- Guangxi Colleges and Universities Key Laboratory of Crop Cultivation and Tillage, Guangxi University, Nanning, China
The application of biosynthesized nano-selenium fertilizers to crops can improve their nutrient levels by increasing their selenium content. However, microorganisms with a high selenite tolerance and rapid reduction rate accompanied with the production of selenium nanoparticles (SeNPs) at the same time have seldom been reported. In this study, a bacterial strain showing high selenite resistance (up to 300 mM) was isolated from a lateritic red soil and identified as Proteus mirabilis QZB-2. This strain reduced nearly 100% of 1.0 and 2.0 mM selenite within 12 and 18 h, respectively, to produce SeNPs. QZB-2 isolate reduced SeO32– to Se0 in the cell membrane with NADPH or NADH as electron donors. Se0 was then released outside of the cell, where it formed spherical SeNPs with an average hydrodynamic diameter of 152.0 ± 10.2 nm. P. mirabilis QZB-2 could be used for SeNPs synthesis owing to its simultaneously high SeO32– tolerance and rapid reduction rate.
Highlights
- Microorganisms with a high selenite tolerance, selenite reduction capacity, and also the ability to produce selenium nanoparticles (SeNPs) have rarely been reported.
- Here, we report a Proteus mirabilis QZB-2 strain with a high selenite resistance (up to 300 mM).
- This strain exhibited excellent SeO32– reduction efficiency, nearly exhausted all of the SeO32– (1 or 2 mM) within 18 h and transformed SeO32– into SeNPs more rapidly than any other bacteria reported to date.
- The SeO32– reduction activity in QZB-2 cells was attributed to the cell membrane fraction, with or without NADH/NADPH serving as electron donors.
- Our results reveal that P. mirabilis QZB-2 is an attractive bacterial candidate for the synthesis of novel nano-Se fertilizers owing to its simultaneously high SeO32– tolerance and rapid reduction rate.
Introduction
Selenium (Se) is an essential trace nutrient for humans and plays an important role in maintaining body health and preventing diseases (Dinh et al., 2018; Yan et al., 2021). However, globally, 0.5–1 billion people suffer from Se deficiency (Li et al., 2021). The biofortification of crops with Se-rich fertilizers is considered to be the most effective way to increase human Se intake (Luo et al., 2019; Zhang et al., 2019a; Li et al., 2021). While fertilizers can contain organic selenium and nano-selenium, selenite (SeO32–) and selenate (SeO42–) are the two major types of inorganic Se used in fertilizers because of their low cost. However, the utilization efficiency of selenite and selenate by plants is relatively low because of their high toxicity (Deng et al., 2017). Soluble nano-Se is less toxic than inorganic and organic Se and has a higher bioavailability (Zahedi et al., 2019; Kumar and Prasad, 2021). The application of nano-Se to foliage or soil can significantly enhance crop yield, quality, and Se content (Li et al., 2020, 2021). Thus, improving the processes for synthesizing of nano-Se fertilizers could enhance the development of Se-rich agricultural products.
Traditional physical and chemical methods to synthesize selenium nanoparticles (SeNPs) are costly and cause pollution. Therefore, biological approaches are generally preferred because they have a low-cost and are eco-friendly (Wang et al., 2018b; Kumar and Prasad, 2021). Studies have revealed that some bacterial strains, such as Pseudomonas spp. (Kuroda et al., 2011; Avendaño et al., 2016), Bacillus spp. (Blum et al., 1998; Oremland et al., 2004; Bao et al., 2016), Clostridium sp. (Bao et al., 2013), Selenihalanaerobacter sp. (Oremland et al., 2004), and Sulfurospirillum sp. (Oremland et al., 2004), can synthesize nano-Se. Most of them use SeO32– as a raw material, but only a few can use both SeO32– and SeO42–. Moreover, most Se-reducing microorganisms have a limited tolerance to SeO32– (≤100 mM) and require 48 h or more to reduce all SeO32– (≥1 mM) to elemental selenium (Se0) (Table 1). Therefore, novel strains with high SeO32– tolerance and robust SeO32– reduction abilities are required to improve SeNP synthesis.
In this study, the aerobic bacterium P. mirabilis QZB-2, isolated from lateritic red soil in Guangxi, China, showed strong tolerance to high concentrations of SeO32– (up to 300 mM). The QZB-2 strain reduced the majority of 1.0 and 2.0 mM SeO32– to Se0 within 12 and 18 h, respectively. This reduction occurred in the cell membrane, after which Se0 was released outside the cell where it formed spherical SeNPs with an average hydrodynamic diameter of 152.0 ± 10.2 nm. Our results reveal that P. mirabilis QZB-2 is a strong bacterial candidate for the synthesis of novel nano-Se fertilizers owing to its simultaneously high SeO32– tolerance and rapid reduction rates.
Materials and Methods
Culture Medium
Luria–Bertani (LB) medium (per liter, pH 7.0–7.2) was used for bacterial enrichment. It contained 5.00 g of yeast extract, 10.00 g of NaCl, and 10.00 g of tryptone per liter. A Na2SeO3 solution was prepared in deionized water and sterilized by filtration.
Isolation and Identification of Selenite-Reducing Bacteria
Soil samples were collected from a naturally occurring Se-rich lateritic red soil (0–15 cm depth) on dry land in Guangxi province, southern China (22°05′31′′ N, 108°32′53′′ E). The total Se in the soil was 0.53 mg/kg. To isolate Se-reducing bacteria, 1 g of the soil sample was suspended in 100 mL of sterilized LB broth supplemented with 1 mM SeO32–, and cultured at 30°C (150 rpm) for 48 h. The bacterial strains were subcultured three times with an inoculum size of 5%. The culture solution was then diluted three times (from 10–5 to 10–7), and 100 μL of each dilution was inoculated on LB agar plates containing 10.00 mM SeO32–. The agar plates were incubated at 30°C for 24 h. Red colonies were continuously picked and subcultured onto new plate until the pure cultures were finally obtained. Of all the monocultures, the QZB-2 isolate was chosen for further experiments because of its high SeO32 tolerance.
To identify the QZB-2 isolate, its cell morphology was determined using an Olympus BH-2 optical microscope. The antibiotic resistance of the QZB-2 strain was tested using 1 and 100 μg/mL tetracycline, ampicillin, chloramphenicol, kanamycin, and gentamycin. Subsequently, the 16S rRNA gene was amplified using universal primers 27F (5′-AGAGTTTGATCMTGGCTCAG-3′) and 1492R (5′-ACGGTTACCT TGTTACGACTT-3′) (Zhang et al., 2019b). The obtained sequence was compared with other previously published sequences of the bacterial 16S rRNA gene in the NCBI. Finally, a phylogenetic tree was constructed using MEGA 7.0 software (Huang et al., 2018b).
Tolerance and Reduction of Selenite by the QZB-2 Strain
Selenite Tolerance
The tolerance of the QZB-2 isolate to SeO32– was assessed by determining the minimal inhibitory concentration (MIC) of SeO32– (Huang et al., 2021). First, the QZB-2 strain was activated in an LB medium and then harvested at 3,500 × g for 5 min. The harvested cells were washed with a phosphate buffer (PBS) and inoculated into fresh LB medium supplemented with different concentrations of SeO32– (0–600 mM). The culture was incubated at 30°C and 150 rpm for 24 h. Subsequently, 100 μL of the culture cells was inoculated onto LB agar plates and incubated for an additional 72 h at 30°C to determine the SeO32– concentration that inhibited the growth of the QZB-2 strain.
Selenite Reduction
The activated QZB-2 strain was inoculated into an LB medium containing 1 or 2 mM SeO32–. Two types of negative controls, without SeO32– or without bacteria, were used. The cultures were incubated at 30°C on a shaker (150 rpm) for 36 h. The SeO32– and Se0 contents, as well as the amount of bacterial growth, were determined every 6 h.
Analytical Methods
Bacterial growth was determined based on the number of colony-forming units (CFUs), which was determined by spreading 100 μL of the diluted culture on LB plates and incubating them at 30°C for 72 h. SeO32– concentrations were determined using an atomic fluorescence morphology analyzer (SA-20; Jitian, Beijing), while Se0 content was measured using a spectrophotometric method, as described by Khoei et al. (2017).
Subcellular Localization of Selenite Reduction
Different fractions of QZB-2 were collected to determine the cell compartment where SeO32– is reduced. First, QZB-2 isolate was incubated in an LB medium for 18 h (stationary phase) and centrifuged at 10,000 × g for 10 min at 4°C. The obtained bacterial cell pellets were washed twice with 0.9% NaCl and then treated with different reagents to extract the periplasmic, membrane, and cytoplasmic fractions, as reported by Khoei et al. (2017). Pellets were treated with lysozyme and EDTA for 10 min, then centrifuged at 20,000 × g for 30 min, after which the supernatant was collected as a periplasmic fraction. Subsequently, the pellets were resuspended in 50 mM NaCl and then disrupted by ultra-sonication for 15 min. The supernatant was harvested as a cytoplasmic fraction following the centrifugation of the suspension at 20,000 × g for 70 min. The membrane fraction was obtained after the pellet were resuspended in 50 mM phosphate-buffered saline (PBS) (pH 7.4).
