- 1School of Life Sciences, Ludong University, Yantai, China
- 2Department of Prosthodontics, Yantai Stomatological Hospital Affiliated to Binzhou Medical University, Yantai, China
- 3Shandong Aquaculture Environmental Control Engineering Laboratory, Yantai, China
- 4Shandong Provincial Key Laboratory of Quality Safety Monitoring and Risk Assessment for Animal Products, Institute of Veterinary Drug Quality Inspection of Shandong Province, Jinan, China
- 5Institute of Animal Sciences, Chinese Academy of Agricultural Sciences, Beijing, China
The beneficial effects of the probiotic strain Lactiplantibacillus plantarum (formerly Lactobacillus plantarum) are based on its adherence and colonization ability in the gut. However, little is known about the migration and long-term gut colonization of the strain. This study evaluated the gut colonization modes of Lactiplantibacillus plantarum RS-09 to identify the strain with long-term gut colonization potential. We established CFDA/SE-labeled RS-09 to study the temporal and spatial distribution of RS-09 in the intestine as well as to analyze its persistence in different parts of the intestine by flow cytometry. This study has shown that the RS-09 strain maintains strong adhesion abilities under acid (pH 2.5) and base (pH 8.5) conditions. In addition, CFDA/SE can be used as an indicator for the labeling of L. plantarum RS-09 in the intestinal tract in vivo. We established a growth kinetics model of RS-09 to elucidate its persistence in the intestine. In vivo persistence experiments showed that the persistence rate of RS-09 was the highest in the cecum (69.5%) and the lowest in the duodenum (12.8%) at 96 h. After 20 days, RS-09 was predominantly localized in the cecum and colon steadily. These studies provide new insights into the long-term persistence of L. plantarum in the gastrointestinal tract. The CFDA/SE label system may be used to study the in vivo colonization dynamics of other probiotic strains.
Introduction
Lactobacillus species are Gram-positive, homofermentative, thermophilic and non-spore-forming rods (Zheng et al., 2020). It is a beneficial microorganism commonly found in the gastrointestinal tract of humans and animals (Seddik et al., 2017). Lactiplantibacillus plantarum, previously named “Lactobacillus plantarum,” is one of the most widely distributed bacterium (Fidanza et al., 2021). It is acid resistant and is commonly found in fermented plant foods such as kimchi, silage, fruits and beverages (Barache et al., 2020). It has important physiological functions. Studies have shown that L. plantarum is a dominant bacterium in the intestine of humans and animals and has strong antibacterial activity against pathogenic microorganisms (Zhu et al., 2014; Li et al., 2016), as well as good tolerance to various stresses, including those present in the gastrointestinal tract. In view of the safety of L. plantarum and its beneficial effects on human health, a large number of studies have been conducted to isolate and screen new strains with probiotic characteristics (Goel et al., 2020; Garcia-Gonzalez et al., 2021) and the ability to sustain processing conditions for culture production (Sauvageau et al., 2012).
According to the Food and Agriculture Organization of the United Nations/World Health Organization (FAO/WHO) regulations (2002), probiotics must have a high tolerance to the gastrointestinal (GIT) conditions as well as high adherence in order to serve as a probiotic and maintain the balance of the intestinal microbiota (Hardy et al., 2013; Monteagudo-Mera et al., 2019). Studies have shown that gastrointestinal conditions (pH, bile, digestive enzymes) and the acid and bile salt tolerance of bacteria have important impacts on the adhesion ability of probiotics (Damaceno et al., 2021; Pumriw et al., 2021; Tarrah et al., 2021). To ensure that L. plantarum is able to survive after passage through the gastrointestinal tract, evaluating the effect of acid-base stress on this organism has important significance for studying its adhesion ability.
The ability to adhere to the intestinal mucosa is another important criterion for screening probiotics (Chua et al., 2020). Adhesion can prolong the amount of time that probiotics are present in the intestines and allows for close contact between probiotics and intestinal epithelial cells. Different methods and models have been used to assess the adhesion of probiotics. HT-29 cell lines are the main cell models for adhesion ability assessments, both within and outside of China because these cell lines consist of mature intestinal epithelial cells (Trukhachev et al., 2021). However, bacterial persistence is easily antagonized by other strains in the intestinal microbiota. It is not sufficient to test probiotic adhesion abilities through in vitro experiments alone and more convincing evidence is needed to confirm in vitro results (Papadimitriou et al., 2015). Therefore, it is important to study the dynamic distribution and persistence patterns of lactobacilli in different segments of the gastrointestinal tract.
In recent years, flow cytometry (FCM) has been widely used for real-time monitoring and quantitative analysis of single cells due to its advantages of high speed, high precision and good accuracy. This technique lays the foundation for studying whether probiotics can adhere to and colonize host cells. For example, the adherence of flourescein isothiocyanate (FITC)-labeled L. plantarum can be clearly observed by fluorescence microscopy, which allows for a quantitative approach for studying adhesion to intestinal epithelial cells. However, FITC has disadvantages, including relatively high levels of photobleaching, high pH sensitivity and a broad spectrum, which make quantitative measurements of this fluorophore inaccurate and thereby reduces the effectiveness of detection (Xing et al., 2018). Carboxyfluorescein diacetate succinimidyl ester (CFDA/SE) is a lipophilic molecule that is minimally fluorescent until it enters cells by passive diffusion and esterases cleave the acetyl groups to form carboxyfluorescein succinimidyl ester (CFSE), which irreversibly reacts with the lysine residues of intracellular proteins to produce fluorescence (Cong et al., 2008). When a CFDA/SE-labeled cell divides, its progeny is endowed with half the number of carboxyfluorescein-tagged molecules and thus each cell division can be assessed by measuring the fluorescence via Flow cytometry. In addition, CFDA/SE efficiently stained bacteria without causing undesirable effects on cell adhesion or viability (Fuller et al., 2000). CFDA/SE has been widely used for bacterial labeling and more than 95% of CFDA/SE-stained bacterial strain incubated in artificial groundwater remained fluorescent for at least 28 days as determined by epifluorescent microscopy and flow cytometry (Fuller et al., 2000). However, no reports are available on the dynamic monitoring of CFDA/SE-labeled L. plantarum in the intestinal tract of animals.
Plant origin lactic acid bacteria (POLAB) have received increasing attention due to the ability to cope with multiple stresses (Lim et al., 2018). POLAB can often produce natural antibacterial and physiologically active substances. In this study, we isolated and purified L. plantarum strains from apple fermented juice. In this work, a reliable, rapid, and simple quantitative method was established using FCM to study the influence of L. plantarum adherence and persistence on intestinal epithelial cells. CFDA/SE fluorescent labeling was used for long-term dynamic monitoring of the distribution and persistence of L. plantarum in the intestine. Thereby, we can understand the adhesion processes of lactic acid bacteria in vivo and by fitting mathematical mode, we can obtain the persistence patterns of lactic acid bacteria in the intestine.
Materials and Methods
Bacterial Strains and Culture Conditions
Lactiplantibacillus plantarum RS-09 is a lactic acid bacterium successfully isolated from fermented apple and stored in our laboratory. The strain is stored in the China General Microbiological Culture Collection Center (CGMCC No. 17118). The glycerol-preserved RS-09 strain was inoculated into freshly prepared MRS broth (Solarbio, Beijing, China) and incubated under anaerobic conditions at anaerobe container system at 37°C for 24 h to obtain the bacterial suspension.
Strain Identification
A fully automated microbial identification system (VITEK 2 Compact, BioMérieux, Lyon, France) was used to analyze the biochemical reactions of the strain. The VITEK® 2 Anaerobic and Corynebacterial identification card was used to identify the isolated strains (Pence and Liesman, 2020). The identification results were compared to the data in the BioMérieux database.
We used the universal primers, 27F (5′-AGAGTTTGATCCTGGCTC AG-3′) and 1492R (5′-TACGGYTACCTTGTTACGACTT-3′), for the amplification of 16S rDNA using the Rapid PCR kit (Qiagen, Germantown, MD, United States). The 16S rDNA was sent to Invitrogen for sequencing. BLAST was used to analyze the sequences in comparison to those in the GenBank. The phylogenetic tree was built via the Neighbor-joining method using the MEGA 7 software.
Cells and Cell Culture
An HT-29 cell lines was cultured in Dulbecco’s modified Eagle’s medium (DMEM, Hyclone, NY, United States) supplemented with fetal bovine serum (Gibco, Grand Island, NY, United States) and incubated at 37°C in a water-jacketed incubator with 5% carbon dioxide. The cells were harvested by trypsinisation (0.25%, Sigma, St. Louis, MO, United States), washed with sterile phosphate buffer saline (PBS, beyotime, Shanghai, China), and counted using a hemocytometer. Cells were fed with a change of medium every 2 days. When reached about 80 per cent confluency, cells were harvested for the next experiment.
Acid and Bile Salt Tolerance
For testing the acid tolerance, the previously described method was followed with some modifications (Hussein et al., 2020). RS-09 was cultured in MRS broth at 37°C for 24 h. After incubation, 200 μL of the bacterial suspension was inoculated into 2 mL MRS broth, supplemented with HCl to mimic the gastric acidic conditions. The pH of the broth was adjusted to 1.5, 2.5, 3.5, 4.5 and 5.5, respectively, with 1 mol/L HCl. The bacterial solution was anaerobically cultured at 37°C for 2 h, 500 μL bacterial suspension was taken immediately before and after the incubation, and 10-fold serially diluted before spread-plating on MRS agar. After incubation at 37°C for 48 h, the colonies were counted. MRS medium (pH = 6.5) was used in the control group, and the survival rate was calculated using the following formula: survival rate (%) = (viable cell count in the treated group/viable cell count in the control group) × 100.
