- 1Department of Environmental Sciences, University of Basel, Basel, Switzerland
- 2Department of Marine Microbiology and Biogeochemistry, NIOZ Royal Institute for Sea Research, Texel, Netherlands
- 3Department of Earth Sciences, Faculty of Geosciences, Utrecht University, Utrecht, Netherlands
Freshwater lakes represent an important source of the potent greenhouse gas methane (CH4) to the atmosphere. Methane emissions are regulated to large parts by aerobic (MOx) and anaerobic (AOM) oxidation of methane, which are important CH4 sinks in lakes. In contrast to marine benthic environments, our knowledge about the modes of AOM and the related methanotrophic microorganisms in anoxic lake sediments is still rudimentary. Here, we demonstrate the occurrence of AOM in the anoxic sediments of Lake Sempach (Switzerland), with maximum in situ AOM rates observed within the surface sediment layers in presence of multiple groups of methanotrophic bacteria and various oxidants known to support AOM. However, substrate-amended incubations (with NO2−, NO3−, SO42−, Fe-, and Mn-oxides) revealed that none of the electron acceptors previously reported to support AOM enhanced methane turnover in Lake Sempach sediments under anoxic conditions. In contrast, the addition of oxygen to the anoxic sediments resulted in an approximately 10-fold increase in methane oxidation relative to the anoxic incubations. Phylogenetic and isotopic evidence indicate that both Type I and Type II aerobic methanotrophs were growing on methane under both oxic and anoxic conditions, although methane assimilation rates were an order of magnitude higher under oxic conditions. While the anaerobic electron acceptor responsible for AOM could not be identified, these findings expand our understanding of the metabolic versatility of canonically aerobic methanotrophs under anoxic conditions, with important implications for future investigations to identify methane oxidation processes. Bacterial AOM by facultative aerobic methane oxidizers might be of much larger environmental significance in reducing methane emissions than previously thought.
Introduction
Methane (CH4) is a powerful greenhouse gas in the atmosphere and a major portion of this gas in aquatic and terrestrial ecosystems is produced biologically in anoxic environments (e.g., anoxic waters and sediments) by anaerobic methanogens. In contrast to oceans, freshwater habitats such as lakes cover only a small portion of the Earth’s surface (Downing et al., 2006), yet, they contribute a significant part to the global emissions of methane to the atmosphere (Bastviken et al., 2004). The comparatively low methane concentrations in both surface and bottom waters of lakes (Blees et al., 2015; Donis et al., 2017) relative to those in anoxic sediments (Blees et al., 2014b) suggest that methane in lake sediments is largely consumed before released to the water column. However, the primary sink for methane in anoxic lake sediments is still not well understood, and the physiological mechanisms and modes of benthic methane oxidation by microbes have not yet been unraveled.
In freshwater lakes, high activities of methane oxidation are often thought to occur at the oxic-anoxic transition zones (Lidstrom and Somers, 1984; Kuivila et al., 1988; Frenzel et al., 1990; Bender and Conrad, 1994; He et al., 2012). Here, methane oxidation is usually carried out by aerobic methane-oxidizing bacteria (MOB), and the methane oxidation with oxygen as electron acceptor (MOx) is considered to be the prime methane sink in lacustrine environments, and thus the mitigator of lacustrine methane emissions. In anoxic lake waters, this pathway can be fueled by oxygen diffusion or intrusion events from the oxic water column (Hanson and Hanson, 1996; Bastviken et al., 2002; Pasche et al., 2011; He et al., 2012; Oswald et al., 2016), or through oxygenic photosynthesis in shallow lakes (Milucka et al., 2015; Oswald et al., 2015; Rissanen et al., 2018). True anaerobic oxidation of methane (AOM) in anoxic environments is usually performed by anaerobic methanotrophic archaea (ANMEs) that are able to couple methane oxidation to the reduction of sulfate (Knittel and Boetius, 2009; Milucka et al., 2012; Wegener et al., 2015). In addition, some archaea were also found to mediate AOM with alternative electron acceptors, such as nitrate (Haroon et al., 2013) and metal oxides (Beal et al., 2009; Ettwig et al., 2016; Cai et al., 2018; Leu et al., 2020), or in a few cases organic electron acceptors, such as humic substances (Scheller et al., 2016; Valenzuela et al., 2019). Methane oxidizing microorganisms may be metabolically quite versatile. For example, recently identified anaerobic methanotrophic archaea in lake sediments, which are phylogenetically closely related to Candidatus Methanoperedens nitroreducens (Haroon et al., 2013), were reported to also perform methane oxidation coupled to sulfate and Mn(IV)-reduction (Su et al., 2020). In addition to archaeal AOM, AOM can be performed by bacteria. Under anoxic conditions, bacteria of the NC10 phylum (i.e., Candidatus Methylomirabilis) can couple aerobic methane oxidation to nitrite reduction (Raghoebarsing et al., 2006; Ettwig et al., 2008), whereby the bacteria produce oxygen for methane oxidation intracellularly via the dismutation of nitric oxide (Ettwig et al., 2010). Thus, nitrite-dependent AOM can employ a pathway similar to that of canonical aerobic methane oxidation, which involves soluble and/or particulate methane monooxygenase enzymes. Such denitrifying methanotrophic bacteria have been recently reported for both lake sediments (Kojima et al., 2012; Deutzmann et al., 2014) and lacustrine water columns (Graf et al., 2018; Mayr et al., 2020; Su et al., 2021). Irrespective of the microbial players involved, in anoxic lake sediments, pathways of AOM like nitrate/nitrite-dependent methane oxidation may be masked by aerobic processes, due to the close proximity of the nitrate/nitrite- and oxygen-consumption zones near sediment-water interfaces, thus making it difficult to distinguish between true AOM and aerobic methane oxidation.
Methane oxidation may be ubiquitous in strictly anoxic lake sediments (Martinez-Cruz et al., 2018), yet, evidence for the occurrence of true AOM (i.e., by the above-mentioned AOM-performing microbes, involving electron acceptors other than oxygen) is still sparse (Crowe et al., 2011; Schubert et al., 2011; Sivan et al., 2011; Norði et al., 2013; Bar-Or et al., 2017; Weber et al., 2017; Su et al., 2020). Moreover, the role of traditionally aerobic methanotrophs in anoxic sediments and their contribution to AOM is still not well understood. Under oxygen limitation, some gamma-proteobacterial MOB (i.e., Methylomonas and Methylomicrobium) seem capable of oxidizing methane with nitrate/nitrite as terminal electron acceptors (Kits et al., 2015a,b), however, the initial attack of methane by the methane monooxygenase still seems to be dependent on oxygen. Similarly, Methylobacter was recently shown to utilize methane for lipid synthesis during long-term anoxic incubations although the responsible electron acceptors for AOM were not investigated (Martinez-cruz et al., 2017). High abundances of Methylobacter were also observed in the deep anoxic hypolimnion of a permanently stratified lake, in association with high methane oxidation rates (Blees et al., 2014b), yet, the exact mechanism or mode of this apparent AOM by aerobic methane oxidizers, and the electron acceptor involved, remained enigmatic. Finally, another putatively gammaproteobacterial lineage, Crenothrix was shown to have the potential to catalyze methane oxidation coupled the reduction of nitrate to N2O under oxygen-deficient conditions (Oswald et al., 2017). It appears that aerobic methanotrophs are versatile in their electron acceptor requirements and are capable to conduct anaerobic respiration in oxygen-deficient or anoxic environments.
In this study, we investigated methane oxidation in anoxic sediments of Lake Sempach in Switzerland, with the particular goal to elucidate the potential electron acceptors and microbial players involved, and to verify whether the apparent anoxic benthic methane sink represents true AOM. Toward this goal, we combined sediment pore water hydrochemical data with in situ AOM rate measurements using radio-labeled methane, as well as slurry incubation experiments to study the impact of alternative oxidants (i.e., sulfate, iron, manganese, nitrite, and nitrate) on AOM. In addition, we characterized the microorganisms that are putatively involved in methane oxidation using approaches including lipid-based stable isotope probing and 16S rRNA gene sequencing.
Materials and Methods
Study Site and Sampling
Lake Sempach is a eutrophic lake located in central Switzerland. For more than three decades, the hypolimnion of this lake has been artificially aerated to maintain oxic bottom water conditions during summer thermal stratification, and to support mixing throughout the water column in winter (Stadelmann, 1988). Alternative oxidants for methane oxidation (e.g., nitrate, nitrite, Fe(III), and Mn(IV)) are found in the shallow sediments and porewaters. In March 2015, sediment cores (inner diameter 62 mm) were recovered with a gravity corer from the deepest site of Lake Sempach. Sediment samples were processed as described previously (Su et al., 2020). In brief, samples for dissolved methane concentrations were collected onsite with cut-off syringes through pre-drilled, tape-covered holes in the sediment core tube. Three milliliter of sediment samples were fixed with 7.0 ml 10% NaOH in 20 ml glass vials, which were then immediately sealed with thick butyl rubber stoppers. Porewater was extracted by centrifuging the 1- or 2-cm segments of a second sediment core under N2 atmosphere, and filtering the supernatant through 0.45 μm filters. Sample aliquots for sulfide concentration measurements were fixed with Zn-acetate (5%) immediately after filtration. Sample aliquots for dissolved iron (Fe2+) and manganese (Mn2+) were fixed with HCl (6 M). The fixed or untreated filtered sample aliquots were then stored at 4°C. Sediment samples for DNA extraction and particulate iron/manganese analyses were collected from a third sediment core, also at 1- or 2-cm intervals, and stored frozen at −20°C until further processing.
Chemical Analyses
Methane concentrations in the headspace of NaOH-fixed samples were measured using a gas chromatograph (GC, Agilent 6890N) with a flame ionization detector, and helium as a carrier gas. Nitrite was determined colorimetrically using the Griess reaction (Hansen and Korolev, 1999). Concentrations of sulfate, nitrate and ammonium were analyzed by ion chromatography (881 IC compact plus, Metrohm, Switzerland). Samples for dissolved iron (Fe2+) and manganese (Mn2+) were analyzed using inductively coupled plasma optical emission spectrometry (ICP-OES), as described before (Su et al., 2020). Sulfide concentrations were analyzed in the laboratory photometrically (Cline, 1969). Reactive iron oxide in the solid phase was extracted using 0.5 M HCl and then reduced to Fe(II) with 1.5 M hydroxylamine. Concentrations of Fe(II) were then determined photometrically using the ferrozine assay (Stookey, 1970). Particulate reactive iron was calculated from the difference between the total Fe(II) concentrations after reduction, and the dissolved Fe(II) in the filtered sample. A total carbon analyzer (Shimadzu, Corp., Kyoto, Japan) was used to quantify dissolved inorganic carbon (DIC) concentrations. The penetration depth of oxygen into the surface sediments was determined using a Clark-type microelectrode sensor (Unisense A/S, Denmark).
