- 1Gene Engineering Laboratory, Feed Research Institute, Chinese Academy of Agricultural Sciences, Beijing, China
- 2Innovative Team of Antimicrobial Peptides and Alternatives to Antibiotics, Feed Research Institute, Chinese Academy of Agricultural Sciences, Beijing, China
- 3Key Laboratory of Feed Biotechnology, Ministry of Agriculture and Rural Affairs, Beijing, China
Staphylococcus aureus (S. aureus) is one of the most common pathogenic bacteria responsible for causing a life-threatening peritonitis disease. NZX, as a variant of fungal defensin plectasin, displayed potent antibacterial activity against S. aureus. In this study, the antibacterial and resistance characteristics, pharmacokinetics, and pharmacodynamics of NZX against the S. aureus E48 and S. aureus E48-induced mouse peritonitis model were studied, respectively. NZX exhibited a more rapid killing activity to S. aureus (minimal inhibitory concentration, 1 μg/ml) compared with linezolid, ampicillin and daptomycin, and serial passaging of S. aureus E48 for 30 days at 1/2 × MIC, NZX had a lower risk of resistance compared with ampicillin and daptomycin. Also, it displayed a high biocompatibility and tolerance to physiological salt, serum environment, and phagolysosome proteinase environment, except for acid environment in phagolysosome. The murine serum protein-binding rate of NZX was 89.25% measured by ultrafiltration method. Based on the free NZX concentration in serum after tail vein administration, the main pharmacokinetic parameters for T1/2, Cmax, Vd, MRT, and AUC ranged from 0.32 to 0.45 h, 2.85 to 20.55 μg/ml, 1469.10 to 2073.90 ml/kg, 0.32 to 0.56 h, and 1.11 to 8.89 μg.h/ml, respectively. Additionally, the in vivo pharmacodynamics against S. aureus demonstrated that NZX administrated two times by tail vein at 20 mg/kg could rescue all infected mice in the lethal mouse peritonitis model. And NZX treatment (20 mg/kg) significantly reduced CFU counts in the liver, lung, and spleen, especially for intracellular bacteria in the peritoneal fluid, which were similar or superior to those of daptomycin. In vivo efficacies of NZX against total bacteria and intracellular bacteria were significantly correlated with three PK/PD indices of ƒAUC/MIC, ƒCmax/MIC, and ƒT% > MIC analyzed by a sigmoid maximum-effect model. These results showed that NZX may be a potential candidate for treating peritonitis disease caused by intracellular S. aureus.
Introduction
Peritonitis is a life-threatening infectious complication encountered in patients, pets, and livestock, mainly from the process of gastrointestinal perforation, intra-abdominal surgery, and peritoneal dialysis, being as some prerequisites for microbes to enter the abdominal cavity (Broughton et al., 2010; Pörner et al., 2021). Staphylococcus aureus (S. aureus) is one of the most common pathogens associated with peritonitis (Salzer, 2018; Marciano et al., 2019; Szeto et al., 2021). Although antibiotic therapy is a common strategy for eliminating bacterial infection, peritonitis caused by S. aureus remains a major complication of peritoneal dialysis with the characteristics of persistence and recurrence (Marciano et al., 2019; Alonso et al., 2021; Szeto et al., 2021). One of the major causes of recurrent peritonitis is from the emergence of antibacterial resistance during antibiotic treatment, especially from methicillin-resistant and multiple resistant strains (Li et al., 2020a; Camargo et al., 2021). In addition, S. aureus can invade and replicate in various host cells, complicating the usage of antibiotics (Zhou et al., 2018; Wang et al., 2021); therapeutic effects were impeded by poor penetration or inferior intracellular stability. Therefore, there is an urgent need to develop new intracellular antibacterial drugs with low resistance and high cellular permeability for the treatment or prevention of S. aureus-related peritonitis diseases.
Antimicrobial peptides (AMPs) possess the advantages of rapid killing, high specificity, and low resistance to pathogens compared to other small molecule drugs (Liu et al., 2021; Moretta et al., 2021; Rima et al., 2021). Additionally, AMPs proved to be potent in inhibiting or eradicating intracellular organisms (Brinch et al., 2009; Wang et al., 2018, 2019; Li et al., 2020b, 2021). Also, the iron triangle theory on health protection from AMPs, antibiotics, and vaccines for maintaining a reasonable equilibrium among pathogens, antimicrobials, and drug resistances has been recently put forward (Zheng et al., 2021; Hao et al., 2022). Given those merits, AMPs have been considered as promising and new therapeutic anti-infection candidates, and should exert more roles in health practices of humans and animals. However, to date, very few new drug applications of AMPs have successfully passed official approval and entered into clinical application despite a lot of AMP-related patents and papers achieved (Mercer and O'Neil, 2013; Costa et al., 2019). One main obstacle is the instability of AMPs due to their fast metabolism in host body; this is a double-edged sword in terms of methodology in evaluation detection and reality in safety monitoring; on one hand, AMPs are hardly accumulated in the host body, thereby reducing drug residues and resistance as their merits (Henninot et al., 2018; Costa et al., 2019); on another hand, their metabolites or small segments in distribution details in a biological matrix are hardly usually detected by modern bioanalytical detection methods (Ewles and Goodwin, 2011). It is known that small molecular AMPs are rapidly metabolized in vivo and degraded into smaller peptide fragments or amino acids and absorbed as nutrients; thus, this situation becomes the blind zone for detection and characterization in trial research and licensing evaluation, although the safety of the degradation products of AMPs in vivo is unquestionable by general knowledge of nutrition metabolism but very difficult for demonstration via analytical detection. Surely, some new principles with more suitable for AMPs are urgent in need, specially for their clinical evaluation protocol. Usually, ELISA and LC–MS/MS analysis methods have been applied for quantifying typical peptides in biological samples (Ewles and Goodwin, 2011; Mercer and O'Neil, 2013), but not suitable for polar small AMPs. The microbiological assay (Chinese Veterinary Pharmacopeia, 2015, Appendix 1201), as a quantity- and function-based dual-label detection method, gives us a different solution facing above dilemma choice; it can concur and merge the determination of drug concentration and its antibacterial activity in biological samples, thus has been chosen for drug pharmacokinetic studies on AMPs, although it cannot clearly differentiate between a prototype drug and its active metabolites (Leroy et al., 1989; Jacobson et al., 2005; Andes et al., 2009; Hengzhuang et al., 2012; Giguère et al., 2017); in fact, it is well-suitable for characterizing the small molecular AMPs with a consensus among researcher peers. Gladly, similar supportive information can be seen from the regulation “Requirements for Application Materials of New Feed Additives of Announcement No. 226 of the Ministry of Agriculture and Rural Affairs of the P. R. China (MARA)”; if the compound or metabolic residue is a normal component of animal body fluid or tissue, or it is absorbed as nutrients according to the physiological mode and level of compounds in vivo, there is no need to evaluate its metabolism and residue in detail. This rule should also be invoked or extrapolated to the evaluation of the pharmacokinetics of AMPs. This would offer a wise way for most small molecular peptides, including AMPs during their access to legal official recognition via clinical evaluation. It is believed that the licensing authorities as CFDA, MARA, FDA, EMA, and PMDA would optimize the relative regulations to overcome the above difficulties, and the effective combination of the multiple pharmacokinetics analytical methods as ELISA, HPLC, LC–MS/MS, microbiological assay, and in vivo imaging might be expected.
Plectasin (MW 4398.80 Da) isolated from Pseudoplectania nigrella has potent antimicrobial activity against gram-positive bacteria in vitro and in vivo, especially for Streptococcus pneumoniae, which is superior to vancomycin and penicillin (Mygind et al., 2005; Schneider et al., 2010). However, plectasin had some limitations before clinical application, such as cytotoxicity and trypsin instability (Yang et al., 2019). NZX (MW 4383.89 Da), a variant of plectasin with three mutation sites (D9S, M13I, and K32R), had the increased net charge (from +1 to +2) and hydrophobicity (from −0.695 to −0.578) (Liu et al., 2020). Compared with rifampicin, NZX displayed more potent antibacterial activity against multi-drug resistant Mycobacterium tuberculosis (M. tuberculosis) (Tenland et al., 2018, 2019). NZX displayed a stronger activity against Staptococcus hyicus (S. hyicus) NCTC 10350 than ceftriaxone both in vitro and in vivo (Liu et al., 2020). In addition, NZX had low cytotoxicity, and good temperature, pH, and protease stability (Tenland et al., 2018; Liu et al., 2020). More importantly, NZX possessed the ability to induce intracellular killing of M. tuberculosis in human macrophages (Tenland et al., 2018) and S. hyicus NCTC 10350 in Hacat cells (Liu et al., 2020); thus, it provides a potential for the treatment of peritonitis diseases caused by intracellular bacterial infection.
In this study, the pharmacodynamics (PD) and pharmacokinetics (PK) of NZX against the S. aureus-induced mouse peritonitis model were built and evaluated for the first time; the main pharmacokinetic parameters and the relationships between PK/PD parameters and in vivo efficacies of NZX against total bacteria and intracellular bacteria were obtained, which will contribute to further clinical evaluation and application.
Materials and Methods
Chemicals, Organisms and Cell Line
NZX was expressed by Pichia pastoris X-33 and purified as described previously (Liu et al., 2020) (>90% purity). Antibacterial agents, including clindamycin, linezolid, daptomycin, and penicillin, were purchased from the China Institute of Veterinary Drug Control (Beijing, China), Dalian Meilun Biotech (Dalian, China), and Sangon Biotech (Shanghai, China) Co., Ltd. The clinical bovine mastitis-isolated S. aureus E48 was provided by Professor Xin Zhao, College of Animal Science and Technology, Northwest A&F University (Yangling, China). S. aureus MSSA ATCC 25923 and S. aureus MRSA ATCC 43300 were purchased from the American Type Culture Collection. The RAW 264.7 cell line was supplied by Peking Union Medical College.
Minimal Inhibitory Concentrations (MICs) Against S. aureus
The MICs of NZX and other antibacterial agents against S. aureus strains were conducted according to the Clinical Laboratory Standards Institute (Watts et al., 2013). In brief, a volume of 90 μl of log-phase S. aureus E48 with a density of 1 × 105 CFU/ml was co-incubated with 10 μl of NZX and antibiotics at final concentrations of 0.5–128 μg/ml and 0.125–64 μg/ml, respectively, including clindamycin, linezolid, daptomycin (with 1.25 mM of calcium chloride for daptomycin), and penicillin for 16–18 h at 37°C in a 96-well microplate. The wells containing antibiotics were set as positive controls. Negative control wells contained a PBS buffer and bacterial suspension without antibacterial agents. The MIC value was defined as the lowest concentration of antibacterial agents at which no visible bacteria were observed. All MIC assays were performed independently in triplicate.