To extract extracellular polymeric substance (EPS), QZB-2 isolate was incubated in an LB medium at 30°C for 5 day. Following this, the cultures were centrifuged at 10,000 × g for 30 min at 4°C to obtain the supernatant. After passing the supernatant through a 0.45 μm filter, the supernatant was mixed with pre-cooled ethanol (1:1) and precipitated at −20°C overnight. Finally, precipitated EPS was collected by centrifugation (10,000 × g, 30 min, 4°C) (Wang et al., 2018b). For supernatant preparation, the stationary phase cultures (18 h) were centrifuged at 10,000 × g for 10 min and 4°C, before the supernatant was passed through a 0.22 μm filter and collected (Khoei et al., 2017).
Activity assays were conducted on a 96-well plate, where 100 μL of intracellular and extracellular fractions were briefly added to each well that contained 88 μL of PBS, 10 μL of SeO32– solution (2.0 mM), and 2 μL of NADH or NADPH (electron donor, 2.0 mM). The plate was then cultivated at 30°C for 72 h. Plates without an electron donor, a cell fraction (the supernatant/cell protein/EPS), or SeO32– were used as negative controls.
Localization and Characterization of Selenium
QZB-2 isolate was cultured in an LB medium, either supplemented with 2 mM SeO32– or without SeO32–. After 24 h, the culture was centrifuged (5,000 × g for 10 min) to collect bacterial cell pellets. For transmission electron microscopy (TEM) analysis, the pellet was fixed with 2.5% glutaraldehyde at 4°C for 24 h and dried in an ultra-low temperature freezer (ALPHAL-4LD PLUS, CHRIST Co., Germany) (Huang et al., 2021). For scanning electron microscopy-energy dispersive X-ray spectrometry (SEM-EDS), the pellet was fixed with 2.5% glutaraldehyde at 4°C for 24 h and then dehydrated in ethanol solutions (30, 50, 70, 90, and 100%) before drying.
Analysis of Selenium Nanoparticles
First, the QZB-2 isolate was incubated in an LB medium containing 2 mM SeO32– for 24 h and centrifuged at 10,000 × g for 10 min at 4°C. The resulting bacterial cell pellets were washed twice with 0.9% NaCl, resuspended in Tris-Cl buffer, and then disrupted by ultra-sonication. The SeNPs were harvested after the cell suspension was centrifuged at 40,000 × g for 40 min at 4°C. Dynamic Light Scattering (DLS) and zeta potential analysis of SeNPs was conducted using a Nano-ZS90X Zeta potential particle size tester (Malvern, Britain) (Lampis et al., 2017). Afterward, the purified SeNPs were dried in an ultra-low temperature freezer (ALPHAL-4LD PLUS, CHRIST Co., Germany) for SEM-EDS, XRD, and FTIR analysis. Furthermore, the morphology and constituent elements analysis of SeNPs were analyzed using SEM-EDS. The compounds of SeNPs were analyzed using a D/Max-3C X-ray diffractometer with Cu-K radiation in the range of 10–80° (2θ) at a scan rate of 2°/min. The possible chemical bonds in SeNPs were investigated using a Fourier transformed infrared (FTIR) spectrophotometer in the range of 4,000–400 cm–1 (Huang et al., 2018a).
Statistical Analyses
The obtained data were analyzed by one-way analysis of variance (ANOVA) using SPSS Statistics 22 software. The level of statistical significance was set at p < 0.05. Graphics were plotted using Origin 8.6.
Results and Discussion
Characterization and Identification of the QZB-2 Strain
In this study, 20 bacterial strains were isolated from naturally occurring Se-rich lateritic red soil in Guangxi, China, using LB plates supplemented with 20 mM SeO32–. Of these strains, the QZB-2 isolate was chosen for further experiments as it exhibited good growth and high SeO32– reduction ability (Figure 1). Phylogenetic analysis indicated that the QZB-2 isolate was closely related to P. mirabilis AGMDRUC5 (MN795608) (Figure 2) and was therefore identified as P. mirabilis QZB-2 (OL629178). Proteus sp. is a rod-shaped gram-negative bacterium that is commonly found in the environment (Drzewiecka, 2016), with some strains exhibiting heavy metal (Cr6+, Cu2+, Zn2+) resistance (Rani et al., 2008; Ge et al., 2013; Islam et al., 2014). Recently, Wang et al. (2018a) reported that P. mirabilis YC801 isolated from insect guts could tolerate 100 mM SeO32–. In this study, MIC assays showed that P. mirabilis QZB-2 could grow and produce Se0 even at a SeO32– concentration of 300 mM (Figure 1). Moreover, P. mirabilis QZB-2 isolated from soil was resistant to several common antibiotics at concentrations from 1 to even 100 μg/mL (Liu et al., 2021; Figure 3). Thus, we further explored the characteristics of SeO32– reduction and SeNPs production in the QZB-2 strain.
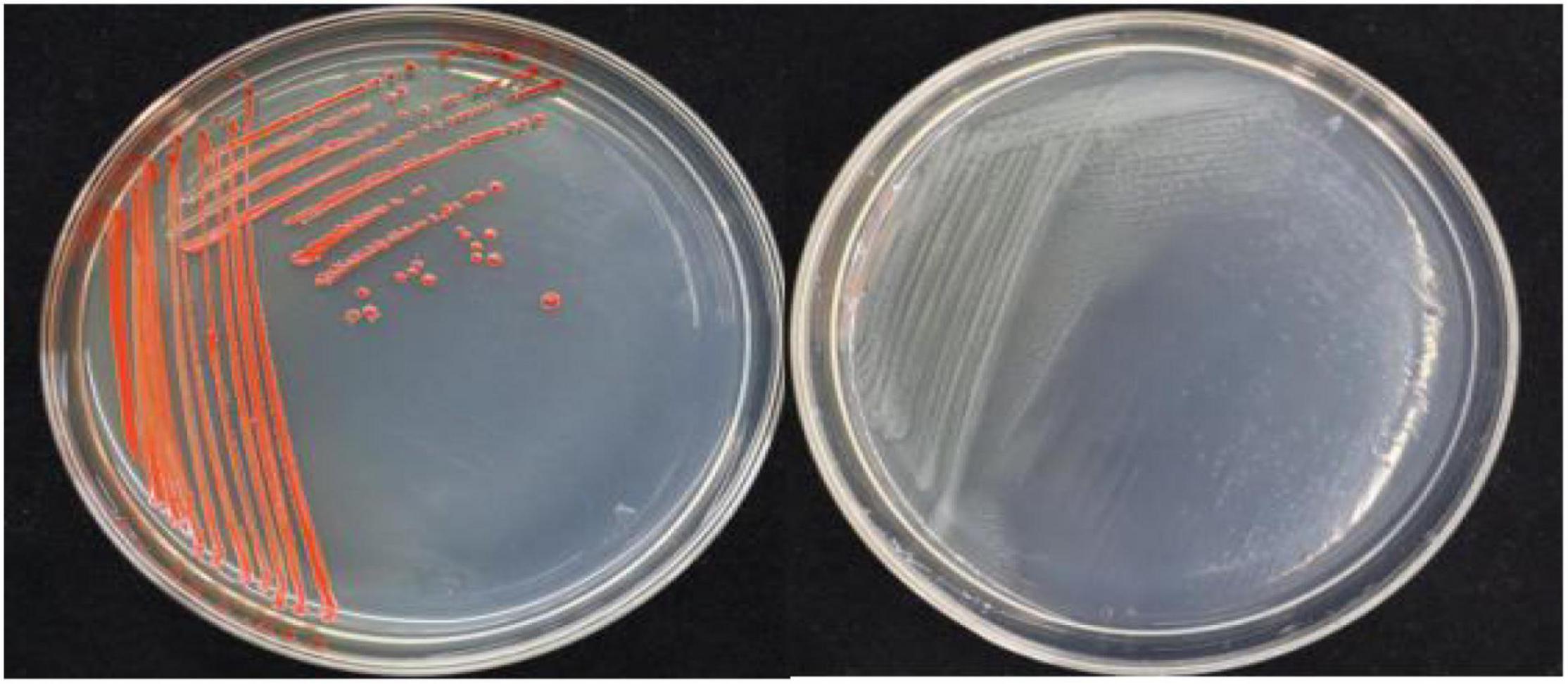
Figure 1. Growth of strain QZB-2 on LB agar plates (left: supplied with 2 mM SeO32–; left: no SeO32–). The red colony color indicates selenite reduction and the formation of elemental selenium.