AOM Rate Measurements
To determine depth-specific AOM rates in Lake Sempach sediments, the subcore-incubation approach was applied (Su et al., 2019). Briefly, subcores were collected by inserting three small plexiglass tubes (inner diameter 16 mm) into the fresh sediment core, and both ends of the core tubes were sealed with black rubber stoppers. After ~1 h preincubation, 20 μl of 14CH4 solution (~2.5 kBq) was injected through each of the predrilled, silicone-sealed ports at 1.5 cm intervals. Subcores were incubated at in situ temperature (4°C) in the dark for 2 days. At the end of the incubation, subcores were extruded, and subsamples were collected at 1 or 2 cm resolution, and directly transferred into 100 ml vials containing 20 ml of the aqueous NaOH (5% w:w) to terminate microbial activity. Radioactivity in different 14C pools in the incubation samples and AOM rates were determined according to Su et al. (2019).
Substrate-Amended Slurry Incubations
Experiment 1 (Sulfate, Iron, and Manganese-Addition Experiments With 14C-Labeled Methane)
Anoxic slurries of ~8 l were prepared by purging a mixture of sediments (surface 10 cm × four sediment cores, ~1,500 cm3) and autoclaved anoxic artificial sulfate-free medium (Su et al., 2020). Homogenized slurries of ~100 ml were subsequently dispensed into 120-ml serum bottles and sealed with butyl rubber stoppers. For the electron-acceptor amended incubations, stock solutions of sulfate, ferrihydrite and birnessite were added to final concentrations of 2, 5, and 5 mM, respectively. For the inhibitor-addition experiment, molybdate was added to a final concentration of 4 mM. NaOH-killed controls (pH > 13) were also prepared and incubated in parallel. All bottles were further purged with N2 to remove any traces of oxygen. After 24-h preincubation, 1.5 ml pure CH4 was injected into the headspace of each bottle, which was gently shaken for 24 h, resulting in a final concentration of 100 μmol/L in the slurries. Finally, all incubation bottles were transferred into an anoxic chamber and, therein, filled bubble-free with anoxic medium (100 μmol/L CH4) before injecting 14CH4 gas tracer. At different time points (30 and 60 days), incubation samples were stopped by adding 5 ml saturated NaOH after creating 20 ml headspace of N2. AOM rates were analyzed and calculated as described previously (Su et al., 2019).
Experiment 2 (Nitrite-, Nitrate-Addition, and Oxic Experiments With 13C-Labeled Methane)
To further test specifically the role of nitrite/nitrate in AOM, we performed slurry incubation experiments with a mixture of sediment and anoxic bottom lake water taken in November 2020. Briefly, 100 ml mixed slurries (1.5 g dw sediment per 120-mL bottle) were degassed with He to remove any traces of oxygen and background methane before transfer to an anoxic bag with continuous N2 flow. Five milliliter of pure 13C-labeled methane (99.8 atom %, Campro Scientific) was injected into the headspace of the bottles, which were amended with anoxic stock solutions of nitrite and nitrate, with final concentrations of 0.48 mM and 2.4 mM, respectively. Incubations took place at room temperature under N2 atmosphere. Nitrite and nitrate were replenished, when consumed or when they remained at low concentrations. Killed controls (autoclaved and 13CH4), live controls (only 13CH4) and controls with only 13C-labeled bicarbonate were also incubated in parallel under the same conditions. For oxic experiments, slurries were spiked with pure oxygen and then injected with 5 ml of pure 13CH4. Oxygen was replenished regularly to maintain oxic condition in the incubation bottles. At different time points, incubation slurries were homogenized and 5 ml of the supernatant was collected under N2 atmosphere, and filtered with a 0.45 μm membrane filter for subsequent sulfate, nitrate, nitrite, DIC concentration measurements, and stable carbon isotope ratio analyses to determine the transfer of 13C into the product DIC pool. For the latter, 0.2–1 ml of water sample was transferred into a 12 ml He-purged exetainer (Labco Ltd.) containing 200 μl zinc chloride (50% w/v), and then acidified with ~200 μl 80% H3PO4. The liberated CO2 was then analyzed in the headspace using a purge-and-trap purification system (Gas Bench) coupled to a GC-IRMS (Thermo Scientific, Delta V Advantage). Absolute 13C-DIC concentrations were determined from the DIC concentrations and the 13C/12C ratios converted from δ13C-DIC values. The temporal change in 13C-DIC with incubation time was then used to calculate slurry-incubation-based potential methane oxidation rates. Temporal solute-concentration and 13C-DIC changes were monitored over a total experimental period of 48 days. Thereafter, incubations were continued without further subsampling. After a total incubation period of approximately 5 months, slurry incubations were sacrificed for lipid analysis and DNA extraction (see below).
Microbial Lipid Extraction and Analysis
At the end of the incubation with 13CH4 (Experiment 2), after 160 days, triplicate slurries were combined and freeze-dried. Samples were then homogenized for lipid extraction using an Accelerated Solvent Extraction system (ASE 350, Dionex Corp., Sunnyvale, CA, United States). Samples were extracted with a solvent mixture of dichloromethane and methanol (9:1, vol/vol) over three extraction cycles at 100°C and maximum pressure of 1,500 psi. Total lipid extracts (TLEs) were collected in 60 ml glass vials, and concentrated using a vacuum evaporator system (Rocket Synergy Evaporator, Genevac Ltd., Ipswich, United Kingdom). Prior to extraction, an internal standard mix (5α-Cholestane, Nonadecanoic acid, and 1-Nonadecanol) was added to each sample for the quantification of individual biomarkers. The TLEs were further evaporated to dryness and then saponified with methanolic KOH-solution (12%) at 80°C for 3 h. Neutral compounds were extracted by liquid–liquid extraction using hexane. After extracting the neutral fraction, fatty acids (FAs) were extracted from the remaining aqueous phase with hexane after acidification (pH ~1). The fatty acid fraction was methylated using BF3 in methanol (14% v/v, Sigma Aldrich) at 80°C for 2 h, and analyzed later as fatty acid methyl esters (FAMEs). Neutral compounds were further separated into hydrocarbon and alcohol fractions over silica glass gel columns, as described by Sicre et al. (1994) with the following modifications. The neutral fraction was dissolved in heptane and transferred onto the wet column. Aliphatic, cyclic, and aromatic hydrocarbons were eluted with 2 ml heptane and 2 ml heptane/toluene (3:1 v/v), ethers and ketones with 2 ml heptane/toluene (1:1 v/v) and 2 ml heptane/ethyl acetate (95:5 v/v), and finally alcohols with 2 ml heptane/ethyl acetate (85:15 v/v) and heptane/ethyl acetate (80:20 v/v). The alcohol fraction was methylated with 100 μl pyridine and 50 μl bis(trimethylsilyl)trifluoracetamide (BSTFA) at 70°C for 1 h.
All fractions were quantified using a TRACE GC Ultra gas chromatograph equipped with a flame ionization detector (GC-FID; Thermo Scientific, Waltham, MA, United States), as described previously (Blees et al., 2014a). Individual lipid compounds were quantified by normalization to the internal standard, and identified by comparing their retention times to those of laboratory standard mixtures, or by gas chromatograph mass spectrometry (GC–MS, Thermo Scientific DSQ II Dual Stage Quadrupole), and the acquired mass spectra were identified through comparison with published data. The double-bond positions of monounsaturated FAs were determined by analyzing their dimethyl disulfide adducts, as described before (Su et al., 2020). Compound-specific stable carbon isotope ratios of individual compounds were determined using a gas chromatograph with split/splitless injector, connected on-line via a GC-Isolink combustion interface to a ConFlo IV and Delta V Advantage isotope ratio mass spectrometer (GC-IRMS, Thermo Scientific, Bremen, Germany). The concentrations of lipids were calibrated by internal standards of known concentrations, and their δ13C values were corrected for the introduction of carbon atoms during derivatization.
DNA Extraction, PCR Amplification, Illumina Sequencing, and Data Analysis
DNA was extracted from both samples of Lake Sempach core sediments and from the slurry sediments at the end of incubations (Experiment 2), using a FastDNA SPIN Kit for Soil (MP Biomedicals) following the manufacturer’s instructions. A two-step PCR approach was applied in order to prepare the library for sequencing at the Genomics Facility Basel. Briefly, a first PCR (25 cycles) was performed using primers 515F-Y and 926R targeting the V4 and V5 regions of the 16S rRNA gene (Parada et al., 2016). Sample indices and Illumina adaptors were added in a second PCR of eight cycles. Purified indexed amplicons were finally pooled at equimolar concentration into one library, and sequenced on an Illumina MiSeq platform using the 2 × 300 bp paired-end protocol (V3 kit). The initial sequence data treatment was performed by J. C. Walser at the Genetic Diversity Centre (GDC), ETH Zurich. After sequencing, quality of the raw reads was checked using FastQC (v 0.11.8; Andrews, 2018). Paired-end read merger (usearch v11.0.667_i86linux64) was used to merge forward and reverse reads into amplicons of about 419 bp length, allowing a minimum overlap of 40 nucleotides and a mismatch density of 0.25. Quality filtering (min Q20, no Ns allowed) was carried out using PRINSEQ (Schmieder and Edwards, 2011). Amplicon sequence variants were determined by denoising using the UNOISE algorithm (UNOISE3, usearch v11.0.667_i86linux64) and are herein referred to as ZOTU (zero-radius OTU). Taxonomic assignment was done using SINTAX v11.0.240_i86linux64 (Edgar, 2016) and the SILVA 16S rRNA reference database v128 (Quast et al., 2013). Subsequent data analyses were carried out with Phyloseq (McMurdie and Holmes, 2013) in the R environment (R Core Team, 2014).1
Results
Rates of AOM and Geochemistry of Lake Sempach Sediments
Concentration of methane in the sediments increased with depth, reaching a maximum of ~1.75 mM at 10 cm depth. Methane oxidation was observed throughout the sediment core. Activity decreased with depth (Figure 1A), with the maximum AOM rates (68.0 ± 19.0 nmol cm−3 d−1) measured in the upper 0–2 cm (Figure 1B). Nitrate was only detected above 3 cm (up to 5 μM) and the sulfate concentration decreased downward from 78 μM at the sediment–water interface to 7 μM at 10 cm (Figure 1C). Both sulfide and nitrite were below the detection limit at all depths. Reducible iron and manganese oxides were most abundant in surface sediments (24.3 and 30.8 μmol g−1 wet sediment respectively), and concentrations of both dissolved iron (Fe2+) and manganese (Mn2+) increased steadily with depth (Figures 1D,E). Even under the condition of fully oxygenated bottom waters, oxygen was rapidly consumed in the uppermost sediment layers and penetrated to a maximum depth of 3 mm (Figure 1F).