Bactericidal Activity and Drug Resistance Experiments
Survival rate and time-killing kinetic curves experiments were conducted as described previously (Li et al., 2020b). Log-phase S. aureus E48 cells with a density of 1 × 105 CFU/ml were treated with NZX, clindamycin, linezolid, daptomycin (with 1.25 mM of calcium chloride for daptomycin), and ampicillin at 1/2 × MIC, 1 × MIC, and 2 × MIC, respectively. After incubation at various times (0, 15, 30, 60, 90, and 120 min), a volume of 100 μl of bacterial suspension was taken out from a flask and diluted to count. All assays were conducted independently in triplicate.
The development of drug resistance of S. aureus E48 induced by NZX and antibiotics was conducted as described previously (Li et al., 2017). The MICs of antibacterial agents were measured according to the MICs testing method as described above. After 16–18-h incubation at 37°C, the bacterial suspensions mixed with NZX, clindamycin, linezolid, daptomycin, and ampicillin at the sub-MIC were collected and transferred to 10 ml of an MHB medium until logarithmic growth. Then, the MIC values of the next passage were conducted again, and the experimental procedures were repeated for 30 days. The development of drug resistance was determined by the fold changes in MICs.
Stability Under Different Physiological Environments
The stability of NZX in the mouse serum was determined when placed at 37°C (Wang et al., 2020). The stock mixture from 25% serum was spiked with 100 μg/ml NZX and vortexed well. The mixtures were incubated at 37°C. At 0, 4, 8, 12, and 24 h, samples were taken out, extracted, and analyzed by reverse-phase high-performance liquid chromatography (RP-HPLC). The results were expressed as the ratio of the peak area of the peptide at various times to that of the initial incubation time.
The physiological salt and serum stability of NZX were performed according to the previous report (Hirsch et al., 2019). In detail, the MIC values of NZX for S. aureus E48 were also determined in the MHB medium adjusted to 150-mM NaCl or 1.25-mM CaCl2, as well as in the MHB medium added into 25% (v/v) sterile mouse serum or in the MHB medium adjusted to 25% (v/v) mouse serum and supplemented with 150-mM NaCl, which represent the physiological salt and serum concentrations, respectively.
To test the effect of phagolysosomal enzyme-cathepsin B (from bovine spleen, Sigma C7800) on the antibacterial activities of peptides, NZX was diluted to 640 μg/ml in a cathepsin buffer (5-mM L-cysteine, 20-mM sodium acetate, 1-mM EDTA, pH of 5.0) and incubated with 16 μg/ml enzyme solution at 37°C for 1 h. The MIC value of NZX against S. aureus E48 was examined. NZX without enzyme solution was set as the negative control. Additionally, to mimic the intracellular condition, the MHB medium was adjusted to a specific pH value of 5.0 to determine the MIC value of NZX against S. aureus E48 in the acidic environment (Wang et al., 2019). In all experiments, clindamycin, linezolid, daptomycin, and ampicillin acted as antibiotic control.
Cytotoxicity, Intracellular Inhibition, and Internalization Assays
The viability of RAW 264.7 cells was determined upon exposure to NZX by a colorimetric 3-(4, 5-dimethyl-2-thiazolyl)-2, 5-diphenyl-2-H-tetrazolium bromide (MTT) method as described previously (Li et al., 2021). All assays were repeated six times. The cell survival rate was calculated using the following formula:
The antibacterial effects of NZX against intracellular S. aureus E48 in RAW 264.7 were determined according to a previous study (Wang et al., 2018). The antibacterial concentrations of NZX against S. aureus E48 were set as 1, 4, 16, 64, and 128 μg/ml. Daptomycin was selected as a control because it is a polypeptide drug similar to NZX. In addition, the internalization and distribution assays of NZX in RAW 264.7 were conducted according to Wang et al. (2019). Briefly, RAW 264.7 cells were grown on culture dishes at 37°C for 24 h. FITC or FITC-NZX (1, 16, and 64 μg/ml) was added and incubated in the culture dishes for 24 h. The cells were washed two times with PBS and quenched by 0.04% trypan blue. Samples were analyzed by flow cytometry to determine the uptake of NZX. In distribution analysis, FITC or FITC-NZX at 64 μg/ml was added and incubated in the culture dishes for 24 h. The cells were washed two times with PBS, stained with 5 μg/ml wheat germ agglutinin (WGA)-conjugated Alexa Fluor 555 (ThermoFisher Scientific, USA) and Hoechst 33342 (ThermoFisher Scientific, USA) for 10 min, and observed by confocal microscopy (Leica TCS SP 5, Germany).
Pharmacokinetics of NZX in Non-Infected Mice
The 6-week-old, specific-pathogen-free, female ICR mice (starting weight, 23 to 27 g) were purchased from Beijing Vital River Laboratory Animal Technology Co., Ltd (Beijing, China). The animals were acclimatized for 2 to 3 days before the experiment. The pharmacokinetics of NZX was evaluated in uninfected female ICR mice that received a single tail vein NZX dose of 5, 10, or 20 mg/kg, respectively. After NZX administration, blood samples were collected by orbital venous from five mice per time point at 0.083 to 2 h intervals over 6 h. After centrifugation at 4,000 rpm for 20 min at 4°C, the serum was immediately separated and frozen at −80°C until analysis. Analyses of total serum concentrations of NZX were conducted by a microbiologic assay using S. aureus ATCC 6538p (CICC 10307) as the test strain (Andes et al., 2009). The developed microbial method proved to be simple, precise, and stable. The limit of quantification (LOQ) and detection (LOD) in the microbiologic assay were 0.5 μg/ml and 0.25 μg/ml, respectively. The protein binding of NZX in serum was measured by the ultrafiltration method using Centrifree Filters (Merck Millipore Ltd., Product code: 4104), according to previously described methods by Brinch et al. (2009). The concentration of NZX in the ultrafiltrate was determined by HPLC methods.
Pharmacodynamics of NZX in the Mouse Peritonitis Model
Each mouse was received a peritonitis by intraperitoneal injection of S. aureus E48 suspended in a PBS buffer (2 × 108 CFU/ml, 0.5 ml). NZX treatment commenced 2 h after inoculation, with 5, 10, and 20 mg/kg doses given through tail vein one time or two times daily (2 h and 8 h after infection) (Liu et al., 2020). After therapy, the survival conditions of the mice were recorded within 7 days.
In this study, untreated mice that received a lethal dose of S. aureus (2 × 108 CFU/ml, 0.5 ml) began to die at 6 to 8 h, and all died before 24 h, which made it difficult for us to obtain the samples when the therapy effects after 24 h administration were analyzed. Therefore, the mice that received half lethal doses of S. aureus E48 (1 × 108 CFU/ml, 0.5 ml) were conducted in the following pharmacodynamics analysis. After 24 h therapy with NZX (5, 10, and 20 mg/kg) or daptomycin (20 mg/kg), organ tissues, including livers, lungs, spleens, hearts, and kidneys, were collected, homogenated, and plated to count bacterial burden. In addition, the total number of bacteria and intracellular bacteria counts in peritoneal fluids were determined as described by Wang et al. (2018). Briefly, the total bacterial CFU in the peritoneal fluid were quantified before any further procedures. In addition, the collected peritoneal fluid was centrifuged and washed to obtain peritoneal macrophage, and then extracellular bacteria were killed after treatment with 50 μg/ml lysostaphin at 37°C for 30 min. The cells were washed two times by PBS and suspended with 1 ml of cold sterile water for lysis, and the lysate was used to determine the intracellular bacterial burden.
Pharmacokinetics and Pharmacodynamics Relationship
A dose fractionation study was applied to determine the PK/PD parameters of ƒAUC/MIC, ƒCmax/MIC, and ƒT% > MIC, and those parameters were predictive of efficacies for NZX against S. aureus E48. Based on the PK study described above, NZX ranging from 5 to 40 mg/kg in 24 h was applied in order to change the PK/PD parameters of ƒAUC/MIC, ƒCmax/MIC, and ƒT% > MIC (Table 1). The correlations between efficacies and each PK/PD index were analyzed by non-linear least-squares regression based on the sigmoidal Emax model (Hill's equation) (Yu et al., 2016). Hill's equation was defined as follows:
where E is the observed antibacterial effect, which describes the change of log10 CFU/ml in the peritoneal fluid after 24 h of NZX treatment compared to the colony counts at the start of therapy. Emax is the change of log10 CFU/ml in the peritoneal fluid after 24 h of NZX treatment when the maximum antibacterial effect has been reached. E0 is the change of log10 CFU/ml in the peritoneal fluid between initial therapy and after 24 h therapy in untreated control animals. Ce is defined as the three PK/PD parameters (ƒAUC/MIC ratio, ƒCmax/MIC ratio, and ƒT % > MIC). EC50 is the value of the PK/PD parameter that is required to achieve 50% of the maximum antibacterial effect. N is the steepness of the fitted concentration-response curve. The coefficient of determination (R2) between efficacy and the PK/PD parameters was used for determining the goodness of fit of the curves. In addition, the parameters required to achieve bacterial stasis (Cs), 1 log10 kill, and 2 log10 kill were calculated interpolation or extrapolation based on Hill's equation.
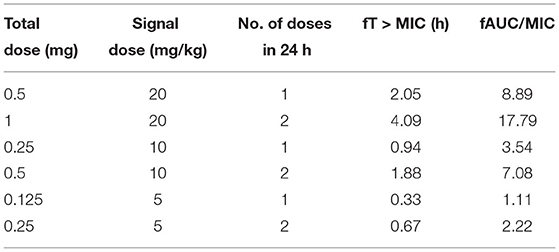
Table 1. Treatment regimens and resulting PK/PD parameters for S. aureus E48 in the mouse peritonitis study.
Statistical Analysis
The pharmacokinetic parameters of NZX in the serum were analyzed using a non-compartment model by WinNonlin software (version 8.1; Pharsight, USA). GraphPad Prism (version 9.0.1) was used for all statistical analyses. One-way ANOVA followed by Dunnett's test was performed to compare the difference between groups. A P-value <0.05 was considered as the significant difference. Kaplan Meier analysis was conducted for the survival curve; log-rank (Mantel-Cox) test analyses were used to compare the survival rate difference.