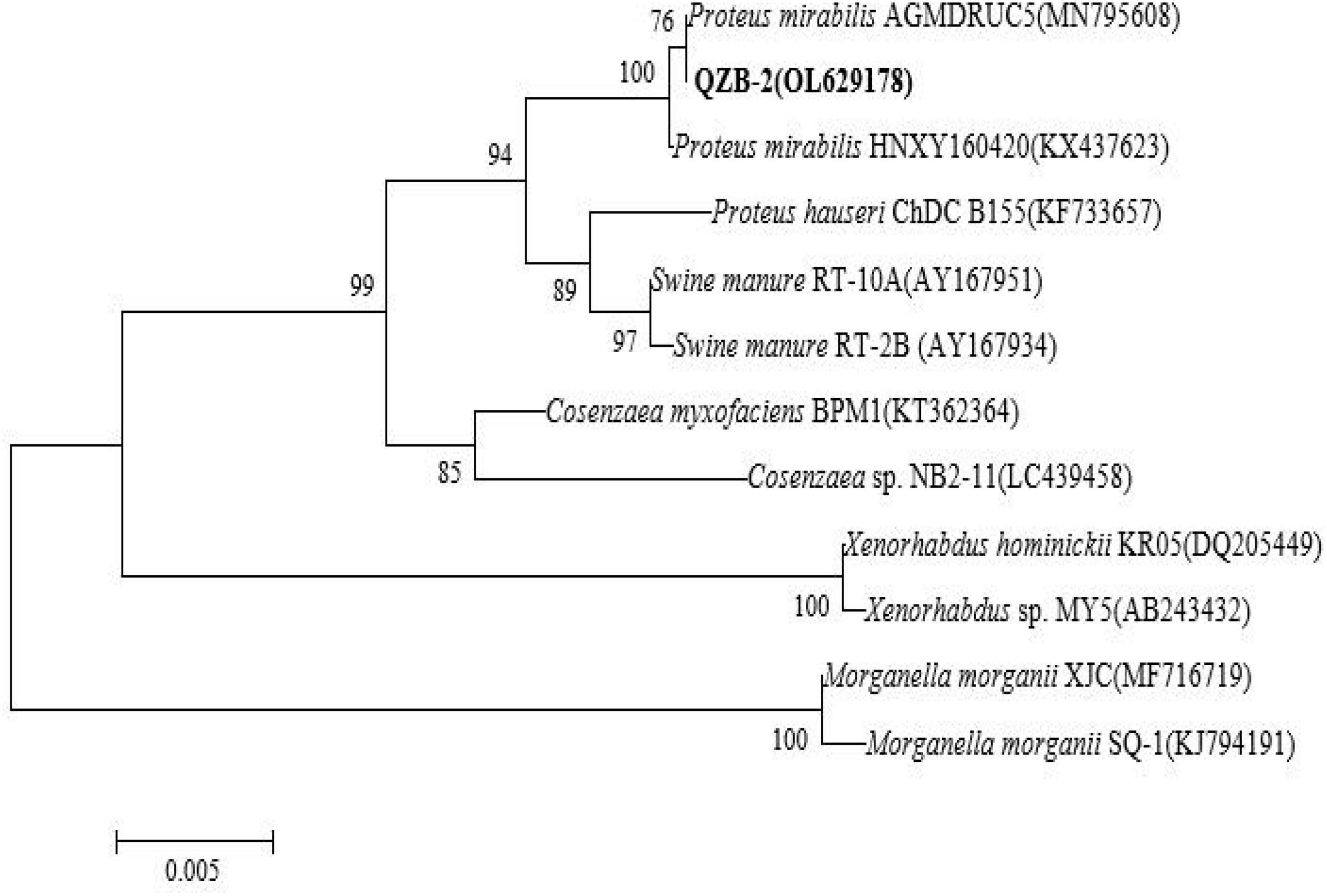
Figure 2. Maximum likelihood tree based on the 16S rRNA gene sequence of isolate QZB-2. The scale bars indicate 0.005 substitutions per site.
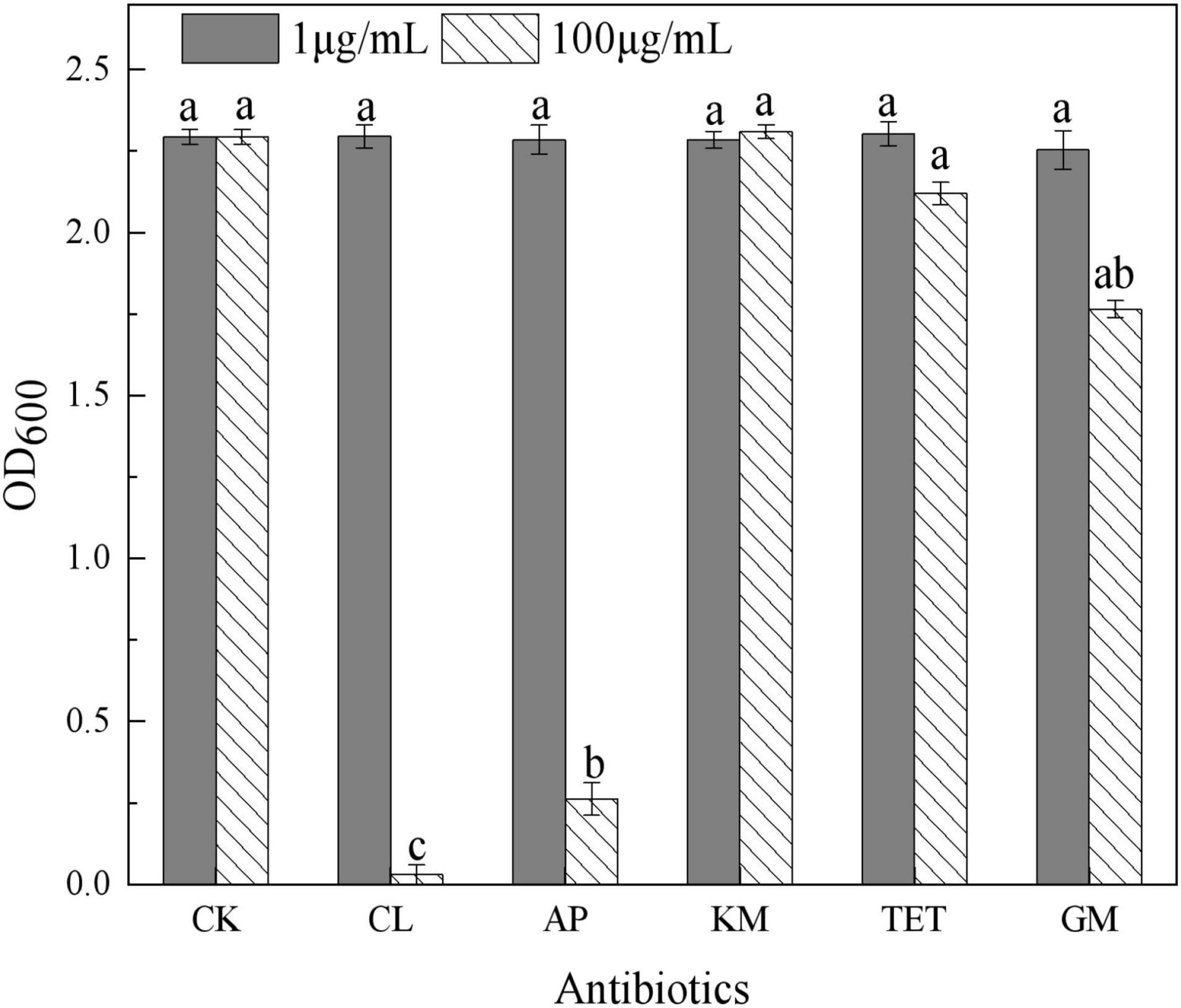
Figure 3. Influence of antibiotics on Proteus mirabilis QZB-2 growth in 24 h (CK: no antibiotics; CL: chloramphenicol; AP: ampicillin; KM: kanamycin; TET: 2.212e; GM: gentamycin). Different letters indicate sinificant differences between treatments at P < 0.05.
Selenite Reduction Characteristics by the QZB-2 Strain
The ability of QZB-2 to reduce SeO32– was studied in liquid LB medium containing 1.0 or 2.0 mM of SeO32–. Our results show that the relative growth curves of QZB-2 in a SeO32– containing culture (both 1 and 2 mM) followed the same pattern as that in cultures without SeO32– (Figure 4). These patterns confirmed that 1.0 and 2.0 mM of SeO32– did not inhibit QZB-2 growth. SeO32– depletion occurred at the start of the growth phase in the culture with 1.0 mM SeO32– (Figure 4A). These findings are similar to those reported by Wang et al. (2018a), who found that SeO32– was reduced by P. mirabilis YC801 after 6 h of incubation. However, P. mirabilis YC801 only reduced approximately 15% of the total SeO32–, while the QZB-2 strain reduced the majority of the SeO32– (88.0%) in 6 h (Figure 4). In most Se-reducing microorganisms, SeO32– reduction occurs at the beginning or during the mid-exponential phase (≥12 h) (Avendaño et al., 2016; Lampis et al., 2017; Ruiz Fresneda et al., 2018). However, these microorganisms require more time (usually over 24 h) to reduce the majority of the SeO32– (1 or 2 mM). In this study, the QZB-2 strain reduced both 1 and 2 mM of SeO32– at the beginning of its growth and nearly exhausted all of the SeO32– within 18 h. These results indicate that P. mirabilis QZB-2 has an excellent SeO32– reduction efficiency.
