7 pointed to acetate consumption, pointing to a delay of the acetate switch, in line with depletion of L-fucose in the medium. We observed a slight increase in succinate concentrations up to day 3, reaching 0.25 mM with C. jejuni isolates NCTC11168, Ca1352 and Ca2426, followed by consumption of succinate in both media (Figure 2). Notably, C. coli Ca0121 produced highest levels of succinate up to day 3 in MEMα and up to day 5 in MEMαF, reaching 0.6 mM and 0.8 mM, respectively, after which succinate levels decreased in both media.
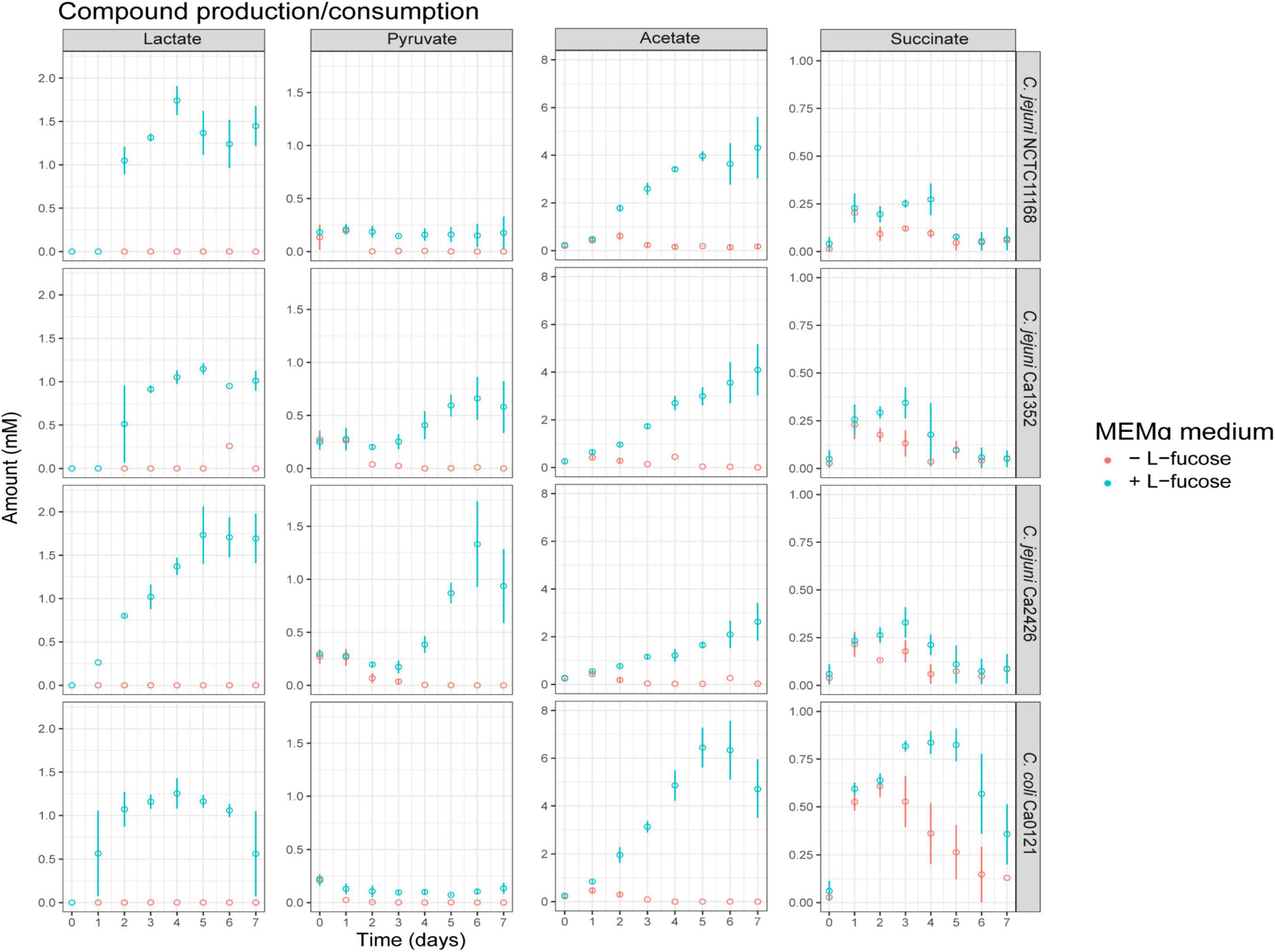
Figure 2. Compound production and consumption of selected Campylobacter isolates in MEMαF medium (blue dots) and MEMα medium (red dots) after a 7-day incubation period. Columns correspond to different compounds and each row shows results for a different isolate. Each value represents the average of three biologically independent replicates, and error bars show the standard deviation.
Analysis of amino acid metabolism showed rapid depletion of serine and aspartic acid for all tested isolates in MEMα and MEMαF (Figure 3 and Supplementary Figure 3). Glutamic acid and proline were depleted after 3 days of incubation, while asparagine decreased also but remained present at low levels (0.1-0.15 mM). Notably, a strong depletion of the sulphur-containing metabolite cystine in MEMαF medium with all C. jejuni isolates was observed in the first 2 or 3 days, whereas no significant consumption was observed in MEMα medium (Figure 3).