Results
Antibacterial Activity Against S. aureus
Antibacterial activity against clinical and reference S. aureus strains was shown in Table 2. NZX displayed stronger antibacterial activity against S. aureus MRSA ATCC 43300 (MIC = 1 μg/ml) than linezolid (MIC = 2 μg/ml) and ampicillin (MIC = 8 μg/ml), especially for clindamycin, which was inactive or only weakly active (MIC > 64 μg/ml), demonstrating that S. aureus MRSA ATCC 43300 is intrinsically resistant to clindamycin. Antibacterial activity of NZX (MIC = 1–2 μg/ml) against S. aureus MSSA ATCC 25923 and S. aureus E48 displayed that it was equivalent to linezolid (MIC = 1–2 μg/ml) and daptomycin (MIC = 0.5–2 μg/ml), but inferior to clindamycin (MIC = 0.25 μg/ml) and ampicillin (MIC = 0.25 μg/ml).
Survival Rate and Development of Drug Resistance of S. aureus E48 in vitro
The antibacterial effects of NZX and antibiotics against S. aureus E48 compared to the initial incubation were shown in Figures 1A–D. Survival rates of S. aureus E48 treatment with linezolid, ampicillin, daptomycin, and clindamycin at 1/2 × −2 × MIC for 2 h were 94.08–99.89%, 83.21–100.31%, 0–83.03%, and 0–65.11%, respectively, which were inferior to those of NZX (0–50.35%) (Figure 1A). Specifically, after treatment with 1/2 × MIC NZX for 2 h or 1 × MIC NZX for 1 h, S. aureus was killed by 49.65% (Figure 1B) or 100% (Figure 1C), respectively, which all showed more potent and rapid bactericidal activity than those four chosen antibiotics. In addition, after incubation with 2 × MIC NZX for 0.5 h, S. aureus was already completely killed; however, the clindamycin and daptomycin groups eliminated bacteria within 2 h, which displayed more efficient activity than linezolid and ampicillin (Figure 1D).
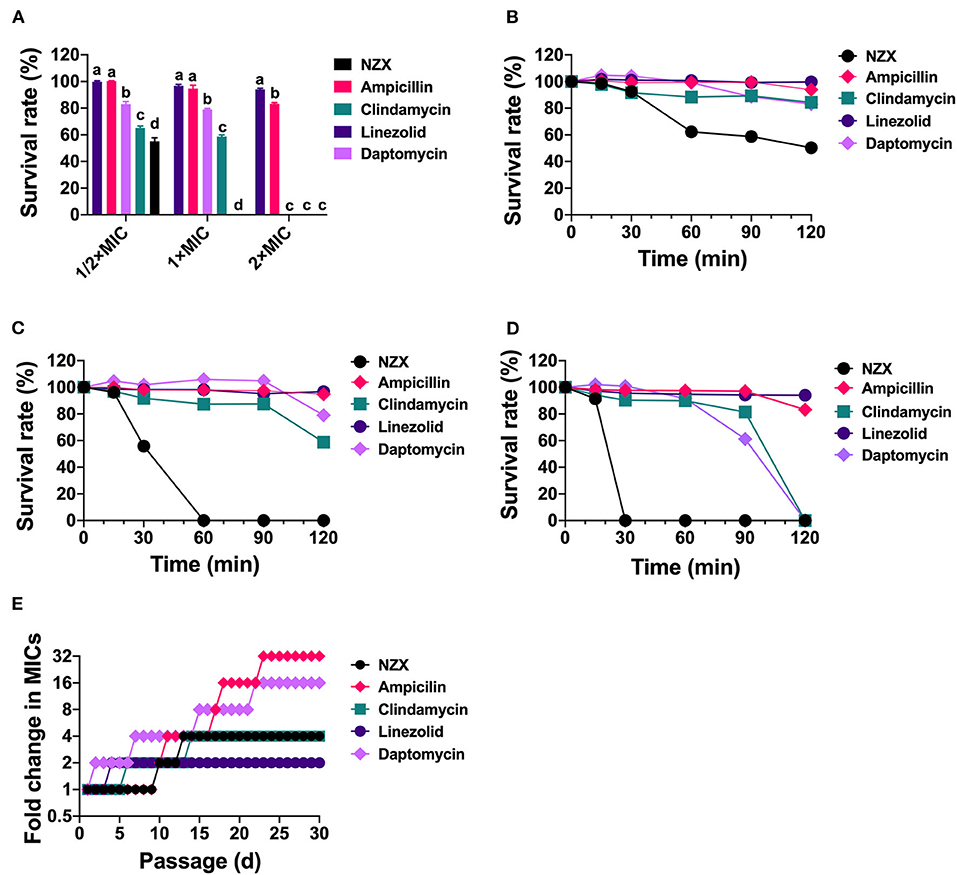
Figure 1. The survival rate and development of drug resistance of S. aureus E48 in vitro. (A) The bacterial cell survival ratio of S. aureus E48 treated with linezolid, ampicillin, daptomycin, clindamycin, and NZX at 1/2 × MIC, 1 × MIC, and 2 × MIC for 120 min. The statistical difference was conducted by one-way ANOVA, with Tukey's multiple comparisons test. Values are expressed as mean ± SD (n = 3), and different letters represent a significant difference in the same concentration (p <0.05). (B–D) The bacterial survival rate of S. aureus E48 treated with 1/2 × MIC (B), 1 × MIC (C), and 2 × MIC (D) linezolid, ampicillin, daptomycin, clindamycin, and NZX for 120 min. Values are expressed as means ± SD (n = 3). (E) Development of drug resistance of S. aureus E48 repeated exposure to linezolid, ampicillin, daptomycin, clindamycin, and NZX for 30-day with sub-MIC levels.
Development of drug resistance of S. aureus E48 was shown in Figure 1E. Results showed that MIC values of linezolid, NZX, clindamycin, daptomycin, and ampicillin against S. aureus E48 after 30-day serial passage were 2, 4, 1, 8, and 16 μg/ml, which resulted in a 2-, 4-, 4-, 16-, and 32-fold increase in the MIC value, respectively. Those results indicated that NZX had a lower risk of resistance compared with ampicillin and daptomycin.
Stability of NZX Under Different Physiological Conditions
The peptide remaining showed that 96.99% of NZX still remained intact after incubation with 25% serum for 24 h (Figure 2A). In addition, there was no change or just 2-fold in the MIC values of NZX and four antibiotics under 25% serum (Figure 2B). Similarly, the MIC values of NZX and four antibiotics in physiological salt environments were unchanged or just 2-fold change, except for daptomycin, which displayed 8–16-fold decrease under the addition of 1.25-mM CaCl2 (Figures 2C–E). The MIC values of NZX, daptomycin, and clindamycin in intracellular lysosomal acid environment at pH 5.0 were up to 4-, 8-, and 8-fold when compared to those of pH 7.4; however, the antibacterial activities of ampicillin and linezolid did not influence (Figure 2F). Furthermore, the antibacterial activities of NZX, daptomycin and linezolid did not change in phagolysosomal proteinase environments, but the MIC values of ampicillin and clindamycin were all down to at least 4-fold (Figure 2G).
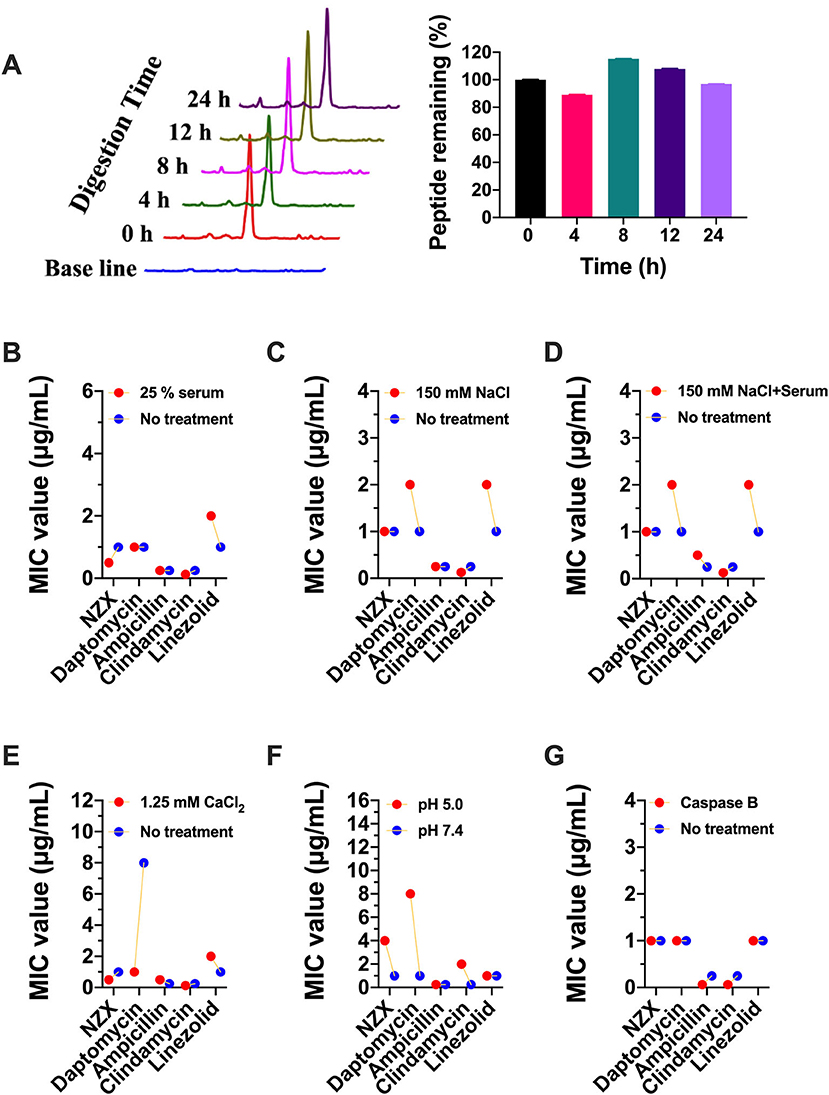
Figure 2. Stability of NZX under different physiological conditions. (A) The peptide remaining after incubation with 25% serum for 24 h in serum. (Left) chromatogram; (Right) histogram. (B–G) The activity of NZX exposure to different physiological conditions for 24 h. No treatment: with the only presence of the MH medium.
Low Toxicity, Intracellular Bactericidal Activity, Uptake, and Location of NZX
As shown in Figure 3A, the viability of RAW 264.7 cells was 85.56~98.96% after treatment with 1~128 μg/ml NZX for 24 h. Furthermore, after exposure to 1~128 μg/ml daptomycin, the cell viability was 87.22~102.19%, which was similar to the NZX treatment. These results demonstrated that NZX and daptomycin had low toxicity to RAW 264.7 cells at tested concentrations.