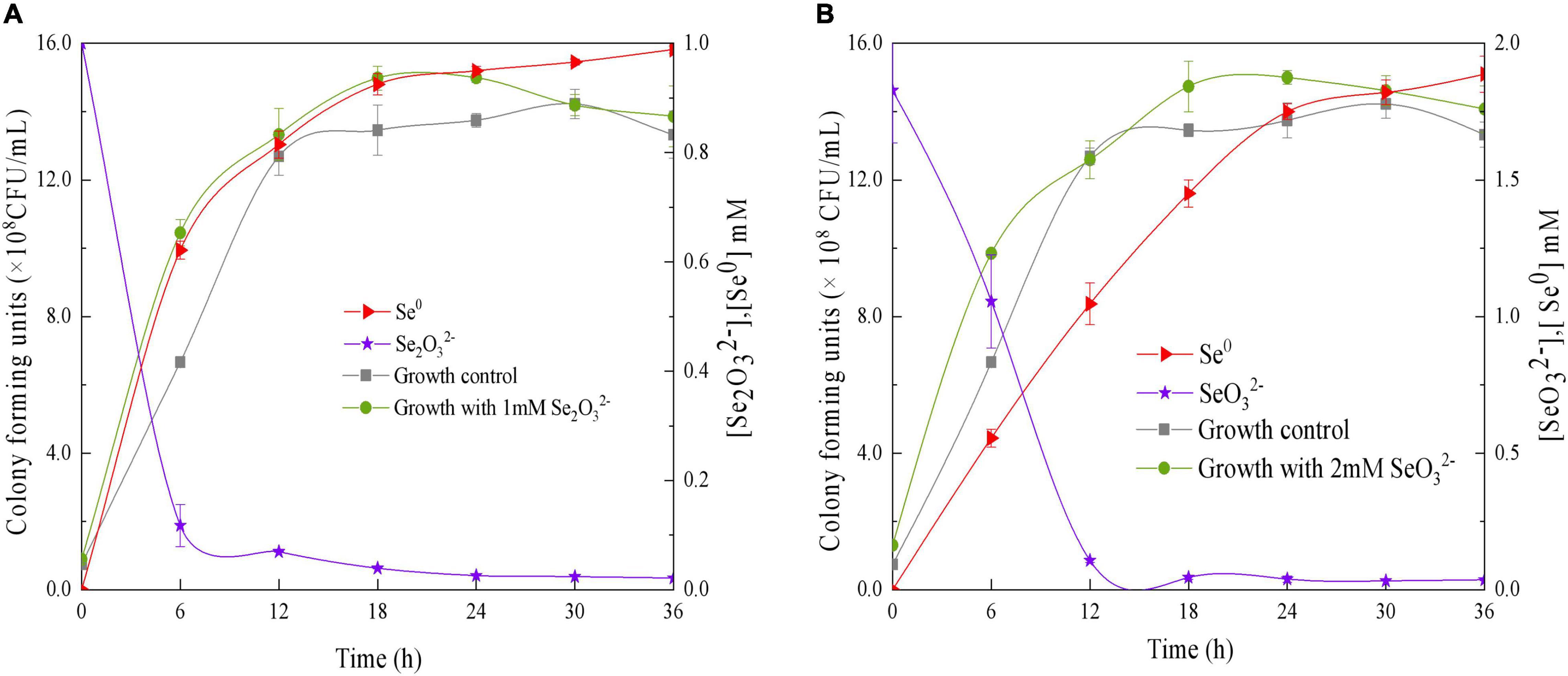
Figure 4. Time courses of bacterial growth, SeO3 2– removal, and Se0 formation by the strain Proteus mirabilis QZB-2 grown in LB medium containing 1 mM (A) and 2 mM (B) SeO32–.
In the QZB-2 strain, SeO32– reduction was coupled with Se0 accumulation; after 6 h, 88% of the 1 mM SeO32– was depleted while 70.45% of which was reduced to Se0 (Figure 4A). Conversely, a delay in Se0 formation has been observed in some bacteria, including B. mycoides SeITE01 (Lampis et al., 2014), S. maltophilia SeITE02 (Lampis et al., 2017), A. faecalis Se03 (Wang et al., 2018b), and P. mirabilis YC801 (Tugarova et al., 2018). We observed that in the QZB-2 strain more than 90% of the reduced SeO32– (1 and 2 mM) was transformed into Se0 after 36 h of incubation (Figure 4). These results demonstrate that P. mirabilis QZB-2 can tolerate high concentrations of SeO32– and transforms SeO32– into SeNPs more rapidly than any other bacteria reported to date.
Subcellular Localization of Selenite Reduction
SeO32– reduction activity in the QZB-2 strain was localized in the cell membrane fraction (Figure 5), whereas in A. faecalis Se03, P. mirabilis YC801, and P. rettgeri HF16 cells, reduction activity is localized in the cytoplasmic fraction (Wang et al., 2018a,b; Huang et al., 2021). This shows that the SeO32– reduction mechanism in QZB-2 is inconsistent with that observed in earlier studies. SeO32– reduction in the QZB-2 strain only occurred when NADH or NADPH was present (Figure 5), which is consistent with reported SeO32– reduction in S. maltophilia SeITE02 (Lampis et al., 2017) and B. fungorum strains (Khoei et al., 2017). Thus, we propose that SeO32– reduction by P. mirabilis QZB-2 occurred in the cell membrane system through the catalytic activity of a reductase, with NADH/NADPH serving as electron donors. Moreover, SEM and EDX analysis show that SeNPs were found on the QZB-2 cell surface (Figures 6A,B), while TEM analysis illustrates that SeNPs were located in the extracellular space or cell membrane (Figure 7). Altogether, these findings suggest that the QZB-2 strain produced SeNPs within the cell and then released them into the medium.
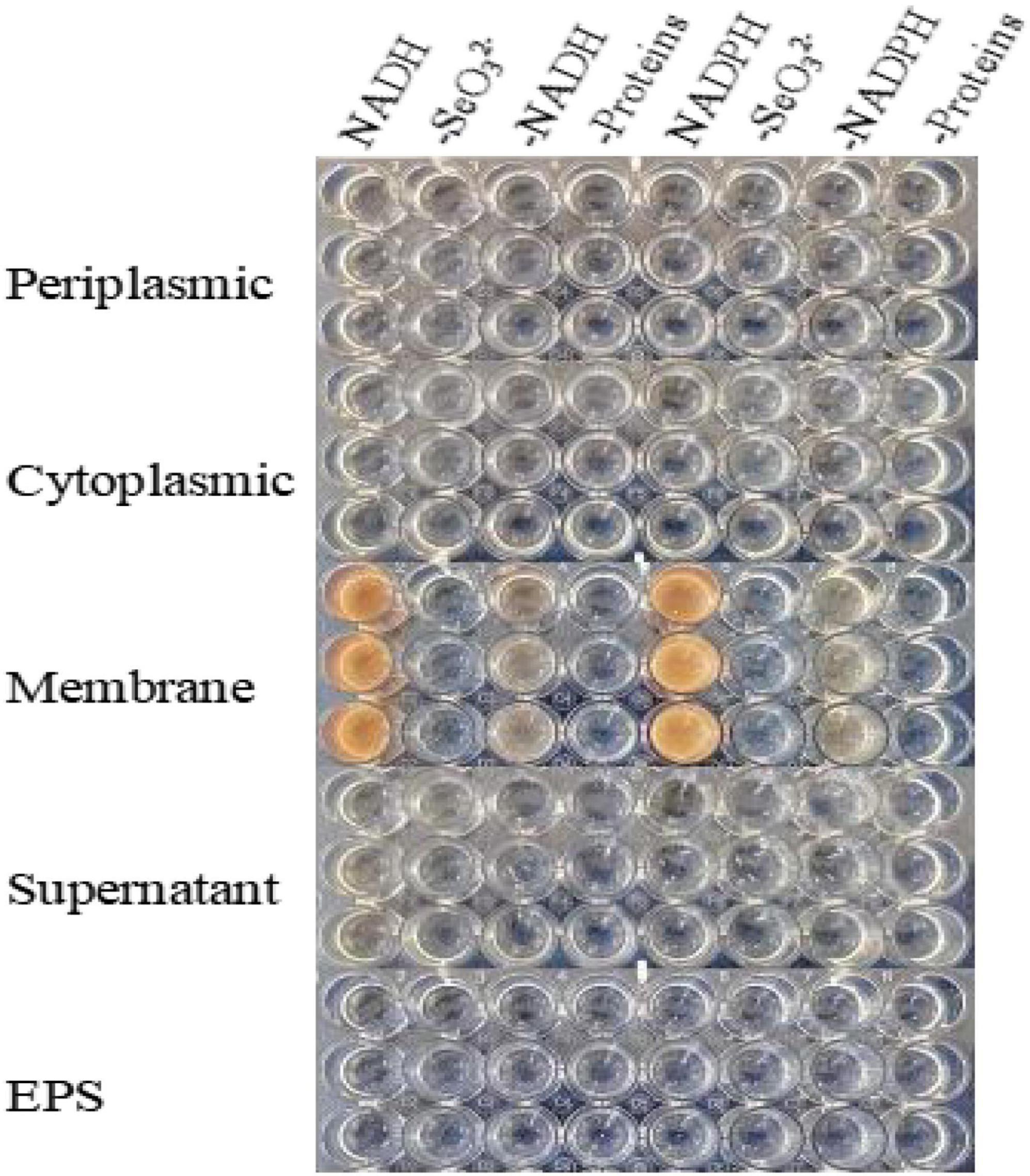
Figure 5. In vitro SeO32– reducing activity assays on different subcellular fractions (cytoplasmic, periplasmic, and membrane), supernatant, and exopolysaccharide (EPS). All experiments were performed in duplicate, with addition of 2 mM SeO32– and 2 mM NADPH or NADH. While 3 following control negatives were performed: without protein fractions, without selenite, without NADPH or NADH.