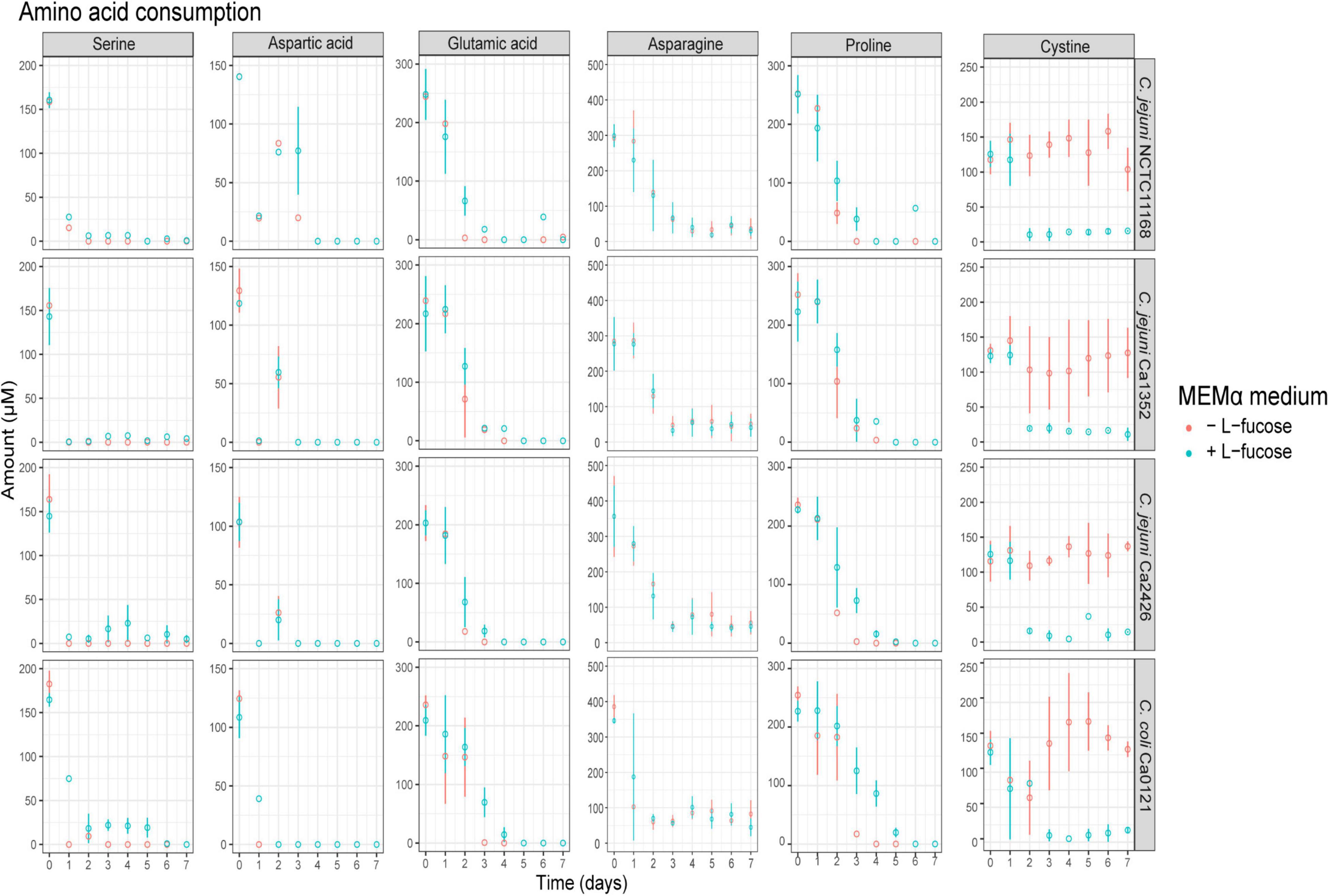
Figure 3. Amino acid production and consumption of selected Campylobacter isolates in MEMαF medium (blue dots) and MEMα medium (red dots) after 7-day incubation period. Columns correspond to different amino acids and each row shows results for a different isolate. Each value represents the average of three biologically independent replicates, and error bars show the standard deviation.
Comparative Analysis of L-Fucose Utilization Clusters in Campylobacter jejuni and Campylobacter coli Isolates
To investigate the genomic differences between the L-fucose utilization clusters of the tested isolates, we aligned each of the protein sequences (Cj0480c – Cj0490) of isolates C. jejuni Ca1352, Ca2426 and C. coli Ca0121 to the respective protein sequences of the reference isolate C. jejuni NCTC11168. The corresponding putative functions of the proteins encoded by the genes together constitute the L-fucose degradation pathway (Figure 4A). Alignments of the gene Cj0489, encoding an aldehyde dehydrogenase, confirmed a previously discovered frameshift in the reference isolate NCTC11168 (Muraoka and Zhang, 2011). However, this frameshift was not observed in C. jejuni Ca1352, Ca2426 and C. coli Ca0121 (Figure 4B). The Cj0489 gene codes for a protein of 479 AA in C. jejuni Ca1352, Ca2426 and C. coli Ca0121, while the frameshift in the C. jejuni NCTC11168 genome, resulted in a shortened Cj0489 gene, coding for a putative protein composed of 77 AA. Based on the presence of a new start codon, a second larger fragment of the Cj0489 gene, renamed Cj0490 (Muraoka and Zhang, 2011; Stahl et al., 2011), is predicted to code for a putative protein of 394 AA. The AA composition of truncated proteins encoded by Cj0489-Cj0490 of C. jejuni NCTC11168 was highly similar to the proteins encoded by the non-truncated Cj0489 gene of C. jejuni Ca1352, Ca2426 and C. coli Ca0121, which was 95%, 95% and 97%, respectively. Next, we aligned all encoded proteins of the L-fucose cluster and calculated the percent identities to the reference isolate NCTC11168 (Figure 4C and Supplementary Table 2). Only Cj0482, which encodes the altronate hydrolase/dehydratase, had 100% amino acid identity in all tested isolates. The altronate hydrolase/dehydratase encoded by Cj0483 displayed > 97% identity in all tested isolates. Clearly, the C. jejuni isolates Ca1352 and Ca2426 harbored more genes that displayed 100% protein identity with NCTC11168 than C. coli Ca0121. The L-fucose utilization cluster of C. coli Ca0121 was genetically the most distant from NCTC11168 with some translated genes having similarities as low as 84% such as the protein encoded by Cj0487.