The bile salt tolerance of the strain was determined according to the method reported by Prins et al. (2010). In brief, the broth was supplemented with bile salts (Solarbio, Beijing, China) to final concentrations of 0.3, 0.5, 1.0, 1.5, and 2.0% (w/v), respectively. Cells grown in MRS broth without bile salt was used as control. The bacterial suspension was cultured for 2 h and inoculated onto MRS agar for colony counting. The survival rate was calculated using the following formula: survival rate (%) = (viable cell count in the treated group/viable cell count in the control group) × 100.
Scanning Electron Microscope Analysis
Lactiplantibacillus plantarum RS-09 was treated at different pH levels for 24 h, and the cells were collected by centrifugation (4,000 g, 10 min). For SEM, the bacteria were fixed with 2.5% glutaraldehyde for 2 h, and washed with phosphate buffer solution (PBS, pH 7.4). The samples were dehydrated with a series of density ethanol solutions (30, 50, 70, 80, 90, and 100%). Finally, the samples were replaced in tertiary-butanol alcohol at concentrations of 25, 50, and 75%. Samples were freeze dried for 24 h by Vacuum Freeze Dryer (Christ, Alpha 1–4 LDplus, Germany). Finally, the samples were covered with gold-plated membrane and observed with a Hitachi S-3400N (Hitachi, Tokyo, Japan).
CFDA/SE Staining of Cultured Bacteria
Lactiplantibacillus plantarum RS-09 was inoculated into freshly prepared MRS medium and anaerobically cultured to log phase at 37°C. The medium was then centrifuged (5,000 g, 10 min, 4°C) and the pellet was washed twice with pre-cooled phosphate-buffered saline (PBS) (pH = 7.2) and resuspended in PBS containing 20 μmol/L CFDA/SE (Solarbio, Beijing, China). After incubation at 37°C for 30 min in the dark, the samples were washed 5 times in PBS to remove unbound CFDA/SE and the bacteria were resuspended in MRS medium at a concentration of 108 CFU/mL, followed by anaerobic culture at 37°C for 0, 24, 48 and 72 h. The bacteria were collected and observed under a fluorescence microscope with excitation at 488 nm and analyze the positive rate by FCM.
Adhesion Assay
Human colon adenocarcinoma HT-29 cells in a monolayer were washed with PBS, and seeded separately in each well of 6-well plates containing cover slips. A monolayer of HT-29 cells (1 × 105 each well) was used for adhesion assays. Labeled or non-labeled L. plantarum strains cell pellet (108 CFU) collected via centrifugation (5,000 g, 10 min, 4°C) was then suspended in a DMEM medium to each well of a 6-well plate containing HT-29 cells and incubated at 37°C with 5% CO2 for 2 h. The 6-well plate was washed five times with PBS to remove non-adherent bacteria. For microscopic examination of adhered non-labeled L. plantarum, the bacteria were treated with Gram staining. The plate was air dried and examined under the inverted microscope (Olympus BX41, Japan, Olympus Corporation). A fluorescent microscope was used to observe the labeled L. plantarum adhering to the HT-29 cells. The adhesion ratio of L. plantarum on HT-29 cells was calculated by comparing the viable count using flow cytometry before and after adhesion. The following formula: survival rate (%) = (viable cell count in the treated group/viable cell count in the control group) × 100. Each assay was conducted at the same time in three independent experiments.
Intestinal Distribution of L. plantarum RS-09 in Mouse
All mouse experimental procedures used in this study were approved by the Laboratory Animal Management Committee of Ludong University. The animal model was established to monitor the temporal and spatial distribution of A fluorescently-labeled L. plantarum RS-09 in the gastrointestinal tract in vivo. A total of 180 C57B/L6 male mice aged 4–5 weeks were randomly divided into two experiments, with 90 mice in each experiments. One was used for FCM analysis and the other was used to obtain frozen tissue sections for observation.
The L. plantarum RS-09 strain was collected during log-phase growth, labeled with CFDA/SE and resuspended to 1 × 1010 CFU/mL in PBS. Then, 500 μL of cell suspension was administered to each mouse in the experimental group, while 500 μL of sterile PBS was administered to each mouse in the control group. At different time points, the contents from different parts of the intestine were collected and flow cytometric analysis was performed to determine the rate of CFDA/SE-labeled L. plantarum. Statistical analysis was performed using logistic regression to determine the relationship between variables (CFDA/SE and microbial count). MATLAB (Release 2018b, The MathWorks Inc., Natick, MA, United States) was used for data analysis to establish a growth kinetics model of CFDA/SE-labeled L. plantarum RS-09 persistence in the intestine. The model of bacterial count was fitted by the Fourier series function. The model equations are:
where a0 models a constant (intercept) term in the data and is associated with the i = 0 cosine term, w is the fundamental frequency of the signal, x is in hours from 0 to 480, n is the number of terms in the series. The values in the ileum were fitted using the sixth order Fourier series, in the cecum using the seventh order Fourier series and in the colon using the fourth order Fourier series.
Flow Cytometric Analysis
All experiments were performed using a FACS Calibur flow cytometer (Becton Dickinson, San Jose, CA, United States) equipped with an air-cooled 15 mW argon ion laser, emitting at a fixed wavelength of 488 nm. Fluorescent filters and detectors were all standard with green fluorescence collected in the FL1 channel (530 F 30 nm). All parameters were collected as logarithmic signals. Data were analyzed using FlowJo Software (FlowJo, LLC, Ashland, OR, United States).
Fluorescent Detection of L. plantarum RS-09 in Histological Slices
Samples of the ileum, cecum, and colon were harvested at different time points for frozen section preparation and observation. The tissue samples were fixed in a 4% formalin solution overnight. The formalin-fixed tissue samples were dehydrated with 10, 20, and 30% sucrose solutions for 30 min each. The tissue samples were embedded with a frozen embedding agent (OCT compound; Sakura, Japan) and frozen at −20°C for at least 30 min. Then, the embedded samples were held for 10 min in a precooled freezing microtome (Leica, Wetzlar, Germany), followed by sectioning. The frozen sections were observed under the fluorescence microscope.
Statistics
Statistical analysis was performed using SPSS V17.0 (SPSS Inc., Chicago, IL, United States) and figures were generated using GraphPad Prism version 5.0 (GraphPad Software Inc., San Diego, CA, United States). The data were expressed as the means ± SDs. The mean values obtained from the experiments were compared using Student’s test or one-way ANOVA. A P-value less than 0.05 was considered significant.
Results
Strain Identification
A fully automated, microbial identification system was used for the identification of the isolated strain. The accuracy of identification by VITEK was 91%. The results showed that the isolated strain was L. plantarum (Table 1). This strain can utilize a variety of sugars and sugar alcohols, including galactose, glucose and mannitol. However, this strain cannot utilize urease, D-xylose, or L-arabinose.
The sequencing results demonstrated that the 16S rDNA contained 1,385 base pairs, which were compared with the sequences in GenBank to draw a phylogenetic tree, as shown in Figure 1. Phylogenetic analysis showed 99% identity to the Lactiplantibacillus plantarum strain. The strain was preliminarily named as Lactiplantibacillus plantarum RS-09. Figure 1 demonstrated the relationship of RS-09 with other closely related L. plantarum using 16S rDNA gene sequences.