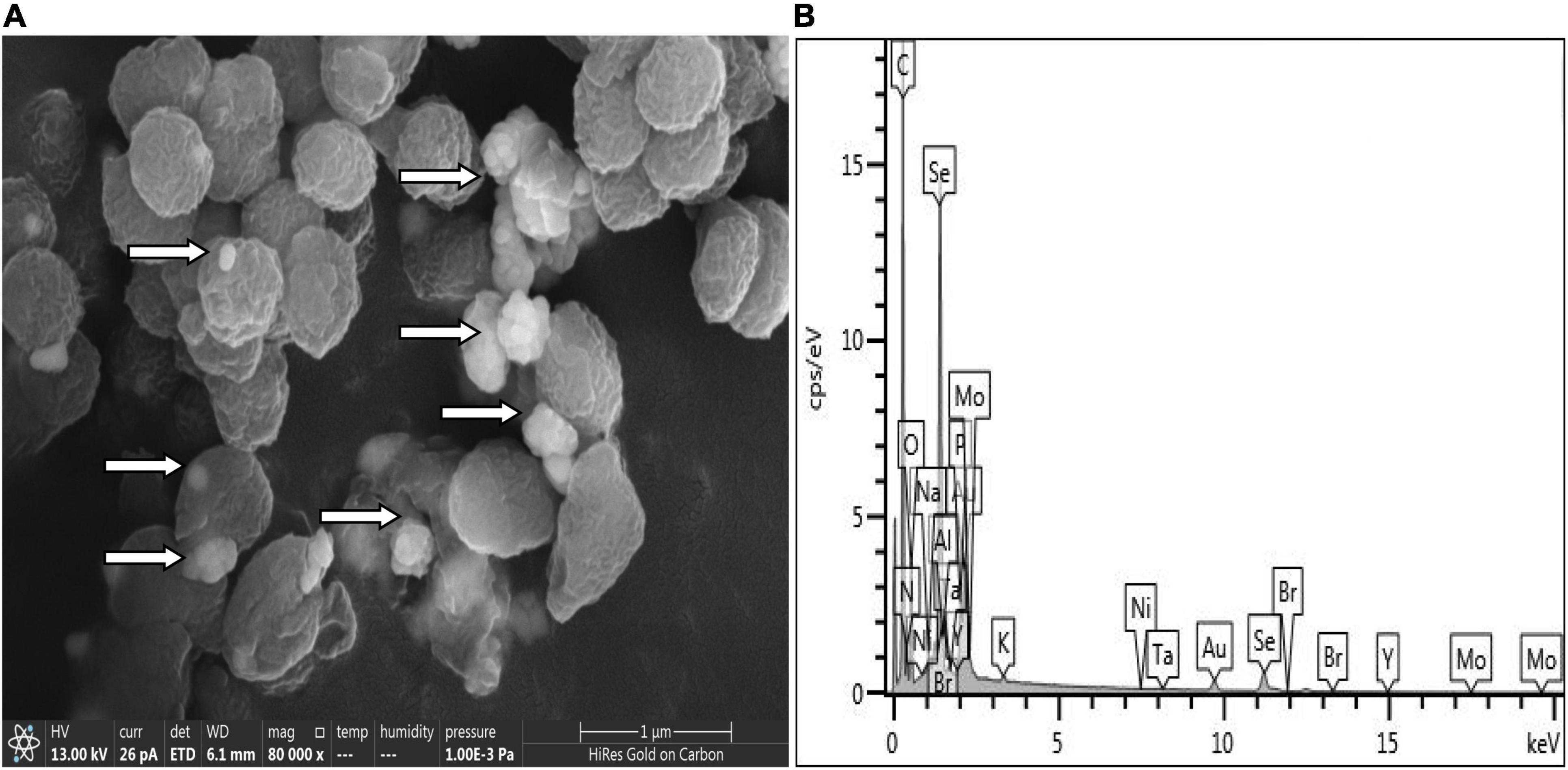
Figure 6. Scanning electron microscopy (SEM) (A) and EDX (B) analysis of Proteus mirabilis QZB-2 cultures grown in presence of 2 mM selenite.
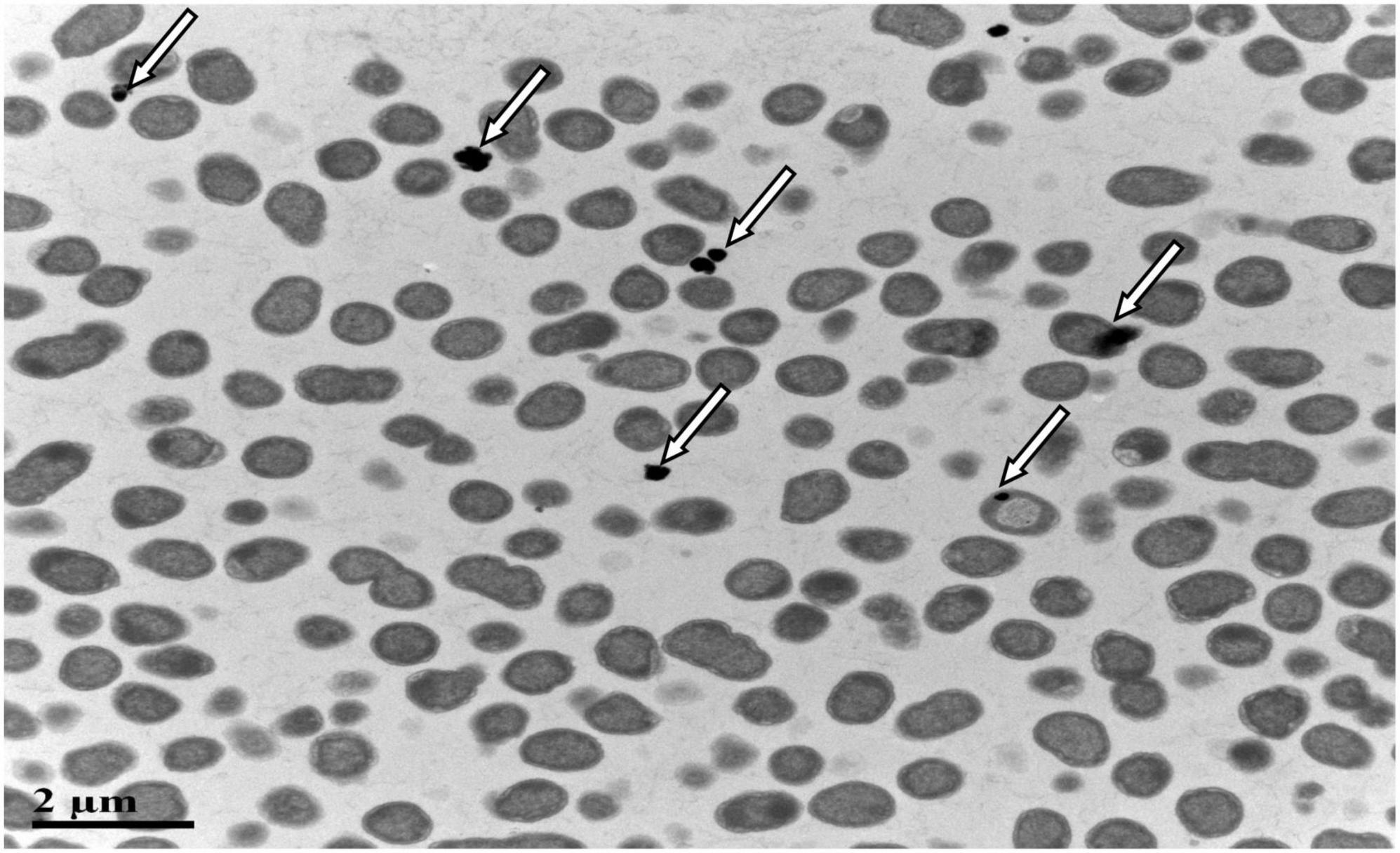
Figure 7. Transmission electron microscopy (TEM) micrographs showing SeNPs produced by Proteus mirabilis QZB-2 after 24 h of incubation with 2 mM sodium selenite.