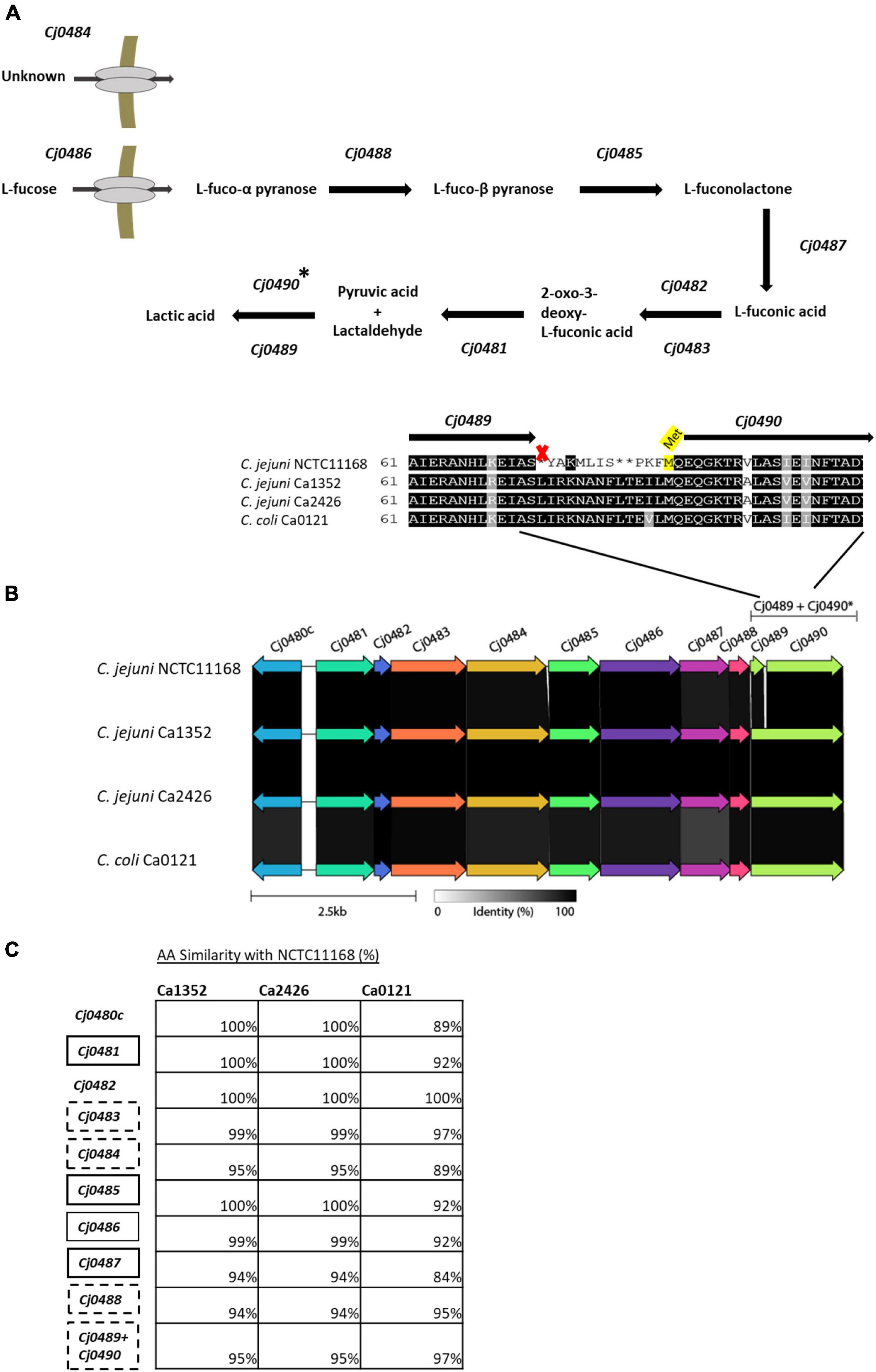
Figure 4. Genomic overview of the L-fucose utilization cluster in the four tested isolates. (A) The predicted L-fucose metabolism cluster based on previously reported research (Stahl et al., 2011; Garber et al., 2020). (B) gene alignments performed with Clinker, AA identity is displayed in gray scale. A zoom-in is shown of the AA sequence of the Cj0489-Cj0490 gene, indicating a frameshift in isolate NCTC11168, resulting in an early stop codon (marked with an X), splitting Cj0489 (77 AA) and Cj0490 (394 AA). A putative start site (Met) of Cj0490 in C. jejuni NCTC11168, is highlighted in yellow. (C) Amino acid alignments of the genes in the isolates; C. jejuni Ca1352 (chicken meat isolate), C. jejuni Ca2426 (sheep manure isolate) and C. coli Ca0121 (pig manure isolate) with isolate C. jejuni NCTC11168 as reference (full alignment in Supplementary Figure 4). The percentages indicate AA similarities between C. jejuni NCTC11168 and Ca1352, Ca2426 or C. coli Ca0121. Similarity of Cj0489-Cj0490 of isolate C. jejuni NCTC11168 is compared to Cj0489 of the other isolates. Black and black dotted - boxes indicate genes to be essential or not for L-fucose metabolism based on mutant studies (34,35,37) (no data available for Cj0482 and Cj0480c).
Notably, the proteins encoded by the L-fucose utilization cluster of C. jejuni Ca1352 and Ca2426 displayed 100% similarity with each other on amino acid level (Supplementary Table 2). Several SNPs were found between the 2 isolates, however, these did not result in single amino-acid polymorphisms.
Discussion
The L-fucose utilization cluster is present in the majority of all C. jejuni and C. coli isolates, 65% and 73%, respectively, and is predominantly found in livestock-associated Campylobacter isolates (Dwivedi et al., 2016). Comparative growth analysis of the four selected fuc + C. jejuni and C. coli isolates showed that cell counts reached up to day 2 and 3 were similar in MEMα and MEMαF. This indicates, despite the initial onset of L-fucose consumption, that the MEMα medium contains sufficient energy/carbon sources to support initial growth in the selected conditions. Impact of L-fucose on growth and survival of C. jejuni and C. coli isolates, became apparent during prolonged incubation up to day 7, concomitant with the significant increase in L-fucose consumption in this period resulting in pyruvate and lactate, that can serve as substrates for growth of Campylobacter. In line with these observations, microscopy analysis of cells in MEMαF showed high proportions of spiral shaped cells, while coccoid-shaped cells were overrepresented in samples from non-supplemented MEMα from day 3 to day 7. This points to a nutrient deficiency in the latter medium, in line with previous studies that showed starvation as a stress factor leading to a change from helical shaped to coccoid shaped cells (Hazeleger et al., 1995; Ikeda and Karlyshev, 2012; Frirdich et al., 2017). In addition, enhanced growth of the C. coli isolate was reflected in a more prominent fraction of spiral shaped cells morphology for a prolonged duration in MEMαF, pointing to a more robust phenotype for this isolate compared the tested C. jejuni isolates. Notably, the number of culturable cells determined on MEMα plates (data not shown) and on CAB plates supplemented with lysed horse blood for optimal recovery, were similar. Similar enhanced performance of the C. coli isolate was observed during growth in Mueller Hinton broths, MH2 and MH3 (Supplementary Figure 2, containing 0.2 and 0.3% beef extract, respectively. No clear effect of the measured L-fucose consumption on growth performance in MH2 and MH3 was observed for the tested C. jejuni isolates, reaching maximum counts of ∼8.0 log CFU/mL, while, C. coli Ca0121 reached highest CFU counts in both MH2 and MH3, and with added L-fucose, even ∼9.5 log CFU/mL was reached in MH3 (Supplementary Figure 2).
HPLC analyses confirmed that the majority of the preferred amino acids were depleted on day 3 in MEMα and MEMαF, in line with previous studies that suggested that amino acids are preferred substrates for growth of Campylobacter (Garber et al., 2020). Notably, cystine, the oxidized dimer form of the amino acid cysteine, was only depleted at day 2 or day 3 when L-fucose was present in the medium and fucose consumption had started, indicating that cystine depletion is linked to L-fucose metabolism, while another study concluded that sulfur-containing metabolites including cystine, result from chemical reactions (van der Hooft et al., 2018). The recent identification and characterization of a dedicated cystine transporter (Cj0025c) in C. jejuni, which is present in all our tested isolates (data not shown), next to the conceivable uptake of cystine via peptide transporters (Vorwerk et al., 2014; Man et al., 2020), may offer support for our observation that cystine depletion is linked to L-fucose metabolism in the tested C. jejuni and C. coli isolates in the current study.
