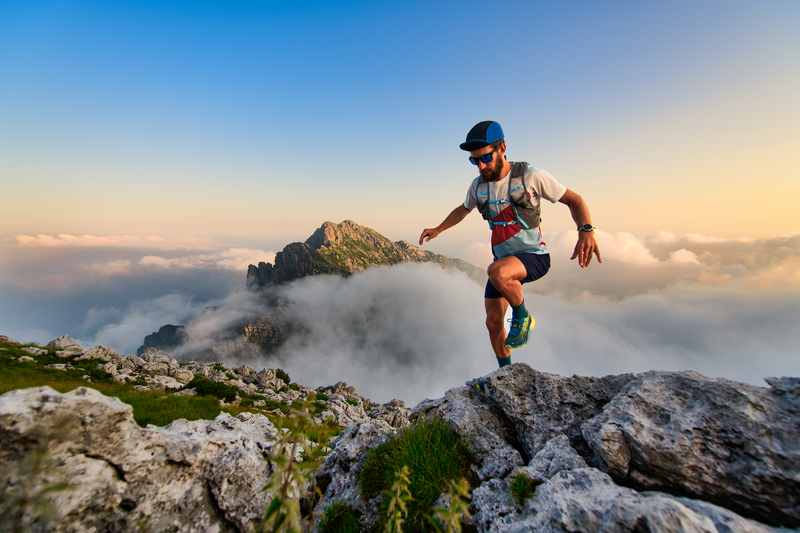
95% of researchers rate our articles as excellent or good
Learn more about the work of our research integrity team to safeguard the quality of each article we publish.
Find out more
ORIGINAL RESEARCH article
Front. Microbiol. , 14 July 2022
Sec. Virology
Volume 13 - 2022 | https://doi.org/10.3389/fmicb.2022.866705
This article is part of the Research Topic HIV-1 Genetic Diversity, Volume II View all 21 articles
The emergence and spread of new HIV-1 variants pose a challenge for the effectiveness of antiretrovirals (ARV) targeting Pol proteins. During viral evolution, non-synonymous mutations have fixed along the viral genome, leading to amino acid (aa) changes that can be variant-specific (V-markers). Those V-markers fixed in positions associated with drug resistance mutations (DRM), or R-markers, can impact drug susceptibility and resistance pathways. All available HIV-1 Pol sequences from ARV-naïve subjects were downloaded from the United States Los Alamos HIV Sequence Database, selecting 59,733 protease (PR), 6,437 retrotranscriptase (RT), and 6,059 integrase (IN) complete sequences ascribed to the four HIV-1 groups and group M subtypes and circulating recombinant forms (CRFs). Using a bioinformatics tool developed in our laboratory (EpiMolBio), we inferred the consensus sequences for each Pol protein and HIV-1 variant to analyze the aa conservation in Pol. We analyzed the Wu–Kabat protein variability coefficient (WK) in PR, RT, and IN group M to study the susceptibility of each site to evolutionary replacements. We identified as V-markers the variant-specific aa changes present in >75% of the sequences in variants with >5 available sequences, considering R-markers those V-markers that corresponded to DRM according to the IAS-USA2019 and Stanford-Database 9.0. The mean aa conservation of HIV-1 and group M consensus was 82.60%/93.11% in PR, 88.81%/94.07% in RT, and 90.98%/96.02% in IN. The median group M WK was 10 in PR, 4 in RT, and 5 in IN. The residues involved in binding or catalytic sites showed a variability <0.5%. We identified 106 V-markers: 31 in PR, 28 in RT, and 47 in IN, present in 11, 12, and 13 variants, respectively. Among them, eight (7.5%) were R-markers, present in five variants, being minor DRM with little potential effect on ARV susceptibility. We present a thorough analysis of Pol variability among all HIV-1 variants circulating to date. The relatively high aa conservation observed in Pol proteins across HIV-1 variants highlights their critical role in the viral cycle. However, further studies are needed to understand the V-markers’ impact on the Pol proteins structure, viral cycle, or treatment strategies, and periodic variability surveillance studies are also required to understand PR, RT, and IN evolution.
HIV is one of the most genetically diverse pathogens due to its high recombination and mutation rates and its rapid replication rate (Hemelaar, 2012; Hemelaar et al., 2019). HIV mutations during replication are favored by the error-prone polymerization by the HIV reverse transcriptase (RT) that lacks proofreading exonuclease activity (Roberts et al., 1988; Bebenek et al., 1993). HIV-1 is responsible for most HIV infections worldwide. It is divided into four groups according to genetic homology: M (major or main), N (non-M, non-O) (Simon et al., 1998), O (outlier) (De Leys et al., 1990), and P (Plantier et al., 2009). Group M is the main HIV group related to the current HIV global pandemic (Hemelaar et al., 2019). This group is subdivided into 10 subtypes (A–D, F–H, and J–L) and 8 sub-subtypes (A1, A2, A3, A4, A5, A6, F1, and F2) (Robertson et al., 2000; Salminen et al., 2000; Yamaguchi et al., 2020), at least 118 circulating recombinant forms (CRFs) (Los Alamos National Laboratory, 2021), and countless unique recombinant forms (URF). The Pol protein has been associated with differences in the replication capacity and disease progression of the different subtypes (Nagata et al., 2017).
The HIV-1 pol gene encodes the three enzymes needed for viral replication: protease (PR), RT, and integrase (IN). These proteins have essential roles in the viral cycle and are the main targets of antiretroviral drugs (ARV) (Huff, 1991; Eron, 2000; El Safadi et al., 2007; Gu et al., 2020; Jóźwik et al., 2020). Molecular detection of Pol mutations associated with ARV resistance has enabled resistance monitoring and individualization of antiretroviral treatment (ART) regimens in HIV-positive subjects (Clarke, 2002). This approach is well extended in middle- and high-income countries, where clinicians often use online resistance interpretation algorithms, such as Stanford HIVdb Program1, to detect drug resistance mutations (DRM) in pol sequences and for HIV fast subtyping. HIV-1 pol diversity within HIV-1 variants is high and could impact ARV susceptibility (Holguín et al., 2006b). Surveillance of DRM in non-B subtypes and recombinants is essential (Holguín and Soriano, 2002; Holguín et al., 2004; Kantor, 2006; Llacer Delicado et al., 2016), as most studies focus on HIV-1 subtype B, more prevalent in Western Europe and the United States (Hemelaar et al., 2019).
The PR (99 aa) is responsible for processing the Gag and Gag–Pol precursors into mature Gag and Pol viral proteins by site-specific cleavage to produce the matrix, capsid, nucleocapsid, P1 and P2 spacer segments and P6 proteins of Gag, and the PR, RT, and IN proteins of Pol (Frankel and Young, 1998; Konvalinka et al., 2015). Variability in specific cleavage sites has been detected across HIV-1 groups, subtypes, and recombinants (Torrecilla et al., 2014). This could affect Gag and Pol proteins’ processing, viral budding, restore viral fitness, and influence the virological outcome of specific ARV (Goodenow et al., 2002; Myint et al., 2004; Holguín et al., 2006a; Dam et al., 2009). PR functions as a dimer with flexible flaps that close down on the active site upon substrate binding. This site resembles other aspartyl proteases with the conserved triad sequence Asp25-Thr26-Gly27 (Navia et al., 1989; Frankel and Young, 1998). There are five FDA-approved protease inhibitors currently recommended in the HHS HIV/AIDS medical practice guidelines (NIH FDA-Approved HIV Medicines, 2022).
The RT catalyzes RNA-dependent and DNA-dependent DNA polymerization reactions (Hu and Hughes, 2012). It is a heterodimer containing subunits p51 (440 aa) and p66 (560 aa), each with a polymerase domain composed of four subdomains (fingers, palm, thumb, and connection) and identical sequences, except for p66 additional RNase H domain (Rodgers et al., 1995). The polymerase active site contains the catalytic triad Asp110, Asp185, and Asp186, conserved in many polymerases (Frankel and Young, 1998). There are two classes of RT inhibitors: nucleoside RT inhibitors (NRTI) and non-nucleoside RT inhibitors (NNRTI), with a total of 10 FDA-approved RT inhibitors currently recommended in the HHS HIV/AIDS medical practice guidelines (NIH FDA-Approved HIV Medicines, 2022).
The IN (288 aa) catalyzes a series of reactions to integrate the viral genome into the host chromosome (Frankel and Young, 1998; Engelman and Singh, 2018). The N-terminal domain (aa 1–55) is dimeric and contains a zinc-binding site: His12, His16, Cys40, and Cys43 (Cai et al., 1997). The catalytic domain (aa 50–212) contains a D–D–E motif (Asp64, Asp116, and Glu152) conserved among integrases, essential for the processing and joining reactions (Dyda et al., 1994; Rice et al., 1996). Finally, the C-terminal domain has non-specific DNA-binding activity (Eijkelenboom et al., 1999). Integrase strand transfer inhibitors (INSTIs) are the most recently developed ARV drugs. There are three INSTIs approved by the FDA and currently recommended in the HHS HIV/AIDS medical practice guidelines (NIH FDA-Approved HIV Medicines, 2022). The three drugs bind to a common D–D–E motif in the IN catalytic domain, causing it to disengage (Sharma et al., 2014).