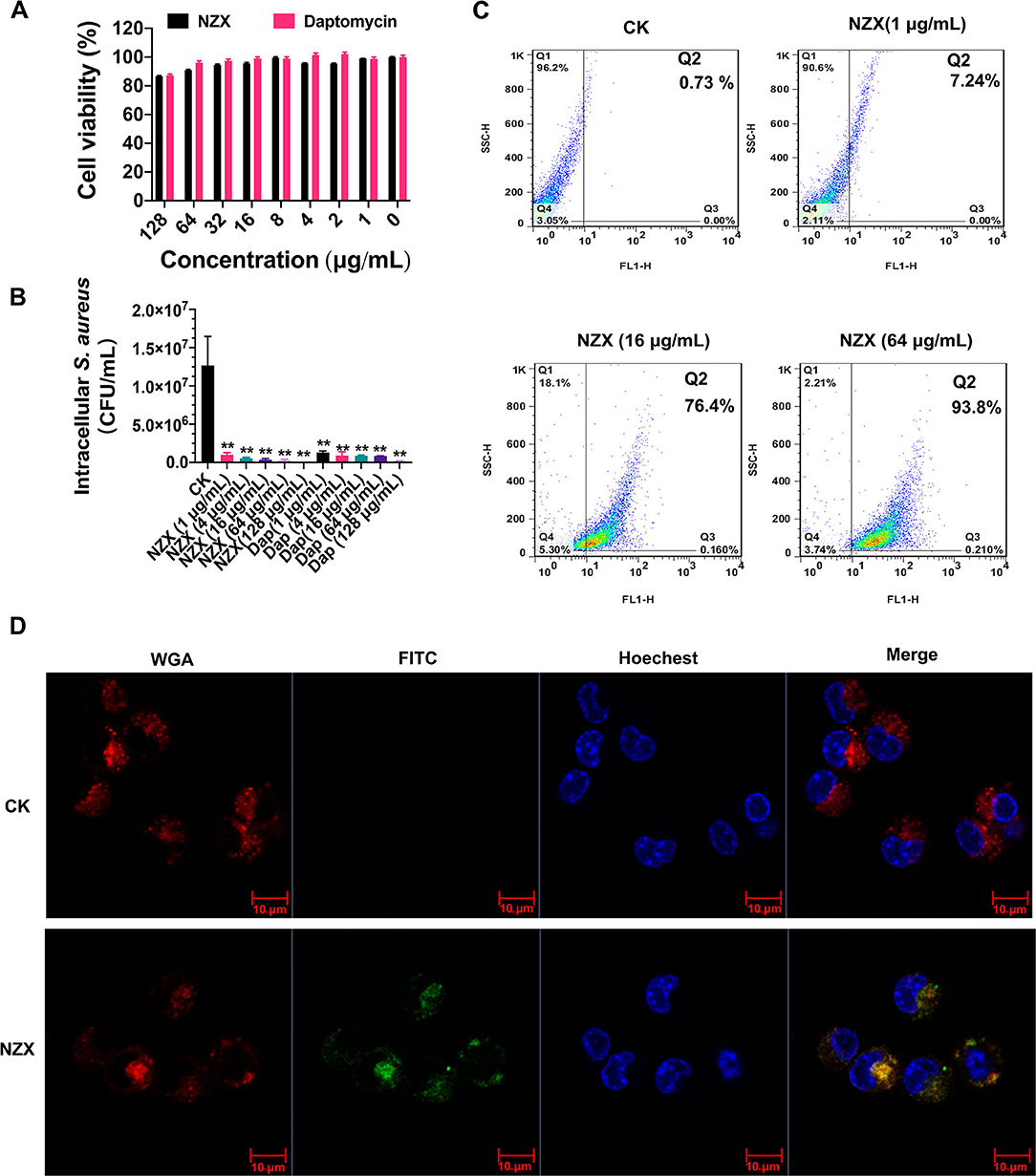
Figure 3. Cell toxicity, intracellular antibacterial activity, cellular uptake, and distribution of NZX in RAW 264.7 cells. (A) Cell viability of RAW 264.7 cells incubated with NZX with concentrations ranging from 1 to 128 μg/ml for 24 h by the MTT method. Daptomycin was set as a positive control. Results from three experiments were expressed as means ± SD (n = 3). (B) Intracellular antibacterial activity of NZX in RAW 264.7 cells infected with S. aureus E48. The activity of NZX and daptomycin against intracellular S. aureus E48 in RAW 264.7 cells was measured after treatment for 24 h. CK: without the presence of any antibacterial agents. (C) Cellular uptake of FITC-labeled NZX. The cells were incubated with 1-, 16-, and 64 μg/ml FITC-labeled NZX for 24 h. The internalization ratio of intracellular FITC-labeled NZX was determined by setting quadrants on the flow cytometry. (D) FITC-labeled NZX distribution in RAW 264.7 cells. The cells were incubated with 64 μg/ml FITC-labeled NZX for 24 h. The localization of FITC-NZX was visualized by confocal microscopy. CK: free FITC without conjugated to NZX. The cells incubated with FITC-NZX presented a green signal; the cell nucleus and membrane were stained with Hoechst 33342 (a blue signal) and wheat germ agglutinin (WGA)-Alexa Fluor® 555 (a red signal), respectively.
The intracellular anti- S. aureus capacity of NZX and daptomycin were determined at different concentrations (1, 4, 16, 64, and 128 μg/ml) using RAW 264.7 cells. As shown in Figure 3B, NZX was effective in mediating intracellular S. aureus load reduction in a concentration-dependent manner with reductions of 91.81~99.81% of intracellular bacteria. Furthermore, at the same concentrations (1, 4, 16, 64, and 128 μg/ml), daptomycin eliminated approximately 89.74~98.53% of intracellular S. aureus. The intracellular bacteria treated with NZX and daptomycin over a wide range of concentrations were all significantly reduced compared with the CK group (Figure 3B). In addition, higher killing potency of NZX against intracellular S. aureus at 16, 64, and 128 μg/ml concentrations was determined compared to the same concentration of daptomycin treatment (Figure 3B).
The uptake efficiency of NZX into RAW 264.7 cells was determined by flow cytometry. After treatment with 1, 16, and 128 μg/ml FITC-NZX for 24 h, the FITC-labeled NZX entered the cell in a dose-dependent way, reaching up to 7.24~93.8% (Figure 3C). Confocal microscopy analysis was further performed to determine the subcellular distribution of NZX in RAW 264.7 cells. As shown in Figure 3D, FITC-labeled NZX was distributed in cell cytosol in a cluster manner, suggesting that the uptake of NZX might be via macropinocytosis and/or endocytosis.
Pharmacokinetics of NZX
Single-dose PK studies of NZX were conducted in non-infected mice. The free drug serum concentrations-time curves of NZX for 6 h following intravenous doses of 5, 10, and 20 mg/kg was shown in Figure 4, and the corresponding PK parameters for NZX were listed in Table 3. Peak concentrations (Cmax) were observed at 5 min after intravenous administration. The area under concentration-time curves (AUC) and Cmax values for the three-dose levels ranged from 1.11 to 8.89 μg·h/ml and 2.85 to 20.55 μg/ml, which were linear over the dose range with a coefficient of determination of 0.9995 and 0.9818, respectively. The elimination half-lives (T1/2) over the three different dose levels ranged from 0.32 to 0.45 h. In addition, the PK parameters for apparent distribution volume (Vd) and mean residence time (MRT) ranged from 1469.10 to 2073.90 ml/kg and 0.32 to 0.56 h, respectively. The murine serum protein binding of NZX was measured to be 89.25% by ultrafiltration method.
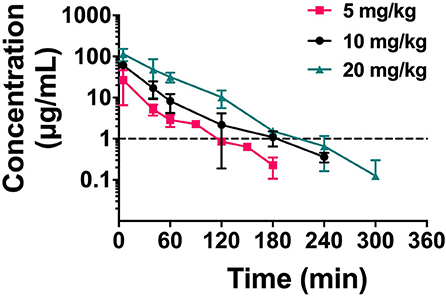
Figure 4. The drug concentration-time curve of NZX. Free serum concentrations for NZX after single intravenous administration of NZX at escalating doses of 5-, 10-, and 20 mg/kg body weight in non-infected mice. Each symbol from five mice indicates the Mean ± SD. The dotted line represents the MIC value of NZX against S. aureus E48.
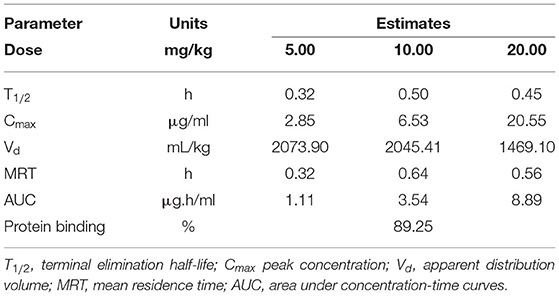
Table 3. Parameter estimates from PK analysis of free-serum NZX concentrations after single intravenous administration of an NZX dose of 5 to 20 mg/kg.
Pharmacodynamics of NZX Against the S. aureus-Induced Mouse Peritonitis Model
In the mouse peritonitis model, ICR mice were intraperitoneally infected with S. aureus E48 to evaluate the therapeutic efficacy of NZX. As shown in Figures 5A,B, the untreated mice were all dead within 24 h after infection. Although after treatment with NZX (5 and 10 mg/kg) and antibiotics (20 mg/kg), including linezolid, ampicillin, and clindamycin by intravenously single-time injection, all the mice were dead; the survival time of the mice extended for 2–4 days (Figure 5A). After treatment with 20 mg/kg NZX, the survival rate of the mice was 33.3%, which was higher than that of treatment with 20 mg/kg daptomycin (16.7%) (Figure 5A). Interestingly, after two-times intravenous injection with NZX and daptomycin at a dose of 20 mg/kg (2 h and 8 h after infection, respectively), both groups of the mice all survived (100%), but the survival rates of the mice treated with ampicillin and clindamycin were 50% and 33.3%, respectively (Figure 5B). In addition, treatment with 5 and 10 mg/kg NZX and 20 mg/kg linezolid did not improve the survival rate of the mice, but all extended lives by 2–6 days (Figure 5B).