Characterization of Selenium Nanoparticles
Results of DLS analysis of the purified SeNPs are presented in Figures 8A,B. They had an average hydrodynamic diameter of 152.0 ± 10.2 nm (Figures 8B,C). Moreover, the existence of Se was determined by EDX analysis, with selenium-specific peaks observed at 1.38 and 11.22 keV (Figure 8D), and Figure 9A shows the XRD traces of SeNPs. The diffraction peaks at 2θ = 23.6, 29.7, 41.3, 43.7, 45.4, 51.9, 56.3, 61.9, 65.2, and 68.6° indicated the presence of the pure selenium in the sample (Shin et al., 2007). In addition, the FTIR spectra of the SeNPs are shown in Figure 9B. The absorption band at 3,280 cm–1 is due to the stretching vibration of protein-modified N-H, amide A, or amide II (Zonaro et al., 2017; Wang et al., 2018b). The weak absorption peaks at 2,960 and 2,927 cm–1 represent the symmetric stretching vibrations of C-H in the sugar ring or peptide chain (Xu et al., 2018). The peaks at 1655, 1533, and 1232 cm–1 are accompanied by a low-intensity band and represent amide I, amide II, and amide III, respectively, which is a typical protein pattern (Kamnev et al., 2017; Wang et al., 2018b). The peak at 1,400 cm–1 is because of the symmetric stretching vibration of carboxylate (COO−), while its asymmetric counterpart can be seen at 1,655 cm–1 (Lampis et al., 2017; Zonaro et al., 2017; Wang et al., 2018b). The bands at 1,071 cm–1 are typical of C–O vibrations in carbohydrates, which may suggest the existence of polysaccharides (Tugarova et al., 2018; Wang et al., 2018b). The results of FTIR analysis clearly show that the surface of the SeNPs produced by P. mirabilis QZB-2 contained organic residues from carbohydrates, lipids, and proteins. The composition of these organic groups was in accordance with those produced by S. maltophilia SeITE02 and P. mirabilis YC801 (Lampis et al., 2017; Wang et al., 2018a). These organic groups could participate in SeO32– reduction, as well as SeNP formation and stabilization processes.
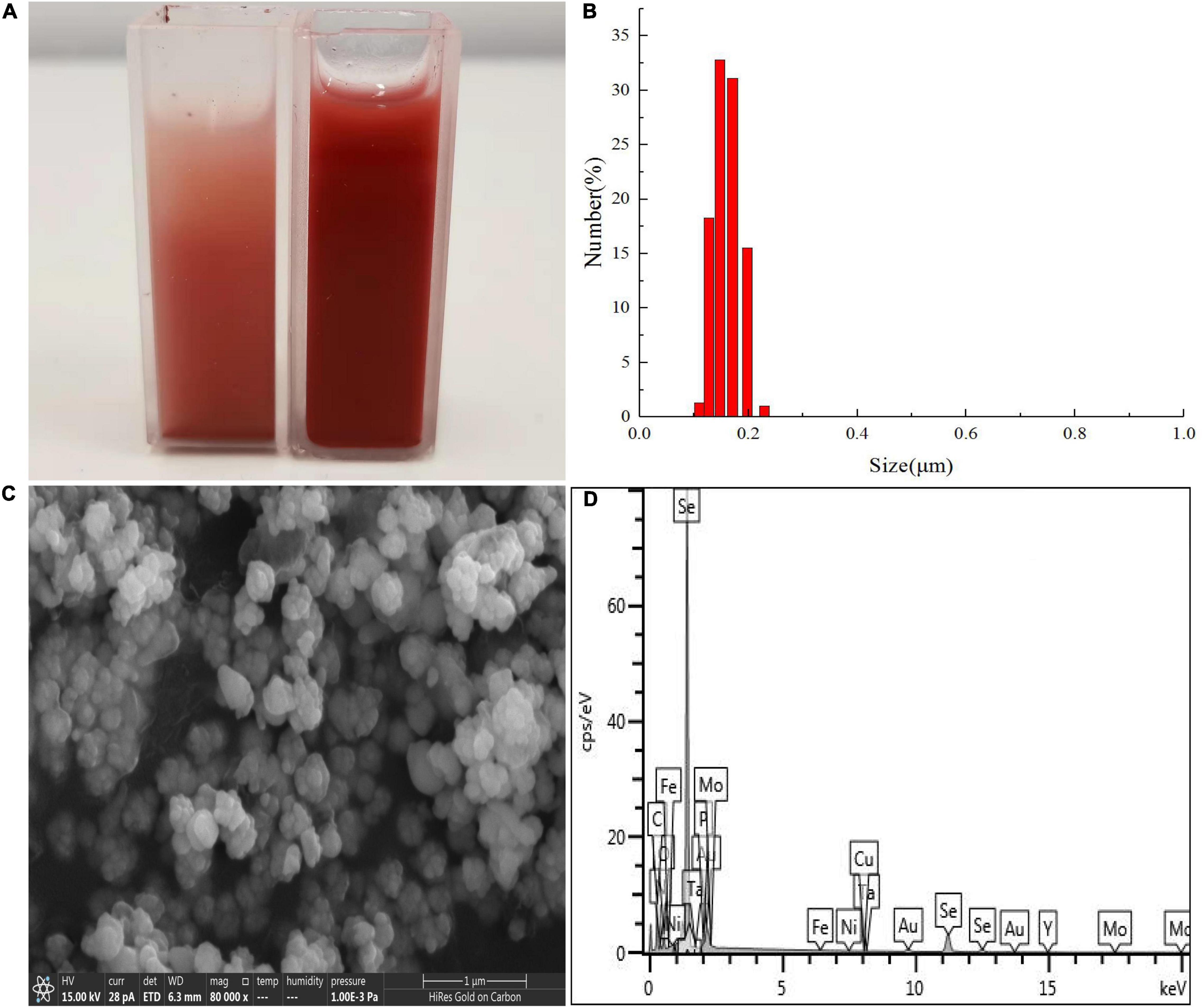
Figure 8. Dynamic light scattering (DLS) spectra of purified SeNPs produced by Proteus mirabilis QZB-2 in LB supplemented with 2.0 mM selenite. (A) Selenite-dosing cells (left) and purified nano-selenium (right); (B) size distribution of purified SeNPs; (C) SEM micrographs of purified SeNPs. (D) EDX analysis of purified SeNPs showing its selenium composition.
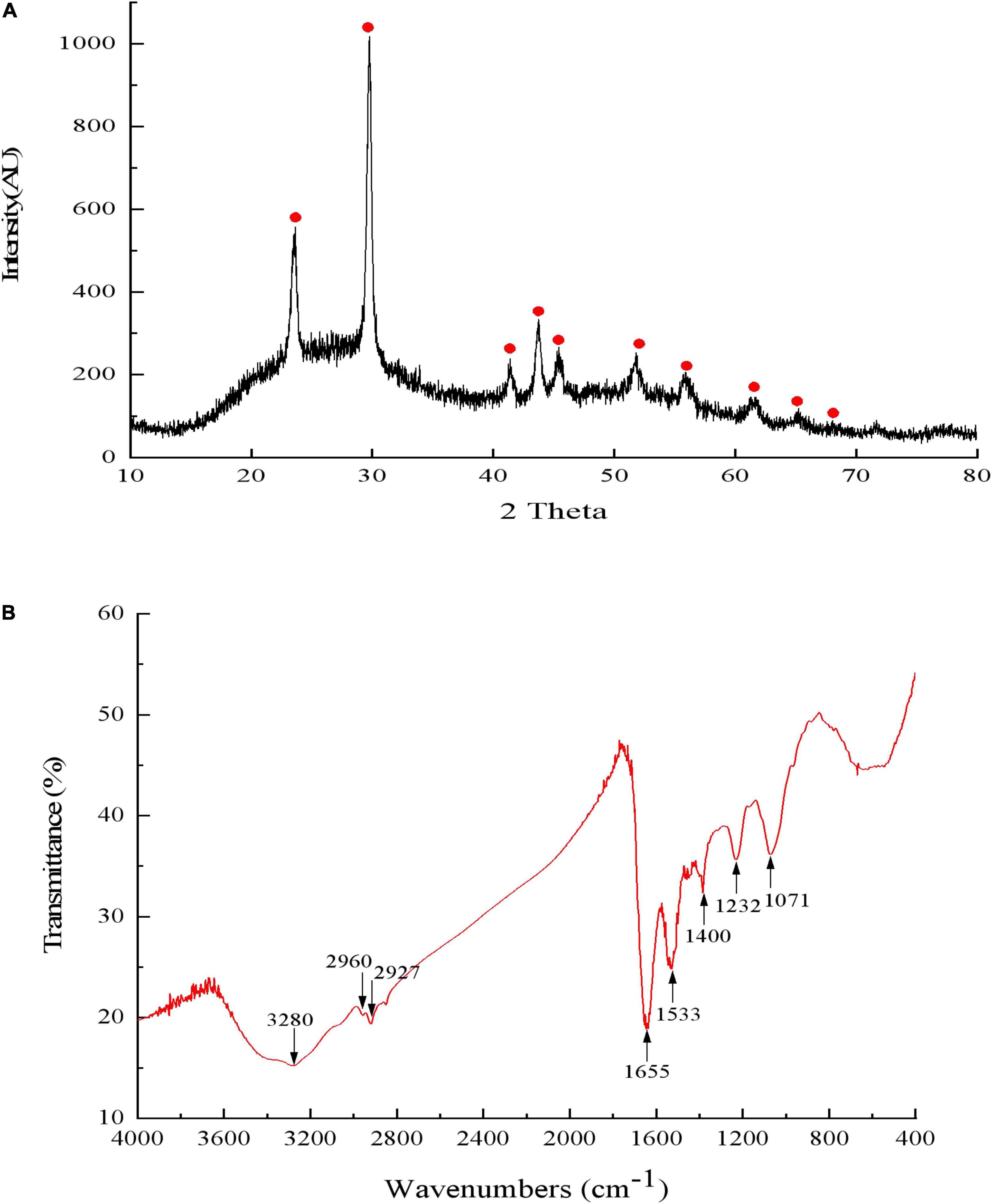
Figure 9. The X-ray diffraction spectrogram (A) and FTIR spectrum (B) of SeNPs synthesized by Proteus mirabilis QZB-2.