Since ARV development, more than 100 DRM have been described and classified as primary or secondary according to their effect on ARV efficacy (Shafer and Schapiro, 2008). During viral evolution, non-synonymous nucleotide mutations have been fixed along the viral genome, leading to aa changes; some of them are variant-specific (V-markers). Some V-markers can be related to drug resistance (R-markers) when fixed in positions associated with ARV resistance in the absence of antiretroviral therapy and may impact drug susceptibility and resistance pathways (Holguín et al., 2006b).
HIV Pol protein has an essential functional role in the viral cycle, being the main target for ARV and often used by clinicians to classify HIV-1 variants. The emergence of new HIV-1 variants and the spread of HIV-1 non-B subtypes and recombinants worldwide pose a challenge for the accuracy and efficiency of ARV, DRM detection, and fast subtyping online tools.
This descriptive study presents a thorough analysis of Pol diversity among HIV-1 variants circulating to date using ARV-naïve pol sequences available in Los Alamos National Laboratory HIV Sequence Database (LANL). We provide the aa conservation rate per residue within variants in PR, RT, and IN proteins. We also identify the V-markers and the R-markers across HIV-1 variants, analyzing the mean conservation of the consensus sequences of each HIV-1 variant and HIV-1 group in the three Pol proteins.
In January 2022, we downloaded from the LANL database2 all the available pol HIV-1 sequences from drug-naïve subjects carrying different HIV-1 variants (groups, subtypes, sub-subtypes, and CRFs), selecting the corresponding genome region (PR, RT, and IN). Before the downloading process, we selected only drug-naïve sequences and only one sequence per patient in the LANL (Los Alamos National Laboratory, 2022b) platform. We also considered as INSTI naïve all participants with IN sequences sampled before 2007, the year of marketing authorization of the first INSTI, raltegravir, and the start date of the first clinical study with an authorized INSTI both in Europe and in the United States. URF sequences and incomplete PR, RT, and IN sequences were not included in this study. The sequences were also sorted by country of origin and organized in geographic regions according to the United Nations geoscheme3 joining the regions of Central America and The Caribbean and the regions of Southern Asia and Southeastern Asia for practical purposes. The maps for Figures 1, 2 were created using MapChart4.
Figure 1. Number of HIV-1 Pol sequences per country included in this study as available in Los Alamos HIV sequence database (LANL) in January 2022. PR, protease; RT, reverse transcriptase; IN, integrase. (A) Protease sequences per country. Total LANL sequences: 59.733. (B) Reverse transcriptase sequences per country. Total LANL sequences: 6.437. (C) Integrase sequences per country. Total LANL sequences: 6.059. Eleven integrase sequences had no record of the country of origin.
Figure 2. Geographic distribution by regions of HIV-1 Pol variants available in Los Alamos HIV sequence database (LANL) in January 2022. HIV-1 variant distribution within regions in PR (A), RT (B), and IN (C). PR, Protease; RT, reverse transcriptase; IN, integrase. Countries are colored by regions according to the United Nations geoscheme (https://unstats.un.org). Geographic regions color code inside the box in (A). Pie graphs show the percentage of the HIV-1 variants per region as available in LANL in January 2022 and the most frequent variant per region. The total number of available LANL sequences per region is in brackets beside the region name. NA, Northern Africa; SA, Southern Africa; EA, Eastern Africa; WA, Western Africa; CA, Central Africa; SAM, South America; CAC, Central America and The Caribbean; NAM, North America; OC, Oceania; NEU, Northern Europe; SEU, Southern Europe; EEU, Eastern Europe; WEU, Western Europe; CAS, Central Asia; SEAS, Southern and Southeastern Asia; EAS, Eastern Asia; WAS, Western Asia.
A sequence analysis was performed with an in-house bioinformatics tool (EpiMolBio) previously designed and used in our laboratory for HIV genetic variability analysis and recently updated for SARS-CoV-2 sequences study (Burgos et al., 2019; Troyano-Hernáez et al., 2019, 2020, 2021a,b,2022). This tool is programmed in JAVA OpenJDK version 11.0.9.1 using IDE NetBeans version 12.2. Among other functions, this tool calculates the conservation of a sequence set compared with a reference sequence and the rate of aa changes for each position within the studied protein. Furthermore, it can infer a consensus from a group of sequences or previously calculated consensuses considering the total number of sequences and the frequency of any specific aa residue per position, avoiding the overestimation of polymorphisms present in variants with a small number of available sequences. We used HIV-1 reference sequence HXB2 (NCBI accession number K03455.1) for the sequences’ alignment and EpiMolBio functions that required a reference sequence, such as conservation analysis and V-marker detection.
We inferred the PR, RT, and IN consensus sequence for HIV-1, each HIV-1 group (M, N, O, and P), and each HIV-1 group M variant (subtype, sub-subtype, CRF) using all downloaded LANL sequences. Group M consensus was generated from the consensus of group M subtypes, sub-subtypes, and CRF. HIV-1 consensus was inferred considering the consensuses of the four groups (M, N, O, and P). We calculated the mean aa conservation of group M and HIV-1 consensus sequences for the three Pol proteins and the variability of the residues involved in binding or catalytic sites. We also studied the average aa conservation of the PR, RT, and IN group M variants with >5 available sequences compared with HXB2 HIV-1 reference sequence.
We identified the presence of single variant markers or V-markers, defined as the natural aa changes specific for each variant and present in >75% of the sequence set for a given position in variants with >5 sequences available in LANL, to avoid biases due to a low number of sequences. We considered as R-markers, the V-markers coinciding with major or minor DRM to the four main ARV families (PI, NRTI, NNRTI, and INSTI) according to the updated version of two sources: Stanford HIV Drug Resistance Database v9.05 and IAS-USA 2019 (Wensing et al., 2019). The sub-classification of DRM into major or minor DRM was done following the Stanford Database 9.0 criteria, which considers the effect on in vitro drug susceptibility, the frequency among patients with virological failure, the presence among untreated persons, and the location of the mutation within the 3D structure protein. Deletions and insertions were not included in this study.
In the pol variants with >5 available sequences, besides detecting V- and R-markers, we also checked for the presence of drug resistance mutations present in the WHO 2009 list for transmitted mutations or TDR (Bennett et al., 2009) and of major and minor DRM present in at least 25% of the sequences for each variant. To study the effect of these DRM and R-markers on ARV susceptibility, we analyzed them with the online resistance interpretation algorithm Stanford HIVdb Program v9.06, which infers susceptibility to 25 ARV from PI, NRTI, NNRTI, and INSTI drug families.
We calculated the Wu–Kabat protein variability coefficient (WK) for group M using all available PR, RT, and IN sequences belonging to this group. WK coefficient allows studying the susceptibility of an aa position to evolutionary replacements (Kabat et al., 1977). It was calculated using the following formula: variability = N × k/n, where N is the number of sequences in the alignment, k is the number of different amino acids at a given position, and n is the absolute frequency of the most common amino acid at that position. Therefore, a WK of 1 indicates the same aa was found for that position in all the sequence set, whereas a WK >1 indicates the relative variability of the respective site, with greater diversity as the WK value increases.
A total of 59,733 PR (32,745 group M non-B subtypes and CRF or non-B variants), 6,437 RT (4,393 non-B variants), and 6,059 IN (4,552 non-B variants) sequences were included in this study (Supplementary Table 1). Subtypes with the greatest sequence representation in group M were subtype B (45.1% in PR, 31.6% in RT, and 23.8% in IN), followed by subtype C (16.6% in PR, 25.0% in RT, and 22.3% in IN). The most represented CRF was recombinant 01_AE (15.2% in PR, 19.0% in RT, and 19.7% in IN).
The country of origin of the LANL available sequences for each Pol protein is illustrated in Figure 1 (complete information in Supplementary Table 2). The geographic distribution by regions of the HIV-1 variants with available pol sequence in LANL is illustrated in Figure 2 (described in Supplementary Table 3). PR sequences showed the highest diversity regarding the number of countries of origin of the sequenced samples (118 countries) compared with RT (50 countries) and IN (85 countries). In the three Pol proteins, China was the country that contributed the most sequences to the LANL database (11% in PR, 22% in RT, and 16% in IN), followed by South Africa in RT (15%) and IN (11%), and the United States in PR (10%), which was in third place in RT (11%) and IN (8%). Thus, the countries present in each geographic region were not homogeneous in the three Pol proteins, and some geographic regions presented different HIV-1 main variants between Pol proteins. However, subtype B was the main variant for PR, RT, and IN in America, Western and Southern Europe, and Oceania, while there was a greater percentage of subtype A6 in Eastern Europe, CRF 01_AE in Southern, Southeastern, and Eastern Asia, and subtype C in Southern and Western Africa. The regions with more variant diversity in the three proteins were Central and Western Africa (Figure 2).