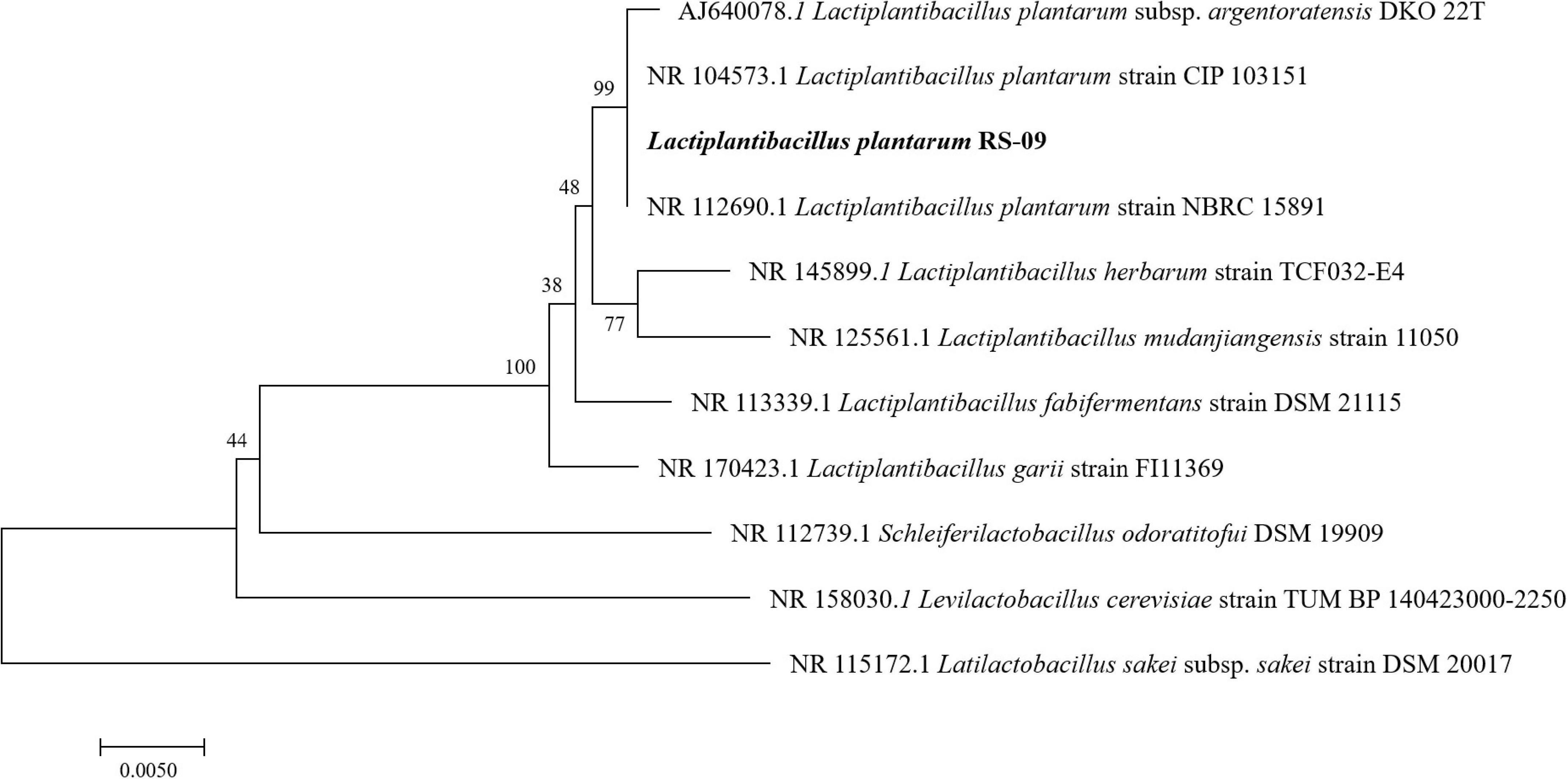
Figure 1. Phylogenetic trees based on 16S rDNA gene sequences showing the relationship between Lactiplantibacillus plantarum RS-09 and closely related species. Neighbor-joining dendrograms were generated with bootstrap trials of 500.
Acid and Bile Tolerances of L. plantarum RS-09
Resistance to gastric pH and bile are key features for bacteria to be able to survive in the gastrointestinal tract (GIT) and are some of the important selection criteria for probiotic. We tested the acid resistance of L. plantarum RS-09 at pH 1.5, 2.5, 3.5, 4.5 and 5.5. The results demonstrated that RS-09 significantly survived in the harsh pH condition (pH 1.5; 87.91%). After incubation at pH 2.5, 3.5, 4.5 and 5.5, the survival rates were greater than 90%, but without statistical difference (P > 0.05) (Table 2).
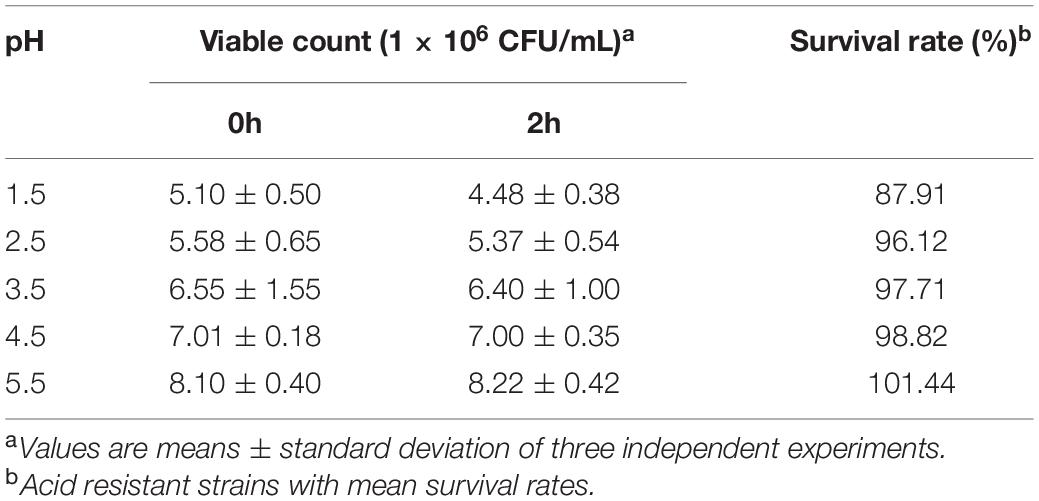
Table 2. Acid tolerance of Lactiplantibacillus plantarum RS-09 at different pH conditions for 2 h (n = 3, mean ± SD).
A 0.3–2.0% bile salt concentration was chosen for simulation of a bile salt challenge. At 37°C, the survival rate of L. plantarum RS-09 in 0.3% bile salt was 71.43%. When the bile salt concentration in the MRS media was increased to 1.5 or 2.0%, the survival rates were 68.95 and 62.76%, but without statistical difference (P > 0.05) (Table 3).
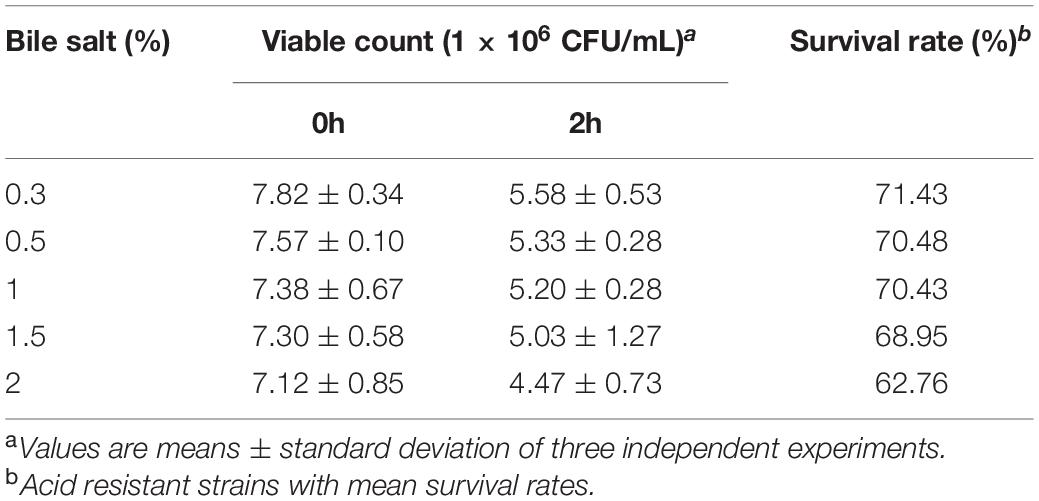
Table 3. Alkali resistance of L. plantarum RS-09 at different bile salt concentrations for 2 h (n = 3, mean ± SD).
Scanning Electron Microscope Analysis of L. plantarum RS-09
To determine if the pH stress did some damage to the cell membranes and affected the adhesive ability of the L. plantarum RS-09, we used SEM to observe the morphological changes of L. plantarum RS-09 cells. As shown in Figure 2, L. plantarum RS-09 is shaped as an elongated rod under acidic conditions but appears as a short rod with thick pili under basic condition. Under strong basic conditions, the structures of some L. plantarum RS-09 cells were fold, with cells wall collapsed (Figure 3). The acid resistance ability was stronger compared with the strains in the alkali stress conditions.
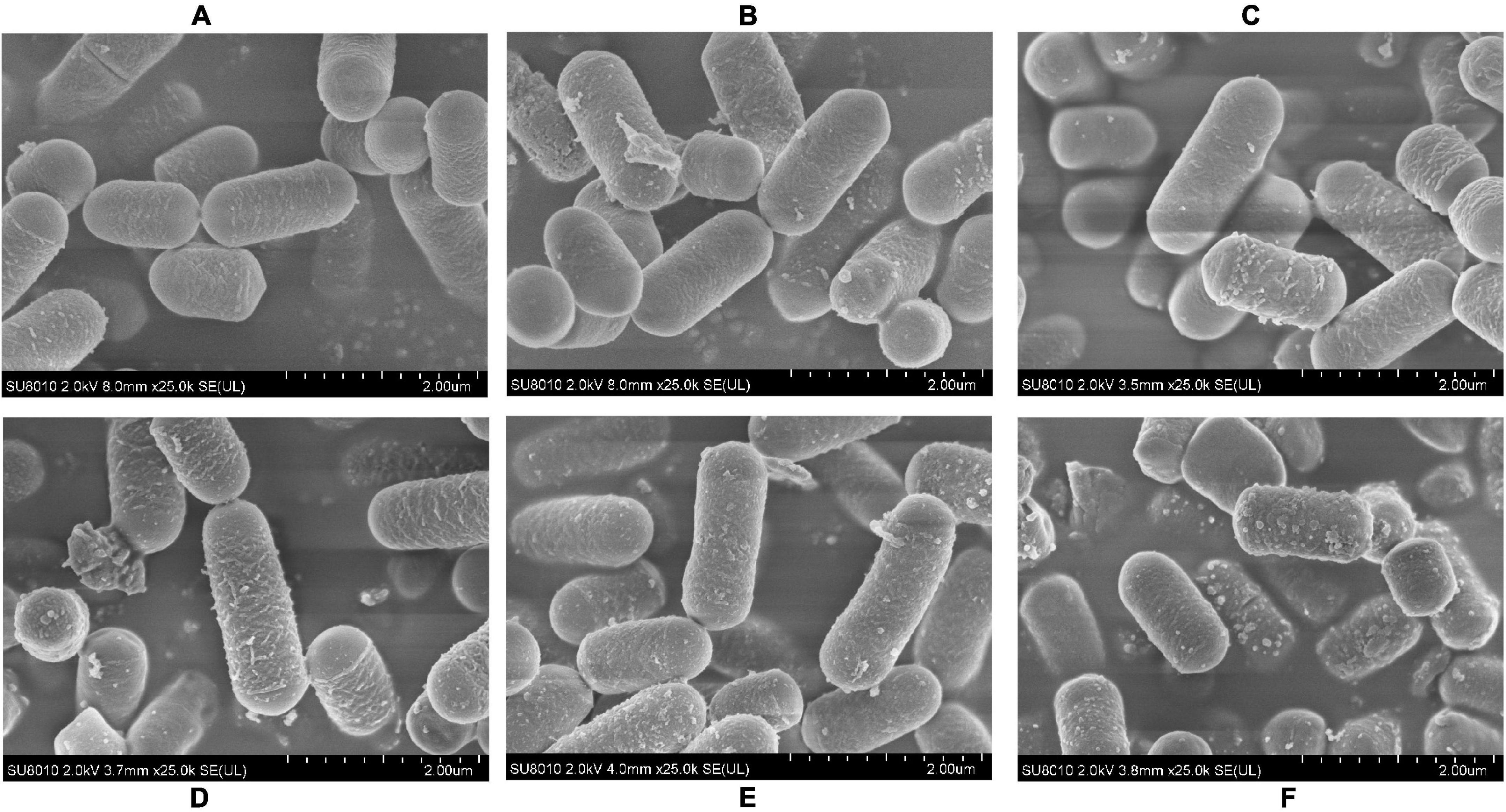
Figure 2. SEM images of L. plantarum RS-09 from control and the acid groups. (A) Control group; (B) pH 5.5 group; (C) pH 4.5 group; (D) pH 3.5 group; (E) pH 2.5 group; (F) pH 1.5 group.