A previous study showed that selenite and selenate were not suitable additives when applied as plant growth fertilizers owing to their high toxicity (Deng et al., 2017). However, when using as a fertilizer, nano-Se could significantly enhance the Se content of crops (Li et al., 2020, 2021), which can safely increase human Se intake. Our study found that strain QZB-2 could tolerate high level of SeO32–; it rapidly reduces SeO32– to Se0 while simultaneously synthesizing SeNPs. This result indicates strain QZB-2 could be used for SeNPs synthesis. However, the application of these synthesized nanoparticles in fertilizer has not been well-characterized and requires further study.
Conclusion
The highly selenite-tolerant (up to 300 mM) strain P. mirabilis QZB-2 was isolated from a naturally occurring Se-rich paddy soil. QZB-2 reduced almost all selenite to form selenium nanoparticles within 18 h. The SeO32– reduction activity in QZB-2 cells was attributed to the cell membrane fraction with NADH/NADPH serving as electron donors. Therefore, the QZB-2 bacterial strain is a promising candidate for the production of novel nano-selenium fertilizers owing to its simultaneously high SeO32– tolerance and rapid reduction rate.
Data Availability Statement
The original contributions presented in the study are publicly available. This data can be found here: OL629178.
Author Contributions
XH and DJ: conceptualization, writing—review and editing, and funding acquisition. JH: methodology, data curation, visualization, supervision, and writing—original draft preparation. MW: software, formal analysis, and investigation. XH, MW, and DJ: validation. XH: resources and project administration. All authors have read and agreed to the published version of the manuscript.
Funding
This work was supported by the National Natural Science Foundation of China (41661076 and 42107333).
Conflict of Interest
The authors declare that the research was conducted in the absence of any commercial or financial relationships that could be construed as a potential conflict of interest.
Publisher’s Note
All claims expressed in this article are solely those of the authors and do not necessarily represent those of their affiliated organizations, or those of the publisher, the editors and the reviewers. Any product that may be evaluated in this article, or claim that may be made by its manufacturer, is not guaranteed or endorsed by the publisher.
Supplementary Material
The Supplementary Material for this article can be found online at: https://www.frontiersin.org/articles/10.3389/fmicb.2022.862130/full#supplementary-material
References
Avendaño, R., Chaves, N., Fuentes, P., Sánchez, E., Jiménez, J. I., and Chavarría, M. (2016). Production of selenium nanoparticles in Pseudomonas putida KT2440. Sci. Rep. 6:37155. doi: 10.1038/srep37155
Bao, P., Huang, H., Hu, Z. Y., Häggblom, M. M., and Zhu, Y. G. (2013). Impact of temperature, CO2 fixation and nitrate reduction on selenium reduction, by a paddy soil Clostridium strain. J. Appl. Microbiol. 114, 703–712. doi: 10.1111/jam.12084
Bao, P., Xiao, K. Q., Wang, H. J., Xu, H., Xu, P. P., Jia, Y., et al. (2016). Characterization and potential applications of a selenium nanoparticle producing and nitrate reducing bacterium Bacillus oryziterrae sp nov. Sci. Rep.-U.K 6:34054. doi: 10.1038/srep34054
Blum, J. S., Bindi, A. B., Buzzelli, J., Stolz, J. F., and Oremland, R. S. (1998). Bacillus arsenicoselenatis, sp. nov. and Bacillus selenitireducens, sp. nov.: two haloalkaliphiles from mono lake, California that respire oxyanions of selenium and arsenic. Arch. Microbiol. 171, 19–30. doi: 10.1007/s002030050673
Deng, X. F., Liu, K. Z., Li, M. F., Zhang, W., Zhao, X. H., Zhao, Z. Q., et al. (2017). Difference of selenium uptake and distribution in the plant and selenium form in the grains of rice with foliar spray of selenite or selenate at different stages. Field Crops Res. 211, 165–171. doi: 10.1016/j.fcr.2017.06.008
Dinh, Q. T., Cui, Z. W., Huang, J., Tran, T. A. T., Wang, D., Yang, W. X., et al. (2018). Selenium distribution in the Chinese environment and its relationship with human health: a review. Environ. Int. 112, 294–309. doi: 10.1016/j.envint.2017.12.035
Drzewiecka, D. (2016). Significance and roles of proteus spp. bacteria in natural environments. Microb. Ecol. 72, 741–758. doi: 10.1007/s00248-015-0720-6
Dungan, R., Yates, S., and Frankenberger, W. (2003). Transformation of selenate and selenite by Stenophomonas maltophilia isolated from a seleniferous agricultural drainage pond sediment. Environ. Microbiol. 5, 287–295. doi: 10.1046/j.1462-2920.2003.00410.x
Fischer, S., Krause, T., Lederer, F., Mohamed, M., Shevchenko, A., Hübner, R., et al. (2019). Bacillus safensis JG-B5T affects the fate of selenium by extracellular production of colloidally less stable selenium nanoparticles. J. Hazard. Mater. 384, 121–146. doi: 10.1016/j.jhazmat.2019.121146
Ge, S. M., Dong, X. J., Zhou, J. M., and Ge, S. C. (2013). Comparative evaluations on bio-treatment of hexavalent chromate by resting cells of Pseudochrobactrum sp. and Proteus sp. in wastewater. J. Environ. Manage. 126, 7–12. doi: 10.1016/j.jenvman.2013.04.011
Huang, S. W., Wang, Y. T., Tang, C. G., Jia, H. L., and Wu, L. F. (2021). Speeding up selenite bioremediation using the highly selenite-tolerant strain Providencia rettgeri HF16-A novel mechanism of selenite reduction based on proteomic analysis. J. Hazard. Mater. 406:124690. doi: 10.1016/j.jhazmat.2020.124690
Huang, X. J., Ni, J. P., Yang, C., Feng, M., Li, Z. L., and Xie, D. T. (2018b). Efficient ammonium removal by bacteria RhodoPseudomonas isolated from natural landscape water: China case study. Water 10:1107. doi: 10.3390/w10081107
Huang, X. J., Feng, M., Ni, C. S., Xie, D. T., and Li, Z. L. (2018a). Enhancement of nitrogen and phosphorus removal in landscape water using polymeric ferric sulfate as well as the synergistic effect of four kinds of natural rocks as promoter. Environ. Sci. Pollut. Re. 25, 12859–12867. doi: 10.1007/s11356-018-1553-x
Islam, F., Yasmeen, T., Riaz, M., Arif, M. S., Ali, S., and Raza, S. H. (2014). Proteus mirabilis alleviates zinc toxicity by preventing oxidative stress in maize (Zea mays) plants. Ecotoxicol. Environ. Saf. 110, 143–152. doi: 10.1016/j.ecoenv.2014.08.020
Kamnev, A. A., Mamchenkova, P. V., Dyatlova, Y. A., and Tugarova, A. V. (2017). FTIR spectroscopic studies of selenite reduction by cells of the rhizobacterium Azospirillum brasilense Sp7 and the formation of selenium nanoparticles. J. Mol. Struct. 1140, 106–112. doi: 10.1016/j.molstruc.2016.12.003
Khoei, N. S., Lampis, S., Zonaro, E., Yrjälä, K., Bernardi, P., and Vallini, G. (2017). Insights into selenite reduction and biogenesis of elemental selenium nanoparticles by two environmental isolates of Burkholderia fungorum. New Biotechnol. 34, 1–11. doi: 10.1016/j.nbt.2016.10.002
Kumar, A., and Prasad, K. S. (2021). Role of nano-selenium in health and environment. J. Biotechnol. 325, 152–163. doi: 10.1016/j.jbiotec.2020.11.004
Kuroda, M., Notaguchi, E., Sato, A., Yoshioka, M., Hasegawa, A., Kagami, T., et al. (2011). Characterization of Pseudomonas stutzeri NT-I capable of removing soluble selenium from the aqueous phase under aerobic conditions. J. Biosci. Bioeng. 112, 259–264. doi: 10.1016/j.jbiosc.2011.05.012
Lampis, S., Zonaro, E., Bertolini, C., Bernardi, P., Butler, C. S., and Vallini, G. (2014). Delayed formation of zero-valent selenium nanoparticles by Bacillus mycoides SeITE01 as a consequence of selenite reduction under aerobic conditions. Microb. Cell Factories 13, 1–14. doi: 10.1186/1475-2859-13-35
Lampis, S., Zonaro, E., Bertolini, C., Cecconi, D., Monti, F., Micaroni, M., et al. (2017). Selenite biotransformation and detoxification by Stenotrophomonas maltophilia SeITE02: novel clues on the route to bacterial biogenesis of selenium nanoparticles. J. Hazard. Mater. 324, 3–14. doi: 10.1016/j.jhazmat.2016.02.035