Supplementary Table 4 reports the inferred consensus sequences for HIV-1, each HIV-1 group and group M variant in the three Pol proteins, showing the most frequent aa found per residue. Group M consensus was inferred using 84 variants’ consensus in PR, 52 in RT, and 86 in IN. HIV-1 consensus and group M consensus sequences of PR, RT, and IN are displayed in Figures 3–5, respectively. The percentage of conservation of the most prevalent aa in each residue is indicated with a color code: dark green (100%), green (≥90–<100%), light green (>75–<90%), yellow (>50–≤75%), and gray (≤50%). The HXB2 reference sequence was included for further guidance. These figures also indicate the positions where major DRM to the four main drug families are located according to Stanford v9.0 and the location of PR, RT, and IN catalytic sites.
Figure 3. Amino acid conservation rate along PR in HIV-1 and group M consensus. aa, amino acid; M, group M consensus. PR, protease (99 aa). Dots in group M represent the same aa as in HIV-1 consensus for that position. HXB2 reference sequence is described below the groups for further guidance. Colors represent the conservation rate. Residues of PR active site (triad Asp25-Thr26-Gly27, conserved among aspartyl proteases) are highlighted in red font. Orange triangles indicate positions where major DRM to PI are located according to Stanford v9.0 (Release Notes - HIV Drug Resistance Database, 2020) and summarized in https://cms.hivdb.org/prod/downloads/resistance-mutation-handout/resistance-mutation-handout.pdf. Aa code: A, alanine; C, cysteine; D, aspartic acid; E, glutamic acid; F, phenylalanine; G, glycine; H, histidine; I, isoleucine; K, lysine; L, leucine; M, methionine; N, asparagine; P, proline; Q, glutamine; R, arginine; S, serine; T, threonine; V, valine; W, tryptophan; Y, tyrosine.
Figure 4. Amino acid conservation rate along RT in HIV-1 and group M consensus. Aa, amino acid; M, group M consensus. RT, reverse transcriptase (440 aa). Dots in group M represent the same aa as in HIV-1 consensus for that position. HXB2 reference sequence is described below the groups for further guidance. Colors represent the conservation rate. Residues of the catalytic triad (Asp110, Asp185, and Asp186) are highlighted in red font. Blue crosses and blue diamonds indicate positions where DRM to NRTI and to NNRTI, respectively, are located according to Stanford v9.0 (Release Notes - HIV Drug Resistance Database, 2020). Aa code according to Figure 3.
Figure 5. Amino acid conservation rate along IN in HIV-1 and group M consensus. Aa, amino acid; M, group M consensus. IN, integrase (288 aa). Dots in group M represent the same aa as in HIV-1 consensus for that position. HXB2 reference sequence is described below the groups for further guidance. Colors represent the conservation rate. Residues of the zinc-binding site (His12, His16, Cys40, and Cys43) and the D–D–E motif of the catalytic domain (Asp64, Asp116, and Glu152) are highlighted in red font. Green circles indicate positions where major INSTIs DRM are located according to Stanford v9.0 (Release Notes - HIV Drug Resistance Database, 2020). Aa code according to Figure 3.
The mean aa conservation of HIV-1 and group M consensus sequences was 82.60 and 93.11% in PR, 88.81 and 94.07% in RT, and 90.98 and 96.02% in IN, respectively. Table 1 describes the variability of PR, RT, and IN active sites and the mean residue variability in HIV-1 consensus for each protein. All the residues involved in binding or catalytic sites showed a variability below 0.5%, indicating a conservation over 95%. The mean aa conservation percentage in Pol residues of PR, RT, and IN is shown in Figures 3–5, respectively.
We included in this analysis 46 variants in PR, 16 in RT, and 36 in IN, all with >5 sequences in LANL. Subtype B, the variant with the highest number of Pol sequences at LANL, was the most conserved variant in the three proteins (93.22% in PR, 96.02% in RT, and 92.09% in IN). The most conserved CRFs were 51_01B in PR (92.80%), 89_BF in RT (94.62%), and 42_BF in IN (94.31%). The least conserved variants were CRF13_cpx in PR (83.5%), subtype G in RT (90.44%), and CRF06_cpx in IN (92.09%). In RT and IN, all the variants had a conservation >90%, whereas, in PR, only 24% of the variants with >5 sequences had a conservation above 90% (Figure 6).
Figure 6. Percentage of aa conservation of PR, RT, and IN across the HIV-1 group M variants with >5 sequences at LANL. X-axis: HIV-1 group M variants with >5 available sequences at LANL (46 in PR, 16 in RT, and 36 in IN). Y-axis: conservation rate for each variant included in this analysis. The horizontal line represents 90% conservation.
Among the variants with >5 available sequences, we found a total of 106 unique single V-markers and 8 R-markers present in >75% sequences (>75% conservation in their respective variants) across PR (Table 2), RT (Table 3), and IN (Table 4) variants. The analysis was performed in group O and 46 group M variants (7 subtypes, 6 sub-subtypes, and 33 CRF) of PR; in 16 group M variants (4 subtypes, 3 sub-subtypes, and 9 CRF) of RT; and in groups N and O, and 36 group M variants (7 subtypes, 5 sub-subtypes, and 24 CRF) of IN.
Table 2. Single V-markers and R-markers in protease found across HIV-1 variants with >5 LANL sequences.
Table 3. Single V-markers and R-markers in reverse transcriptase found across HIV-1 variants with >5 LANL sequences.
Table 4. Single V-markers and R-markers in integrase found across HIV-1 variants with >5 LANL sequences.
We detected 31 V-markers in PR (6 of them being R-markers), 28 V-markers in RT (1 R-marker), and 47 in IN (1 R-marker). None of the R-markers corresponded to major DRM, being all of them minor DRM according to Stanfordv 9.0. No V-markers were observed in the PR active site (Asp25, Thr26, and Gly27), in the RT catalytic triad (Asp110, Asp185, and Asp186), the IN zinc-binding site (His12, His16, Cys40, and Cys43), or the D–D–E motif (Asp64, Asp116, and Glu152) of the IN catalytic domain.
The 31 V-markers in PR were present in 11 variants (9 CRF, subtype J, and group O), being group O the variant with most V-markers: 15 (Table 2). Six (19.3%) of the 31 PR V-markers corresponded to R-markers: Four were detected in group O (K43T/Q58E/H69R/A71V) and two in group M complex recombinants CRF13_cpx (V77I) and CRF60_BC (L10V). The R-markers in group O were present in 83.3% (H69R), 87.5% (K43T), and 100% (Q58E, A71V) of this group’s PR sequences. L10V was found in 95.5% of CRF60_BC PR sequences and V77I in 96.6% of CRF13_cpx sequences. In RT, we detected a total of 28 V-markers in 12 group M variants (5 subtypes and 7 CRF). Only one (3.6%) of them was an R-marker: V179E, found in 100% of CRF55_01B RT sequences (Table 3). The largest number of V-markers was found in IN: 47 total V-markers in two non-M groups and 11 group M variants (3 subtypes and 8 CRF) (Table 4). Group O presented most V-markers (25/47, 53.2%). The only R-marker detected was E157Q in 100% of CRF03_A6B sequences.
No TDR present in the WHO list or major DRM to PI, NNRTI, NRTI, or INSTI were found in ≥25% of sequences belonging to PR, RT, or IN variants with >5 available sequences. However, we found seven minor DRM in the three Pol proteins. Two minor DRM to PI were detected in PR: Q58E and K43T, coinciding with two of group O R-markers in PR (Table 2). Another two minor DRM to NNRTI were found in RT: V179E in CRF06_cpx (29.4% sequences), coinciding with the only R-marker found in RT in CRF55_01B (Table 2), and V106I in sub-subtype F1 (42.5%). We found one minor DRM to NRTI, A62V, present in 47% of RT sequences of sub-subtype A6. Finally, two minor DRM to INSTI were detected in IN: G163R in CRF17_BF1 (28.6% sequences) and CRF89_BF1 (50%), and M50I in group O (49%), subtype A (60%), subtype C (48%), CRFs 11_cpx (31.2%), CRF22_01A1 (78.6%), and CRF63_02A6 (76.6%).
Figure 7 describes the group M variability WK coefficient plot in the three pol proteins using all available LANL sequences for this group (26,988 PR, 2,044 RT, and 1,507 IN), including 84/52/86 HIV-1 group M variants in PR/RT/IN: 9/5/9 subtypes, 8/6/7 sub-subtypes, and 67/41/70 CRF. The WK values for each residue and studied protein are described in Supplementary Table 5.