Metabolite HPLC analyses of cultures grown in MEMα without and with added L-fucose demonstrated that lactate (1-1.5 mM), pyruvate (0.2-1 mM) and acetate (4-6 mM), were mainly detected when C. jejuni and C. coli strains were grown in MEMαF medium and following depletion of amino acids. Lactate and pyruvate, respective end product and intermediate of the predicted L-fucose metabolism pathway, can be further metabolized by Campylobacter, however, their accumulation in the medium has not been reported in previous studies (Mendz et al., 1997; Stahl et al., 2011; Thomas et al., 2011; Garber et al., 2020).
A schematic overview is presented that highlights the impact of L-fucose utilization on (general) metabolism in the tested C. jejuni and C. coli isolates (Figure 5). L-fucose is taken up and metabolized in 5 steps to pyruvate and lactaldehyde, with the latter compound converted in step 6 into lactate. This consumption of L-fucose results in efflux and uptake/metabolism of lactate and succinate for all tested isolates, pyruvate and acetate efflux in C. jejuni Ca1352 and Ca2426, acetate efflux and uptake/metabolism in C. jejuni NCTC11168 and C. coli Ca0121 and low pyruvate efflux in C. jejuni NCTC11168 and C. coli Ca0121, leading to, in some cases, transiently higher concentrations of lactate, pyruvate, acetate and succinate in the medium, suggesting feedback inhibition during initial phases of fucose utilization. Lastly, cystine consumption was found solely dependent on L-fucose metabolism in all tested isolates.
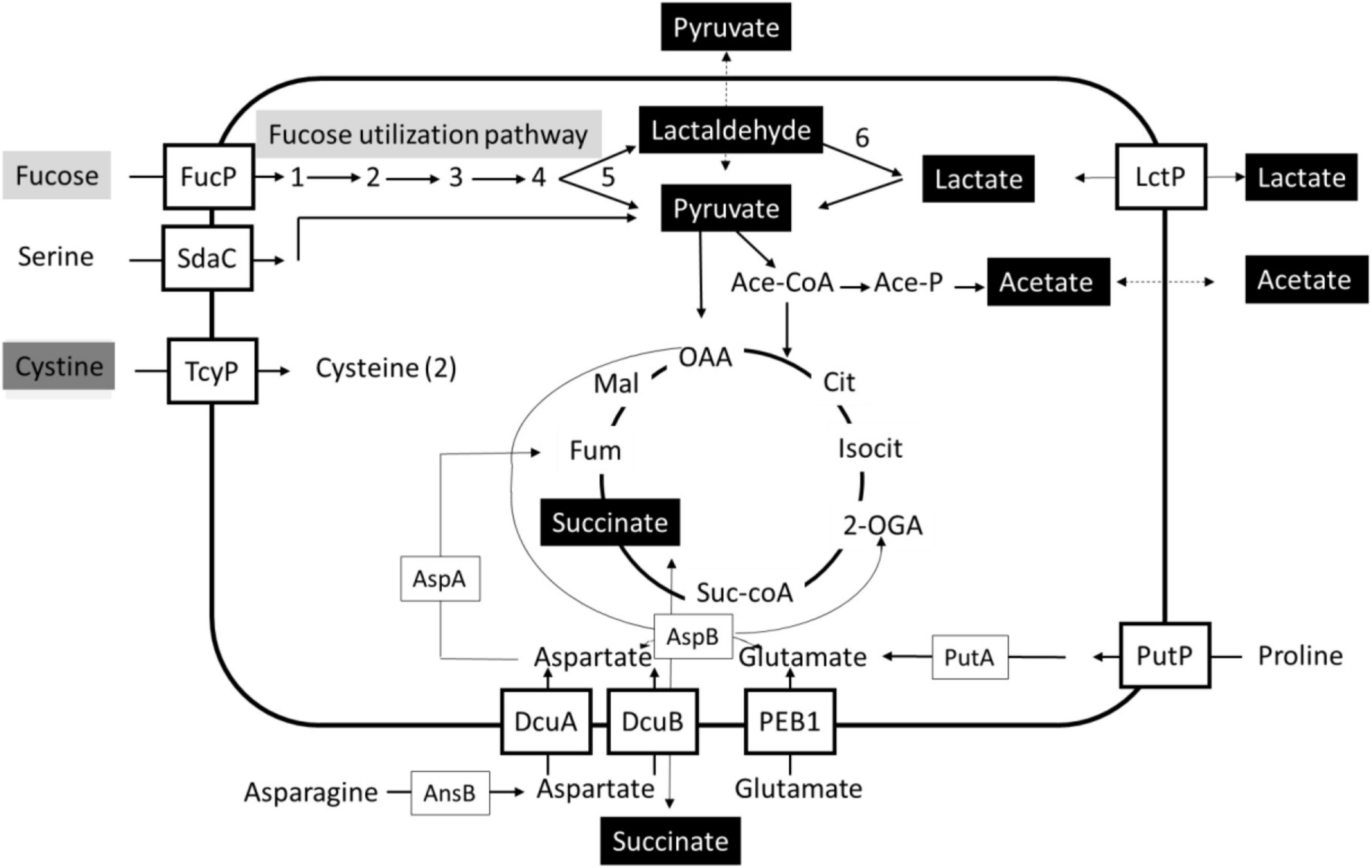
Figure 5. Schematic overview of uptake and metabolism uniquely coupled to fucose utilization in Campylobacter. Bidirectional arrows indicate efflux and uptake/metabolism of lactate and succinate for all tested isolates in MEMαF, pyruvate and acetate efflux in C. jejuni Ca1352 and Ca2426 in MEMαF, acetate efflux and uptake/metabolism in C. jejuni NCTC11168 and C. coli Ca0121 in MEMαF and succinate efflux and uptake/metabolism in all tested isolates in MEMα and in MEMαF, with slightly higher extracellular levels. The gray boxes indicate L-fucose transport and metabolism, the black boxes indicate compounds of which the production and metabolism is linked to L-fucose metabolism, and the dark grey box indicates uptake and intracellular reduction resulting in two (2) cysteines, uniquely linked to L-fucose utilization. Uptake and metabolism of serine, asparagine, aspartate, glutamate, proline and cystine with respective putative transporters are indicated. Figure adapted from Hofreuter (2014) and Guccione et al. (2017).