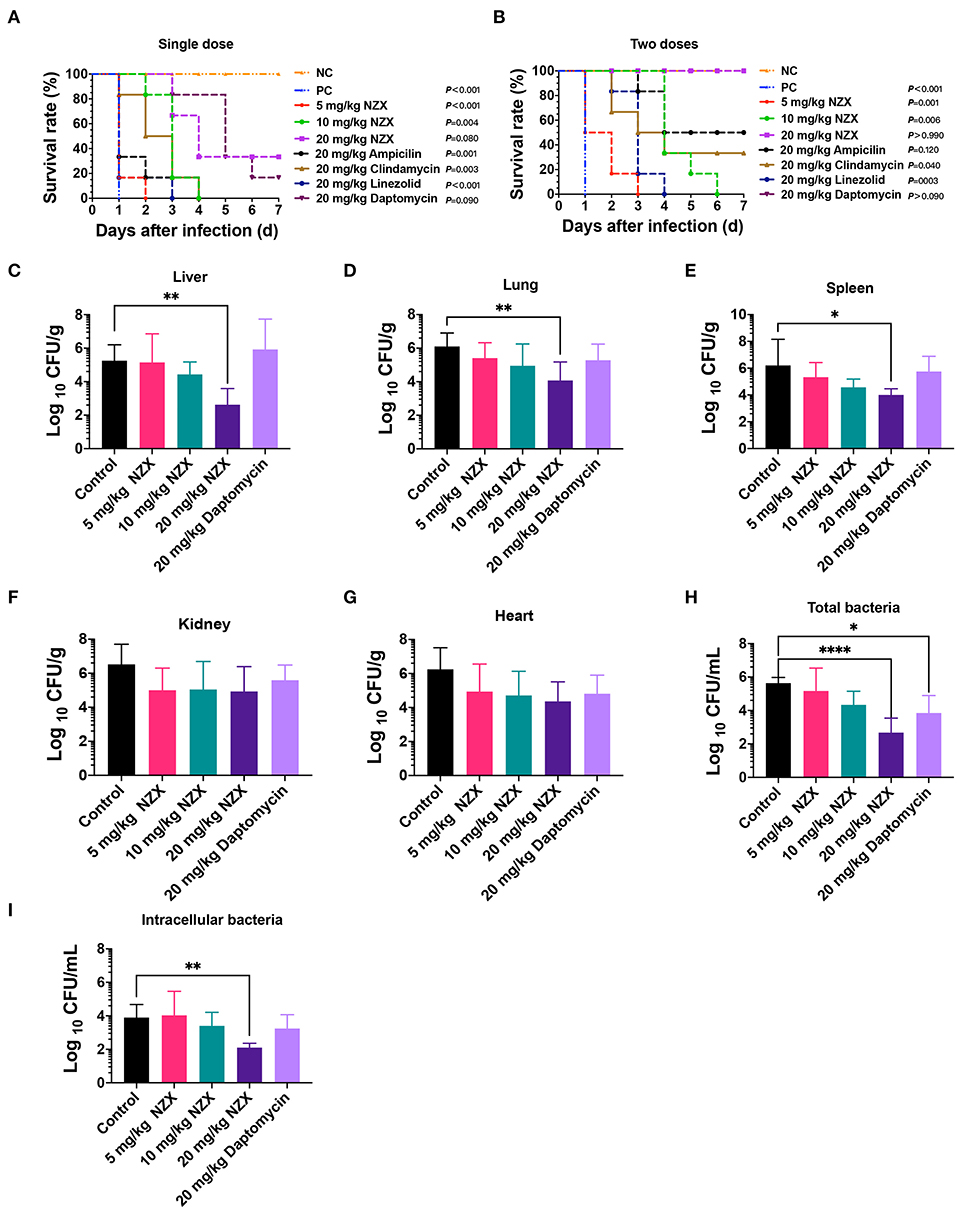
Figure 5. Pharmacodynamics of NZX against S. aureus-induced mouse peritonitis model. (A,B) The survival rate of ICR mice (n = 6) that were challenged intraperitoneally with S. aureus E48 (2 × 108 CFU/ml, 500 μl) for 7-day post-infection. NZX at doses of 5, 10, and 20 mg/kg were administered intravenously single dose (A) (2 h) and two doses (B) (2 and 8 h) post-infection. The PC group: the infected but untreated mice; NC: the uninfected mice. (C–G) The bacterial burden of organ tissues and peritoneal fluid in the ICR mice infected intraperitoneally with S. aureus E48 (1 × 108CFU/ml, 500 μl). The bacterial counts in the liver (C), lung (D), spleen (E), kidneys (F), heart (G), total bacteria (H), and intracellular bacteria (I) in the peritoneal fluid were analyzed after treatment with NZX (5, 10, and 20 mg/kg) or daptomycin (20 mg/kg) for 24 h. The untreated mice were used as the negative control. The statistical difference was conducted by one-way ANOVA, with Tukey's multiple comparisons test. Values are expressed as means ± SD (n = 6); P-value <0.05 was considered significant; *p <0.05; **p <0.01; ****p <0.0001.
The bacterial implantation levels in the liver, lung, and spleen after 24 h were 5.25 ± 0.96, 6.10 ± 0.80, and 6.21 ± 1.95 Log10 CFU/g, respectively (Figures 5C–E). After treatment with NZX, CFU counts in liver, lung, and spleen reduced by −0.10 ± 1.70~2.63 ± 0.97 Log10CFU/g, 0.69 ± 0.91~2.03 ± 1.10 Log10 CFU/g, and 0.88 ± 1.09~2.20 ± 0.46 Log10 CFU/g, respectively, which displayed a dose-dependent effect (Figures 5C–E). Comparing NZX at the 20 mg/kg treatment group with the untreated group, all significant decrease in bacteria burdens of those tissues was observed (Figures 5C–E). Nevertheless, no obvious difference between daptomycin and the untreated group was observed (Figures 5C–E). In addition, therapeutic effects of NZX against organ tissues of the kidneys and heart were also studied, but bacteria burdens of those tissues did not get obviously decreased (Figures 5F,G).
The therapeutic effects of NZX or daptomycin on the total bacteria and intracellular bacteria in the peritoneal fluid were shown in Figures 5H,I. Total bacterial and intracellular bacteria burdens of the untreated mice in the peritoneal fluid were 5.64 ± 0.34 and 3.90 ± 0.78 Log10 CFU/ml (Figures 5H,I). After treatment with NZX at doses of 5, 10, and 20 mg/kg, the total bacterial and intracellular bacteria burdens reduced by 0.47 ± 1.37~2.96 ± 0.87 Log10 CFU/ml and−0.13 ± 1.44~1.79 ± 0.26 Log10 CFU/ml, respectively, and the 20 mg/kg NZX treatment group displayed significant difference with the untreated group, which showed a concentration-dependent effect (Figures 5H,I). The total bacterial and intracellular bacteria burdens of 20 mg/kg daptomycin reduced by 1.79 ± 1.05 Log10 CFU/ml and 0.66 ± 0.83 Log10 CFU/ml, respectively (Figures 5H,I). A significant decrease between daptomycin and the untreated group was observed in the total bacteria, but no significant difference in intracellular bacteria (Figures 5H,I).
Pharmacokinetics and Pharmacodynamics Index Determination
The relationships between the PK/PD parameters and efficacy were presented in Figure 6, and the corresponding pharmacological descriptors were shown in Tables 4, 5, respectively. Maximal effects (Emax) were observed for NZX against total bacteria and intracellular bacteria, which correspond to or close to the actual limit of detection (Figure 6 and Table 5). Results showed that the strongest relationship between the three PK/PD parameters (ƒAUC/MIC, ƒT% > MIC, and ƒCmax > MIC) and NZX efficacy against total bacteria was observed, with R2 values of 0.91, 0.91, and 0.72, respectively (Table 4). Similarly, the strongest relationship between the three PK parameters and efficacy against intracellular bacteria was noted, with R2 values of 0.81, 0.81, and 0.66, respectively (Table 4). The parameters required to achieve bacterial stasis (Cs), 1 log10 kill and 2 log10 kill were shown in Table 5. The static dose ƒAUC/MIC ratio, the ƒT% > MIC ratio, and the ƒCmax > MIC ratio for total bacteria were 3.574, 4.274, and 3.971, respectively (Table 4). The 1 log10 kill ƒAUC/MIC ratio, the ƒT% > MIC ratio, and the ƒCmax > MIC ratio for total bacteria were 3.896, 4.434, and 6.595, respectively (Table 5). The 2 log10 kill ƒAUC/MIC ratio, the ƒT% > MIC ratio, and the ƒCmax > MIC ratio for total bacteria were 4.778, 5.759, and 24.492, respectively (Table 5). Moving to intracellular bacteria, the static dose ƒAUC/MIC ratio, the ƒT% > MIC ratio, and the ƒCmax > MIC ratio were 3.473, 3.670, and 3.090, respectively (Table 5). The 1 log10 kill ƒAUC/MIC ratio, the ƒT% > MIC ratio, and the ƒCmax > MIC ratio were 3.569, 4.010, and 4.734, respectively (Table 5). The 2 log10 kill ƒAUC/MIC ratio, the ƒT% > MIC ratio, and the ƒCmax > MIC ratio were 3.698, 4.502, and 13.877, respectively (Table 5).
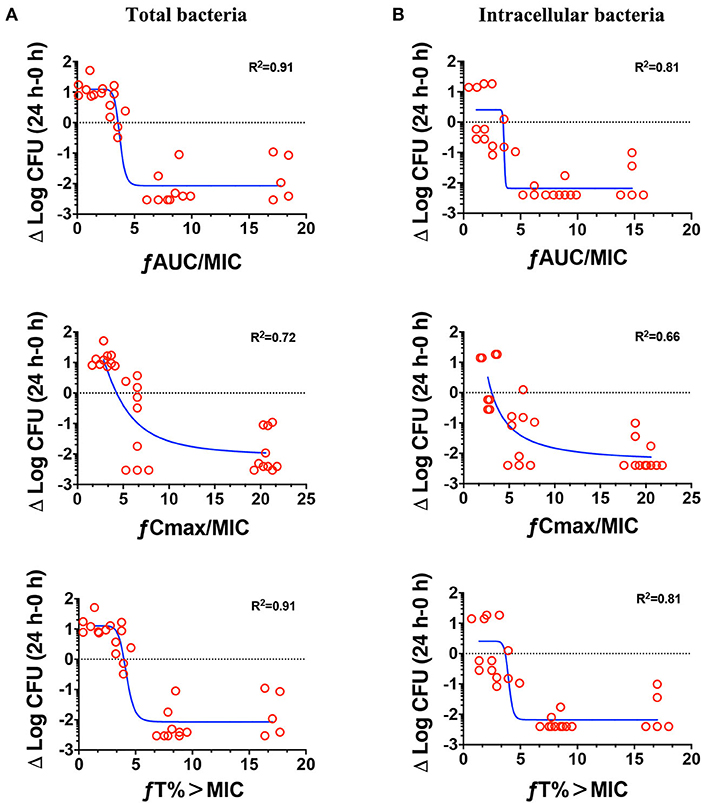
Figure 6. Pharmacokinetics and pharmacodynamics index determination. Correlations between the pharmacokinetic parameters (24 h ƒAUC/MIC, ƒCmax/MIC, and ƒT% > MIC) and efficacies for total bacteria (A) and intracellular activity (B) over 24 h against S. aureus E48 (MIC = 1 μg/ml) in the mouse peritonitis model. The ordinate indicated the total bacteria and intracellular bacteria counts change in peritoneal lavage fluids between the treated for 24 h animals and those at the start of therapy. Each symbol indicates the bacteria of each mouse. The curves indicate the best fit using the sigmoid Emax model. R2 value represents the magnitude of correlation.