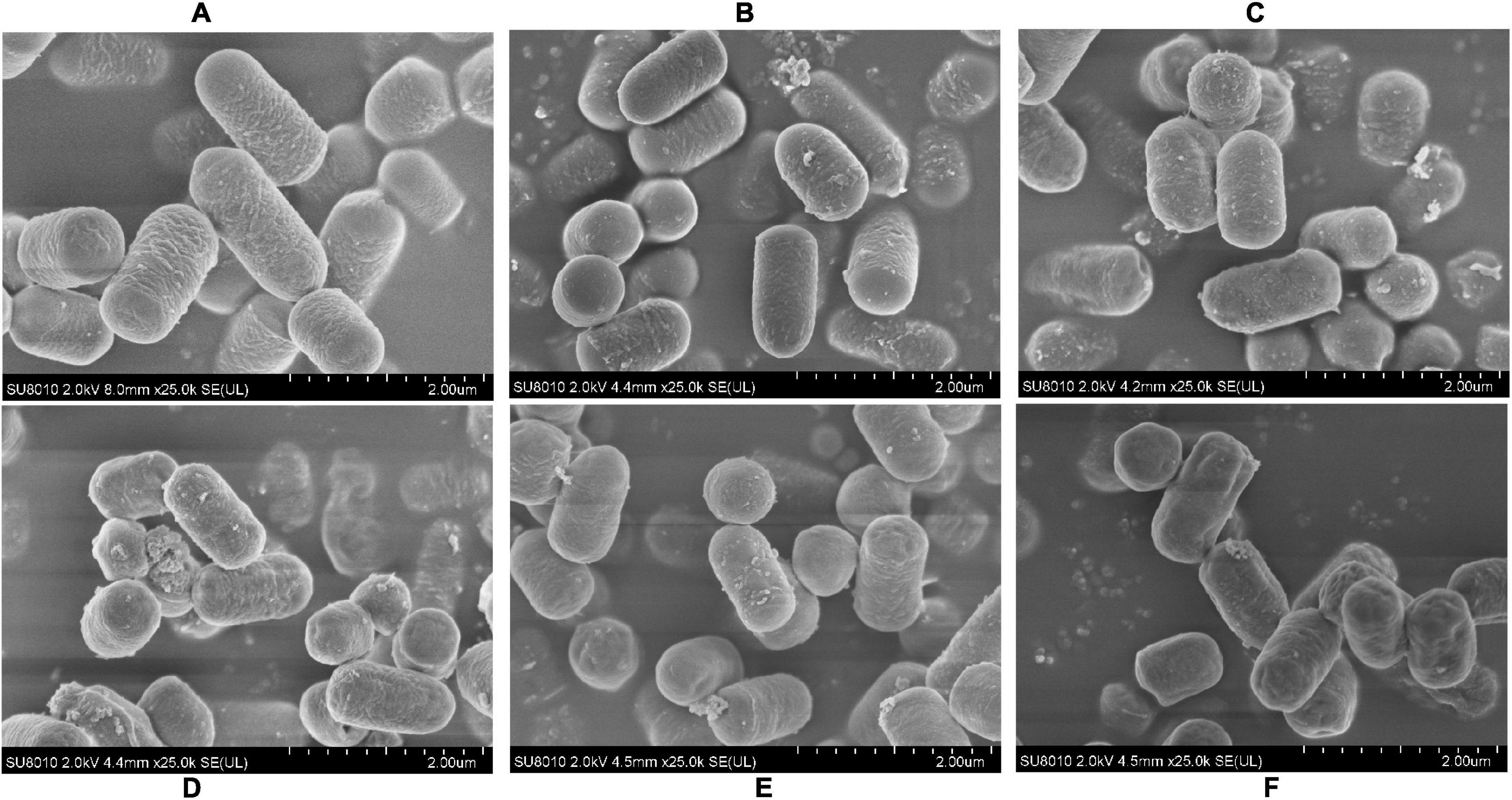
Figure 3. SEM images of L. plantarum RS-09 from control and bile salt groups. (A) Control group; (B) 0.3% bile salt; (C) 0.5% bile salt; (D) 1% bile salt; (E) 1.5% bile salt; (F) 2% bile salt.
CFDA/SE Can Efficiently Label L. plantarum RS-09
The CFDA-SE label was stable and has been described for various cells. To determine how about the CFDA-SE labeling stability, cells were cultured at low density and at indicated time points, analyzed by flow cytometry and fluorescence microscopy. With each cell division, the label was diluted, as it was divided between daughter cells. Initially, 99% of the cells were labeled (Figure 4). The intensity of CFDA-SE fluorescence was still high in each cell during 72 h. These results are consistent with those obtained by fluorescence microscopy, indicating that CFDA/SE can effectively label L. plantarum for long-term monitoring of these strains.
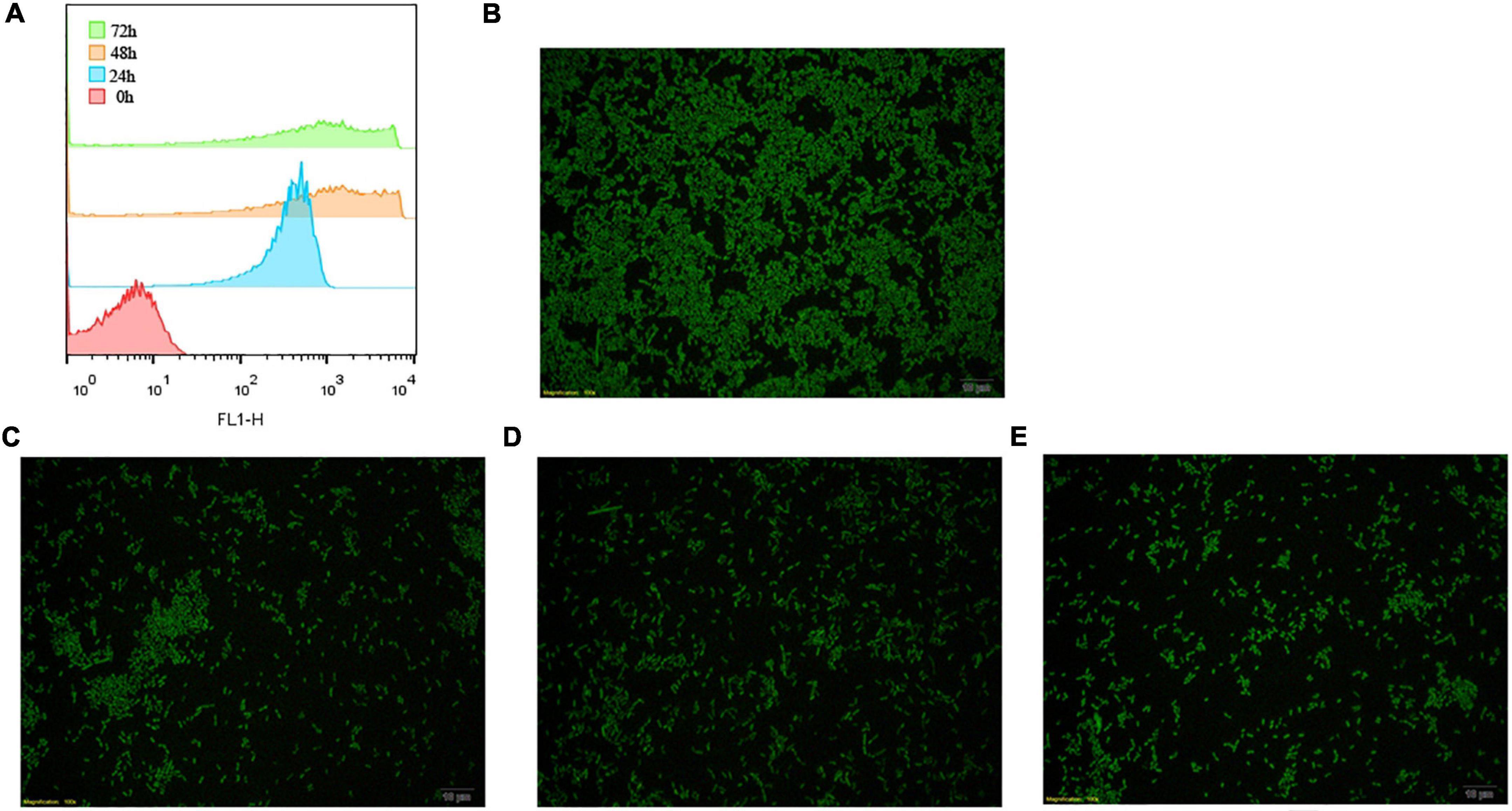
Figure 4. Fluorescent detection of labeled L. plantarum RS-09. (A) Flow cytometry results for the detection of fluorescently labeled RS-09; (B–E) CFDA/SE labeled L. plantarum cultured in MRS for 0, 24, 48, and 72 h investigated under fluorescence microscopy.