The L-fucose utilization cluster consists of 11 genes (Cj0480c-Cj0490) for C. jejuni NCTC11168 and 10 genes (Cj0480c-Cj0489) for C. jejuni Ca1352, Ca2426 and C. coli Ca0121 and is predicted to metabolize L-fucose into lactic acid (Stahl et al., 2011; Garber et al., 2020). Interestingly, the utilization cluster contains two sets of genes with putative overlapping functions, Cj0482 and Cj0483, and Cj0489 and Cj0490. Both Cj0482 (88 AA) and Cj0483 (389 AA), are two separated genes that are annotated as altronate hydrolases/dehydratases with similar ORFs in all tested isolates (Figure 4). STRING analyses displayed a possible N-terminus (Cj0482) and possible C-terminus (Cj0483) of the predicted altronate hydrolase. In C. jejuni, only knockout studies of Cj0483 have been performed, and this gene was found to be not essential for the metabolism of L-fucose (Stahl et al., 2011). Stahl et al. (2011) also showed, by using microarrays, that both Cj0482 and Cj0483 were upregulated in the presence of L-fucose, 1.9 log2 and 4.4 log2 fold-change respectively (Stahl et al., 2011). No knockout studies were performed with a Cj0482 deletion mutant, however, due to the observed activity of this gene we hypothesize that both genes encode enzymes that can perform transformation of L-fuconate into 2-oxo-3-deoxy-L-fuconate. Comparative analysis shows that only in C. jejuni NCTC11168, the gene Cj0489 presents a 1 bp deletion frameshift that results in an early stop, splitting Cj0489 into Cj0489 and Cj0490, both annotated as aldehyde dehydrogenase (Javed et al., 2010; Muraoka and Zhang, 2011). In C. jejuni Ca1352, C. jejuni Ca2426 and C. coli Ca0121, Cj0489 does not contain a frameshift and conceivably encodes the intact, 479 amino acid aldehyde dehydrogenase (Figure 4B). Previous studies in C. jejuni NCTC11168 showed that deletion of Cj0489 or Cj0490 did not impair growth in the presence of L-fucose (Stahl et al., 2011; Dwivedi et al., 2016; Garber et al., 2020). Our HPLC data confirmed production of lactate in MEMαF, the final product of the L-fucose utilization pathway in all tested isolates, including NCTC11168, suggesting that L-fucose metabolism is not hampered in C. jejuni NCTC11168 that contains the frameshift. A recent study performed by Pascoe et al. (2019) reported the outcome of a domestication study analyzing 23 whole genome sequenced C. jejuni NCTC11168 isolates collected from a range of research laboratories across the United Kingdom (Pascoe et al., 2019). Our analysis of the L-fucose utilization cluster in these 23 C. jejuni NCTC11168 isolates showed that the fucose utilization clusters including the frameshift were 100% identical. This points to selection pressure on maintaining this L-fucose cluster with the frameshift in Cj0489. Whether the Cj0489 and Cj0490 genes in C. jejuni NCTC11168 encode (a) functional enzyme(s), or that there is an alternative lactaldehyde dehydrogenase induced in this isolate, remains to be elucidated.
Our comparative genotyping analysis of the four L-fucose utilization clusters, revealed that the cluster of C. jejuni NCTC11168 had several additional genomic differences in comparison with C. jejuni Ca1352, Ca2426 and C. coli Ca0121. Notably, genomic comparison analyses showed that the L-fucose utilization clusters of Ca1352 and Ca2426 were 100% similar, presenting only synonymous SNPs, in line with observed similarity in phenotypic behavior of these two isolates. The L-fucose utilization cluster of C. coli Ca0121 was the most distant from the cluster of C. jejuni NCTC11168. Our results showed that, in the presence of L-fucose, C. coli Ca0121 was able to reach the highest CFU counts, maintained a spiral morphology and completely metabolized available L-fucose in the tested conditions. These results suggest a possible link between the observed C. coli Ca0121 phenotype and changes in amino acid composition of enzymes in the L-fucose utilization cluster that could point to altered enzyme levels and/or functionality. However, it should be noted that the housekeeping genes of C. jejuni and C. coli share 86.5% nucleotide sequence identity and that differences in growth, morphology, survival and metabolism may also be influenced by other genomic differences (Ketley and Konkel, 2005; Sheppard et al., 2008). A recent in vitro study showed that locally liberated L-fucose by secreted fucosidases from other species can increase growth and invasion of fuc + C. jejuni strains at the intestinal epithelial interface (Garber et al., 2020; Luijkx et al., 2020). Similar experiments have not been reported for C. coli, and combined with the observation that C. coli lacks most of the virulence genes described for C. jejuni (Bravo et al., 2021; Elmi et al., 2021), impact of L-fucose utilization on in vitro invasion studies and human infection requires further study.
In conclusion, our study demonstrated that possessing the L-fucose cluster is not only beneficial to C. jejuni NCTC11168 but also to other tested C. jejuni isolates Ca1352 and Ca2426, and C. coli isolate Ca0121. All tested isolates originated from different hosts, showed enhanced survival and prolonged spiral shaped morphology in the presence of L-fucose, with the C. coli isolate having the most robust phenotype. Further research into C. jejuni and C. coli isolates may reveal whether specific L-fucose utilization cluster genotypes link with specific phenotypic behavior including inter-host and environmental transmission, and pathogenesis.
Data Availability Statement
The datasets presented in this study can be found in online repositories. The names of the repository/repositories and accession number(s) can be found below: https://www.ncbi.nlm.nih.gov/, ERR4224873 https://www.ncbi.nlm.nih.gov/, ERR4224716 https://www.ncbi.nlm.nih.gov/, ERR4224751.
Author Contributions
PM designed and executed the experiments, carried out the data analysis, interpreted the results and wrote the manuscript. AZ provided BioIT feedback and reviewed the manuscript and provided feedback on the manuscript. WJ-R, HB, and TA supervised this study, critically reviewed the manuscript and provided feedback. All authors read and approved the final manuscript.
Funding
This research was financially supported by the Graduate School VLAG (Wageningen University & Research, Wageningen, Netherlands).
Conflict of Interest
The authors declare that the research was conducted in the absence of any commercial or financial relationships that could be construed as a potential conflict of interest.
Publisher’s Note
All claims expressed in this article are solely those of the authors and do not necessarily represent those of their affiliated organizations, or those of the publisher, the editors and the reviewers. Any product that may be evaluated in this article, or claim that may be made by its manufacturer, is not guaranteed or endorsed by the publisher.
Acknowledgments
AZ acknowledges financial support by the Netherlands’ Organization for Health Research and Development (ZonMw) with grant no. 50-52200-98-316 (project name: “DEPiCT – Discerning Environmental Pathways of Campylobacter Transmission”).