Figure 7. Wu–Kabat variability coefficient plot of PR, RT, and IN group M sequences. (A) Wu–Kabat variability coefficient plot of PR (99 aa). (B) Wu–Kabat variability coefficient plot of RT (440 aa). (C) Wu–Kabat variability coefficient plot of IN (288 aa). X-axis, amino acid position; Y-axis, WK variability coefficient.
The median variability coefficient in PR group M sequences was 10.26. The highest WK coefficient was 52 in residue 63, followed by WK 35 in site 69 (Figure 7A). The lowest WK was 3 in site 27, part of the triad Asp25, Thr26, and Gly27 in the PR active site. The other two residues of this triad (Asp25 and Thr26) presented a WK of 9 and 7, respectively. None of the 99 residues along PR were completely conserved (WK 1). Most PR residues had a WK between 10 and 20 (Figure 8).
Figure 8. Proportion of Wu–Kabat variability coefficient values in PR, RT, and IN residues. Each box represents the proportion of residues within each protein that present a Wu–Kabat coefficient value within the range indicated beneath the figure and colored accordingly. Protease (99 aa), reverse transcriptase (440 aa), integrase (288 aa). WK, WK variability coefficient.
The median WK along RT in group M sequences was 4.03. Site 245 presented the highest WK coefficient (WK 47.61), followed by site 207 (WK 46.84) and site 211 (WK 37.2) (Figure 7B). The smallest WK was 1, present in 20 of the 440 RT residues. The RT catalytic triad Asp110, Asp185, and Asp186 had a WK of 2, 3, and 4, respectively. Most RT residues had a WK between 1 and 5 (Figure 8).
IN median variability coefficient in group M sequences was 5. The highest WK coefficient was 29.35, located in residue 136, followed by site 125 (WK 16.6) and site 234 (WK 16.22) (Figure 7C). The smallest WK was 1, present in sites 131 and 235. The residues of the IN zinc-binding site, namely, His12, His16, and Cys40, showed a WK coefficient of 4, 7, and 5, respectively. The IN catalytic domain’s Asp64, Asp116, and Glu152 motif presented a WK of 4, 3, and 6, respectively. Most IN residues had a WK between 1 and 5 (Figure 8).
This descriptive study analyzes the Pol diversity among HIV-1 variants, providing the aa conservation rate per residue and HIV-1 variant in PR, RT, and IN proteins. A better understanding of HIV variability is important, since it has been reported that HIV-1 transmissibility, replication, and disease progression can differ between HIV-1 variants (Renjifo et al., 2004; Baeten et al., 2007; Bennett et al., 2009; Ng et al., 2014). The HIV-1 Pol proteins PR, RT, and IN are essential for viral replication and are the main targets of ARV (Huff, 1991; Eron, 2000; El Safadi et al., 2007; Gu et al., 2020; Jóźwik et al., 2020). Since Pol variability can impact ARV monitoring and efficacy, conservation studies must consider all circulating HIV-1 variants worldwide.
The consensus sequences of HIV proteins and their conservation studies allow a better understanding of structural, functional, and immunogenic potential differences across HIV-1 groups, subtypes, sub-subtypes, and recombinants and have been previously analyzed in other HIV-1 proteins (Li et al., 2013; Sliepen et al., 2019; Zhang et al., 2021). A recent work by Linchangco et al. reconstructed 90 HIV-1 subtype and CRF consensus sequences from 3,470 full HIV genomes downloaded from LANL (Linchangco et al., 2021). Our study updates and expands the knowledge regarding HIV Pol variability, including 59,733 PR, 6,437 RT, and 6,059 IN sequences from more than 100 different variants, including all the currently available HIV-1 groups, subtypes, and CRF in LANL. Moreover, Supplementary Table 4 summarizes the aa conservation in each Pol protein and each variant to help identify the conservation or consensus aa in any Pol residue and HIV-1 variant of interest.
The sequences were processed by an in-house bioinformatics tool (EpiMolBio) developed for HIV and SARS-CoV-2 variability analysis. In the most extensive panel of HIV-1 variants analyzed to date, we have also identified the natural polymorphisms that can be considered as genetic markers of each HIV-1 variant (V-markers) and those that correspond to major or minor DRM (R-markers) across HIV-1 groups, and group M subtypes and recombinants. We also present the consensus PR, RT, and IN sequences for HIV-1, HIV-1 groups, and variants, and the Wu–Kabat variability coefficient for group M in the three studied Pol proteins. This information is helpful to improve the understanding of structural, functional, and immunogenic differences across HIV-1 groups, subtypes, sub-subtypes, and recombinants and their impact on drug susceptibility and resistance pathways (Nagata et al., 2017; Sliepen et al., 2019; Zhang et al., 2021).
In previous studies, the variability in Pol proteins was low but slightly higher in PR compared with RT and IN (Turner et al., 2004; Rhee et al., 2016). In this study, the mean aa conservation of group M consensus sequences was high (>90%) in the three studied proteins, being slightly lower for PR (93% vs. 94% in RT and 96% in IN). As expected, HIV-1 consensus sequences were slightly less conserved (>80%) as non-M groups were included in the consensus. Still, the conservation rate followed the same order as in group M consensus, with less conservation in PR (83% vs. 88% in RT and 96% in IN). We also observed a low variability (below 0.5%) in the residues involved in binding or catalytic sites after testing all the available Pol sequences, highlighting the fragility of these important functional sites (Table 1).
HIV-1 variants have different global prevalence (Hemelaar et al., 2019) and levels of HIV-1 genetic diversity (Abecasis et al., 2009). HIV-1 group M subtype C is the most prevalent variant in the ongoing HIV pandemic, causing around 50% of worldwide infections (Hemelaar et al., 2019). In addition, subtype C is the most prevalent variant in Southern Africa and India; subtype A in some countries of Eastern Africa, Russia, and Eastern Europe; subtype B in the rest of Europe, the Americas, and Oceania; CRF01_AE in Asia; and CRF02_AG in Western Africa (Bbosa et al., 2019). However, HIV genomic sequencing is more widespread in economically developed nations, which explains that in our Pol dataset the most represented HIV-1 variant was subtype B, despite the fact that this variant only causes around 12% of the 38 million infections globally (Hemelaar et al., 2019), followed by the most abundant variant subtype C and recombinant CRF01_AE with the highest number of sequences belonging to China and the United States, according to the sequence availability in LANL. The main limitation in this study is the low number of sequences available in LANL for some non-B subtypes and CRF (Hemelaar et al., 2019), due to their low prevalence in the pandemic or because they are circulating in areas with none or scarce HIV sequencing.
Across group M variants with >5 available sequences in LANL, subtype B was the most conserved variant (>92%) in the three Pol proteins as expected, since the reference strain for the alignments was the subtype B HXB2 isolate. Again, PR showed slightly greater variability: While in RT and IN all the included variants had a conservation >90%, in PR only 24% of the variants were conserved in >90% of their sequences.
The Wu–Kabat protein variability coefficient (WK) was analyzed in PR, RT, and IN group M to study the susceptibility of each aa position to evolutionary replacements. The median variability coefficient in PR (WK10) was higher than that in IN (WK5) and RT (WK4). All 99 PR residues presented some degree of variability as none had a coefficient of 1. PR also presented the site with the highest WK value (52 in residue 63). Most IN (92%) and RT (88%) sites showed a WK below 10, while almost half (48%) of PR residues had a WK between 11 and 20, being the Pol protein with more sites prone to evolutionary replacements.
Although similar mutations occur in subtype B and non-subtype-B viruses and drug resistance evolution is comparable in both groups, subtype-specific mutation rates have been identified, with differences that could affect genotypic interpretation and DRM monitoring (Kantor et al., 2005; Kantor, 2006; Yebra et al., 2010). We found 31 total V-markers in PR, 28 V-markers in RT, and 47 in IN. Only eight were R-markers; none considered major DRM, being minor DRM with low impact in ARV susceptibility. In a previous study on HIV-2 variability (Troyano-Hernáez et al., 2021a), the R-markers corresponding to DRM to PI, NRTI, and INSTIs appeared not to have a significant impact on ARV susceptibility as well. However, HIV-2 presents natural polymorphisms related to drug resistance that make it naturally resistant to NNRTI, certain PI, and fusion inhibitor enfuvirtide (Tuaillon et al., 2004; Desbois et al., 2008; Menéndez-Arias and Álvarez, 2014).