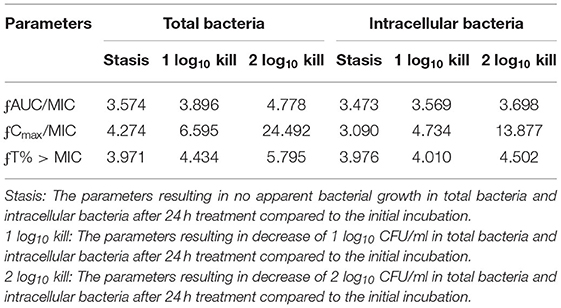
Table 5. The parameters required to achieve bacterial stasis or 1 log10 kill or 2 log10 kill for total bacteria and intracellular bacteria.
Discussion
NZX, a variant of plectasin, has garnered interest as an ideal therapeutic drug against S. aureus because of its ability to kill intracellular bacteria and good biocompatibility (Tenland et al., 2018; Liu et al., 2020). Compared with plectasin (MIC, 4 μg/ml), NZX displayed a 4-fold higher antimicrobial activity (Table 2) (Yang et al., 2019). Furthermore, plectasin at a concentration of 2 × MIC killed approximately 85.85% of S. aureus E48 within 1 h, and eliminated bacteria within 2 h, which exhibited inferior bactericidal activity compared with NZX (Figures 1A–D) (Yang et al., 2019). This is because that replacement of D, M, and K residues in plectasin at positions 9, 13, and 31 by S, I, and R increased the net charge and hydrophobicity of NZX (Liu et al., 2020). Increased net charge and hydrophobicity improved the antibacterial activity of peptides (Higgs et al., 2007; Zhou et al., 2019; Tan et al., 2021); thus NZX showed more effective and rapid antibacterial activity than plectasin at the same concentration.
Unlike conventional antibiotics, AMPs usually kill pathogenic bacteria by physically disrupting the cell membrane, causing leakage of contents, and interfering with intracellular metabolic pathways (Gan et al., 2021; Jiang et al., 2021). Due to the unique mechanism of action, AMPs are not easy to cause bacterial mutations or develop only limited resistance with fitness cost (Choi and Ko, 2015; Nang et al., 2018; Gan et al., 2021; Jiang et al., 2021). Spohn et al. (2019) systematically investigated the resistance evolution of 14 AMPs and 12 antibiotics against Escherichia coli in vitro, demonstrating that AMPs had a lower risk of resistance than those of antibiotics (Spohn et al., 2019). Similarly, in this study, NZX had a lower risk of resistance compared with those of ampicillin and daptomycin (Figure 1E). Drug stability is vital for clinical application. NZX displayed good tolerance to serum, physiological salt, and proteinase conditions, but poor in the phagolysosomal acid environment (Figure 2), which were in accordance with the previous studies (Wang et al., 2018; Li et al., 2021).
The intracellular survival mechanism of S. aureus contributes to pathogen dissemination among organ tissues, resulting in disease progression or recurrence (Löffler et al., 2014; Horn et al., 2018; Wang et al., 2021) and even being at a chronic situation. Plectasin, NZ2114, and MP1102 can kill intracellular S. aureus in human THP-1 macrophages and RAW 264.7 cells, respectively (Brinch et al., 2009, 2010; Wang et al., 2018). NZX also significantly reduced the intracellular S. aureus in RAW 264.7 cells (Figure 3B). Different from intracellular activities of NZ2114 (Brinch et al., 2010), NZX exhibited higher killing potency than that of daptomycin. Entering into host cells and contacting with the pathogen are prerequisites of drugs for intracellular S. aureus elimination (Carryn et al., 2003). Both MP1102 and NZ2114 can enter into RAW 264.7 cells in a cluster manner and a dose-dependent way, and distributed in the cytoplasm (Wang et al., 2018). Similarly, NZX was distributed and internalized in the same manner (Figures 3C,D). In addition, internalization mechanisms exhibited that the entrance of MP1102 and NZ2114 into RAW 264.7 cells were mainly dependent on clathrin-mediated endocytosis and micropinocytosis in an energy- and temperature-dependent manner (Wang et al., 2018). As an analog of NZ2114 and MP1102 peptide, so should be NZX, but further research is needed.
In this study, the protein-binding degree of NZX (89.25%) was similar to plectasin (90%) in human and mice serum, and higher than that of NZ2114 (80%) (Andes et al., 2009; Brinch et al., 2009), indicating that it could bind to serum protein components, such as albumin, apolipoproteins or glycoproteins. As the dominant role of those free constituents in pharmacodynamics and pharmacokinetics, a plethora of studies indicated that the binding degree of drugs to serum protein will influence their pharmacological parameters and antibacterial activity, but those impacts on their activity were multifaceted and more complicated (Zeitlinger et al., 2008; Beer et al., 2009; Dalhoff, 2018). Some studies demonstrated that antibacterial agents with high protein binding had a potent and enhanced bactericidal ability, and some drugs above 90% plasma protein binding had also been approved, which did not disturb its suitability for systemic application (Perl et al., 1990; Cheah et al., 2015; Dalhoff, 2018; Hirsch et al., 2019). Similar to plectasin and NZ2114 with short half-lives (Andes et al., 2009; Brinch et al., 2009), NZX was rapidly metabolized by a mouse (Figure 4). Owing to peptides typically with <10 kDa in molecular mass, they could be freely filtered by the kidney and glomerulus, and were easily metabolized or degraded by the ubiquitous proteases (Lin, 2009; Meibohm and Zhou, 2012; Diao and Meibohm, 2013). These characteristics might answer the questions from the antibacterial peptide in preclinical pharmacokinetics. To overcome quick elimination of NZX in mouse serum, some strategies extending half-life have been tried to improve the pharmacokinetic characteristics of peptides (Diao and Meibohm, 2013; Kanugo and Misra, 2020).
The ability of NZX to decrease bacterial burden in organ tissues depends on its distribution. Benincasa et al. demonstrated that Bac7(1-35)-Alexa680 peptide via intraperitoneal injection could only reach kidneys and liver (Benincasa et al., 2010). Bailleul et al. demonstrated fluorescence-labeled AvBD7 by intraperitoneal injection could reach kidneys, liver, spleen, and mesenteric lymph nodes (Bailleul et al., 2019). Different from those reports (Benincasa et al., 2010; Bailleul et al., 2019), a substantial amount of NZX was observed in the kidneys and spleen at a rank of the second and third most fluorescent organs after the lung by in vivo imaging (Supplementary Figure S1), which were in accordance with the antibacterial activity of NZX in those organs (Figures 5C–G). That means that each AMP distribution in body depends on its individual characteristics. We know that intracellular drug concentration is vital to the treatment of intracellular bacteria, but it is extremely difficult to obtain intracellular pharmacokinetic characteristics of drugs in vivo (Levison and Levison, 2009). Therefore, serum drug levels were usually used as the first choice for predicting intracellular concentrations regardless of their correlation (Levison and Levison, 2009). Different from Brinch et al. (2009), who demonstrated that ƒCmax/MIC was the most important parameter for the activity of plectasin against total bacteria and intracellular bacteria, we found that all three PK/PD parameters showed the strongest correlation with efficacies (Figure 6 and Table 4). In general, our work mainly focused on the relationship between efficacy and PK/PD parameters against S. aureus in a mouse peritonitis model; their direct comparisons to other peptides need more data from different animal models in the future.
Conclusion
NZX displayed a narrow antimicrobial spectrum, high potency, and rapid antibacterial activity against the tested S. aureus with a lower frequency of resistant mutations compared with ampicillin and daptomycin. Stability results in vitro showed that NZX displayed good biocompatibility and tolerance to physiological salt, serum, and phagolysosomal cathepsin, except for the phagolysosomal acid environment. In addition, NZX could enter into RAW 264.7 cells in a dose-dependent manner and exhibited higher killing potency against intracellular S. aureus in vitro than daptomycin. Pharmacokinetics results of NZX in vivo showed that serum protein binding was 89.25%, and corresponding parameters of the AUC and Cmax and T1/2 for the escalating NZX doses ranged from 1.11 to 8.89 μg.h/ml, 2.85 to 20.55 μg/ml, and 0.32 to 0.5 h, respectively. Together with the pharmacodynamics results in vivo, NZX administered intravenously two times could rescue all infected mice equivalent to daptomycin and NZX treatment, significantly reduced CFU counts in organ tissues and in the peritoneal fluid, which were superior or similar to those of treatment with daptomycin. These in vivo efficacies were significantly correlated with three PK/PD indices of ƒAUC/MIC, ƒCmax/MIC, and ƒT% > MIC, as analyzed by a sigmoid maximum-effect model. Altogether, this study demonstrated that NZX could act as a new antimicrobial agent to protect host against infectious diseases caused by S. aureus.
Data Availability Statement
The original contributions presented in the study are included in the article/Supplementary Material, further inquiries can be directed to the corresponding author/s.
Ethics Statement
The animal study was reviewed and approved by the Animal Care and Use Committee of the Feed Research Institute, Chinese Academy of Agricultural Sciences (Permit Number: 20150309).
Author Contributions
XZ, DT, and JW conceived and designed the experiments. XZ carried out all the experiments. XZ, DT, and NY guided the methods and contributed to writing. YH and RM contributed materials and reagents. JW contributed to funding acquisition. All the authors contributed to the article and approved the submitted version.