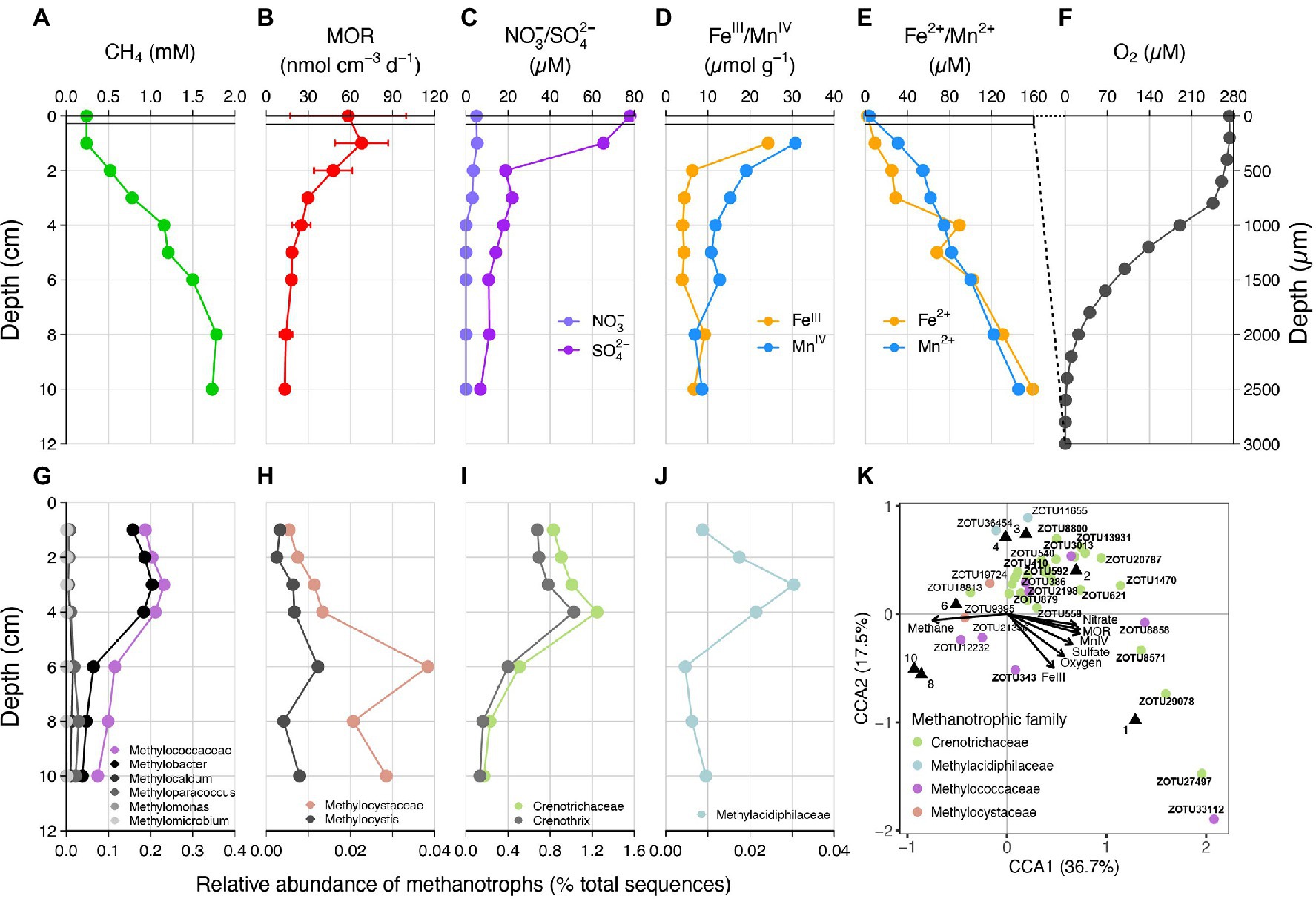
Figure 1. (A–F) Depth profiles of methane, methane oxidation rates (MOR), porewater nitrate/sulfate, particular iron(III)/manganese(IV) oxides, dissolved iron(II)/manganese(II) and oxygen in the sediment of Lake Sempach. Error bars represent standard deviations of triplicate rate measurements. No nitrite was detected. (G–J) Relative abundances of known families of metahanotrophes and their subgroups at the genus level: (G) Methylococcaceae or Type I gamma-proteobacterial MOB, (H) Methylocystis or Type II alpha-proteobacterial MOB, (I) Crenotrichaceae, (J) Methylacidiphilaceae, (K) canonical correspondence analysis (CCA), showing the relationship between MOR and methanotrophic species and the effect of nutrient concentrations on their distribution. ZOTU abundances were normalized and Hellinger-transformed prior to CCA analysis. A total of 42 ZOTUs accounting for > 92% of the methanotrophic assemblage were used for the analysis, and bold text represents taxa belonging to the genus Methylobacter or Crenothrix. Black triangles denote sediment samples at different depths.
Diversity and Abundance of Methanotrophs in Lake Sediments
Analysis of sediment microbial community, based on 16SrRNA gene sequencing, revealed the presence of multiple groups of aerobic methanotrophs, including Type I gamma- and Type II alpha-proteobacterial MOB (Figures 1G,H; Supplementary Table 1). Type I MOB consist of Methylococcaceae, of which the genus Methylobacter was the dominating cluster. The relative abundances of Methylococcaceae ranged from 0.07% to 0.23%, with peak abundance occurring at 3 cm depth. In comparison, Type II alpha-MOB Methylocystis was found at much lower relative abundances, with a maximum of 0.01% observed at 6 cm depth. A large number of 16S rRNA gene sequences (0.17%–1.25%, Figure 1I) belonged to Crenotrichaceae, of which Crenothrix was the predominant genus with amplified sequence variants sharing high sequence similarities (96%–100%) with what has been described recently as major methane oxidizers in stratified lakes (Oswald et al., 2017). In addition, a very small proportion of sequences (up to 0.03%) were affiliated with Methylacidiphilaceae within the Verrucomicrobia phylum. Methylacidiphilaceae were reported to perform aerobic methane oxidation in acidic geothermal environments (Dunfield et al., 2007). A canonical correspondence analysis (CCA) was performed to identify any given correlation between the concentrations of potential electron acceptors, methane oxidation rates, and specific methanotrophic taxa (Figure 1K). The CCA triplot shows that members belonging to Crenothrix or Methylobacter were positively correlated to the methane oxidation rates, and negatively correlated to methane concentrations. The plot also demonstrates that Crenothrix and Methylobacter were more likely to be found in surface sediments with higher concentrations of potential electron acceptors (i.e., oxygen, nitrate, sulfate, iron, and manganese).
Effect of Potential Electron Acceptor Addition on AOM Rates
To investigate the potential electron acceptors for AOM, sediments of the top 10 cm (i.e., where in situ rates were measured; Figure 1B) were incubated with different electron acceptors. In the first experiment with 14C-labeled methane, no apparent increase in AOM rate was observed with any potential electron acceptor amendment, compared with the live controls (no electron acceptors added; Figure 2A). In fact, incubations of both 30 and 60 days revealed very similar potential AOM rates between the untreated controls and amendments with sulfate and molybdate. However, the addition of iron oxides to the slurries did not stimulate methane oxidation but rather resulted in systematically lower AOM rates relative to the control experiments. Moreover, in incubations with added manganese oxide, methane oxidation was completely inhibited and substantial amounts of sulfate were produced (Figure 2B). In the second experiment using 13C-labeled methane, we further tested the role of nitrate/nitrite in AOM, in direct comparison with aerobic methane oxidation after O2 injection. Similar to 14C-labeled methane incubation experiments, AOM occurred in the anoxic live controls (without any electron acceptors added). Incubations amended with nitrate and nitrite showed similar trends with respect to the control, hence, there was no obvious stimulation of methane oxidation by either of the NOx compounds (Figure 3). For both untreated controls and nitrate/nitrite amendments, AOM rates were low during the first 4 days of the incubation period, but then increased dramatically between 4 and 8 days. Thereafter, they remained relatively low again (i.e., only subtle 13DIC accumulation between Day 8 and the end of the incubation). In contrast, methane oxidation was linear throughout the incubation period under oxic conditions, and the rates were almost an order of magnitude higher than the maximum rate in the control and NOx-amended incubations. Strikingly, sulfate was fully depleted after 8 days in the live controls (but not in the killed control), whereas there was extensive sulfate production in incubation bottles amended with nitrate or oxygen. When nitrite was added, net sulfate production was also observed, at least during some phases (e.g., initially and between days 17 and 40; Figure 3D), and at lower rates.
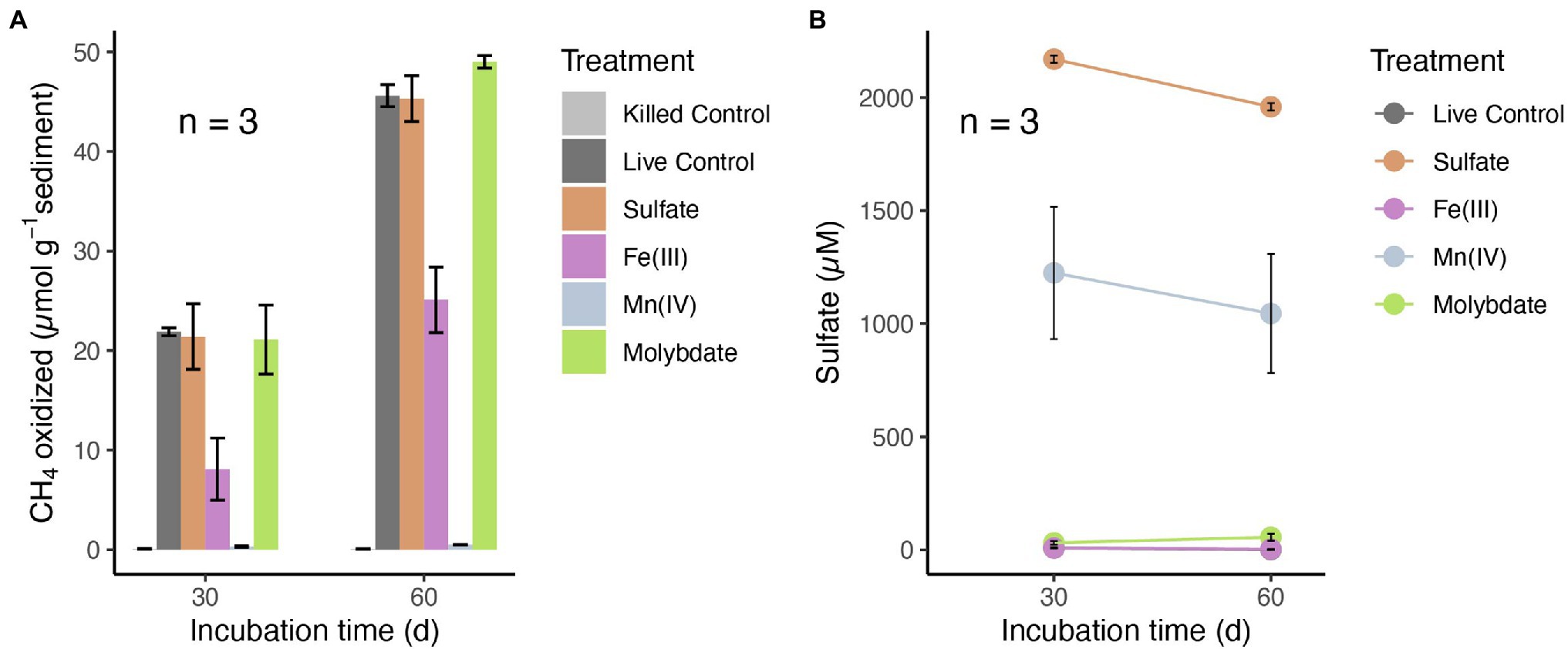
Figure 2. (A) Methane oxidation in anoxic slurries with 14C-labeled methane and different electron acceptors: sulfate (2 mM), amorphous iron(III) oxides (5 mM) and manganese(IV) oxides (5 mM) after 30 and 60 days, respectively. (B) Sulfate concentrations in the incubation supernatants of different treatments after 30 and 60 days. Control incubations were conducted without electron acceptors added. No methane oxidation rates were detected in base-killed controls (pH > 13). Error bars represent standard error of the mean (n = 3). Sulfate was below or close to the detection limit in the live controls and in incubations with Fe(III) (points are overlapping).