Six R-markers were found in PR (K43T/Q58E/H69R/A71V in group O, V77I in CRF13_cpx, and L10V in CRF60_BC), one in RT (V179E in CRF55_01B), and one in IN (E157Q in CRF03_A6B). None of the R-markers in PR’s group O conferred intermediate or high-level resistance to PI alone or combined. K43T and Q58E are accessory non-polymorphic mutations that confer potential or low-level resistance to nelfinavir (NFV) and tipranavir/ritonavir (TPV/r) and other PIs (Rhee et al., 2003, 2010; Baxter et al., 2006; Bennett et al., 2009). H69R is a minor mutation affecting TPV/r according to IAS (Wensing et al., 2019) (not included in Stanford). A71V is a polymorphic accessory mutation associated with an increase of viral replication in the presence of other PI resistance mutations (Nijhuis et al., 1999; Rhee et al., 2003). V77I was present in 96.6% of CRF13_cpx PR sequences and is considered a minor mutation affecting indinavir/ritonavir according to IAS2019 (Wensing et al., 2019) (not included in Stanford). L10V was found in 95.5% of CRF60_BC PR sequences, being a polymorphic accessory mutation that may reduce PI susceptibility or increase the replication of viruses containing PI resistance mutations (Stanford University, 2022). Regarding the R-marker found in RT, V179E is a DRM to NNRTI, considered a non-polymorphic accessory mutation associated with potential low-level resistance to efavirenz (EFV), etravirine (ETR), nevirapine (NVP), and rilpivirine (RPV) (Rhee et al., 2003; Tambuyzer et al., 2009). As for the R-marker present in IN, E157Q is an accessory mutation with little effect by itself on the response to INSTI therapy, conferring potential low-level resistance to elvitegravir (EVG) and raltegravir (RAL) (Anstett et al., 2016; Stanford University, 2022; Charpentier et al., 2018).
Similarly, when analyzing the seven DRM found in ≥25% of the sequences in variants with >5 available PR, RT, or IN sequences, none corresponded to major DRM. Three were R-markers: the previously described accessory DRM to PI, Q58E and K43T, and the accessory DRM to NNRTI V179E. In RT, we identified another accessory DRM to NNRTI, V106I, in sub-subtype F1. This mutation is present in 1–2% of naïve patients and contributes to reduced NNRTI susceptibility combined with other mutations, such as V179D, not found in the available F1 sequences (Rhee et al., 2003; Gatanaga et al., 2010). Alone it has little effect on NNRTI susceptibility conferring potential low-level resistance to doravirine (DOR), ETR, NVP, and RPV (Release Notes—HIV Drug Resistance Database). A62V (sub-subtype A6) is an accessory mutation that often occurs together with the multi-NRTI resistance mutations K65R or Q151M (Svarovskaia et al., 2008). However, these mutations were not found in this subtype among our sequence sets. A62V is widespread in subtype A viruses belonging to the former Soviet Union countries but is otherwise non-polymorphic (Carr et al., 2005). Two accessory DRM to INSTI were detected in IN: G163R, found in two CRFs, and M50I, in six variants. G163R is a non-polymorphic mutation that confers low-level resistance to EVG and RAL and usually appears in combination with N155H (Gatell et al., 2010; Stanford University, 2022), not found in these CRFs. M50I is a polymorphic mutation that may reduce dolutegravir (DTG) susceptibility in combination with R263K (Wares et al., 2014), absent in all the variants carrying M50I.
We present a thorough descriptive analysis of Pol variability among all HIV-1 variants circulating to date. The relatively high aa conservation observed in Pol proteins across HIV-1 variants highlights their critical role in the viral cycle. The variant-specific polymorphisms (V-markers) found in Pol presented little or no predicted impact on clinical ARV efficacy. Our data support previous studies reporting limited evidence of associations between HIV-1 subtypes and treatment failure (Rockstroh et al., 2011; Poon et al., 2019). However, it has been reported that some natural polymorphisms in Pol can promote alternative resistance pathways (Kantor and Katzenstein, 2003; Holguín et al., 2006b; Sanches et al., 2007; Sánchez et al., 2020), affect inhibitor binding (Tran et al., 2020), be present in Pol epitopes interacting with the immune system (Los Alamos National Laboratory, 2022a), or affect protein structure and conformation (Bandaranayake et al., 2008; Coman et al., 2008a,b; Kear et al., 2009). For example, some HIV-1 variants in our study (group O, subtype J, CRF13_cpx, 19_cpx, 49_cpx, and 51_01B, see Table 2) presented V-markers within the PR flaps (PR residues 37-71), the regions mediating accessibility of substrate to the PR active site (Hornak et al., 2006). However, the impact of these V-markers on the flap conformational changes of the corresponding variant is still unknown. Further research is required to evaluate the impact of the different levels of aa conservation in the PR, RT, and IN across HIV-1 variants and to evaluate the influence of each specific V-markers found at Pol in the viral replication cycle, protein structure, and function, as well as in the interactions with antiretroviral drugs or with the immune system.
The original contributions presented in this study are included in the article/Supplementary Material, further inquiries can be directed to the corresponding author. The datasets analyzed for this study can be found in the Los Alamos National Laboratory database (https://www.hiv.lanl.gov).
The viral sequences were retrieved from public databases, and no human studies or animal studies were performed in this manuscript.
PT-H analyzed the HIV Pol LANL sequences, validated some EpiMolBio functions necessary for the sequences analyses, performed the computations, discussed results, and wrote the first version of the manuscript. RR downloaded and aligned the HIV Pol LANL sequences, developed the in-house EpiMolBio bioinformatics program, and validated the EpiMolBio functions necessary for the sequences analyses. AH designed and supervised the study, discussed results, reviewed and edited the manuscript, and applied for funding, being responsible for project administration. All authors approved the submitted final version.
This research was supported by Instituto de Salud Carlos III (PI18/00904 Plan Estatal de Investigación Científica y Técnica y de Innovación 2013–2016) and co-financed by the European Regional Development Fund “A way to achieve Europe” (ERDF). This study was also included in the “Subprograma de Inmigración y Salud” from CIBERESP (Spain). PT-H was funded by ISCIII-Programa Estatal de Promoción del Talento-AES Río Hortega exte. CM19/00057. RR was funded by FONDOS FUR 2020/0285. The funders had no role in the design of the study; in the collection, analyses, or interpretation of data; in the writing of the manuscript; or in the decision to publish the results.
The authors declare that the research was conducted in the absence of any commercial or financial relationships that could be construed as a potential conflict of interest.
All claims expressed in this article are solely those of the authors and do not necessarily represent those of their affiliated organizations, or those of the publisher, the editors and the reviewers. Any product that may be evaluated in this article, or claim that may be made by its manufacturer, is not guaranteed or endorsed by the publisher.
The Supplementary Material for this article can be found online at: https://www.frontiersin.org/articles/10.3389/fmicb.2022.866705/full#supplementary-material
Abecasis, A. B., Vandamme, A.-M., and Lemey, P. (2009). Quantifying differences in the tempo of human immunodeficiency virus type 1 subtype evolution. J. Virol. 83, 12917–12924. doi: 10.1128/JVI.01022-09
Anstett, K., Cutillas, V., Fusco, R., Mesplède, T., and Wainberg, M. A. (2016). Polymorphic substitution E157Q in HIV-1 integrase increases R263K-mediated dolutegravir resistance and decreases DNA binding activity. J. Antimicrob. Chemother. 71, 2083–2088. doi: 10.1093/jac/dkw109
Baeten, J. M., Chohan, B., Lavreys, L., Chohan, V., McClelland, R. S., Certain, L., et al. (2007). HIV-1 subtype D infection is associated with faster disease progression than subtype A in spite of similar plasma HIV-1 loads. J. Infect. Dis. 195, 1177–1180. doi: 10.1086/512682
Bandaranayake, R. M., Prabu-Jeyabalan, M., Kakizawa, J., Sugiura, W., and Schiffer, C. A. (2008). Structural analysis of human immunodeficiency virus type 1 CRF01_AE protease in complex with the substrate p1-p6. J. Virol. 82, 6762–6766. doi: 10.1128/JVI.00018-08
Baxter, J. D., Schapiro, J. M., Boucher, C. A. B., Kohlbrenner, V. M., Hall, D. B., Scherer, J. R., et al. (2006). Genotypic changes in human immunodeficiency virus type 1 protease associated with reduced susceptibility and virologic response to the protease inhibitor tipranavir. J. Virol. 80, 10794–10801. doi: 10.1128/JVI.00712-06
Bbosa, N., Kaleebu, P., and Ssemwanga, D. (2019). HIV subtype diversity worldwide. Curr. Opin. HIV AIDS 14, 153–160. doi: 10.1097/COH.0000000000000534
Bebenek, K., Abbotts, J., Wilson, S. H., and Kunkel, T. A. (1993). Error-prone polymerization by HIV-1 reverse transcriptase. Contribution of template-primer misalignment, miscoding, and termination probability to mutational hot spots. J. Biol. Chem. 268, 10324–10334.