Funding
This work was supported by the National Natural Science Foundation of China (Grant No. 31872393), the Innovation Program of Agricultural Science and Technology AMPs and Alternatives to Antibiotics for Animal Usage (ASTIP) in CAAS (Grant No. CAAS-ASTIP-2013-FRI-02), and its key projects (Grant Nos. CAAS-ZDXT201808 and CAAS-ZDRW202111).
Conflict of Interest
The authors declare that the research was conducted in the absence of any commercial or financial relationships that could be construed as a potential conflict of interest.
Publisher's Note
All claims expressed in this article are solely those of the authors and do not necessarily represent those of their affiliated organizations, or those of the publisher, the editors and the reviewers. Any product that may be evaluated in this article, or claim that may be made by its manufacturer, is not guaranteed or endorsed by the publisher.
Supplementary Material
The Supplementary Material for this article can be found online at: https://www.frontiersin.org/articles/10.3389/fmicb.2022.865774/full#supplementary-material
References
Alonso, J. M., Martins, E. S., Peccinini, R. G., Rosa, G. S., Guerra, S. T., Ribeiro, M. G., et al. (2021). Plasma and peritoneal ceftriaxone concentrations after intraperitoneal administration in horses with septic peritonitis. J. Equine Vet. Sci. 96, 103310. doi: 10.1016/j.jevs.2020.103310
Andes, D., Craig, W., Nielsen, L. A., and Kristensen, H. H. (2009). In vivo pharmacodynamic characterization of a novel plectasin antibiotic, NZ2114, in a murine infection model. Antimicrob. Agents Chemother. 53, 3003–3009. doi: 10.1128/aac.01584-08
Bailleul, G., Guabiraba, R., Virlogeux-Payant, I., Lantier, I., Trotereau, J., Gilbert, F. B., et al. (2019). Systemic administration of avian defensin 7: distribution, cellular target, and antibacterial potential in mice. Front Microbiol. 10, 541. doi: 10.3389/fmicb.2019.00541
Beer, J., Wagner, C. C., and Zeitlinger, M. (2009). Protein binding of antimicrobials: methods for quantification and for investigation of its impact on bacterial killing. AAPS J. 11, 1–12. doi: 10.1208/s12248-008-9072-1
Benincasa, M., Pelillo, C., Zorzet, S., Garrovo, C., Biffi, S., Gennaro, R., et al. (2010). The proline-rich peptide Bac7(1-35) reduces mortality from Salmonella Typhimurium in a mouse model of infection. BMC Microbiol. 10, 178. doi: 10.1186/1471-2180-10-178
Brinch, K. S., Sandberg, A., Baudoux, P., Van Bambeke, F., Tulkens, P. M., Frimodt-Møller, N., et al. (2009). Plectasin shows intracellular activity against Staphylococcus Aureus in human THP-1 monocytes and in a mouse peritonitis model. Antimicrob. Agents Chemother. 53, 4801–4808. doi: 10.1128/aac.00685-09
Brinch, K. S., Tulkens, P. M., Van Bambeke, F., Frimodt-Møller, N., Høiby, N., and Kristensen, H. H. (2010). Intracellular activity of the peptide antibiotic Nz2114: studies with Staphylococcus Aureus and human THP-1 monocytes, and comparison with daptomycin and vancomycin. J. Antimicrob. Chemother. 65, 1720–1724. doi: 10.1093/jac/dkq159
Broughton, A., Verger, C., and Goffin, E. (2010). Pets-related peritonitis in peritoneal dialysis: companion animals or trojan horses? Semin. Dial. 23, 306–316. doi: 10.1111/j.1525-139X.2010.00726.x
Camargo, C. H., de Souza da Cunha, M. L. R., Caramori, J. C. T., Mondelli, A. L., Montelli, A. C., and Barretti, P. (2021). Incidence and characteristics of methicillin-resistant coagulase-negative Staphylococcus Aureus in peritoneal dialysis-associated peritonitis in a single center using molecular methods. Int. Urol. Nephrol. 53, 373–380. doi: 10.1007/s11255-020-02605-9
Carryn, S., Chanteux, H., Seral, C., Mingeot-Leclercq, M. P., Van Bambeke, F., and Tulkens, P. M. (2003). Intracellular pharmacodynamics of antibiotics. Infect. Dis. Clin. North Am. 17, 615–634. doi: 10.1016/s0891-5520(03)00066-7
Cheah, S. E., Wang, J., Nguyen, V. T., Turnidge, J. D., Li, J., and Nation, R. L. (2015). New pharmacokinetic/pharmacodynamic studies of systemically administered colistin against Pseudomonas Aeruginosa and Acinetobacter Baumannii in mouse thigh and lung infection models: smaller response in lung infection. J. Antimicrob. Chemother. 70, 3291–3297. doi: 10.1093/jac/dkv267
Choi, M. J., and Ko, K. S. (2015). Loss of hypermucoviscosity and increased fitness cost in colistin-resistant Klebsiella Pneumoniae sequence type 23 strains. Antimicrob. Agents Chemother. 59, 6763–6773. doi: 10.1128/aac.00952-15
Costa, F., Teixeira, C., Gomes, P., and Martins, M. C. L. (2019). Clinical application of AMPs. Adv. Exp. Med. Biol. 1117, 281–298. doi: 10.1007/978-981-13-3588-4_15
Dalhoff, A.. (2018). Seventy-five years of research on protein binding. Antimicrob. Agents Chemother. 62. doi: 10.1128/aac.01663-17
Diao, L., and Meibohm, B. (2013). Pharmacokinetics and pharmacokinetic-pharmacodynamic correlations of therapeutic peptides. Clin. Pharmacokinet. 52, 855–868. doi: 10.1007/s40262-013-0079-0
Ewles, M., and Goodwin, L. (2011). Bioanalytical approaches to analyzing peptides and proteins by LC–MS/MS. Bioanalysis 3, 1379–1397. doi: 10.4155/bio.11.112
Gan, B. H., Gaynord, J., Rowe, S. M., Deingruber, T., and Spring, D. R. (2021). The multifaceted nature of antimicrobial peptides: current synthetic chemistry approaches and future directions. Chem. Soc. Rev. 50, 7820–7880. doi: 10.1039/d0cs00729c
Giguère, S., Burton, A. J., Berghaus, L. J., and Haspel, A. D. (2017). Comparative pharmacokinetics of minocycline in foals and adult horses. J. Vet. Pharmacol. Ther. 40, 335–341. doi: 10.1111/jvp.12366
Hao, Y., Wang, J., de la Fuente-Nunez, C., and Franco, O. (2022). Editorial: antimicrobial peptides: molecular design, structure-function relationship, and biosynthesis optimization. Front. Microbiol. 13, 888540. doi: 10.3389/fmicb.2022.888540
Hengzhuang, W., Wu, H., Ciofu, O., Song, Z., and Høiby, N. (2012). In vivo pharmacokinetics/pharmacodynamics of colistin and imipenem in Pseudomonas Aeruginosa biofilm infection. Antimicrob. Agents Chemother. 56, 2683–2690. doi: 10.1128/aac.06486-11
Henninot, A., Collins, J. C., and Nuss, J. M. (2018). The current state of peptide drug discovery: back to the future? J. Med. Chem. 61, 1382–1414. doi: 10.1021/acs.jmedchem.7b00318
Higgs, R., Lynn, D. J., Cahalane, S., Alaña, I., Hewage, C. M., James, T., et al. (2007). Modification of chicken avian beta-defensin-8 at positively selected amino acid sites enhances specific antimicrobial activity. Immunogenetics 59, 573–580. doi: 10.1007/s00251-007-0219-5
Hirsch, R., Wiesner, J., Marker, A., Pfeifer, Y., Bauer, A., Hammann, P. E., et al. (2019). Profiling antimicrobial peptides from the medical maggot lucilia sericata as potential antibiotics for mdr gram-negative bacteria. J. Antimicrob. Chemother. 74, 96–107. doi: 10.1093/jac/dky386
Horn, J., Stelzner, K., Rudel, T., and Fraunholz, M. (2018). Inside job: Staphylococcus Aureus host-pathogen interactions. Int. J. Med. Microbiol. 308, 607–624. doi: 10.1016/j.ijmm.2017.11.009
Jacobson, E., Gronwall, R., Maxwell, L., Merrit, K., and Harman, G. (2005). Plasma concentrations of enrofloxacin after single-dose oral administration in loggerhead sea turtles (Caretta Caretta). J. Zoo Wildl. Med. 36, 628–634. doi: 10.1638/04093.1
Jiang, Y., Chen, Y., Song, Z., Tan, Z., and Cheng, J. (2021). Recent advances in design of antimicrobial peptides and polypeptides toward clinical translation. Adv. Drug Deliv. Rev. 170, 261–280. doi: 10.1016/j.addr.2020.12.016
Kanugo, A., and Misra, A. (2020). New and novel approaches for enhancing the oral absorption and bioavailability of protein and peptides therapeutics. Ther. Deliv. 11, 713–732. doi: 10.4155/tde-2020-0068
Leroy, P., Decolin, D., Nicolas, S., Archimbault, P., and Nicolas, A. (1989). Residue determination of two co-administered antibacterial agents–cephalexin and colistin–in calf tissues using high-performance liquid chromatography and microbiological methods. J. Pharm. Biomed. Anal. 7, 1837–1846. doi: 10.1016/0731-7085(89)80201-8
Levison, M. E., and Levison, J. H. (2009). Pharmacokinetics and pharmacodynamics of antibacterial agents. Infect. Dis. Clin. North Am. 23, 791–815. doi: 10.1016/j.idc.2009.06.008
Li, H., Wieser, A., Zhang, J., Liss, I., Markwardt, D., Hornung, R., et al. (2020a). Patients with cirrhosis and sbp: increase in multidrug-resistant organisms and complications. Eur. J. Clin. Invest. 50, e13198. doi: 10.1111/eci.13198
Li, J., Shang, L., Lan, J., Chou, S., Feng, X., Shi, B., et al. (2020b). Targeted and intracellular antibacterial activity against S. Agalactiae of the chimeric peptides based on pheromone and cell-penetrating peptides. ACS Appl. Mater. Interfaces 12, 44459–44474. doi: 10.1021/acsami.0c12226