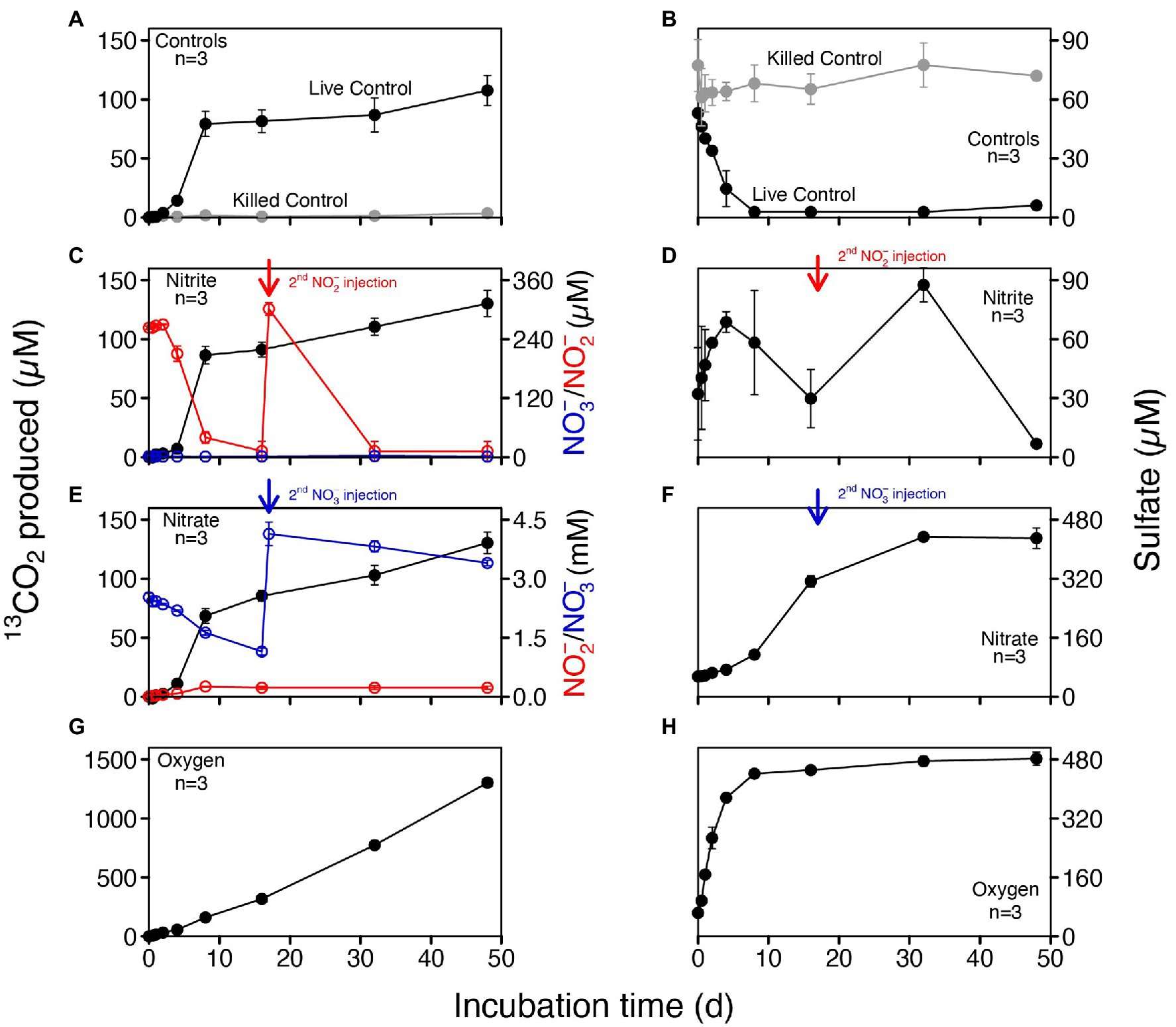
Figure 3. Concentrations of produced 13CO2 and sulfate in anoxic slurries amended with 13C-labeled methane and different electron acceptors (nitrite, nitrate and oxygen). (A,B) Controls including killed controls (autoclaved) and live controls (without electron acceptors added). (C,D) Nitrite addition; nitrite was replenished after 17 days. (E,F) Nitrate addition; nitrate was replenished after 17 days. (G,H) Oxic incubations. No nitrite and nitrate were detected in both controls and slurries with oxygen.
Lipid Biomarkers in Incubations With 13CH4
At the end of the 13CH4 incubations (after 160 days), the abundance and carbon isotopic composition of MOx/AOM diagnostic biomarkers (specific fatty acids and alcohols) were examined in the slurry sediments to constrain the methanotrophs involved and to infer carbon flow through the microbial community. The overall concentrations of lipid biomarkers in the different treatments were quite similar, except for incubations with oxygen, where concentrations were somewhat lower than in the other treatments (Figure 4). In the untreated control and the nitrite-amended experiment, the most strongly 13C-enriched fatty acids were monounsaturated hexadecanoic acids (C16:1ω7 and C16:1ω5) and (ω-1)-OH-C28:0, with δ13C values ranging from 531‰ to 1,558‰. By comparison, the highest 13C incorporation in incubations with nitrate was observed for (ω-1)-OH-C28:0 (1524‰), followed by 10-Methyl-hexadecanoic acid (10-Me-C16:0, 367‰). Most strikingly, δ13C analyses of biomarker extracts from the oxic incubations revealed significantly stronger 13C labeling of most fatty acids (compound-specific δ13C values between 6,800‰ and 14,500‰; Figure 4M) compared to the controls and to incubations with nitrite/nitrate. In order to assess concurrent autotrophic carbon assimilation, we performed additional slurry incubations with 13C-labeled bicarbonate only. No 13C incorporation at all was observed for (ω-1)-OH-C28:0 (−39‰), and the δ13C value of 10-Me-C16:0 (164‰) suggests only minor 13C-label incorporation. Generally, δ13C values of compounds diagnostic for methanotrophic microorganisms in incubations with 13C-bicarbonate were substantially lower than in the live controls and those 13CH4 incubations with nitrite/nitrate (Figure 4I).
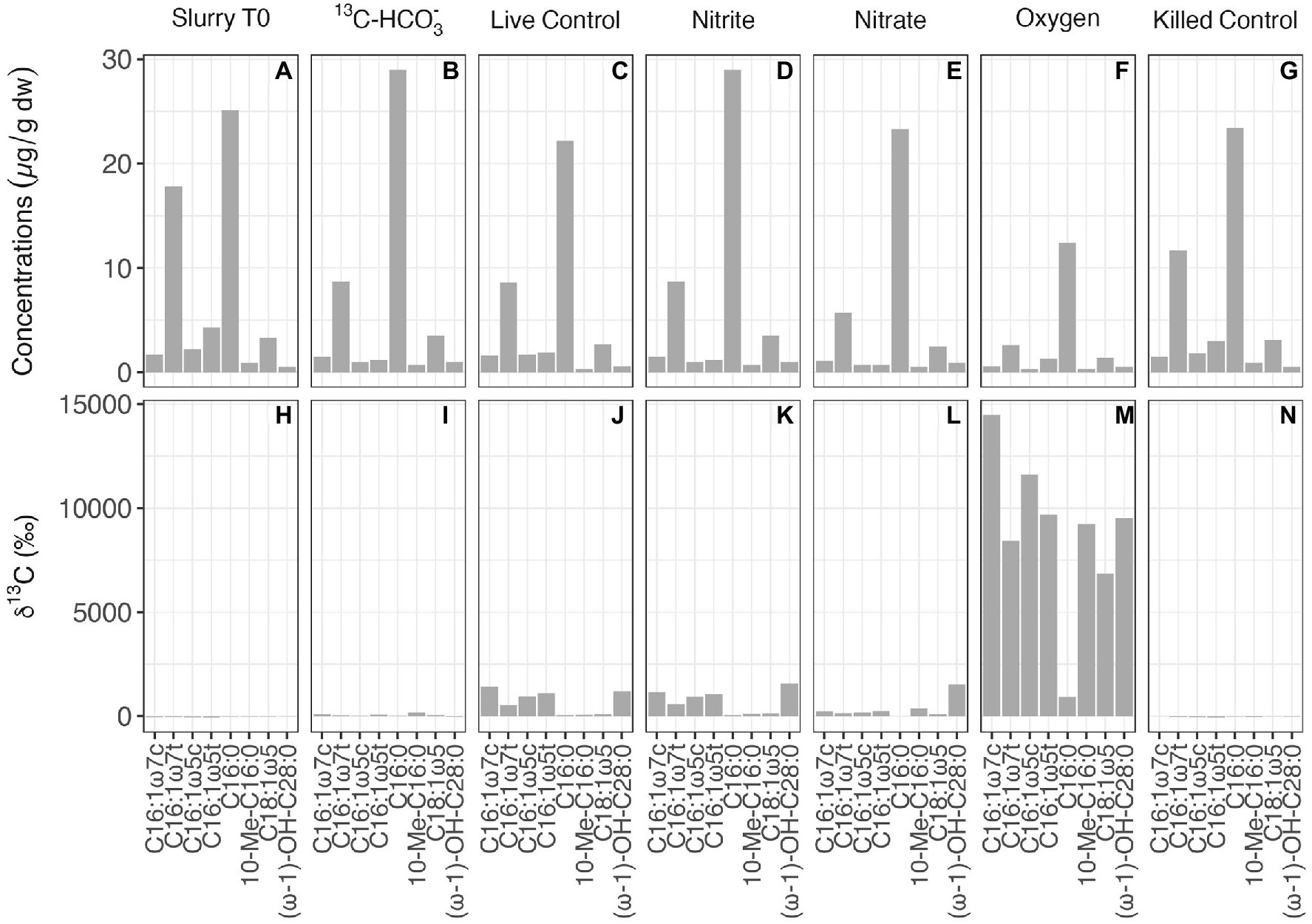
Figure 4. Concentrations (A–G) and compound-specific δ13C values (H–N) of fatty acids diagnostic for putative methanotrophs in the initial slurry sample (Slurry T0), and in 13CH4 incubations with different treatments after 160 days.