Supplementary Material
The Supplementary Material for this article can be found online at: https://www.frontiersin.org/articles/10.3389/fmicb.2022.872207/full#supplementary-material
Footnotes
- ^ www.benchling.com
- ^ https://string-db.org/
- ^ https://github.com/gamcil/clinker
- ^ http://arete.ibb.waw.pl/PL/html/boxshade.html
References
Becker, D. J., and Lowe, J. B. (2003). Fucose: biosynthesis and biological function in mammals. Glycobiology 13, 41R–53R. doi: 10.1093/glycob/cwg054
Black, R. E., Levine, M. M., Clements, M. L., Hughes, T. P., and Blaser, M. J. (1988). Experimental Campylobacter jejuni infection in humans. J. Infect. Dis. 157, 472–479. doi: 10.1093/infdis/157.3.472
Boren, T., Falk, P., Roth, K. A., Larson, G., and Normark, S. (1993). Attachment of Helicobacter pylori to human gastric epithelium mediated by blood group antigens. Science 262, 1892–1895. doi: 10.1126/science.8018146
Bravo, V., Katz, A., Porte, L., Weitzel, T., Varela, C., Gonzalez-Escalona, N., et al. (2021).. Genomic analysis of the diversity, antimicrobial resistance and virulence potential of clinical Campylobacter jejuni and Campylobacter coli strains from Chile. PLoS Negl. Trop. Dis. 15:e0009207. doi: 10.1371/journal.pntd.0009207
Bui, X. T., Qvortrup, K., Wolff, A., Bang, D. D., and Creuzenet, C. (2012). Effect of environmental stress factors on the uptake and survival of Campylobacter jejuni in Acanthamoeba castellanii. BMC Microbiol. 12:232. doi: 10.1186/1471-2180-12-232
Clark, C. G., Lawrence Price, R. A., Woodward, D. L., Melito, P. L., Rodgers, F. G., Jamieson, F., et al. (2003). Characterization of waterborne outbreak–associated Campylobacter jejuni, Walkerton, Ontario. Emerg. Infect. Dis. 9:1232. doi: 10.3201/eid0910.020584
Day, C. J., Tiralongo, J., Hartnell, R. D., Logue, C. A., Wilson, J. C., von Itzstein, M., et al. (2009). Differential carbohydrate recognition by Campylobacter jejuni isolate 11168: influences of temperature and growth conditions. PLoS One 4:e4927. doi: 10.1371/journal.pone.0004927
Devleesschauwer, B., Bouwknegt, M., Mangen, M. J. J., and Havelaar, A. H. (2017). “Health and economic burden of Campylobacter,” in Campylobacter: Features, Detection and Prevention of Foodborne Disease, ed. G. Klein (Cambridge, MA: Academic Press), 27–40. doi: 10.1016/b978-0-12-803623-5.00002-2
Di Tommaso, P., Moretti, S., Xenarios, I., Orobitg, M., Montanyola, A., Chang, J. M., et al. (2011). T-Coffee: a web server for the multiple sequence alignment of protein and RNA sequences using structural information and homology extension. Nucleic Acids Res. 39, W13–W17. doi: 10.1093/nar/gkr245
Dwivedi, R., Nothaft, H., Garber, J., Xin Kin, L., Stahl, M., Flint, A., et al. (2016). L-fucose influences chemotaxis and biofilm formation in Campylobacter jejuni. Mol. Microbiol. 101, 575–589. doi: 10.1111/mmi.13409
Elmi, A., Nasher, F., Dorrell, N., Wren, B., and Gundogdu, O. (2021). Revisiting Campylobacter jejuni virulence and fitness factors: role in sensing, adapting, and competing. Front. Cell. Infect. Microbiol. 895:607704. doi: 10.3389/fcimb.2020.607704
European Food and Safety Authority (2019). European food safety 3. Authority the European union one health 2018 Zoonoses report. EFSA J. 17:e05926. doi: 10.2903/j.efsa.2019.5926
Frirdich, E., Biboy, J., Huynh, S., Parker, C. T., Vollmer, W., and Gaynor, E. C. (2017). Morphology heterogeneity within a Campylobacter jejuni helical population: the use of calcofluor white to generate rod-shaped C. jejuni 81-176 clones and the genetic determinants responsible for differences in morphology within 11168 isolates. Mol. Microbiol. 104, 948–971. doi: 10.1111/mmi.13672
Galanis, E. (2007). Campylobacter and bacterial gastroenteritis. CMAJ 177, 570–571. doi: 10.1503/cmaj.070660
Gao, B., Vorwerk, H., Huber, C., Lara-Tejero, M., Mohr, J., Goodman, A. L., et al. (2017). Metabolic and fitness determinants for in vitro growth and intestinal colonization of the bacterial pathogen Campylobacter jejuni. PLoS Biol. 15:e2001390. doi: 10.1371/journal.pbio.2001390
Garber, J. M., Nothaft, H., Pluvinage, B., Stahl, M., Bian, X., Porfirio, S., et al. (2020). The gastrointestinal pathogen Campylobacter jejuni metabolizes sugars with potential help from commensal Bacteroides vulgatus. Commun. Biol. 3, 1–11. doi: 10.1038/s42003-019-0727-5
Garénaux, A., Jugiau, F., Rama, F., De Jonge, R., Denis, M., Federighi, M., et al. (2008). Survival of Campylobacter jejuni isolates from different origins under oxidative stress conditions: effect of temperature. Curr. Microbiol. 56, 293–297. doi: 10.1007/s00284-007-9082-8
Guccione, E. J., Kendall, J. J., Hitchcock, A., Garg, N., White, M. A., Mulholland, F., et al. (2017). Transcriptome and proteome dynamics in chemostat culture reveal how Campylobacter jejuni modulates metabolism, stress responses and virulence factors upon changes in oxygen availability. Environ. Microbiol. 19, 4326–4348. doi: 10.1111/1462-2920.13930