Bennett, D. E., Camacho, R. J., Otelea, D., Kuritzkes, D. R., Fleury, H., Kiuchi, M., et al. (2009). Drug resistance mutations for surveillance of transmitted HIV-1 drug-resistance: 2009 update. PLoS One 4:e4724. doi: 10.1371/journal.pone.0004724
Burgos, M., Llácer, T., Reinosa, R., Rubio-Garrido, M., González, A., and Holguín, A. (2019). “Impaired genotypic resistance interpretation due to HIV-1 variant specific Markers,” in Proceedings of the 10th IAS Conference on HIV Science, Ciudad de México.
Cai, M., Zheng, R., Caffrey, M., Craigie, R., Clore, G. M., and Gronenborn, A. M. (1997). Solution structure of the N-terminal zinc binding domain of HIV-1 integrase. Nat. Struct. Biol. 4, 567–577. doi: 10.1038/nsb0797-567
Carr, J. K., Nadai, Y., Eyzaguirre, L., Saad, M. D., Khakimov, M. M., Yakubov, S. K., et al. (2005). Outbreak of a West African recombinant of HIV-1 in Tashkent, Uzbekistan. J. Acquir. Immune Defic. Syndr. 39, 570–575.
Charpentier, C., Malet, I., Andre-Garnier, E., Storto, A., Bocket, L., Amiel, C., et al. (2018). Phenotypic analysis of HIV-1 E157Q integrase polymorphism and impact on virological outcome in patients initiating an integrase inhibitor-based regimen. J. Antimicrob. Chemother. 73, 1039–1044. doi: 10.1093/jac/dkx511
Clarke, J. R. (2002). Molecular diagnosis of HIV. Expert Rev. Mol. Diagn. 2, 233–239. doi: 10.1586/14737159.2.3.233
Coman, R. M., Robbins, A. H., Fernandez, M. A., Gilliland, C. T., Sochet, A. A., Goodenow, M. M., et al. (2008a). The contribution of naturally occurring polymorphisms in altering the biochemical and structural characteristics of HIV-1 subtype C protease. Biochemistry 47, 731–743. doi: 10.1021/bi7018332
Coman, R. M., Robbins, A. H., Goodenow, M. M., Dunn, B. M., and McKenna, R. (2008b). High-resolution structure of unbound human immunodeficiency virus 1 subtype C protease: implications of flap dynamics and drug resistance. Acta Crystallogr. D Biol. Crystallogr. D 64, 754–763. doi: 10.1107/S090744490801278X
Dam, E., Quercia, R., Glass, B., Descamps, D., Launay, O., Duval, X., et al. (2009). Gag mutations strongly contribute to HIV-1 resistance to protease inhibitors in highly drug-experienced patients besides compensating for fitness loss. PLoS Pathog. 5:e1000345. doi: 10.1371/journal.ppat.1000345
De Leys, R., Vanderborght, B., Vanden Haesevelde, M., Heyndrickx, L., van Geel, A., Wauters, C., et al. (1990). Isolation and partial characterization of an unusual human immunodeficiency retrovirus from two persons of west-central African origin. J. Virol. 64, 1207–1216. doi: 10.1128/JVI.64.3.1207-1216.1990
Desbois, D., Roquebert, B., Peytavin, G., Damond, F., Collin, G., Bénard, A., et al. (2008). In vitro phenotypic susceptibility of human immunodeficiency virus type 2 clinical isolates to protease inhibitors. Antimicrob. Agents Chemother. 52, 1545–1548. doi: 10.1128/AAC.01284-07
Dyda, F., Hickman, A. B., Jenkins, T. M., Engelman, A., Craigie, R., and Davies, D. R. (1994). Crystal structure of the catalytic domain of HIV-1 integrase: similarity to other polynucleotidyl transferases. Science 266, 1981–1986. doi: 10.1126/science.7801124
Eijkelenboom, A. P., Sprangers, R., Hård, K., Puras Lutzke, R. A., Plasterk, R. H., Boelens, R., et al. (1999). Refined solution structure of the C-terminal DNA-binding domain of human immunovirus-1 integrase. Proteins 36, 556–564.
El Safadi, Y., Vivet-Boudou, V., and Marquet, R. (2007). HIV-1 reverse transcriptase inhibitors. Appl. Microbiol. Biotechnol. 75, 723–737. doi: 10.1007/s00253-007-0919-7
Engelman, A. N., and Singh, P. K. (2018). Cellular and molecular mechanisms of HIV-1 integration targeting. Cell. Mol. Life Sci. 75, 2491–2507. doi: 10.1007/s00018-018-2772-5
Eron, J. J. J. (2000). HIV-1 protease inhibitors. Clin. Infect. Dis. 30, (Suppl. 2), S160–S170. doi: 10.1086/313853
Frankel, A. D., and Young, J. A. (1998). HIV-1: fifteen proteins and an RNA. Annu. Rev. Biochem. 67, 1–25. doi: 10.1146/annurev.biochem.67.1.1
Gatanaga, H., Ode, H., Hachiya, A., Hayashida, T., Sato, H., and Oka, S. (2010). Combination of V106I and V179D polymorphic mutations in human immunodeficiency virus type 1 reverse transcriptase confers resistance to efavirenz and nevirapine but not etravirine. Antimicrob. Agents Chemother. 54, 1596–1602. doi: 10.1128/AAC.01480-09
Gatell, J. M., Katlama, C., Grinsztejn, B., Eron, J. J., Lazzarin, A., Vittecoq, D., et al. (2010). Long-term efficacy and safety of the HIV integrase inhibitor raltegravir in patients with limited treatment options in a Phase II study. J. Acquir. Immune Defic. Syndr. 53, 456–463. doi: 10.1097/qai.0b013e3181c9c967
Goodenow, M. M., Bloom, G., Rose, S. L., Pomeroy, S. M., O’Brien, P. O., Perez, E. E., et al. (2002). Naturally occurring amino acid polymorphisms in human immunodeficiency virus type 1 (HIV-1) Gag p7(NC) and the C-cleavage site impact Gag-Pol processing by HIV-1 protease. Virology 292, 137–149. doi: 10.1006/viro.2001.1184
Gu, S.-X., Zhu, Y.-Y., Wang, C., Wang, H.-F., Liu, G.-Y., Cao, S., et al. (2020). Recent discoveries in HIV-1 reverse transcriptase inhibitors. Curr. Opin. Pharmacol. 54, 166–172. doi: 10.1016/j.coph.2020.09.017
Hemelaar, J. (2012). The origin and diversity of the HIV-1 pandemic. Trends Mol. Med. 18, 182–192. doi: 10.1016/j.molmed.2011.12.001
Hemelaar, J., Elangovan, R., Yun, J., Dickson-Tetteh, L., Fleminger, I., Kirtley, S., et al. (2019). Global and regional molecular epidemiology of HIV-1, 1990-2015: a systematic review, global survey, and trend analysis. Lancet Infect. Dis. 19, 143–155. doi: 10.1016/S1473-3099(18)30647-9
Holguín, A., Alvarez, A., and Soriano, V. (2006a). Variability in the P6gag domains of HIV-1 involved in viral budding. AIDS 20, 624–627. doi: 10.1097/01.aids.0000210619.75707.21
Holguín, A., Ramirez de Arellano, E., Rivas, P., and Soriano, V. (2006b). Efficacy of antiretroviral therapy in individuals infected with HIV-1 non-B subtypes. AIDS Rev. 8, 98–107.
Holguín, A., Paxinos, E., Hertogs, K., Womac, C., and Soriano, V. (2004). Impact of frequent natural polymorphisms at the protease gene on the in vitro susceptibility to protease inhibitors in HIV-1 non-B subtypes. J. Clin. Virol. 31, 215–220. doi: 10.1016/j.jcv.2004.03.015
Holguín, A., and Soriano, V. (2002). Resistance to antiretroviral agents in individuals with HIV-1 non-B subtypes. HIV Clin. Trials 3, 403–411. doi: 10.1310/7bwp-0x7f-nxna-qrnp
Hornak, V., Okur, A., Rizzo, R. C., and Simmerling, C. (2006). HIV-1 protease flaps spontaneously open and reclose in molecular dynamics simulations. Proc. Natl. Acad. Sci. U.S.A. 103, 915–920. doi: 10.1073/pnas.0508452103
Hu, W.-S., and Hughes, S. H. (2012). HIV-1 reverse transcription. Cold Spring Harb. Perspect. Med. 2:a006882. doi: 10.1101/cshperspect.a006882
Huff, J. R. (1991). HIV protease: a novel chemotherapeutic target for AIDS. J. Med. Chem. 34, 2305–2314. doi: 10.1021/jm00112a001
Jóźwik, I. K., Passos, D. O., and Lyumkis, D. (2020). Structural biology of HIV integrase strand transfer inhibitors. Trends Pharmacol. Sci. 41, 611–626. doi: 10.1016/j.tips.2020.06.003
Kabat, E. A., Wu, T. T., and Bilofsky, H. (1977). Unusual distributions of amino acids in complementarity-determining (hypervariable) segments of heavy and light chains of immunoglobulins and their possible roles in specificity of antibody-combining sites. J. Biol. Chem. 252, 6609–6616.