Microbial Communities in Incubations With 13CH4
Woesearchaeota and Euryarchaeota were the most abundant archaeal phyla in the beginning of the incubation (Figure 5A). The archaeal community structure in both the live control and incubation with 13C-bicarbonate, after 160 days of incubation remained similar to that of Slurry T0. No sequences of typical anaerobic archaeal methanotrophs such as ANME-1, −2, −3 and Candidatus Methanoperedens have been detected. As for bacterial composition at the class level (Figure 5B), Gammaproteobacteria comprised a smaller proportion after nitrite/nitrate and oxygen additions. In oxic slurries, Alphaproteobacteria accounted for a larger proportion of the bacterial community compared to the pre-incubation situation. At family level, Crenotrichaceae and Methylococcaceae were the dominant groups among the known methanotrophs in the initial slurry sample (Figure 5C), consistent with the diversity and abundance of methanotrophs in the sediment profiles (see Figures 1G–J). After 160 days, Crenotrichaceae and Methylococcaceae remained dominant within the known methanotrophic families in all the treatments. Although representing a minor portion of the methanotrophs, the relative abundances of Methylocystis slightly increased in incubations with nitrate/nitrite and oxygen when compared to Slurry T0. In oxic slurries, the fractional abundance of Methylococcaceae was higher relative to Slurry T0 and the other treatments, and the dominance of Crenotrichaceae seemed to be reduced (Figure 5C). In addition, the anaerobic nitrite-dependent methanotroph Candidatus Methylomirabilis sp. was detected in the incubation with nitrate situation at a low relative abundance (1.36% of known methanotrophs; Figure 5C).
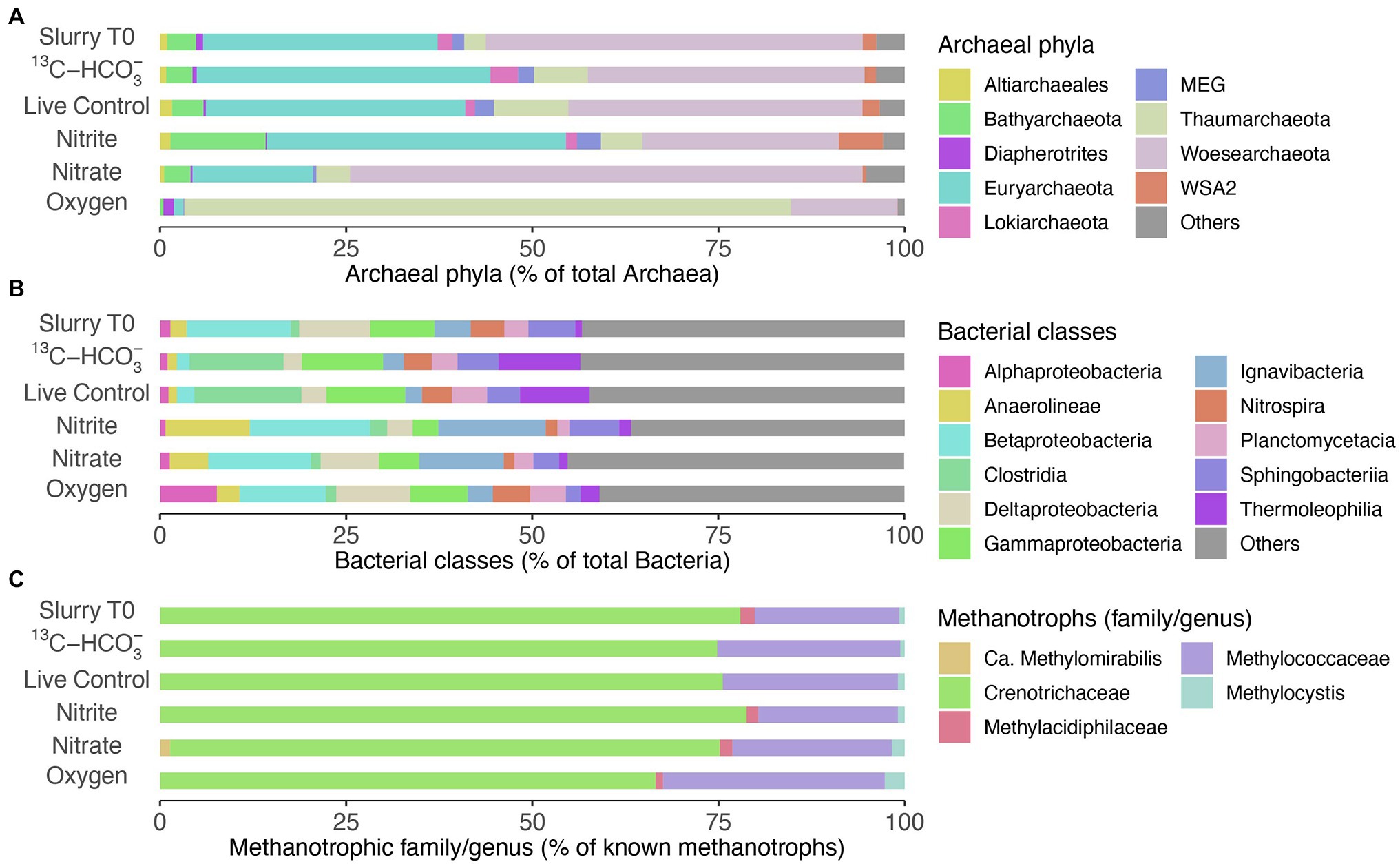
Figure 5. Microbial communities in slurry samples before (Slurry T0) and after incubation (160 days) with different treatments. (A) Relative abundances of the most abundant phyla within the Archaea (>90% of total archaeal sequences). (B) Relative abundances of top bacterial classes (>50% of total bacterial sequences). (C) Fractional abundances of known methanotrophic families/genera, Methylocystis was dominant within the family Methylocystaceae. Data are based on read abundances of 16S rRNA gene sequences.
Discussion
In Lake Sempach, despite the continuous aeration of the deep hypolimnion, oxygen is rapidly depleted in the uppermost sediment layers (maximum O2 penetration depth of 3 mm). Based on the measured methane turnover rates as a function of depth (Figure 1B), maximum methane oxidation activity was located at depths near the water–sediment interface, but apparent AOM (though at lower rates) was also indicated in the deeper, anoxic sediment layers. This is different from marine and some exceptional freshwater settings (e.g., Su et al., 2020), where highest AOM rates occur within the sulfate methane transition zones, well below the sediment surface (Iversen and Jørgensen, 1985) or close to the surface sediments at cold methane seeps (Elvert et al., 2000). Within oxic-anoxic transition zones of lake sediments, oxygen likely serves as the most important electron acceptor and aerobic methanotrophs play a dominant role in reducing the overall methane fluxes (Bender and Conrad, 1994; He et al., 2012). In anoxic sediments, however, the pathway of methane oxidation with regard to the electron acceptors involved remains to be fully explored. Notably, the highest AOM rates in surface sediment layers corresponded to the concentration maxima of nitrate, sulfate, iron(III)- and manganese(IV)-oxides, suggesting their potential roles for AOM. Despite the clear evidence of AOM in anoxic zones, no ANME-1 or 2, key microbial drivers of AOM in marine settings, were detected throughout the sediment core by 16S rRNA gene amplicon sequencing with primers that match with a large fraction of the deposited sequences of anaerobic methanotrophic archaeal clades (Su et al., 2020). Neither could we detect Ca. Methylomirabilis nor Ca. Methanoperedens (Ettwig et al., 2010; Haroon et al., 2013; Su et al., 2020). Instead, the anoxic sediments contained high abundances of gamma-MOB and some alpha-MOB, which are both traditionally considered as aerobic methanotrophs (Hanson and Hanson, 1996; Stoecker et al., 2006). Most importantly, the sediments contained high relative abundances of Crenothrix, also gamma-MOB thought to be aerobes (i.e., requiring oxygen for methane activation), and regularly found as contaminant in drinking water systems (Hanson and Hanson, 1996; Stoecker et al., 2006). The relative abundances of Methylococcaceae, Crenothrix and Methylacidiphilaceae, respectively, were higher in upper sediment layers, while Methylocystaceae, or more precisely, Methylocystis were more abundant in lower portions of the sediments. The vertical distribution of various methanotrophic groups may indicate their different affinities toward methane, as evidenced, at least in part, by some studies showing that Type I methanotrophs were able to outcompete Type II MOB at low concentrations of methane (Amaral and Knowles, 1995; Henckel et al., 2000). However, the presence of diverse aerobic methanotrophs in anoxic sediments led us to speculate that some of them, if not all, might have an alternative anaerobic lifestyle.
Indeed, increasing evidence has demonstrated the occurrences of gamma-MOB in anoxic lake waters and sediments (Blees et al., 2014b; Milucka et al., 2015; Oswald et al., 2016; Martinez-cruz et al., 2017; van Grinsven et al., 2021). In O2-free incubations with Lake Sempach sediments, the incorporation of the 13CH4-carbon into specific lipid biomarkers provides clear evidence for microbial methane assimilation. The monounsaturated fatty acids C16:1ω7 and C16:1ω5, which are typically indicative of Type I aerobic methanotrophs (Bowman et al., 1994; Hanson and Hanson, 1996), were most enriched in 13C. In theory, the 13C enrichment could also be attributed to the assimilation of 13CO2 from methane oxidation into the biomass by autotrophic microorganism, as for example found for certain sulfate reducing bacteria (Wegener et al., 2008). Indeed, it has been reported that specific lipids such as C16:1ω7 and C16:1ω5 are associated with SRB that were involved in sulfate-dependent AOM at methane-rich seeps (Elvert et al., 2003). However, no, or very low, incorporation of 13C into these lipid biomarkers was observed in the incubation with 13C-labeled bicarbonate only. This indicates that the 13C-enriched lipids in the 13CH4 incubations were not derived from bicarbonate-assimilating SRB, but can rather be attributed to the direct assimilation of methane-derived carbon by Type I gamma-MOB. Direct uptake of 13C-labeled methane by alpha-MOB in the anoxic sediments was also observed, evidenced by the strong 13C-enrichment of long-chain (ω-1)-hydroxy fatty acids, such as (ω-1)-OH-C28:0. This lipid compound is mainly found in Type II methane-utilizing bacteria including Methylosinus and Methylocystis (Skerratt et al., 1992). Hence, the observed 13C incorporation into these compounds is consistent with our findings regarding the composition of the methanotrophic community in the live control incubation slurry, which comprised relatively high abundances of both Methylococcaceae and Methylocystaceae. Methylococcaceae were dominated by Methylobacter and Methylocystis was the most abundant genus within Methylocystaceae (data not shown). The molecular data combined with the finding of 13C-enriched lipid biomarkers further confirmed methane metabolism by Type I and Type II MOB in absence of oxygen. However, we were not able to verify methane assimilation into the biomass of Crenothrix, because no diagnostic lipids have been reported for them to date. Moreover, Crenotrichaceae likely also include bacteria that are not strict methanotrophs. Yet, due to the apparent capacity of at least some of the Crenotrichaceae genera to also oxidize methane anaerobically (Oswald et al., 2017), and given their high relative abundances in the anoxic sediments, however, we speculate that this methanotrophic group may comprise the most important methane consumers in Lake Sempach sediments. Overall, our molecular data and lipid biomarker analysis provide multiple lines of evidence that AOM in Lake Sempach sediments is most likely mediated by methanotrophs that are canonically considered aerobes.