Hara-Kudo, Y., and Takatori, K. (2011). Contamination level and ingestion dose of foodborne pathogens associated with infections. Epidemiol. Infect. 139, 1505–1510. doi: 10.1017/S095026881000292X
Hazeleger, W. C., Janse, J. D., Koenraad, P., Beumer, R. R., Rombouts, F. M., and Abee, T. (1995). Temperature-dependent membrane fatty acid and cell physiology changes in coccoid forms of Campylobacter jejuni. Appl. Environ. Microbiol. 61, 2713–2719. doi: 10.1128/aem.61.7.2713-2719.1995
Hofreuter, D. (2014). Defining the metabolic requirements for the growth and colonization capacity of Campylobacter jejuni. Front. Cell. Infect. Microbiol. 4:137. doi: 10.3389/fcimb.2014.00137
Hofreuter, D., Novik, V., and Galán, J. E. (2008). Metabolic diversity in Campylobacter jejuni enhances specific tissue colonization. Cell Host Microbe 4, 425–433. doi: 10.1016/j.chom.2008.10.002
Hugdahl, M. B., Beery, J., and Doyle, M. (1988). Chemotactic behavior of Campylobacter jejuni. Infect. Immun. 56, 1560–1566. doi: 10.1128/iai.56.6.1560-1566.1988
Ikeda, N., and Karlyshev, A. V. (2012). Putative mechanisms and biological role of coccoid form formation in Campylobacter jejuni. Eur. J. Microbiol. Immunol. 2, 41–49. doi: 10.1556/EuJMI.2.2012.1.7
Javed, M. A., Grant, A. J., Bagnall, M. C., Maskell, D. J., Newell, D. G., and Manning, G. (2010). Transposon mutagenesis in a hyper-invasive clinical isolate of Campylobacter jejuni reveals a number of genes with potential roles in invasion. Microbiology 156, 1134–1143. doi: 10.1099/mic.0.033399-0
Kaakoush, N. O., Castaño-Rodríguez, N., Mitchell, H. M., and Man, S. M. (2015). Global epidemiology of Campylobacter infection. Clin. Microbiol. Rev. 28, 687–720. doi: 10.1128/CMR.00006-15
Karagiannis, I., Sideroglou, T., Gkolfinopoulou, K., Tsouri, A., Lampousaki, D., Velonakis, E., et al. (2010). A waterborne Campylobacter jejuni outbreak on a Greek island. Epidemiol. Infect. 138, 1726–1734. doi: 10.1017/S0950268810002116
Kenyon, J., Inns, T., Aird, H., Swift, C., Astbury, J., Forester, E., et al. (2020). Campylobacter outbreak associated with raw drinking milk, North West England, 2016. Epidemiol. Infect. 148:e13. doi: 10.1017/S0950268820000096
Ketley, J. M., and Konkel, M. E. (2005). Campylobacter: Molecular and Cellular Biology. Wymondham: Horizon Bioscience.
Kuhn, K. G., Falkenhorst, G., Emborg, H.-D., Ceper, T., Torpdahl, M., Krogfelt, K., et al. (2017). Epidemiological and serological investigation of a waterborne Campylobacter jejuni outbreak in a Danish town. Epidemiol. Infect. 145, 701–709. doi: 10.1017/S0950268816002788
Kuusi, M., Klemets, P., Miettinen, I., Laaksonen, I., Sarkkinen, H., Hänninen, M. L., et al. (2004). An outbreak of gastroenteritis from a non-chlorinated community water supply. J. Epidemiol. Commun. Health 58, 273–277. doi: 10.17269/s41997-020-00300-x
Luijkx, Y. M., Bleumink, N. M., Jiang, J., Overkleeft, H. S., Wösten, M. M., Strijbis, K., et al. (2020). Bacteroides fragilis fucosidases facilitate growth and invasion of Campylobacter jejuni in the presence of mucins. Cell. Microbiol. 22:e13252. doi: 10.1111/cmi.13252
Man, L., Dale, A. L., Klare, W. P., Cain, J. A., Sumer-Bayraktar, Z., Niewold, P., et al. (2020). Proteomics of Campylobacter jejuni growth in deoxycholate reveals Cj0025c as a cystine transport protein required for wild-type human infection phenotypes. Mol. Cell. Proteomics 19, 1263–1280. doi: 10.1074/mcp.RA120.002029
Man, S. M. (2011). The clinical importance of emerging Campylobacter species. Nat. Rev. Gastroenterol. Hepatol. 8:669. doi: 10.1038/nrgastro.2011.191
Mendz, G. L., Ball, G. E., and Meek, D. J. (1997). Pyruvate metabolism in Campylobacter spp. Biochim. Biophys. Acta 1334, 291–302. doi: 10.1016/s0304-4165(96)00107-9
Mihaljevic, R. R., Sikic, M., Klancnik, A., Brumini, G., Mozina, S. S., and Abram, M. (2007). Environmental stress factors affecting survival and virulence of Campylobacter jejuni. Microb. Pathog. 43, 120–125. doi: 10.1016/j.micpath.2007.03.004
Montgomery, M. P., Robertson, S., Koski, L., Salehi, E., Stevenson, L. M., Silver, R., et al. (2018). Multidrug-resistant Campylobacter jejuni outbreak linked to puppy exposure—United States, 2016–2018. Morb. Mortal. Wkly. Rep. 67:1032. doi: 10.15585/mmwr.mm6737a3
Moore, J. E., Corcoran, D., Dooley, J. S., Fanning, S., Lucey, B., Matsuda, M., et al. (2005). Campylobacter. Vet. Res. 36, 351–382.
Mughini-Gras, L., Pijnacker, R., Coipan, C., Mulder, A. C., Veludo, A. F., de Rijk, S., et al. (2020). Sources and transmission routes of campylobacteriosis: a combined analysis of genome and exposure data. J. Infect. 82, 216–226. doi: 10.1016/j.jinf.2020.09.039
Muraoka, W. T., and Zhang, Q. (2011). Phenotypic and genotypic evidence for L-fucose utilization by Campylobacter jejuni. J. Bacteriol. 193, 1065–1075. doi: 10.1128/JB.01252-10
Nachamkin, I., Szymanski, C. M., and Blaser, M. J. (2008). Campylobacter. Washington, DC: ASM Press.