Kantor, R. (2006). Impact of HIV-1 pol diversity on drug resistance and its clinical implications. Curr. Opin. Infect. Dis. 19, 594–606. doi: 10.1097/QCO.0b013e3280109122
Kantor, R., and Katzenstein, D. (2003). Polymorphism in HIV-1 non-subtype B protease and reverse transcriptase and its potential impact on drug susceptibility and drug resistance evolution. AIDS Rev. 5, 25–35.
Kantor, R., Katzenstein, D. A., Efron, B., Carvalho, A. P., Wynhoven, B., Cane, P., et al. (2005). Impact of HIV-1 subtype and antiretroviral therapy on protease and reverse transcriptase genotype: results of a global collaboration. PLoS Med. 2:e112. doi: 10.1371/journal.pmed.0020112
Kear, J. L., Blackburn, M. E., Veloro, A. M., Dunn, B. M., and Fanucci, G. E. (2009). Subtype polymorphisms among HIV-1 protease variants confer altered flap conformations and flexibility. J. Am. Chem. Soc. 131, 14650–14651. doi: 10.1021/ja907088a
Konvalinka, J., Kräusslich, H.-G., and Müller, B. (2015). Retroviral proteases and their roles in virion maturation. Virology 479-480, 403–417. doi: 10.1016/j.virol.2015.03.021
Li, G., Verheyen, J., Rhee, S.-Y., Voet, A., Vandamme, A.-M., and Theys, K. (2013). Functional conservation of HIV-1 Gag: implications for rational drug design. Retrovirology 10:126. doi: 10.1186/1742-4690-10-126
Linchangco, G. V. J., Foley, B., and Leitner, T. (2021). Updated HIV-1 consensus sequences change but stay within similar distance from worldwide samples. Front. Microbiol. 12:828765. doi: 10.3389/fmicb.2021.828765
Llacer Delicado, T., Torrecilla, E., and Holguin, A. (2016). Deep analysis of HIV-1 natural variability across HIV-1 variants at residues associated with integrase inhibitor (INI) resistance in INI-naive individuals. J. Antimicrob. Chemother. 71, 362–366. doi: 10.1093/jac/dkv333
Los Alamos National Laboratory (2021). HIV Circulating Recombinant Forms (CRFs). Available Online at: https://www.hiv.lanl.gov/content/sequence/HIV/CRFs/CRFs.html [accessed January 10, 2022].
Los Alamos National Laboratory (2022a). Interactive Epitope Maps. Available Online at: https://www.hiv.lanl.gov/content/immunology/maps/maps.html [accessed May 24, 2022].
Los Alamos National Laboratory (2022b). Main Search Interface of HIV Sequence Database. Available Online at: https://www.hiv.lanl.gov/components/sequence/HIV/search/search.html [accessed May 24, 2022].
Menéndez-Arias, L., and Álvarez, M. (2014). Antiretroviral therapy and drug resistance in human immunodeficiency virus type 2 infection. Antiviral Res. 102, 70–86. doi: 10.1016/j.antiviral.2013.12.001
Myint, L., Matsuda, M., Matsuda, Z., Yokomaku, Y., Chiba, T., Okano, A., et al. (2004). Gag non-cleavage site mutations contribute to full recovery of viral fitness in protease inhibitor-resistant human immunodeficiency virus type 1. Antimicrob. Agents Chemother. 48, 444–452. doi: 10.1128/AAC.48.2.444-452.2004
Nagata, S., Imai, J., Makino, G., Tomita, M., and Kanai, A. (2017). Evolutionary analysis of HIV-1 pol proteins reveals representative residues for viral subtype differentiation. Front. Microbiol. 8:2151. doi: 10.3389/fmicb.2017.02151
Navia, M. A., Fitzgerald, P. M., McKeever, B. M., Leu, C. T., Heimbach, J. C., Herber, W. K., et al. (1989). Three-dimensional structure of aspartyl protease from human immunodeficiency virus HIV-1. Nature 337, 615–620. doi: 10.1038/337615a0
Ng, O. T., Laeyendecker, O., Redd, A. D., Munshaw, S., Grabowski, M. K., Paquet, A. C., et al. (2014). HIV type 1 polymerase gene polymorphisms are associated with phenotypic differences in replication capacity and disease progression. J. Infect. Dis. 209, 66–73. doi: 10.1093/infdis/jit425
NIH FDA-Approved HIV Medicines (2022). Available Online at: https://hivinfo.nih.gov/understanding-hiv/fact-sheets/fda-approved-hiv-medicines [accessed January 20, 2022].
Nijhuis, M., Schuurman, R., de Jong, D., Erickson, J., Gustchina, E., Albert, J., et al. (1999). Increased fitness of drug resistant HIV-1 protease as a result of acquisition of compensatory mutations during suboptimal therapy. AIDS 13, 2349–2359. doi: 10.1097/00002030-199912030-00006
Plantier, J.-C., Leoz, M., Dickerson, J. E., De Oliveira, F., Cordonnier, F., Lemée, V., et al. (2009). A new human immunodeficiency virus derived from gorillas. Nat. Med. 15, 871–872. doi: 10.1038/nm.2016
Poon, A. F. Y., Ndashimye, E., Avino, M., Gibson, R., Kityo, C., Kyeyune, F., et al. (2019). First-line HIV treatment failures in non-B subtypes and recombinants: a cross-sectional analysis of multiple populations in Uganda. AIDS Res. Ther. 16:3. doi: 10.1186/s12981-019-0218-2
Release Notes - HIV Drug Resistance Database (2020). Available Online at: https://hivdb.stanford.edu/page/release-notes/ (accessed May 20, 2020).
Renjifo, B., Gilbert, P., Chaplin, B., Msamanga, G., Mwakagile, D., Fawzi, W., et al. (2004). Preferential in-utero transmission of HIV-1 subtype C as compared to HIV-1 subtype A or D. AIDS 18, 1629–1636. doi: 10.1097/01.aids.0000131392.68597.34
Rhee, S.-Y., Gonzales, M. J., Kantor, R., Betts, B. J., Ravela, J., and Shafer, R. W. (2003). Human immunodeficiency virus reverse transcriptase and protease sequence database. Nucleic Acids Res. 31, 298–303. doi: 10.1093/nar/gkg100
Rhee, S.-Y., Sankaran, K., Varghese, V., Winters, M. A., Hurt, C. B., Eron, J. J., et al. (2016). HIV-1 protease, reverse transcriptase, and integrase variation. J. Virol. 90, 6058–6070. doi: 10.1128/JVI.00495-16
Rhee, S.-Y., Taylor, J., Fessel, W. J., Kaufman, D., Towner, W., Troia, P., et al. (2010). HIV-1 protease mutations and protease inhibitor cross-resistance. Antimicrob. Agents Chemother. 54, 4253–4261. doi: 10.1128/AAC.00574-10
Rice, P., Craigie, R., and Davies, D. R. (1996). Retroviral integrases and their cousins. Curr. Opin. Struct. Biol. 6, 76–83. doi: 10.1016/s0959-440x(96)80098-4
Roberts, J. D., Bebenek, K., and Kunkel, T. A. (1988). The accuracy of reverse transcriptase from HIV-1. Science 242, 1171–1173. doi: 10.1126/science.2460925
Robertson, D. L., Anderson, J. P., Bradac, J. A., Carr, J. K., Foley, B., Funkhouser, R. K., et al. (2000). HIV-1 nomenclature proposal. Science 288, 55–56. doi: 10.1126/science.288.5463.55d
Rockstroh, J. K., Teppler, H., Zhao, J., Sklar, P., Miller, M. D., Harvey, C. M., et al. (2011). Clinical efficacy of raltegravir against B and non-B subtype HIV-1 in phase III clinical studies. AIDS 25, 1365–1369. doi: 10.1097/QAD.0b013e328348065a
Rodgers, D. W., Gamblin, S. J., Harris, B. A., Ray, S., Culp, J. S., Hellmig, B., et al. (1995). The structure of unliganded reverse transcriptase from the human immunodeficiency virus type 1. Proc. Natl. Acad. Sci. U.S.A. 92, 1222–1226. doi: 10.1073/pnas.92.4.1222
Salminen, M. O., Ehrenberg, P. K., Mascola, J. R., Dayhoff, D. E., Merling, R., Blake, B., et al. (2000). Construction and biological characterization of infectious molecular clones of HIV-1 subtypes B and E (CRF01_AE) generated by the polymerase chain reaction. Virology 278, 103–110. doi: 10.1006/viro.2000.0640
Sanches, M., Krauchenco, S., Martins, N. H., Gustchina, A., Wlodawer, A., and Polikarpov, I. (2007). Structural characterization of B and non-B subtypes of HIV-protease: insights into the natural susceptibility to drug resistance development. J. Mol. Biol. 369, 1029–1040. doi: 10.1016/j.jmb.2007.03.049
Sánchez, D., Arazi Caillaud, S., Zapiola, I., Fernandez Giuliano, S., Bologna, R., Mangano, A., et al. (2020). Impact of genotypic diversity on selection of subtype-specific drug resistance profiles during raltegravir-based therapy in individuals infected with B and BF recombinant HIV-1 strains. J. Antimicrob. Chemother. 75, 1567–1574. doi: 10.1093/jac/dkaa042
Shafer, R. W., and Schapiro, J. M. (2008). HIV-1 drug resistance mutations: an updated framework for the second decade of HAART. AIDS Rev. 10, 67–84.