According to the CCA triplot (Figure 1K), members of Crenothrix and Methylobacter were more abundantly found at sediment depths with higher methane oxidation rates, and were associated with elevated concentrations of potential alternative electron acceptors in the anoxic sediments. However, in none of the electron acceptor-amended slurry incubations, i.e., neither with 13CH4 (nitrite/nitrate added) nor with 14CH4 (sulfate, iron Fe- or manganese Mn-oxides added), stimulation of anaerobic methane turnover by any of the common anaerobic electron acceptors was indicated. This suggests an AOM pathway in Lake Sempach sediments that may be different from AOM reported for most investigated systems (Boetius et al., 2000; Orphan et al., 2002; Raghoebarsing et al., 2006; Vaksmaa et al., 2017; Su et al., 2020). In fact, the addition of metal oxides led to systematically lower AOM rates (iron) or complete inhibition (manganese). These observations appear, at first sight, at odds with recent studies showing the stimulation of both iron and manganese additions for aerobic methanotrophs under anoxic conditions (Oswald et al., 2016; Bar-Or et al., 2017; Zheng et al., 2020). Yet, the inhibition of AOM as a result of excess “anaerobic electron acceptors” was also observed in previous studies (Segarra et al., 2013; Milucka et al., 2015; Rissanen et al., 2018). It is likely that the addition of metal oxides to the slurries changed the redox condition of the systems, and favored the growth of iron- or manganese-utilizing microorganisms that could outcompete methane oxidizers for essential nutrients, but further investigation is needed to reveal their effect on AOM. Notably, in 13CH4 incubations without electron acceptors added, the co-occurrence of increasing 13CO2 and decreasing sulfate concentrations over the first 8 days of incubation suggests a possible link between methane oxidation and sulfate reduction, yet other lines of evidence suggest that this was not the case. That is, the relatively large amounts of sulfate produced in nitrate-amended bottles, likely by the oxidation of sulfide with nitrate (Su et al., 2020), did not stimulate 13CO2 production significantly, when compared to the live controls, suggesting that sulfate was at least not a limiting electron acceptor for AOM in Lake Sempach sediments. That sulfate reduction is, in fact, not directly involved in driving AOM in Lake Sempach sediments is further supported by the observation that similar methane turnover rates were measured for incubations with and without molybdate, a competitive inhibitor for microbial sulfate reduction (Wilson and Bandurski, 1958).
Despite the fact that methanotrophic bacteria are typically aerobes, some of them seem to be able to survive long periods of oxygen starvation (Roslev and King, 1995; Blees et al., 2014b), and may even switch from respiring oxygen to nitrite or nitrate for methane oxidation, or fermentation (Kits et al., 2015a,b; Oswald et al., 2017; van Grinsven et al., 2021). Both Type I MOB and Crenothrix were detected in our incubations, and their preference for O2 as oxidant of methane was clearly demonstrated for the Lake Sempach sediments (Figure 3G). However, how they (and/or other methane oxidizing microorganisms in the Lake Sempach sediments) drive methane oxidation in the absence of O2 remains uncertain. As outlined before, AOM coupled to nitrite or nitrate respiration seemed unlikely as neither of these electron acceptors enhanced methane oxidation rates significantly, although we cannot completely rule out this mode of AOM. While nitrite-dependent Ca. Methylomirabilis was not detected at all in any of the nitrite additions, it was present after incubation with nitrate addition, though at very low abundance. This finding is also consistent with the lipid data for the nitrate-amended anoxic incubations, which revealed a stronger 13C enrichment in the fatty acid 10-methylhexadecanoic acid (10-Me-C16:0), putatively diagnostic for Ca. Methylomirabilis oxyfera (Kool et al., 2012). It is plausible that these denitrifying methanatrophic bacteria contributed a small portion to the overall methane oxidation.
At this point, based on our slurry incubation results, we are not able to better constrain the electron acceptors involved in AOM in Lake Sempach sediments, and we can only speculate about what sustains the observed methane turnover in anoxic incubations. A recent study suggests that AOM can proceed in the absence of inorganic electron acceptors, via “reverse methanogenesis” (Blazewicz et al., 2012). This AOM pathway may offer a possible explanation for the observed “ineffectiveness” of all tested electron acceptors to stimulate AOM. Alternatively, other electron acceptors that were already present in the lake sediments (e.g., organic matter) could be involved. Indeed, humic substances have been recognized as a viable electron acceptor through the reduction of their quinone moieties (Lovley et al., 1996; Scott et al., 1999), and organic electron acceptors such as humic acids were suggested to play a role in AOM in wetlands (Smemo and Yavitt, 2011; Blodau and Deppe, 2012; Valenzuela et al., 2019). In addition, anaerobic methanotrophic archaea in marine sediments were recently demonstrated to use humic-substance analogs (i.e., AQDS and humic acids) as electron acceptors in the absence of sulfate (Scheller et al., 2016). If humic (or similar organic) substances can indeed serve as methane oxidizing agent, and given the widespread distribution of these compounds in freshwater environments as important component of the sedimentary organic matter pools (Ishiwatari, 1985), such mode of AOM may play an underappreciated role in reducing methane emissions in anoxic lake sediments. Clearly, further investigations are required to explore their role in lacustrine AOM.
Most strikingly, additions of oxygen to our incubations with 13CH4 resulted in an approximately tenfold increase in 13CO2 production relative to the live controls or amendments with nitrite/nitrate. This clearly shows that aerobic methanotrophs were actively involved in methane oxidation, when replenished with oxygen, and methane metabolism of these methanotrophic bacteria was evidenced by the substantial 13C-enrichment of diagnostic fatty acids. Our results thus highlight that there can be a strong potential for bacterial AOM in anoxic sediments, which, at least in Lake Sempach, seems to be mostly sustained by aerobic gamma proteobacterial Type I MOB and Crenothrix. Cryptic oxygen production and the effect on MOx (Milucka et al., 2015) was excluded since the incubations were kept in the dark. The fact that these microbes are found in sediments where O2 is fully depleted, and that they can resume full aerobic metabolic activity upon the addition of O2, attests to their resilience against O2 limitation. We propose that a potential capacity to switch to anaerobic modes of methane oxidation or fermentation may be key to this resilience, allowing them to metabolize also in the absence of O2, as genomically evidenced by a recent study in arctic lake sediments (He et al., 2021).
Methane carbon assimilation rates in the oxic incubations were an order of magnitude higher than those in anoxic incubations. This is expected, since aerobic respiration provides much more energy for growth than respiration with alternative electron acceptors. While the result of the oxic incubation experiment confirm that aerobic methane oxidation is much more efficient than AOM in consuming methane, it needs to be noted that the vast majority of methane is produced, and accumulates, in the anoxic part of lake sediments. Here, AOM, just as in the marine environment, may serve as the important first step in a two-component benthic methane filter system. In the context of this ecosystem function, the metabolic versatility and apparent capacity of canonically aerobic methanotrophs to perform AOM has important implications for the natural mitigation of CH4 emission from lake waters to the atmosphere.
Data Availability Statement
The datasets presented in this study can be found in online repositories. The names of the repository/repositories and accession number(s) can be found at: https://www.ncbi.nlm.nih.gov/, SRR15689022–SRR15689034.
Author Contributions
GS, JZ, and ML conceived the research. GS performed all the experiments, data analyses and interpretation with support from JZ, ML, and HN, and wrote the manuscript with comments and inputs from ML, JZ, and HN. All authors contributed to the article and approved the submitted version.
Funding
This research was supported by the China Scholarship Council (CSC). Additional funds came from the Department of Environmental Sciences, University of Basel.
Conflict of Interest
The authors declare that the research was conducted in the absence of any commercial or financial relationships that could be construed as a potential conflict of interest.
Publisher’s Note
All claims expressed in this article are solely those of the authors and do not necessarily represent those of their affiliated organizations, or those of the publisher, the editors and the reviewers. Any product that may be evaluated in this article, or claim that may be made by its manufacturer, is not guaranteed or endorsed by the publisher.
Acknowledgments
The authors would like to thank Thomas Kuhn, Axel Birkholz, and Judith Kobler Waldis for technical support in the laboratory. The authors are grateful to Ruth Strunk and Nikolaus Kuhn for their help with DIC concentrations measurements. Markus Elvert and Nikolai Pimenov are thanked for their thoughtful comments on an earlier version of the manuscript. Robert Lovas, Jana Tischer, Adeline Cojean, and Yuki Weber are acknowledged for their great help during the campaigns on Lake Sempach.
Supplementary Material
The Supplementary Material for this article can be found online at: https://www.frontiersin.org/articles/10.3389/fmicb.2022.864630/full#supplementary-material
Footnotes
References
Amaral, J. A., and Knowles, R. (1995). Growth of methanotrophs in methane and oxygen counter gradients. FEMS Microbiol. Lett. 126, 215–220. doi: 10.1111/j.1574-6968.1995.tb07421.x
Andrews, S. (2018). FastQC: a quality control tool for high throughput sequence data. Available at: https://www.bioinformatics.babraham.ac.uk/projects/fastqc/ (Accessed July 27, 2021).