Lactiplantibacillus plantarum RS-09 Adhesion Ability
One of the important properties of lactobacilli is their ability to adhere to the target sites for their colonization in the gut for expressing optimal functionality. The ability of L. plantarum RS-09 to adhere to HT-29 cells is shown in Figure 5. Gram staining showed that L. plantarum RS-09 was highly adherent to the HT-29 cells (Figure 5). To further observe the ability of L. plantarum RS-09 to adhere to cells, the CFDA/SE-labeled strain was incubated with the cells for 2 h, followed by observation by fluorescence microscopy (Figure 5) and FCM analysis for bacterial count. In our study, adhesion of L. plantarum RS-09 on HT-29 cells was quantified (Table 4). The results showed that the adhesion rate of RS-09 to HT-29 cells was 7.85%, respectively. Medium with different pH conditions was used to observe the ability of the RS-09 strain to adhere to intestinal epithelial cells. The results showed that under strong acid and weak base conditions, the RS-09 stain still had a high rate of adhesion, where the adhesion rates were 7.73% at pH 2.5 and 5.01% at pH 8.5. The adhesion ability under acid resistance was stronger compared with the strains in the alkali stress conditions.
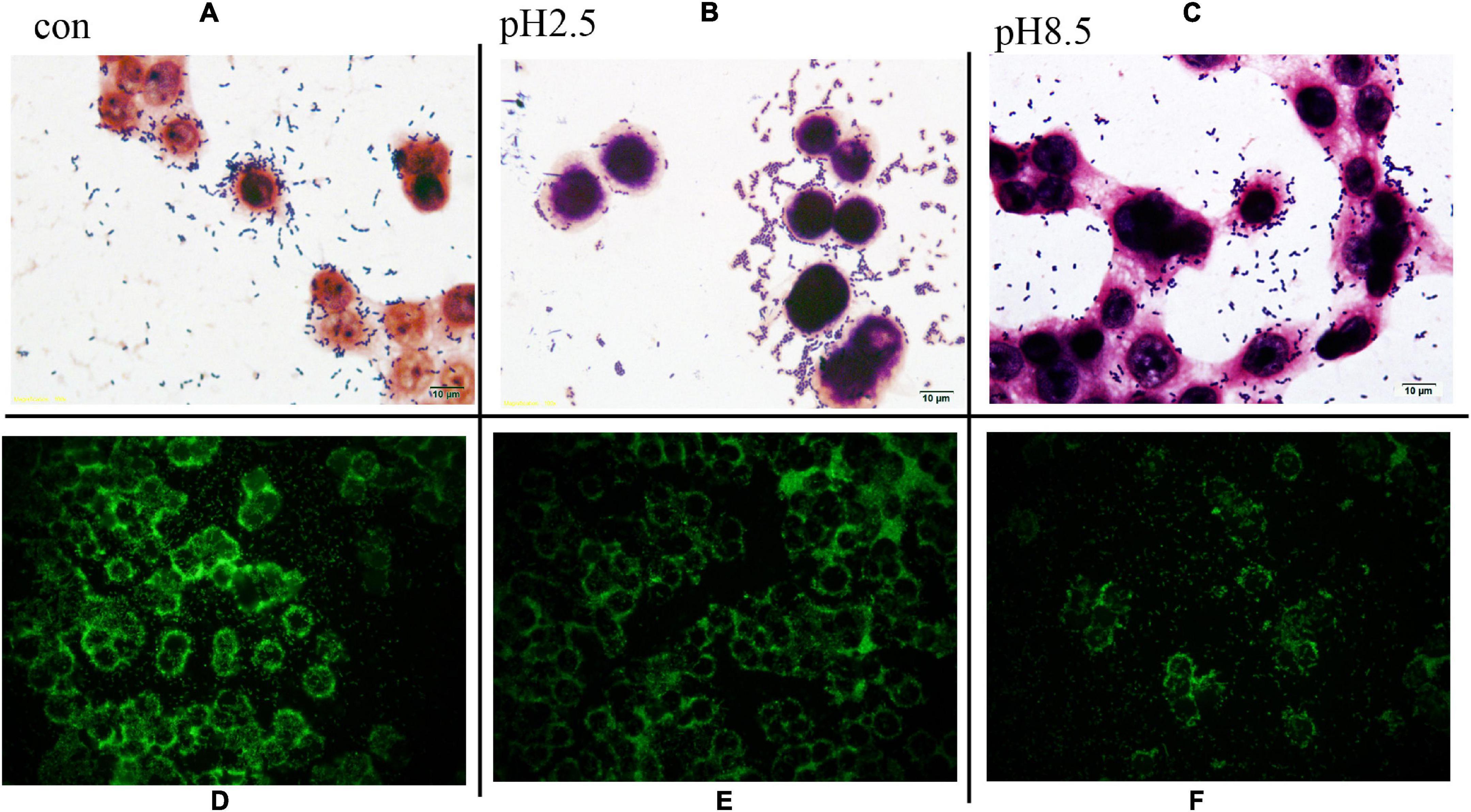
Figure 5. L. plantarum RS-09 adhesion assay on HT-29 cells under different pH stress. (A–C) Adhesion observation by Gram stain. Scale bars, 10 μm (insert, 1,000×). (D–F) Fluorescence microscopic to analyze L. plantarum RS-09 adhesion on HT-29 cells. In each case, same number of Lactobacillus cells (1 × 108 CFU/mL) were applied and treated equally. Scale bars, 20 μm (original magnification, 400×).
Using CFDA/SE-Labeled L. plantarum RS-09 to Study Persistence Patterns
To better confirm the adherence and persistence process of L. plantarum RS-09 in the gastrointestinal tract, CFDA/SE-labeled strains can be used for long-term monitoring due to their stable fluorescence. The intestinal contents were analyzed by FCM at 2, 8, 12, 24, 48, 72 and 96 h. The concentrations of L. plantarum in different segments of the intestine are shown in Figure 6. Two hours after oral administration, the labeled strain was detected in all intestinal contents, with a positive rate of over 60%. In the small intestine (duodenum, jejunum, and ileum), the concentration of the RS-09 bacteria decreased over time, reaching the lowest value at 48 h, with positive rate 3.98, 17.0, and 27.3% relatively. However, in the large intestine (cecum, colon and rectum), the fluorescence intensity first increased and then decreased, reaching the lowest value 48 h, with positive rate 42.33, 35.63, and 34.8% relatively. On 96 h, L. plantarum RS-09 was predominantly localized in the cecum, colon and rectum, with positive rate 69.4, 71.9, and 74.2% relatively.