Sharma, A., Slaughter, A., Jena, N., Feng, L., Kessl, J. J., Fadel, H. J., et al. (2014). A new class of multimerization selective inhibitors of HIV-1 integrase. PLoS Pathog. 10:e1004171. doi: 10.1371/journal.ppat.1004171
Simon, F., Mauclère, P., Roques, P., Loussert-Ajaka, I., Müller-Trutwin, M. C., Saragosti, S., et al. (1998). Identification of a new human immunodeficiency virus type 1 distinct from group M and group O. Nat. Med. 4, 1032–1037. doi: 10.1038/2017
Sliepen, K., Han, B. W., Bontjer, I., Mooij, P., Garces, F., Behrens, A.-J., et al. (2019). Structure and immunogenicity of a stabilized HIV-1 envelope trimer based on a group-M consensus sequence. Nat. Commun. 10:2355. doi: 10.1038/s41467-019-10262-5
Stanford University (2022). HIV Drug Resistance Database. Available online at: https://hivdb.stanford.edu/ (accessed June 16, 2022).
Svarovskaia, E. S., Feng, J. Y., Margot, N. A., Myrick, F., Goodman, D., Ly, J. K., et al. (2008). The A62V and S68G mutations in HIV-1 reverse transcriptase partially restore the replication defect associated with the K65R mutation. J. Acquir. Immune Defic. Syndr. 48, 428–436. doi: 10.1097/QAI.0b013e31817bbe93
Tambuyzer, L., Azijn, H., Rimsky, L. T., Vingerhoets, J., Lecocq, P., Kraus, G., et al. (2009). Compilation and prevalence of mutations associated with resistance to non-nucleoside reverse transcriptase inhibitors. Antivir. Ther. 14, 103–109.
Torrecilla, E., Llácer Delicado, T., and Holguín, Á. (2014). New findings in cleavage sites variability across groups, subtypes and recombinants of human immunodeficiency virus type 1. PLoS One 9:e88099. doi: 10.1371/journal.pone.0088099
Tran, T. T., Liu, Z., and Fanucci, G. E. (2020). Conformational landscape of non-B variants of HIV-1 protease: a pulsed EPR study. Biochem. Biophys. Res. Commun. 532, 219–224. doi: 10.1016/j.bbrc.2020.08.030
Troyano-Hernáez, P., Reinosa, R., Burgos, M. C., and Holguín, Á. (2021a). Short communication: update in natural antiretroviral resistance-associated mutations among HIV type 2 variants and discrepancies across HIV type 2 resistance interpretation tools. AIDS Res. Hum. Retroviruses 37, 793–795. doi: 10.1089/AID.2020.0180
Troyano-Hernáez, P., Reinosa, R., and Holguín, Á. (2021b). Evolution of SARS-CoV-2 envelope, membrane, nucleocapsid, and spike structural proteins from the beginning of the pandemic to September 2020: a global and regional approach by epidemiological week. Viruses 13:243. doi: 10.3390/v13020243
Troyano-Hernáez, P., Reinosa, R., and Holguín, Á. (2019). “Marcadores genéticos en la proteína de la Cápside p24 en los grupos, subtipos, sub-subtipos y recombinantes del VIH-1,” in Proceedings of the XI CONGRESO NACIONAL GeSIDA, Toledo, 124–125.
Troyano-Hernáez, P., Reinosa, R., and Holguín, Á. (2020). “Mutaciones en la proteína Spike de SARS-CoV-2 por Comunidades Autónomas en secuencias españolas recogidas hasta junio 2020,” in Proceedings of the I Congreso Nacional COVID-19, Toledo, 76.
Troyano-Hernáez, P., Reinosa, R., and Holguín, Á. (2022). HIV capsid protein genetic diversity across HIV-1 variants and impact on new capsid-inhibitor lenacapavir. Front. Microbiol. 13:854974. doi: 10.3389/fmicb.2022.854974
Tuaillon, E., Gueudin, M., Lemee, V., Gueit, I., Roques, P., Corrigan, G. E., et al. (2004). Phenotypic susceptibility to nonnucleoside inhibitors of virion-associated reverse transcriptase from different HIV types and groups. J. Acquir. Immune Defic. Syndr. 37, 1543–1549. doi: 10.1097/00126334-200412150-00001
Turner, D., Roldan, A., Brenner, B., Moisi, D., Routy, J.-P., and Wainberg, M. A. (2004). Variability in the PR and RT genes of HIV-1 isolated from recently infected subjects. Antivir. Chem. Chemother. 15, 255–259. doi: 10.1177/095632020401500504
Wares, M., Mesplède, T., Quashie, P. K., Osman, N., Han, Y., and Wainberg, M. A. (2014). The M50I polymorphic substitution in association with the R263K mutation in HIV-1 subtype B integrase increases drug resistance but does not restore viral replicative fitness. Retrovirology 11:7. doi: 10.1186/1742-4690-11-7
Wensing, A. M., Calvez, V., Ceccherini-Silberstein, F., Charpentier, C., Gunthard, H. F., Paredes, R., et al. (2019). 2019 update of the drug resistance mutations in HIV-1. Top. Antivir. Med. 27, 111–121.
Yamaguchi, J., Vallari, A., McArthur, C., Sthreshley, L., Cloherty, G. A., Berg, M. G., et al. (2020). Brief report: complete genome sequence of CG-0018a-01 establishes HIV-1 subtype L. J. Acquir. Immune Defic. Syndr. 83, 319–322. doi: 10.1097/QAI.0000000000002246
Yebra, G., de Mulder, M., del Romero, J., Rodríguez, C., and Holguín, A. (2010). HIV-1 non-B subtypes: high transmitted NNRTI-resistance in Spain and impaired genotypic resistance interpretation due to variability. Antiviral Res. 85, 409–417. doi: 10.1016/j.antiviral.2009.11.010
Zhang, Y., Murakoshi, H., Chikata, T., Akahoshi, T., Van Tran, G., Nguyen, T. V., et al. (2021). Effect of difference in consensus sequence between HIV-1 subtype A/E and subtype B viruses on elicitation of gag-specific CD8(+) T cells and accumulation of HLA-associated escape mutations. J. Virol. 95:e02061-20. doi: 10.1128/JVI.02061-20
Keywords: HIV-1, Pol, protease, integrase, conservation, variants, resistance, reverse transcriptase
Citation: Troyano-Hernáez P, Reinosa R and Holguín A (2022) Genetic Diversity and Low Therapeutic Impact of Variant-Specific Markers in HIV-1 Pol Proteins. Front. Microbiol. 13:866705. doi: 10.3389/fmicb.2022.866705
Received: 31 January 2022; Accepted: 06 June 2022;
Published: 14 July 2022.
Edited by:
Joris Hemelaar, University of Oxford, United KingdomReviewed by:
Diako Ebrahimi, Texas Biomedical Research Institute, United StatesCopyright © 2022 Troyano-Hernáez, Reinosa and Holguín. This is an open-access article distributed under the terms of the Creative Commons Attribution License (CC BY). The use, distribution or reproduction in other forums is permitted, provided the original author(s) and the copyright owner(s) are credited and that the original publication in this journal is cited, in accordance with accepted academic practice. No use, distribution or reproduction is permitted which does not comply with these terms.
*Correspondence: Africa Holguín, YWZyaWNhLmhvbGd1aW5Ac2FsdWQubWFkcmlkLm9yZw==
Disclaimer: All claims expressed in this article are solely those of the authors and do not necessarily represent those of their affiliated organizations, or those of the publisher, the editors and the reviewers. Any product that may be evaluated in this article or claim that may be made by its manufacturer is not guaranteed or endorsed by the publisher.
Research integrity at Frontiers
Learn more about the work of our research integrity team to safeguard the quality of each article we publish.