Bar-Or, I., Elvert, M., Eckert, W., Kushmaro, A., Vigderovich, H., Zhu, Q., et al. (2017). Iron-coupled anaerobic oxidation of methane performed by a mixed bacterial-archaeal community based on poorly reactive minerals. Environ. Sci. Technol. 51, 12293–12301. doi: 10.1021/acs.est.7b03126
Bastviken, D., Cole, J., Pace, M., and Tranvik, L. (2004). Methane emissions from lakes: dependence of lake characteristics, two regional assessments, and a global estimate. Glob. Biogeochem. Cycles 18, 1–12. doi: 10.1029/2004GB002238
Bastviken, D., Ejlertsson, J., and Tranvik, L. (2002). Measurement of methane oxidation in lakes: a comparison of methods. Environ. Sci. Technol. 36, 3354–3361. doi: 10.1021/es010311p
Beal, E. J., House, C. H., and Orphan, V. J. (2009). Manganese- and iron-dependent marine methane oxidation. Science 325, 184–187. doi: 10.1126/science.1169984
Bender, M., and Conrad, R. (1994). Methane oxidation activity in various soils and freshwater sediments: occurrence, characteristics, vertical profiles, and distribution on grain size fractions. J. Geophys. Res. 99:16531. doi: 10.1029/94JD00266
Blazewicz, S. J., Petersen, D. G., Waldrop, M. P., and Firestone, M. K. (2012). Anaerobic oxidation of methane in tropical and boreal soils: ecological significance in terrestrial methane cycling. J. Geophys. Res. Biogeo. 117, 1–9. doi: 10.1029/2011JG001864
Blees, J., Niemann, H., Erne, M., Zopfi, J., Schubert, C. J., and Lehmann, M. F. (2015). Spatial variations in surface water methane super-saturation and emission in Lake Lugano, southern Switzerland. Aquat. Sci. 77, 535–545. doi: 10.1007/s00027-015-0401-z
Blees, J., Niemann, H., Wenk, C. B., Zopfi, J., Schubert, C. J., Jenzer, J. S., et al. (2014a). Bacterial methanotrophs drive the formation of a seasonal anoxic benthic nepheloid layer in an alpine lake. Limnol. Oceanogr. 59, 1410–1420. doi: 10.4319/lo.2014.59.4.1410
Blees, J., Niemann, H., Wenk, C. B., Zopfi, J., Schubert, C. J., Kirf, M. K., et al. (2014b). Micro-aerobic bacterial methane oxidation in the chemocline and anoxic water column of deep south-alpine Lake Lugano (Switzerland). Limnol. Oceanogr. 59, 311–324. doi: 10.4319/lo.2014.59.2.0311
Blodau, C., and Deppe, M. (2012). Humic acid addition lowers methane release in peats of the Mer Bleue bog, Canada. Soil Biol. Biochem. 52, 96–98. doi: 10.1016/j.soilbio.2012.04.023
Boetius, A., Ravenschlag, K., Schubert, C. J., Rickert, D., Widdel, F., Gieseke, A., et al. (2000). A marine microbial consortium apparently mediating anaerobic oxidation of methane. Nature 407, 623–626. doi: 10.1038/35036572
Bowman, J. P., Sly, L. T., Nichols, P. D., and Hayward, A. C. (1994). Erratum: Revised taxonomy of the methanotrophs: description of Methylobacter gen. nov., emendation of Methylococcus, validation of Methylosinus and Methylocystis species, and a proposal that the family Methylococcaceae includes only the group I methanotro. Int. J. Syst. Bacteriol. 44:375. doi: 10.1099/00207713-44-2-375
Cai, C., Leu, A. O., Xie, G.-J., Guo, J., Feng, Y., Zhao, J.-X., et al. (2018). A methanotrophic archaeon couples anaerobic oxidation of methane to Fe(III) reduction. ISME J. 12, 1929–1939. doi: 10.1038/s41396-018-0109-x
Cline, J. D. (1969). Spectrophotometric determination of hydrogen sulfide in natural waters. Limnol. Oceanogr. 14, 454–458. doi: 10.4319/lo.1969.14.3.0454
Crowe, S. A., Katsev, S., Leslie, K., Sturm, A., Magen, C., Nomosatryo, S., et al. (2011). The methane cycle in ferruginous Lake Matano. Geobiology 9, 61–78. doi: 10.1111/j.1472-4669.2010.00257.x
Deutzmann, J. S., Stief, P., Brandes, J., and Schink, B. (2014). Anaerobic methane oxidation coupled to denitrification is the dominant methane sink in a deep lake. Proc. Natl. Acad. Sci. 111, 18273–18278. doi: 10.1073/pnas.1411617111
Donis, D., Flury, S., Stöckli, A., Spangenberg, J. E., Vachon, D., and McGinnis, D. F. (2017). Full-scale evaluation of methane production under oxic conditions in a mesotrophic lake. Nat. Commun. 8, 1661–1611. doi: 10.1038/s41467-017-01648-4
Downing, J. A., Prairie, Y. T., Cole, J. J., Duarte, C. M., Tranvik, L. J., Striegl, R. G., et al. (2006). The global abundance and size distribution of lakes, ponds, and impoundments. Limnol. Oceanogr. 51, 2388–2397. doi: 10.4319/lo.2006.51.5.2388
Dunfield, P. F., Yuryev, A., Senin, P., Smirnova, A. V., Stott, M. B., Hou, S., et al. (2007). Methane oxidation by an extremely acidophilic bacterium of the phylum Verrucomicrobia. Nature 450, 879–882. doi: 10.1038/nature06411
Edgar, R. (2016). SINTAX: a simple non-Bayesian taxonomy classifier for 16S and ITS sequences. bioRxiv [Preprint]. doi: 10.1101/074161
Elvert, M., Boetius, A., Knittel, K., and Jørgensen, B. B. (2003). Characterization of specific membrane fatty acids as chemotaxonomic markers for sulfate-reducing bacteria involved in anaerobic oxidation of methane. Geomicrobiol J. 20, 403–419. doi: 10.1080/01490450303894
Elvert, M., Suess, E., Greinert, J., and Whiticar, M. J. (2000). Archaea mediating anaerobic methane oxidation in deep-sea sediments at cold seeps of the eastern Aleutian subduction zone. Org. Geochem. 31, 1175–1187. doi: 10.1016/S0146-6380(00)00111-X
Ettwig, K. F., Butler, M. K., Le Paslier, D., Pelletier, E., Mangenot, S., Kuypers, M. M. M., et al. (2010). Nitrite-driven anaerobic methane oxidation by oxygenic bacteria. Nature 464, 543–548. doi: 10.1038/nature08883
Ettwig, K. F., Shima, S., Van De Pas-Schoonen, K. T., Kahnt, J., Medema, M. H., Op Den Camp, H. J. M., et al. (2008). Denitrifying bacteria anaerobically oxidize methane in the absence of Archaea. Environ. Microbiol. 10, 3164–3173. doi: 10.1111/j.1462-2920.2008.01724.x
Ettwig, K. F., Zhu, B., Speth, D., Keltjens, J. T., Jetten, M. S. M., and Kartal, B. (2016). Archaea catalyze iron-dependent anaerobic oxidation of methane. Proc. Natl. Acad. Sci. 113, 12792–12796. doi: 10.1073/pnas.1609534113
Frenzel, P., Thebrath, B., and Conrad, R. (1990). Oxidation of methane in the oxic surface layer of a deep lake sediment (Lake Constance). FEMS Microbiol. Lett. 73, 149–158. doi: 10.1111/j.1574-6968.1990.tb03935.x
Graf, J. S., Mayr, M. J., Marchant, H. K., Tienken, D., Hach, P. F., Brand, A., et al. (2018). Bloom of a denitrifying methanotroph, “Candidatus Methylomirabilis limnetica,” in a deep stratified lake. Environ. Microbiol. 20, 2598–2614. doi: 10.1111/1462-2920.14285
Hansen, H. P., and Korolev, F. (1999). “Determination of nutrients,” in Methods of Seawater Analysis. 3rd Edn. eds. K. Grasshoff, M. Ehrhardt, and K. Kremling (Weinheim, Germany: Wiley-VCH), 159–228.
Hanson, R. S., and Hanson, T. E. (1996). Methanotrophic bacteria. Microbiol. Rev. 60, 439–471. doi: 10.1128/mr.60.2.439-471.1996
Haroon, M. F., Hu, S., Shi, Y., Imelfort, M., Keller, J., Hugenholtz, P., et al. (2013). Anaerobic oxidation of methane coupled to nitrate reduction in a novel archaeal lineage. Nature 500, 567–570. doi: 10.1038/nature12375
He, R., Wang, J., Pohlman, J. W., Jia, Z., Chu, Y. X., Wooller, M. J., et al. (2021). Metabolic flexibility of aerobic methanotrophs under anoxic conditions in Arctic lake sediments. ISME J. 16, 78–90. doi: 10.1038/s41396-021-01049-y
He, R., Wooller, M. J., Pohlman, J. W., Quensen, J., Tiedje, J. M., and Leigh, M. B. (2012). Diversity of active aerobic methanotrophs along depth profiles of arctic and subarctic lake water column and sediments. ISME J. 6, 1937–1948. doi: 10.1038/ismej.2012.34
Henckel, T., Roslev, P., and Conrad, R. (2000). Effects of O2 and CH4 on presence and activity of the indigenous methanotrophic community in rice field soil. Environ. Microbiol. 2, 666–679. doi: 10.1046/j.1462-2920.2000.00149.x
Ishiwatari, R. (1985). “Geochemistry of humic substances in lake sediments,” in Humic Substances in Soil, Sediment, and Water: Geochemistry, Isolation, and Characterization. ed. D. M. McKnight (New York: John Wiley), 147–180.
Iversen, N., and Jørgensen, B. B. (1985). Anaerobic methane oxidation rates at the sulfate-methane transition in marine sediments from Kattegat and Skagerrak (Denmark)1. Limnol. Oceanogr. 30, 944–955. doi: 10.4319/lo.1985.30.5.0944
Kits, K. D., Campbell, D. J., Rosana, A. R., and Stein, L. Y. (2015a). Diverse electron sources support denitrification under hypoxia in the obligate methanotroph Methylomicrobium album strain BG8. Front. Microbiol. 6:1072. doi: 10.3389/fmicb.2015.01072
Kits, K. D., Klotz, M. G., and Stein, L. Y. (2015b). Methane oxidation coupled to nitrate reduction under hypoxia by the Gammaproteobacterium Methylomonas denitrificans, sp. nov. type strain FJG1. Environ. Microbiol. 17, 3219–3232. doi: 10.1111/1462-2920.12772
Knittel, K., and Boetius, A. (2009). Anaerobic oxidation of methane: progress with an unknown process. Annu. Rev. Microbiol. 63, 311–334. doi: 10.1146/annurev.micro.61.080706.093130
Kojima, H., Tsutsumi, M., Ishikawa, K., Iwata, T., and Mußmann, M. (2012). Distribution of putative denitrifying methane oxidizing bacteria in sediment of a freshwater lake, Lake Biwa. Syst. Appl. Microbiol. 35, 233–238. doi: 10.1016/j.syapm.2012.03.005
Kool, D. M., Zhu, B., Rijpstra, W. I. C., Jetten, M. S. M., Ettwig, K. F., and Sinninghe Damsté, J. S. (2012). Rare branched fatty acids characterize the lipid composition of the intra-aerobic methane oxidizer “Candidatus Methylomirabilis oxyfera”. Appl. Environ. Microbiol. 78, 8650–8656. doi: 10.1128/AEM.02099-12
Kuivila, K. M., Murray, J. W., Devol, A. H., Lidstrom, M. E., and Reimers, C. E. (1988). Methane cycling in the sediments of Lake Washington. Limnol. Oceanogr. 33, 571–581. doi: 10.4319/lo.1988.33.4.0571
Leu, A. O., Cai, C., McIlroy, S. J., Southam, G., Orphan, V. J., Yuan, Z., et al. (2020). Anaerobic methane oxidation coupled to manganese reduction by members of the Methanoperedenaceae. ISME J. 14, 1030–1041. doi: 10.1038/s41396-020-0590-x
Lidstrom, M. E., and Somers, L. (1984). Seasonal study of methane oxidation in Lake Washington. Appl. Environ. Microbiol. 47, 1255–1260. doi: 10.1128/aem.47.6.1255-1260.1984
Lovley, D. R., Coates, J. D., Blunt-Harris, E. L., Phillips, E. J. P., and Woodward, J. C. (1996). Humic substances as electron acceptors for microbial respiration. Nature 382, 445–448. doi: 10.1038/382445a0