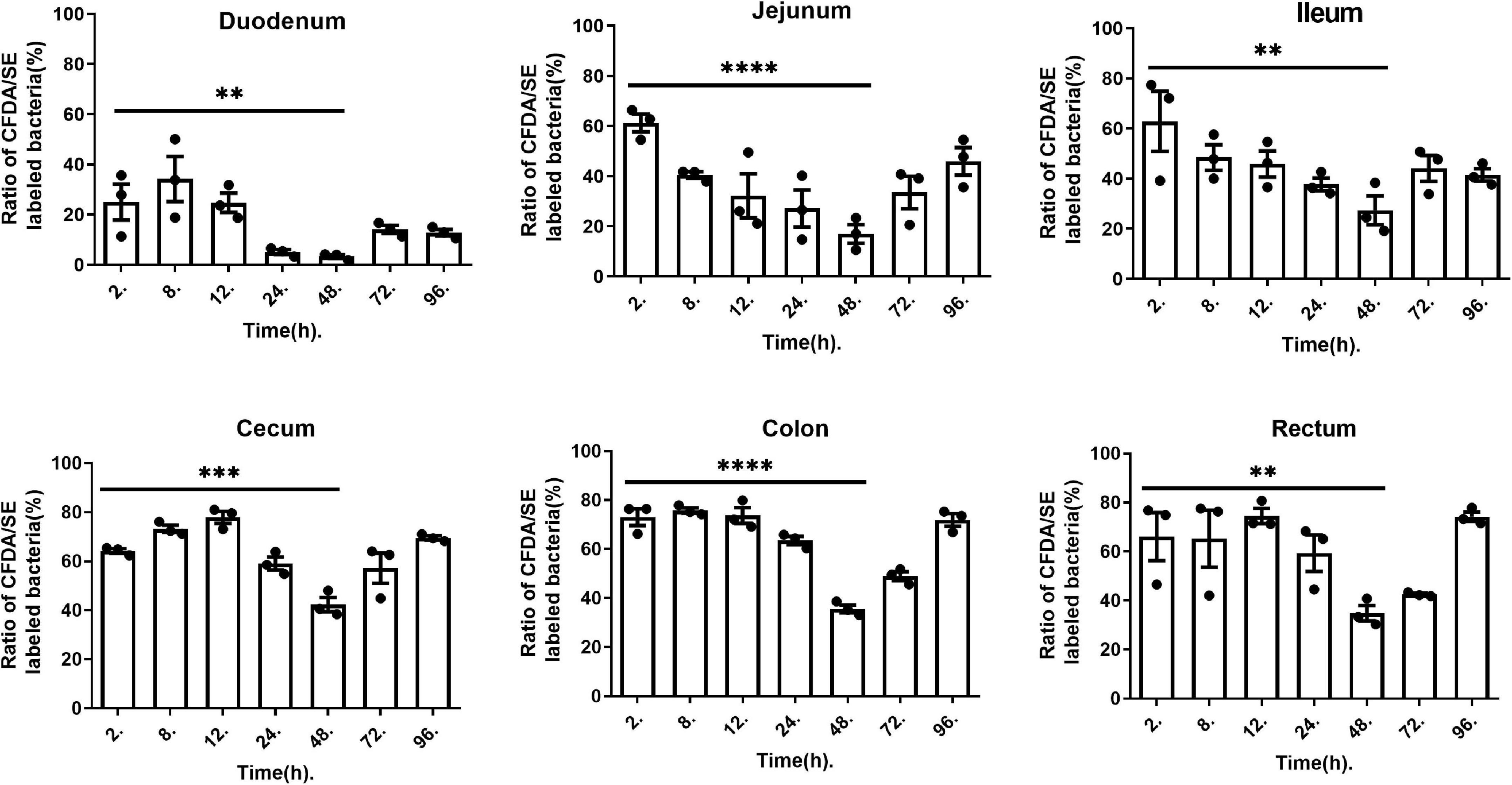
Figure 6. L. plantarum RS-09 fluorescence at different time points and measured in different organs. Data are presented as mean ± SEM. One-way ANOVA in SPSS Statistic. **p < 0.01, ***p < 0.001, and ****p < 0.0001.
To further analyze the persistence process and pattern of L. plantarum RS-09 in the intestinal tract, we monitored the strains for 20 days and established a kinetic model. FCM results showed changes of L. plantarum RS-09 persistence in various segments of the intestine. Figure 7 illustrates a 480-h curve about the bacterial intensity and distribution in the intestine. The curve was fitted using Fourier Series Models by Matlab. Fluorescence value correlated with the number of viable bacteria (CFU/0.1 g) isolated from different parts of the intestine at corresponding time points. The strongest correlation was calculated for cecum (R2 = 0.9722) and ileum (R2 = 0.8702), but lower for colon (R2 = 0.7505). Fourier model of L. plantarum RS-09 dynamics show that an obvious decrease on the number of RS-09 was found at 48 h in the intestine, and the change in bacterial concentration increased after 48 h. The fluorescent signal of L. plantarum RS-09 in cecum displayed levels similar to those observed in colon and ileum from day 14 until 20. After 20 days, RS-09 could colonize in the intestinal tract steadily.
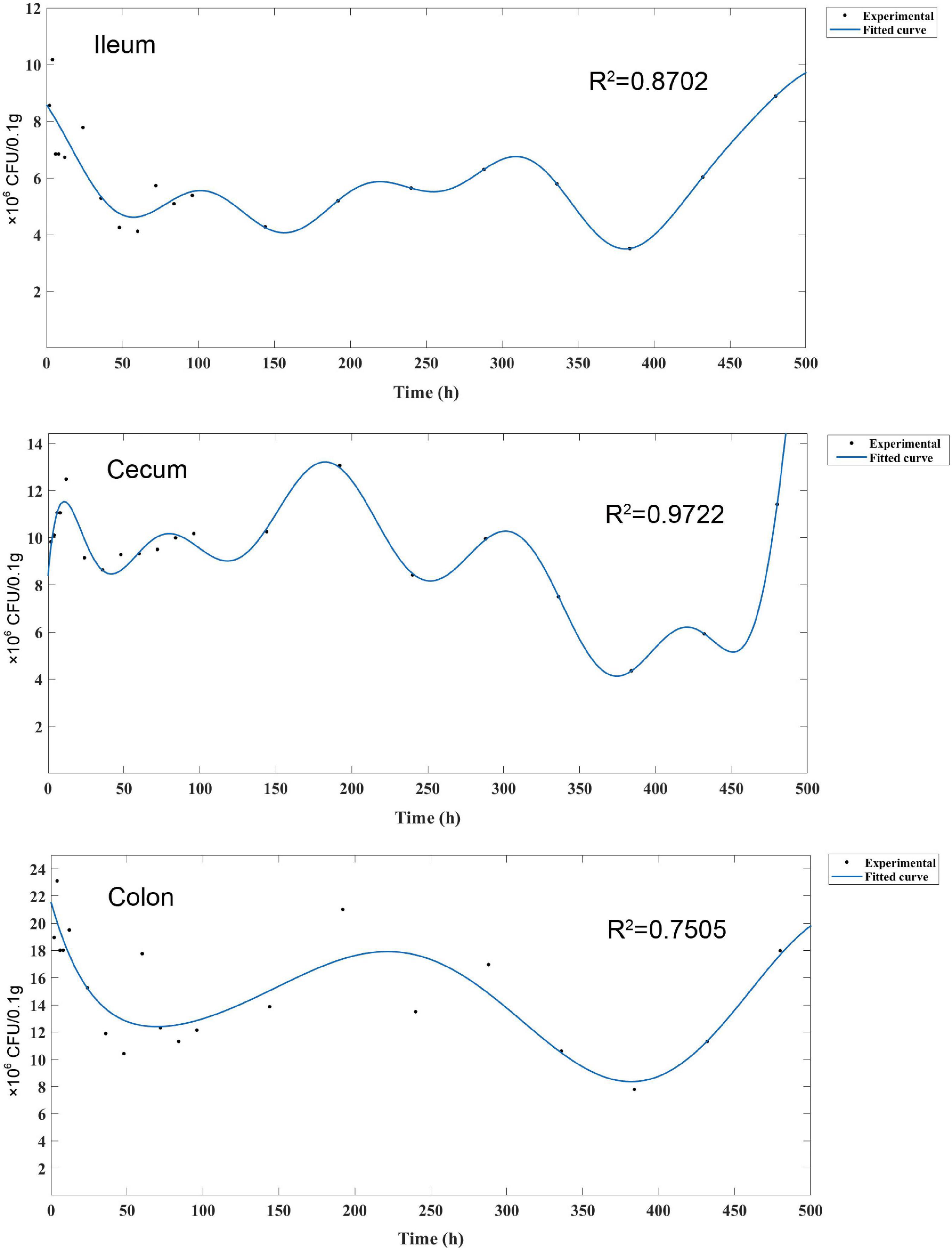
Figure 7. Fourier series model for experimental measured data. Fourier model of L. plantarum RS-09 dynamics show high harmonics. The measured Fourier transform is the average over Fourier transforms of bacterial count levels that were measured three times.
Monitoring L. plantarum RS-09 in the Gastrointestinal Tract of Mouse
At 96 h after oral administration, a fluorescence microscope was used to observe CFDA/SE-labeled L. plantarum RS-09 in the ileum, cecum, and colon, which are the sites with long persistence. The results showed that the fluorescently-labeled strain could be observed in the intestinal mucosa and submucosa (Figure 8). In the ileum, cecum and colon, the fluorescently-labeled strain was presented in the intestinal mucus and lumen and closely attached to the epithelial cells. In addition, the labeled bacteria were observed in the lamina propria and muscularis mucosae. These results were consistent with those of FCM.