Li, T., Wang, Z., Han, H., Teng, D., Mao, R., Hao, Y., et al. (2021). Potent intracellular antibacterial activity of a marine peptide-N6NH2 and its D-enantiomer against multidrug-resistant Aeromonas Veronii. Appl. Microbiol. Biotechnol. 105, 2351–2361. doi: 10.1007/s00253-021-11176-3
Li, Z., Mao, R., Teng, D., Hao, Y., Chen, H., Wang, X., et al. (2017). Antibacterial and immunomodulatory activities of insect defensins-DLP2 and DLP4 against multidrug-resistant Staphylococcus Aureus. Sci. Rep. 7, 12124. doi: 10.1038/s41598-017-10839-4
Lin, J. H.. (2009). Pharmacokinetics of biotech drugs: peptides, proteins and monoclonal antibodies. Curr. Drug Metab. 10, 661–691. doi: 10.2174/138920009789895499
Liu, H., Yang, N., Mao, R., Teng, D., Hao, Y., Wang, X., et al. (2020). A new high-yielding antimicrobial peptide NZX and its antibacterial activity against Staphylococcus Hyicus in vitro/vivo. Appl. Microbiol. Biotechnol. 104, 1555–1568. doi: 10.1007/s00253-019-10313-3
Liu, Y., Shi, J., Tong, Z., Jia, Y., Yang, B., and Wang, Z. (2021). The revitalization of antimicrobial peptides in the resistance era. Pharmacol Res. 163, 105276. doi: 10.1016/j.phrs.2020.105276
Löffler, B., Tuchscherr, L., Niemann, S., and Peters, G. (2014). Staphylococcus Aureus persistence in non-professional phagocytes. Int. J. Med. Microbiol. 304, 170–176. doi: 10.1016/j.ijmm.2013.11.011
Marciano, S., Díaz, J. M., Dirchwolf, M., and Gadano, A. (2019). Spontaneous bacterial peritonitis in patients with cirrhosis: incidence, outcomes, and treatment strategies. Hepat. Med. 11, 13–22. doi: 10.2147/hmer.S164250
Meibohm, B., and Zhou, H. (2012). Characterizing the impact of renal impairment on the clinical pharmacology of biologics. J. Clin. Pharmacol. 52, 54s−62s. doi: 10.1177/0091270011413894
Mercer, D. K., and O'Neil, D. A. (2013). Peptides as the next generation of anti-infectives. Future Med. Chem. 5, 315–337. doi: 10.4155/fmc.12.213
Moretta, A., Scieuzo, C., Petrone, A. M., Salvia, R., Manniello, M. D., Franco, A., et al. (2021). Antimicrobial peptides: a new hope in biomedical and pharmaceutical fields. Front. Cell. Infect. Microbiol. 11, 668632. doi: 10.3389/fcimb.2021.668632
Mygind, P. H., Fischer, R. L., Schnorr, K. M., Hansen, M. T., Sönksen, C. P., Ludvigsen, S., et al. (2005). Plectasin is a peptide antibiotic with therapeutic potential from a Saprophytic fungus. Nature. 437, 975–980. doi: 10.1038/nature04051
Nang, S. C., Morris, F. C., McDonald, M. J., Han, M. L., Wang, J., Strugnell, R. A., et al. (2018). Fitness cost of Mcr-1-mediated polymyxin resistance in Klebsiella Pneumoniae. J. Antimicrob. Chemother. 73, 1604–1610. doi: 10.1093/jac/dky061
Perl, T. M., Pfaller, M. A., Houston, A., and Wenzel, R. P. (1990). Effect of serum on the in vitro activities of 11 broad-spectrum antibiotics. Antimicrob. Agents Chemother. 34, 2234–2239. doi: 10.1128/aac.34.11.2234
Pörner, D., Von Vietinghoff, S., Nattermann, J., Strassburg, C. P., and Lutz, P. (2021). Advances in the pharmacological management of bacterial peritonitis. Expert Opin. Pharmacother. 22, 1567–1578. doi: 10.1080/14656566.2021.1915288
Rima, M., Rima, M., Fajloun, Z., Sabatier, J. M., Bechinger, B., and Naas, T. (2021). Antimicrobial peptides: a potent alternative to antibiotics. Antibiotics 10, 1095. doi: 10.3390/antibiotics10091095
Salzer, W. L.. (2018). Peritoneal dialysis-related peritonitis: challenges and solutions. Int. J. Nephrol. Renovasc. Dis. 11, 173–186. doi: 10.2147/IJNRD.S123618
Schneider, T., Kruse, T., Wimmer, R., Wiedemann, I., Sass, V., Pag, U., et al. (2010). Plectasin, a fungal defensin, targets the bacterial cell wall precursor lipid II. Science 328, 1168–1172. doi: 10.1126/science.1185723
Spohn, R., Daruka, L., Lázár, V., Martins, A., Vidovics, F., Grézal, G., et al. (2019). Integrated evolutionary analysis reveals antimicrobial peptides with limited resistance. Nat. Commun. 10, 4538. doi: 10.1038/s41467-019-12364-6
Szeto, C. C., Ng, J. K., Wing-Shing Fung, W., Chan, G. C., Cheng, P. M., Lai, K. B., et al. (2021). Extended antibiotic therapy for the prevention of relapsing and recurrent peritonitis in peritoneal dialysis patients: a randomized controlled trial. Clin. Kidney J. 14, 991–997. doi: 10.1093/ckj/sfaa256
Tan, P., Fu, H., and Ma, X. (2021). Design, optimization, and nanotechnology of antimicrobial peptides: from exploration to applications. Nano Today 39, 101229. doi: 10.1016/j.nantod.2021.101229
Tenland, E., Krishnan, N., Rönnholm, A., Kalsum, S., Puthia, M., Mörgelin, M., et al. (2018). A novel derivative of the fungal antimicrobial peptide plectasin is active against Mycobacterium Tuberculosis. Tuberculosis 113, 231–238. doi: 10.1016/j.tube.2018.10.008
Tenland, E., Pochert, A., Krishnan, N., Umashankar Rao, K., Kalsum, S., Braun, K., et al. (2019). Effective delivery of the anti-Mycobacterial peptide NZX in mesoporous silica nanoparticles. PLoS ONE 14, e0212858. doi: 10.1371/journal.pone.0212858
Wang, M., Fan, Z., and Han, H. (2021). Autophagy in Staphylococcus Aureus infection. Front. Cell. Infect. Microbiol. 11, 750222. doi: 10.3389/fcimb.2021.750222
Wang, X., Teng, D., Wang, X., Hao, Y., Chen, H., Mao, R., et al. (2019). Internalization, distribution, and activity of peptide H2 against the intracellular multidrug-resistant bovine mastitis-causing bacterium Staphylococcus Aureus. Sci. Rep. 9, 7968. doi: 10.1038/s41598-019-44459-x
Wang, X., Wang, X., Teng, D., Mao, R., Hao, Y., Yang, N., et al. (2018). Increased intracellular activity of MP1102 and NZ2114 against Staphylococcus Aureus in vitro and in vivo. Sci. Rep. 8, 4204. doi: 10.1038/s41598-018-22245-5
Wang, Z., Liu, X., Da, T., Mao, R., Hao, Y., Yang, N., et al. (2020). Development of chimeric peptides to facilitate the neutralisation of lipopolysaccharides during bactericidal targeting of multidrug-resistant Escherichia Coli. Commun. Biol. 3, 41. doi: 10.1038/s42003-020-0761-3
Watts, J. L., Shryock, T. R., Apley, M., Bade, D. L., Brown, S. D., Gray, J. T., et al. (2013). Performance Standards for Antimicrobial Disk and Dilution Susceptibility Test for Bacteria Isolated from Animals; Approved Standard. Wayne, PA: NCCLS.
Yang, N., Teng, D., Mao, R., Hao, Y., Wang, X., Wang, Z., et al. (2019). A Recombinant fungal defensin-like peptide-P2 combats multidrug-resistant Staphylococcus Aureus and biofilms. Appl. Microbiol. Biotechnol. 103, 5193–5213. doi: 10.1007/s00253-019-09785-0
Yu, Y., Zhou, Y. F., Li, X., Chen, M. R., Qiao, G. L., Sun, J., et al. (2016). Dose assessment of cefquinome by pharmacokinetic/pharmacodynamic modeling in mouse model of Staphylococcus Aureus mastitis. Front. Microbiol. 7, 1595. doi: 10.3389/fmicb.2016.01595
Zeitlinger, M., Sauermann, R., Fille, M., Hausdorfer, J., Leitner, I., and Müller, M. (2008). Plasma protein binding of fluoroquinolones affects antimicrobial activity. J. Antimicrob. Chemother. 61, 561–567. doi: 10.1093/jac/dkm524
Zheng, X., Teng, D., Mao, R., Hao, Y., Yang, N., Hu, F., et al. (2021). A study on fungal defensin against multidrug-resistant Clostridium Perfringens and its treatment on infected poultry. Appl. Microbiol. Biotechnol.19, 7265–7282. doi: 10.1007/s00253-021-11500-x
Zhou, J., Liu, Y., Shen, T., Chen, L., Zhang, C., Cai, K., et al. (2019). Enhancing the antibacterial activity of PMAP-37 by increasing its hydrophobicity. Chem. Biol. Drug Des. 94, 1986–1999. doi: 10.1111/cbdd.13601
Keywords: fungal defensin NZX, Staphylococcus aureus, intracellular bactericidal activity, pharmacokinetics, pharmacodynamics, mouse peritonitis model
Citation: Zheng X, Yang N, Mao R, Hao Y, Teng D and Wang J (2022) Pharmacokinetics and Pharmacodynamics of Fungal Defensin NZX Against Staphylococcus aureus-Induced Mouse Peritonitis Model. Front. Microbiol. 13:865774. doi: 10.3389/fmicb.2022.865774
Received: 30 January 2022; Accepted: 13 April 2022;
Published: 01 June 2022.
Edited by:
Josep M. Sierra, University of Barcelona, SpainReviewed by:
Helen Fuchs, Rhode Island Hospital, United StatesCesar de la Fuente-Nunez, University of Pennsylvania, United States
Copyright © 2022 Zheng, Yang, Mao, Hao, Teng and Wang. This is an open-access article distributed under the terms of the Creative Commons Attribution License (CC BY). The use, distribution or reproduction in other forums is permitted, provided the original author(s) and the copyright owner(s) are credited and that the original publication in this journal is cited, in accordance with accepted academic practice. No use, distribution or reproduction is permitted which does not comply with these terms.
*Correspondence: Jianhua Wang, d2FuZ2ppYW5odWFAY2Fhcy5jbg==; d2FuZ2ppYW5odWEucGVraW5nQHFxLmNvbQ==; Da Teng, dGVuZ2RhQGNhYXMuY24=