Martinez-Cruz, K., Leewis, M., Charold, I., Sepulveda-Jauregui, A., Walter, K., Thalasso, F., et al. (2017). Anaerobic oxidation of methane by aerobic methanotrophs in sub-Arctic lake sediments. Sci. Total Environ. 607-608, 23–31. doi: 10.1016/j.scitotenv.2017.06.187
Martinez-Cruz, K., Sepulveda-Jauregui, A., Casper, P., Anthony, K. W., Smemo, K. A., and Thalasso, F. (2018). Ubiquitous and significant anaerobic oxidation of methane in freshwater lake sediments. Water Res. 144, 332–340. doi: 10.1016/j.watres.2018.07.053
Mayr, M. J., Zimmermann, M., Guggenheim, C., Brand, A., and Bürgmann, H. (2020). Niche partitioning of methane-oxidizing bacteria along the oxygen–methane counter gradient of stratified lakes. ISME J. 14, 274–287. doi: 10.1038/s41396-019-0515-8
McMurdie, P. J., and Holmes, S. (2013). Phyloseq: an R package for reproducible interactive analysis and graphics of microbiome census data. PLoS One 8:e61217. doi: 10.1371/journal.pone.0061217
Milucka, J., Ferdelman, T. G., Polerecky, L., Franzke, D., Wegener, G., Schmid, M., et al. (2012). Zero-valent sulphur is a key intermediate in marine methane oxidation. Nature 491, 541–546. doi: 10.1038/nature11656
Milucka, J., Kirf, M., Lu, L., Krupke, A., Lam, P., Littmann, S., et al. (2015). Methane oxidation coupled to oxygenic photosynthesis in anoxic waters. ISME J. 9, 1991–2002. doi: 10.1038/ismej.2015.12
Norði, K. À., Thamdrup, B., and Schubert, C. J. (2013). Anaerobic oxidation of methane in an iron-rich Danish freshwater lake sediment. Limnol. Oceanogr. 58, 546–554. doi: 10.4319/lo.2013.58.2.0546
Orphan, V. J., House, C. H., Hinrichs, K.-U., McKeegan, K. D., and DeLong, E. F. (2002). Multiple archaeal groups mediate methane oxidation in anoxic cold seep sediments. Proc. Natl. Acad. Sci. 99, 7663–7668. doi: 10.1073/pnas.072210299
Oswald, K., Graf, J. S., Littmann, S., Tienken, D., Brand, A., Wehrli, B., et al. (2017). Crenothrix are major methane consumers in stratified lakes. ISME J. 11, 2124–2140. doi: 10.1038/ismej.2017.77
Oswald, K., Milucka, J., Brand, A., Hach, P., Littmann, S., Wehrli, B., et al. (2016). Aerobic gammaproteobacterial methanotrophs mitigate methane emissions from oxic and anoxic lake waters. Limnol. Oceanogr. 61, S101–S118. doi: 10.1002/lno.10312
Oswald, K., Milucka, J., Brand, A., Littmann, S., Wehrli, B., Kuypers, M. M. M., et al. (2015). Light-dependent aerobic methane oxidation reduces methane emissions from seasonally stratified lakes. PLoS One 10:e0132574. doi: 10.1371/journal.pone.0132574
Parada, A. E., Needham, D. M., and Fuhrman, J. A. (2016). Every base matters: assessing small subunit rRNA primers for marine microbiomes with mock communities, time series and global field samples. Environ. Microbiol. 18, 1403–1414. doi: 10.1111/1462-2920.13023
Pasche, N., Schmid, M., Vazquez, F., Schubert, C. J., Wüest, A., Kessler, J. D., et al. (2011). Methane sources and sinks in Lake Kivu. J. Geophys. Res. Biogeo. 116, 1–16. doi: 10.1029/2011JG001690
Quast, C., Pruesse, E., Yilmaz, P., Gerken, J., Schweer, T., Yarza, P., et al. (2013). The SILVA ribosomal RNA gene database project: improved data processing and web-based tools. Nucleic Acids Res. 41, D590–D596. doi: 10.1093/nar/gks1219
Raghoebarsing, A. A., Pol, A., van de Pas-Schoonen, K. T., Smolders, A. J. P., Ettwig, K. F., Rijpstra, W. I. C., et al. (2006). A microbial consortium couples anaerobic methane oxidation to denitrification. Nature 440, 918–921. doi: 10.1038/nature04617
R Core Team (2014). R: A language and environment for statis- tical computing. R Foundation for Statistical Computing. Available at: www.r-project.org/
Rissanen, A. J., Saarenheimo, J., Tiirola, M., Peura, S., Aalto, S. L., Karvinen, A., et al. (2018). Gammaproteobacterial methanotrophs dominate methanotrophy in aerobic and anaerobic layers of boreal lake waters. Aquat. Microb. Ecol. 81, 257–276. doi: 10.3354/ame01874
Roslev, P., and King, G. M. (1995). Aerobic and anaerobic starvation metabolism in methanotrophic bacteria. Appl. Environ. Microbiol. 61, 1563–1570. doi: 10.1128/aem.61.4.1563-1570.1995
Scheller, S., Yu, H., Chadwick, G. L., McGlynn, S. E., and Orphan, V. J. (2016). Artificial electron acceptors decouple archaeal methane oxidation from sulfate reduction. Science 351, 703–707. doi: 10.1126/science.aad7154
Schmieder, R., and Edwards, R. (2011). Quality control and preprocessing of metagenomic datasets. Bioinformatics 27, 863–864. doi: 10.1093/bioinformatics/btr026
Schubert, C. J., Vazquez, F., Lösekann-Behrens, T., Knittel, K., Tonolla, M., and Boetius, A. (2011). Evidence for anaerobic oxidation of methane in sediments of a freshwater system (Lago di Cadagno). FEMS Microbiol. Ecol. 76, 26–38. doi: 10.1111/j.1574-6941.2010.01036.x
Scott, D. T., McKnight, D. M., Blunt-Harris, E. L., and Kolesar, S. E. (1999). Quinone moieties act as electron acceptors in the reduction of humic substances by humics-reducing microorganisms. Environ. Sci. Technol. 33:372. doi: 10.1021/es982014z
Segarra, K. E. A., Comerford, C., Slaughter, J., and Joye, S. B. (2013). Impact of electron acceptor availability on the anaerobic oxidation of methane in coastal freshwater and brackish wetland sediments. Geochim. Cosmochim. Acta 115, 15–30. doi: 10.1016/j.gca.2013.03.029
Sicre, M.-A., Tian, R. C., and Saliot, A. (1994). Distribution of sterols in the suspended particles of the Chang Jiang Estuary and adjacent East China Sea. Org. Geochem. 21, 1–10. doi: 10.1016/0146-6380(94)90083-3
Sivan, O., Adler, M., Pearson, A., Gelman, F., Bar-Or, I., John, S. G., et al. (2011). Geochemical evidence for iron-mediated anaerobic oxidation of methane. Limnol. Oceanogr. 56, 1536–1544. doi: 10.4319/lo.2011.56.4.1536
Skerratt, J. H., Nichols, P. D., Bowman, J. P., and Sly, L. I. (1992). Occurrence and significance of long-chain (ω-1)-hydroxy fatty acids in methane-utilizing bacteria. Org. Geochem. 18, 189–194. doi: 10.1016/0146-6380(92)90129-L
Smemo, K. A., and Yavitt, J. B. (2011). Anaerobic oxidation of methane: An underappreciated aspect of methane cycling in peatland ecosystems? Biogeosciences 8, 779–793. doi: 10.5194/bg-8-779-2011
Stoecker, K., Bendinger, B., Schöning, B., Nielsen, P. H., Nielsen, J. L., Baranyi, C., et al. (2006). Cohn’s Crenothrix is a filamentous methane oxidizer with an unusual methane monooxygenase. Proc. Natl. Acad. Sci. U. S. A. 103, 2363–2367. doi: 10.1073/pnas.0506361103
Stookey, L. L. (1970). Ferrozine-A new spectrophotometric reagent for iron. Anal. Chem. 42, 779–781. doi: 10.1021/ac60289a016
Su, G., Lehmann, M. F., Tischer, J., Weber, Y., Walser, J. C., Niemann, H., et al. (2021). Water column stability as an important factor controlling nitrite-dependent anaerobic methane oxidation in stratified lake basins. bioRxiv [Preprint]. doi: 10.1101/2021.11.09.467825
Su, G., Niemann, H., Steinle, L., Zopfi, J., and Lehmann, M. F. (2019). Evaluating radioisotope-based approaches to measure anaerobic methane oxidation rates in lacustrine sediments. Limnol. Oceanogr. Methods 17, 429–438. doi: 10.1002/lom3.10323
Su, G., Zopfi, J., Yao, H., Steinle, L., Niemann, H., and Lehmann, M. F. (2020). Manganese/iron-supported sulfate-dependent anaerobic oxidation of methane by archaea in lake sediments. Limnol. Oceanogr. 65, 863–875. doi: 10.1002/lno.11354
Vaksmaa, A., Guerrero-Cruz, S., van Alen, T. A., Cremers, G., Ettwig, K. F., Lüke, C., et al. (2017). Enrichment of anaerobic nitrate-dependent methanotrophic ‘Candidatus Methanoperedens nitroreducens’ archaea from an Italian paddy field soil. Appl. Microbiol. Biotechnol. 101, 7075–7084. doi: 10.1007/s00253-017-8416-0
Valenzuela, E. I., Avendaño, K. A., Balagurusamy, N., Arriaga, S., Nieto-Delgado, C., Thalasso, F., et al. (2019). Electron shuttling mediated by humic substances fuels anaerobic methane oxidation and carbon burial in wetland sediments. Sci. Total Environ. 650, 2674–2684. doi: 10.1016/J.SCITOTENV.2018.09.388
van Grinsven, S., Sinninghe Damsté, J. S., Harrison, J., Polerecky, L., and Villanueva, L. (2021). Nitrate promotes the transfer of methane-derived carbon from the methanotroph Methylobacter sp. to the methylotroph Methylotenera sp. in eutrophic lake water. Limnol. Oceanogr. 66, 878–891. doi: 10.1002/lno.11648
Weber, H. S., Habicht, K. S., and Thamdrup, B. (2017). Anaerobic methanotrophic archaea of the ANME-2d cluster are active in a low-sulfate, iron-rich freshwater sediment. Front. Microbiol. 8:619. doi: 10.3389/fmicb.2017.00619
Wegener, G., Krukenberg, V., Riedel, D., Tegetmeyer, H. E., and Boetius, A. (2015). Intercellular wiring enables electron transfer between methanotrophic archaea and bacteria. Nature 526, 587–590. doi: 10.1038/nature15733
Wegener, G., Niemann, H., Elvert, M., Hinrichs, K. U., and Boetius, A. (2008). Assimilation of methane and inorganic carbon by microbial communities mediating the anaerobic oxidation of methane. Environ. Microbiol. 10, 2287–2298. doi: 10.1111/j.1462-2920.2008.01653.x
Wilson, L. G., and Bandurski, R. S. (1958). Enzymatic reactions involving sulfate, sulfite, selenate and molybdate. J. Biol. Chem. 233, 975–981. doi: 10.1016/S0021-9258(18)64689-7
Keywords: aerobic methanotrophs, anaerobic oxidation of methane, lake sediment, methane oxidation rates, electron acceptors, lipid biomarkers, microbial community
Citation: Su G, Zopfi J, Niemann H and Lehmann MF (2022) Multiple Groups of Methanotrophic Bacteria Mediate Methane Oxidation in Anoxic Lake Sediments. Front. Microbiol. 13:864630. doi: 10.3389/fmicb.2022.864630
Edited by:
Peng Xing, Nanjing Institute of Geography and Limnology (CAS), ChinaReviewed by:
Mary Beth Leigh, University of Alaska Fairbanks, United StatesRuo He, Zhejiang Gongshang University, China
Copyright © 2022 Su, Zopfi, Niemann and Lehmann. This is an open-access article distributed under the terms of the Creative Commons Attribution License (CC BY). The use, distribution or reproduction in other forums is permitted, provided the original author(s) and the copyright owner(s) are credited and that the original publication in this journal is cited, in accordance with accepted academic practice. No use, distribution or reproduction is permitted which does not comply with these terms.
*Correspondence: Guangyi Su, guangyi.su@unibas.ch