Park, S. F. (2002). The physiology of Campylobacter species and its relevance to their role as foodborne pathogens. Int. J. Food Microbiol. 74, 177–188. doi: 10.1016/s0168-1605(01)00678-x
Parsons, C. M. (1984). Influence of caecectomy and source of dietary fibre or starch on excretion of endogenous amino acids by laying hens. Br. J. Nutr. 51, 541–548. doi: 10.1079/bjn19840059
Pascoe, B., Williams, L. K., Calland, J. K., Meric, G., Hitchings, M. D., Dyer, M., et al. (2019). Domestication of Campylobacter jejuni NCTC11168. Microb. Genom. 5:e000279. doi: 10.1099/mgen.0.000279
Pedati, C., Koirala, S., Safranek, T., Buss, B. F., and Carlson, A. V. (2019). Campylobacteriosis outbreak associated with contaminated municipal water supply—Nebraska, 2017. Morb. Mortal. Wkly. Rep. 68:169. doi: 10.15585/mmwr.mm6807a1
Pickard, J. M., and Chervonsky, A. V. (2015). Intestinal fucose as a mediator of host–microbe symbiosis. J. Immunol. 194, 5588–5593. doi: 10.4049/jimmunol.1500395
Rees, J. H., Soudain, S. E., Gregson, N. A., and Hughes, R. A. (1995). Campylobacter jejuni infection and Guillain–Barré syndrome. New Engl. J. Med. 333, 1374–1379. doi: 10.1056/NEJM199511233332102
Rodrigues, R. C., Haddad, N., Chevret, D., Cappelier, J. M., and Tresse, O. (2016). Comparison of proteomics profiles of Campylobacter jejuni strain Bf under microaerobic and aerobic conditions. Front. Microbiol. 7:1596. doi: 10.3389/fmicb.2016.01596
Sheppard, S. K., McCarthy, N. D., Falush, D., and Maiden, M. C. (2008). Convergence of Campylobacter species: implications for bacterial evolution. Science 320, 237–239. doi: 10.1126/science.1155532
Solomon, E. B., and Hoover, D. G. (1999). Campylobacter jejuni: a bacterial paradox. J. Food Saf. 19, 121–136. doi: 10.1111/j.1745-4565.1999.tb00239.x
Špaèková, M., Koláøová, K., and Gašpárek, M. (2019). Incidence and analysis of campylobacteriosis cases in the Czech Republic in 1997-2017. Epidemiol. Mikrobiol. Imunol. 68, 122–130.
Stahl, M., Butcher, J., and Stintzi, A. (2012). Nutrient acquisition and metabolism by Campylobacter jejuni. Front. Cell. Infect. Microbiol. 2:5. doi: 10.3389/fcimb.2012.00005
Stahl, M., Friis, L. M., Nothaft, H., Liu, X., Li, J., Szymanski, C. M., et al. (2011). L-fucose utilization provides Campylobacter jejuni with a competitive advantage. Proc. Natl. Acad. Sci. U.S.A. 108, 7194–7199. doi: 10.1073/pnas.1014125108
Sulaeman, S., Hernould, M., Schaumann, A., Coquet, L., Bolla, J. M., De, E., et al. (2012). Enhanced adhesion of Campylobacter jejuni to abiotic surfaces is mediated by membrane proteins in oxygen-enriched conditions. PLoS One. 7:e46402. doi: 10.1371/journal.pone.0046402
Tack, D. M., Marder, E. P., Griffin, P. M., Cieslak, P. R., Dunn, J., Hurd, S., et al. (2019). Preliminary incidence and trends of infections with pathogens transmitted commonly through food—foodborne diseases active surveillance network, 10 US Sites, 2015–2018. Morb. Mortal. Wkly. Rep. 68:369. doi: 10.15585/mmwr.mm6816a2
Thomas, M. T., Shepherd, M., Poole, R. K., van Vliet, A. H., Kelly, D. J., and Pearson, B. M. (2011). Two respiratory enzyme systems in Campylobacter jejuni NCTC11168 contribute to growth on L-lactate. Environ. Microbiol. 13, 48–61. doi: 10.1111/j.1462-2920.2010.02307.x
Turonova, H., Briandet, R., Rodrigues, R., Hernould, M., Hayek, N., Stintzi, A., et al. (2015). Biofilm spatial organization by the emerging pathogen Campylobacter jejuni: comparison between NCTC 11168 and 81-176 strains under microaerobic and oxygen-enriched conditions. Front. Microbiol. 6:709. doi: 10.3389/fmicb.2015.00709
van der Hooft, J. J., Alghefari, W., Watson, E., Everest, P., Morton, F. R., Burgess, K. E., et al. (2018). Unexpected differential metabolic responses of Campylobacter jejuni to the abundant presence of glutamate and fucose. Metabolomics 14:144. doi: 10.1007/s11306-018-1438-5
Vegge, C. S., Jansen van Rensburg, M. J., Rasmussen, J. J., Maiden, M. C., Johnsen, L. G., Danielsen, M., et al. (2016). Glucose metabolism via the Entner-Doudoroff pathway in Campylobacter: a rare trait that enhances survival and promotes biofilm formation in some isolates. Front. Microbiol. 7:1877. doi: 10.3389/fmicb.2016.01877
Vellinga, A., and Van Loock, F. (2002). The dioxin crisis as experiment to determine poultry-related Campylobacter enteritis. Emerg. Infect. Dis. 8:19. doi: 10.3201/eid0801.010129
Vorwerk, H., Huber, C., Mohr, J., Bunk, B., Bhuju, S., Wensel, O., et al. (2015). A transferable plasticity region in Campylobacter coli allows isolates of an otherwise non-glycolytic food-borne pathogen to catabolize glucose. Mol. Microbiol. 98, 809–830. doi: 10.1111/mmi.13159
Vorwerk, H., Mohr, J., Huber, C., Wensel, O., Schmidt-Hohagen, K., Gripp, E., et al. (2014). Utilization of host-derived cysteine-containing peptides overcomes the restricted sulphur metabolism of Campylobacter jejuni. Mol. Microbiol. 93, 1224–1245. doi: 10.1111/mmi.12732
Wagley, S., Newcombe, J., Laing, E., Yusuf, E., Sambles, C. M., Studholme, D. J., et al. (2014). Differences in carbon source utilisation distinguish Campylobacter jejuni from Campylobacter coli. BMC Microbiol. 14:262. doi: 10.1186/s12866-014-0262-y
Keywords: L-fucose consumption, animal and human origin, fitness, metabolism, HPLC, Campylobacter jejuni, Campylobacter coli
Citation: Middendorf PS, Jacobs-Reitsma WF, Zomer AL, den Besten HMW and Abee T (2022) Comparative Analysis of L-Fucose Utilization and Its Impact on Growth and Survival of Campylobacter Isolates. Front. Microbiol. 13:872207. doi: 10.3389/fmicb.2022.872207
Received: 09 February 2022; Accepted: 31 March 2022;
Published: 29 April 2022.
Edited by:
Dario De Medici, National Institute of Health (ISS), ItalyReviewed by:
Odile Tresse, INRA Centre Angers-Nantes Pays de la Loire, FranceAndreas Erich Zautner, University Hospital Magdeburg, Germany
Copyright © 2022 Middendorf, Jacobs-Reitsma, Zomer, den Besten and Abee. This is an open-access article distributed under the terms of the Creative Commons Attribution License (CC BY). The use, distribution or reproduction in other forums is permitted, provided the original author(s) and the copyright owner(s) are credited and that the original publication in this journal is cited, in accordance with accepted academic practice. No use, distribution or reproduction is permitted which does not comply with these terms.
*Correspondence: Tjakko Abee, dGpha2tvLmFiZWVAd3VyLm5s; Heidy M. W. den Besten, SGVpZHkuZGVuYmVzdGVuQHd1ci5ubA==