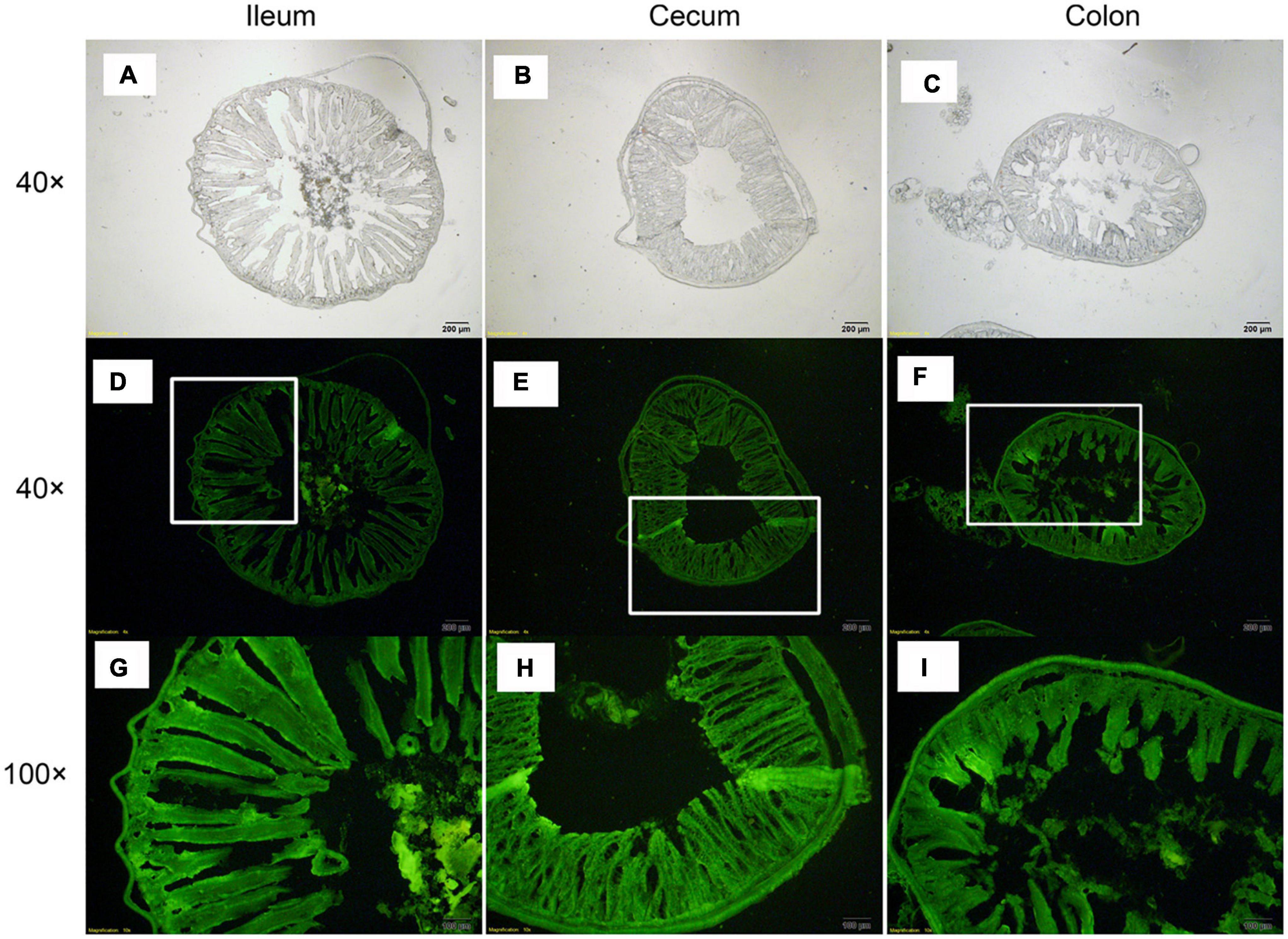
Figure 8. Distribution of L. plantarum RS-09 in frozen sections of intestinal tissue. (A,D,G) L. plantarum RS-09 in the ileum was assessed; (B,E,H) L. plantarum RS-09 in the cecum was assessed; (C,F,I) L. plantarum RS-09 in the colon was assessed. Scale bars, 200 μm (top; original magnification, ×40) and 100 μm (bottom; original magnification, ×100).
Discussion
In this study, we created a novel method for real-time quantitative analysis of the adhesion process of probiotics in the gastrointestinal tract. By using CFDA/SE-labeled L. plantarum RS-09, we analyzed the spatiotemporal persistence patterns of L. plantarum in the gastrointestinal tract to establish a kinetics model of bacterial strain growth. These studies provide new insights into the long-term persistence of L. plantarum in the gastrointestinal tract.
Probiotic strains tolerance to acidic environment and bile salts are two criteria for a candidate probiotic strain to survive the GIT (Nami et al., 2019). The strain of L. plantarum described in this study was isolated from fermented apple juice and appears to have strong resistance to acid and bile salts. Lactic acid bacteria isolated from fermented apple juice have been adapted to the harsh fermentation conditions and, therefore, it is easier for them to survive, adhere to, and colonize the gastrointestinal tract compared to other strains. Many studies have shown that the isolated lactic acid bacteria from plant fermentation products have strong probiotic functions (Caggia et al., 2015; Ferrari Ida et al., 2016). In addition, bile tolerance can contribute to the ability of lactobacilli to persist in the gastrointestinal tract (Spivey et al., 2014). Previous reports considered Lc. mesenteroides as a bad probiotic candidate because of its low colonization rates of the large intestine, probably due to poor tolerance to acid and bile salt (Barache et al., 2020). In our study, RS-09 showed acid tolerance when grown at pH 2.5 or 3.5 and did not encounter growth reduction in the presence of 0.3% bile salts. This augments the potential capability of the strain to survive similar harsh conditions found in the GIT.
To date, the methods for the determination of bacterial adherence, including microscopic counting, double-antibody labeling and enzyme activity detection, all have disadvantages (Falk et al., 1994). For instance, microscopic counting is a cumbersome and subjective method to quantify adherent bacteria. FCM with double-antibody labeling to detect bacterial adherence is susceptible to non-specific binding of primary and secondary antibodies, which affects the ability to accurately determine adherence. The FITC method has been used to label microorganisms for quantitative analysis, but the fluorescence intensity decreased within 6–12 h and the signal gradually faded with the growth of the bacteria because of the short half-life of FITC in living systems (Kasugai et al., 2000). This is not conducive to long-term observations. In our study, CFDA/SE was used to label L. plantarum RS-09. This is a method modified from the study by Hodgkin et al. (1996), in which they used CFDA/SE to label lymphocytes for detecting lymphocyte proliferation/differentiation or for in vivo tracking. Among fluorescent dyes, CFDA/SE dyes have many advantages such as strong fluorescence, low toxicity and good durability. In addition, FCM detection of CFDA/SE-labeled strains can quantify both the ratio of bacteria to cells and the number of bacteria adhering to each cell. The results of the L. plantarum RS-09 adherence rate can be analyzed by fluorescence microscopy and FCM. Our results indicate that using CFDA/SE to label RS-09 with the use of a fluorescence-activated cell sorter (FACS) to analyze the adherence of strains to epithelial cells is a sensitive and durable technique and provides a more stable fluorescent labeling model.
The tolerance to the gastrointestinal environment of Lactobacillus species is related with its adhesion to intestinal epithelial cells. Based on scanning electron microscope (SEM) analysis, the morphology of RS-09 became short under bile salt stress. This result was consistent with another study that L. plantarum ATCC14917 cells was long rods in the acid and control groups, but short rods in the alkali group (Wang et al., 2018). HT-29 cells are ideal models for studying bacterial adherence in vitro and the RS-09 strain was highly adherent to these cells. The results showed that the adherence of the RS-09 strain to the HT-29 cells was minimally affected by the pH of the testing conditions. This lays the foundation for the persistence of this strain in the gastrointestinal tract.
Studies have shown that the survival rate of bacteria in the gastrointestinal tract is crucial for adherence. Fewer bacteria are generally present in the duodenum because of the low pH in the stomach and the fluidity of the intestinal contents (Breeuwer et al., 1996). However, the persistence rate of L. plantarum RS-09 in the duodenum at 96 h was 12.8% in this study. Van Zyl et al. (2018) also revealed the presence of viable cells of L. plantarum 423 in the small intestine at 4 and 24 h post gavage by the CFU counts, but no bioluminescent signals could be detected. This might be caused by reduced cell activity or the inefficient bioluminescence emission during passage in the stomach and duodenum (Van Zyl et al., 2018). This indicates that the RS-09 strain has a strong resistance to acid and bile salts, allowing this strain to have a probiotic effect further in the intestinal tract after surviving the gastric acid environment. Meanwhile it implies that the fluorescent signal of CFDA/SE is stable under the low pH gastrointestinal environment. At 96 h, fluorescent cells of RS-09 were predominantly localized in the cecum and colon, which are consistent with L. plantarum 423.
Although we can use HT-29 cells to evaluate the in vitro performance of L. plantarum, the comments of Greene and Klaenhammer that an in vitro cell model has limitations in its ability to reflect in vivo features are true (Hertel et al., 1993). In previous studies, the adhesion and persistence of gastrointestinal probiotics in the body were analyzed by collecting stool samples and performing plate counting to determine the number of colonizing bacteria. However, this method does not truly reflect the persistence in the intestine, and it is impossible to analyze the regional and temporal characteristics of probiotic persistence. In this study, a CFDA/SE-labeled strain administered to mice was used to quantify the strain in the intestinal tract via a real-time analysis of the intestinal contents by FCM. Earlier reports also have shown that FITC can label the bacterial strain and monitor the distribution of Lactobacillus kefiranofaciens ZW3 in the ileum and colon by detecting contents (Xing et al., 2018). FITC fluorescence imaging measured only the labeled cells, but not the overall level of colonization. While CFDA-SE is an effective and popular dye to monitor cells division. When a CFDA-SE -labeled cell divides, its progeny is endowed with half the number of carboxyfluorescein-tagged molecules.
Our study showed that the cecum is the segment with highest number of viable bacteria over time. The murine cecum may be the site where microorganisms adapt to the gastrointestinal environment and where the activation of genes required for colonization of the colon occur (Cronin et al., 2008). In addition, during long-term monitoring, we noticed that the fluorescence intensity of the RS-09 strain was lowest in all segments of the intestine 48 h after administration, after which it rebounded. This finding is consistent with that of the study by Han et al. (2015), in which the FCM detection rate of GFP-tagged L. plantarum reached the lowest value in the duodenum and ileum of goats after 42 h and then started to increase by 72 h. The specific mechanism is still unclear. After oral administration of high-dose probiotics, it may take 48 h for the bacteria to adapt to the gastrointestinal environment. In addition, the persistence patterns of L. plantarum RS-09 in the small intestine (duodenum, jejunum and ileum) and the large intestine (cecum, colon and rectum) were different. This result was consistent with another study that treated mice with the probiotic Lacticaseibacillus rhamnosus, where the number of colonization strains increased from the proximal to the distal small intestine (duodenum < jejunum < ileum) (Li et al., 2019). The persistence rate of L. plantarum in the large intestine was higher than that in the small intestine. This may be related to the structure of the intestine. The small intestine is slender, long, and impacted by bile, pancreatic juices, and various digestive enzymes. Thus, the persistence rate of probiotics is lower in the small intestine than in the large intestine.