Li, B. Z., Liu, N., Li, Y. Q., Jing, W. X., Fan, J. H., Li, D., et al. (2014a). Reduction of selenite to red elemental selenium by RhodoPseudomonas palustris strain N. PloS one. 9:e95955. doi: 10.1371/journal.pone.0095955
Li, D., Zhou, C. R., Zhang, J. B., An, Q. S., Wu, Y. L., Li, J. Q., et al. (2020). Nanoselenium foliar applications enhance the nutrient quality of pepper by activating the capsaicinoid synthetic pathway. J. Agric. Food Chem. 68, 9888–9895. doi: 10.1021/acs.jafc.0c03044
Li, D. B., Cheng, Y. Y., Wu, C., Li, W. W., Li, N., Yang, Z. C., et al. (2014b). Selenite reduction by Shewanella oneidensis MR-1 is mediated by fumarate reductase in periplasm. Sci. Rep. 4, 1–7. doi: 10.1038/srep03735
Li, J. H., Yang, W. P., Guo, A. N., Qi, Z. W., Chen, J., Huang, T. M., et al. (2021). Combined foliar and soil selenium fertilizer increased the grain yield, quality, total Se, and organic Se content in naked oats. J. Cereal Sci. 100:103265. doi: 10.1016/j.jcs.2021.103265
Liu, C., Tan, L., Zhang, L. M., Tian, W. Q., and Ma, L. Q. (2021). A review of the distribution of antibiotics in water in different regions of China and current antibiotic degradation pathways. Front. Env. Sci.Switz. 9:692298. doi: 10.3389/fenvs.2021.692298
Luo, H. W., Du, B., He, L. X., Zheng, A. X., Pan, S. G., and Tang, X. R. (2019). Foliar application of sodium selenate induces regulation in yield formation, grain quality characters and 2-acetyl-1-pyrroline biosynthesis in fragrant rice. BMC Plant Biol. 19, 1–12. doi: 10.1186/s12870-019-2104-4
Oremland, R. S., Herbel, M. J., Blum, J. S., Langley, S., Beveridge, T. J., Ajayan, P. M., et al. (2004). Structural and spectral features of selenium nanospheres produced by Se-respiring bacteria. Appl. Environ. Microbiol. 70, 52–60. doi: 10.1128/AEM.70.1.52-60.2004
Rani, A., Shouche, Y. S., and Goel, R. (2008). Declination of copper toxicity in pigeon pea and soil system by growth-promoting Proteus vulgaris KNP3 Strain. Curr. Microbiol. 57, 78–82. doi: 10.1007/s00284-008-9156-2
Ruiz Fresneda, M. A., Delgado Martín, J., Gómez Bolívar, J., Fernández Cantos, M. V., Bosch-Estévez, G., Martínez Moreno, M. F., et al. (2018). Green synthesis and biotransformation of amorphous Se nanospheres to trigonal 1D Se nanostructures: impact on Se mobility within the concept of radioactive waste disposal. Environ. Sci. Nano. 5, 2103–2116. doi: 10.1039/C8EN00221E
Shin, Y. S., Blackwood, J. M., Bae, I. T., Arey, B. W., and Exarhos, G. J. (2007). Synthesis and stabilization of selenium nanoparticles on cellulose nanocrystal. Mater Lett. 61, 4297–4300. doi: 10.1016/j.matlet.2007.01.091
Song, D. G., Li, X. X., Cheng, Y. Z., Xiao, X., Lu, Z. Q., Wang, Y. Z., et al. (2017). Aerobic biogenesis of selenium nanoparticles by Enterobacter cloacae Z0206 as a consequence of fumarate reductase mediated selenite reduction. Sci. Rep. 7:3239. doi: 10.1038/s41598-017-03558-3
Soudi, M., Ghazvini, P., Khajeh, K., and Gharavi, S. (2008). Bioprocessing of seleno-oxyanions and tellurite in a novel Bacillus sp strain STG-83: a solution to removal of toxic oxyanions in presence of nitrate. J. Hazard. Mater. 165, 71–77. doi: 10.1016/j.jhazmat.2008.09.065
Tugarova, A. V., Mamchenkova, P. V., Dyatlova, Y. A., and Kamnev, A. A. (2018). FTIR and raman spectroscopic studies of selenium nanoparticles synthesised by the bacterium Azospirillum thiophilum. Spectrochim. Acta Part A 192, 458–463. doi: 10.1016/j.saa.2017.11.050
Wang, Y. T., Shu, X., Hou, J. Y., Lu, W. L., Zhao, W. W., Huang, S. W., et al. (2018a). Selenium nanoparticle synthesized by Proteus mirabilis YC801: an efficacious pathway for selenite biotransformation and detoxification. Int. J, Mol, Sci. 19:3809. doi: 10.3390/ijms19123809
Wang, Y. T., Shu, X., Zhou, Q., Fan, T., Wang, T. C., Chen, X., et al. (2018b). Selenite reduction and the biogenesis of selenium nanoparticles by Alcaligenesfaecalis Se03 isolated from the gut of Monochamus alternatus (Coleoptera: Cerambycidae). Int. J. Mol. Sci. 19:2799. doi: 10.3390/ijms19092799
Xu, C. L., Qiao, L., Guo, Y., Ma, L., and Cheng, Y. Y. (2018). Preparation, characteristics and antioxidant activity of polysaccharides and proteins-capped selenium nanoparticles synthesized by Lactobacillus casei ATCC 393. Carbohydr. Polym. 195, 576–585. doi: 10.1016/j.carbpol.2018.04.110
Yan, J., Chen, X. J., Zhu, T. G., Zhang, Z. P., and Fan, J. B. (2021). Effects of selenium fertilizer application on yield and selenium accumulation characteristics of different japonica rice varieties. Sustainability 13:10284. doi: 10.3390/su131810284
Zahedi, S. M., Hosseini, M. S., Meybodi, N. D. H., and Teixeira da Silva, J. A. (2019). Foliar application of selenium and nano-selenium affects pomegranate (Punica granatum cv. Malase Saveh) fruit yield and quality. S. Afr. J. Bot. 124, 350–358. doi: 10.1016/j.sajb.2019.05.019
Zhang, H. Q., Zhao, Z. Q., Zhang, X., Zhang, W., Huang, L. Q., Zhang, Z. Z., et al. (2019a). Effects of foliar application of selenate and selenite at different growth stages on Selenium accumulation and speciation in potato (Solanum tuberosum L.). Food Chem. 286, 550–556. doi: 10.1016/j.foodchem.2019.01.185
Zhang, J., Wang, Y., Shao, Z. Y., Li, J., Zan, S. T., Zhou, S. B., et al. (2019b). Two selenium tolerant Lysinibacillus sp. strains are capable of reducing selenite to elemental Se efficiently under aerobic conditions. J. Environ. Sci. 77, 238–249. doi: 10.1016/j.jes.2018.08.002
Zhang, Y. Q., and Frankenberger, W. (2006). Removal of selenate in river and drainage waters by Citrobacter braakii enhanced with zero-valent iron. J. Agric. Food Chem. 54, 152–156. doi: 10.1021/jf058124o
Zhang, Y. Q., and Frankenberger, W. (2007). Supplementing Bacillus sp. RS1 with Dechloromonas sp. HZ for enhancing selenate reduction in agricultural drainage water. Sci. Total Environ. 372, 397–405. doi: 10.1016/j.scitotenv.2006.10.027
Zheng, S. X., Su, J., Wang, L., Yao, R., Wang, D., Deng, Y. J., et al. (2014). Selenite reduction by the obligate aerobic bacterium Comamonas testosteroni S44 isolated from a metal-contaminated soil. BMC Microbiol. 14:204. doi: 10.1186/s12866-014-0204-8
Zhu, Y. Y., Ren, B. Y., Li, H. F., Lin, Z. Q., Bañuelos, G., Li, L., et al. (2018). Biosynthesis of selenium nanoparticles and effects of selenite, selenate, and selenomethionine on cell growth and morphology in Rahnella aquatilis HX2. Appl. Microbiol. Biotechnol. 102, 6191–6205. doi: 10.1007/s00253-018-9060-z
Zonaro, E., Piacenza, E., Presentato, A., Monti, F., Dell’Anna, R., Lampis, S., et al. (2017). Ochrobactrum sp. MPV1 from a dump of roasted pyrites can be exploited as bacterial catalyst for the biogenesis of selenium and tellurium nanoparticles. Microb. Cell Fact. 16:215. doi: 10.1186/s12934-017-0826-2
Keywords: Proteus mirabilis, selenite reduction, membrane, selenium nanoparticles, reduction efficiency
Citation: Huang JL, Jiang DH, Wang MS and Huang XJ (2022) Highly Selenite-Tolerant Strain Proteus mirabilis QZB-2 Rapidly Reduces Selenite to Selenium Nanoparticles in the Cell Membrane. Front. Microbiol. 13:862130. doi: 10.3389/fmicb.2022.862130
Received: 25 January 2022; Accepted: 14 March 2022;
Published: 11 April 2022.
Edited by:
Qiaoyun Huang, Huazhong Agricultural University, ChinaReviewed by:
Judith Maria Braganca, Birla Institute of Technology and Science, IndiaKe-Qing Xiao, University of Leeds, United Kingdom
Copyright © 2022 Huang, Jiang, Wang and Huang. This is an open-access article distributed under the terms of the Creative Commons Attribution License (CC BY). The use, distribution or reproduction in other forums is permitted, provided the original author(s) and the copyright owner(s) are credited and that the original publication in this journal is cited, in accordance with accepted academic practice. No use, distribution or reproduction is permitted which does not comply with these terms.
*Correspondence: DaiHua Jiang, dhjiang2008@gxu.edu.cn; XueJiao Huang, hxuejiao0412@sina.com