Tissue sections were observed under a microscope to assess the specific contact sites between the bacteria, the submucosal cells, and cells from the muscularis mucosae (Vinderola et al., 2004). Considering the complex microbial environment in the stomach and intestines, we are unable to fully understand the fate of lactobacilli. Also, CFDA-SE-stain is suitable for cryosectioning applications and can withstand the processing with snap freezing and sectioning (Lönnqvist et al., 2019). Therefore, the fluorescence microscopy findings in the frozen sections will help us to understand the specific persistence sites and modes of action of microorganisms in vivo. Overall, the gastrointestinal persistence of L. plantarum RS-09 compared well to several other commercial probiotic strains, including L. plantarum 299v, L. gasseri SBT2055 and L. rhamnosus GG.
Conclusion
This study was carried out mainly to elucidate the in vitro and in vivo patterns of adherence and persistence of L. plantarum RS-09. We used, for the first time, bacterial CFDA/SE labeling technology to analyze the persistence of probiotics in a long- term in vivo tracking experiment. This model can be used to monitor the survival of strains in vivo and to further study the interactions between microorganisms and hosts by means of this FCM technique.
Data Availability Statement
The original contributions presented in the study are included in the article/supplementary material, further inquiries can be directed to the corresponding author/s.
Ethics Statement
The animal study was reviewed and approved by the Laboratory Animal Welfare and Ethics Committee of Ludong University.
Author Contributions
XyZ and CZ designed the experiments. LY, JZ, XY, and GC carried out the experiments. HZ, WT, and YL analyzed the experimental results. MW and HJ wrote the manuscript. LJ and XxZ reviewed and edited the manuscript. All authors contributed to the article and approved the submitted version.
Funding
This research was financially by the Natural Science Foundation of Shandong Province of China (No. ZR2020QC227), the Key Research and Development Plan of Yantai (Nos. 2020XDRH101, 2021YT06000060, and 2021XDHZ076), and the Innovation Team Project for Modern Agricultural Industrious Technology System of Shandong Province (SDAIT-11-10).
Conflict of Interest
The authors declare that the research was conducted in the absence of any commercial or financial relationships that could be construed as a potential conflict of interest.
Publisher’s Note
All claims expressed in this article are solely those of the authors and do not necessarily represent those of their affiliated organizations, or those of the publisher, the editors and the reviewers. Any product that may be evaluated in this article, or claim that may be made by its manufacturer, is not guaranteed or endorsed by the publisher.
References
Barache, N., Ladjouzi, R., Belguesmia, Y., Bendali, F., and Drider, D. (2020). Abundance of Lactobacillus plantarum Strains with Beneficial Attributes in Blackberries (Rubus sp.), Fresh Figs (Ficus carica), and Prickly Pears (Opuntia ficus-indica) Grown and Harvested in Algeria. Probiot. Antimicrob. Proteins 12, 1514–1523. doi: 10.1007/s12602-020-09632-z
Breeuwer, P., Drocourt, J., Rombouts, F. M., and Abee, T. (1996). A Novel Method for Continuous Determination of the Intracellular pH in Bacteria with the Internally Conjugated Fluorescent Probe 5 (and 6-)-Carboxyfluorescein Succinimidyl Ester. Appl. Environ. Microbiol. 62, 178–183. doi: 10.1128/aem.62.1.178-183.1996
Caggia, C., De Angelis, M., Pitino, I., Pino, A., and Randazzo, C. L. (2015). Probiotic features of Lactobacillus strains isolated from Ragusano and Pecorino Siciliano cheeses. Food Microbiol. 50, 109–117. doi: 10.1016/j.fm.2015.03.010
Chua, J. C. L., Hale, J. D. F., Silcock, P., and Bremer, P. J. (2020). Bacterial survival and adhesion for formulating new oral probiotic foods. Crit. Rev. Food Sci. Nutr. 60, 2926–2937. doi: 10.1080/10408398.2019.1669528
Cong, L., Sun, D., Zhang, Z., Jiao, W., Rizzolo, L. J., and Peng, S. (2008). A novel rabbit model for studying RPE transplantation. Invest Ophthalmol. Vis. Sci. 49, 4115–4125. doi: 10.1167/iovs.08-1976
Cronin, M., Sleator, R. D., Hill, C., Fitzgerald, G. F., and Van Sinderen, D. (2008). Development of a luciferase-based reporter system to monitor Bifidobacterium breve UCC2003 persistence in mice. BMC Microbiol. 8:161. doi: 10.1186/1471-2180-8-161
Damaceno, Q. S., Gallotti, B., Reis, I. M. M., Totte, Y. C. P., Assis, G. B., Figueiredo, H. C., et al. (2021). Isolation and Identification of Potential Probiotic Bacteria from Human Milk. Probiot. Antimicrob. Proteins [Epub online ahead of print]. doi: 10.1007/s12602-021-09866-5
Falk, P., Boren, T., Haslam, D., and Caparon, M. (1994). Bacterial adhesion and colonization assays. Methods Cell Biol. 45, 165–192. doi: 10.1016/s0091-679x(08)61851-8
Ferrari Ida, S., De Souza, J. V., Ramos, C. L., Da Costa, M. M., Schwan, R. F., and Dias, F. S. (2016). Selection of autochthonous lactic acid bacteria from goat dairies and their addition to evaluate the inhibition of Salmonella typhi in artisanal cheese. Food Microbiol. 60, 29–38. doi: 10.1016/j.fm.2016.06.014
Fidanza, M., Panigrahi, P., and Kollmann, T. R. (2021). Lactiplantibacillus plantarum-Nomad and Ideal Probiotic. Front. Microbiol. 12:712236. doi: 10.3389/fmicb.2021.712236
Fuller, M. E., Streger, S. H., Rothmel, R. K., Mailloux, B. J., Hall, J. A., Onstott, T. C., et al. (2000). Development of a vital fluorescent staining method for monitoring bacterial transport in subsurface environments. Appl. Environ. Microbiol. 66, 4486–4496. doi: 10.1128/AEM.66.10.4486-4496.2000
Garcia-Gonzalez, N., Battista, N., Prete, R., and Corsetti, A. (2021). Health-Promoting Role of Lactiplantibacillus plantarum Isolated from Fermented Foods. Microorganisms 9:349. doi: 10.3390/microorganisms9020349
Goel, A., Halami, P. M., and Tamang, J. P. (2020). Genome Analysis of Lactobacillus plantarum Isolated From Some Indian Fermented Foods for Bacteriocin Production and Probiotic Marker Genes. Front. Microbiol. 11:40. doi: 10.3389/fmicb.2020.00040
Han, X., Wang, L., Li, W., Li, B., Yang, Y., Yan, H., et al. (2015). Use of green fluorescent protein to monitor Lactobacillus plantarum in the gastrointestinal tract of goats. Braz. J. Microbiol. 46, 849–854. doi: 10.1590/s1517-838246320140556
Hardy, H., Harris, J., Lyon, E., Beal, J., and Foey, A. D. (2013). Probiotics, prebiotics and immunomodulation of gut mucosal defences: homeostasis and immunopathology. Nutrients 5, 1869–1912. doi: 10.3390/nu5061869
Hertel, C., Ludwig, W., Pot, B., Kersters, K., and Schleifer, K.-H. (1993). Differentiation of Lactobacilli Occurring in Fermented Milk Products by Using Oligonucleotide Probes and Electrophoretic Protein Profiles. Syst. Appl. Microbiol. 16, 463–467. doi: 10.1016/S0723-2020(11)80281-X
Hodgkin, P. D., Lee, J. H., and Lyons, A. B. (1996). B cell differentiation and isotype switching is related to division cycle number. J. Exp. Med. 184, 277–281. doi: 10.1084/jem.184.1.277
Hussein, W. E., Abdelhamid, A. G., Rocha-Mendoza, D., Garcia-Cano, I., and Yousef, A. E. (2020). Assessment of Safety and Probiotic Traits of Enterococcus durans OSY-EGY, Isolated From Egyptian Artisanal Cheese, Using Comparative Genomics and Phenotypic Analyses. Front. Microbiol. 11:608314. doi: 10.3389/fmicb.2020.608314
Kasugai, S., Fujisawa, R., Waki, Y., Miyamoto, K., and Ohya, K. (2000). Selective drug delivery system to bone: small peptide (Asp)6 conjugation. J. Bone Miner Res. 15, 936–943. doi: 10.1359/jbmr.2000.15.5.936
Li, C., Bei, T., Niu, Z., Guo, X., Wang, M., Lu, H., et al. (2019). Adhesion and Colonization of the Probiotic Lactobacillus rhamnosus Labeled by Dsred2 in Mouse Gut. Curr. Microbiol. 76, 896–903. doi: 10.1007/s00284-019-01706-8
Li, P., Gu, Q., and Zhou, Q. (2016). Complete genome sequence of Lactobacillus plantarum LZ206, a potential probiotic strain with antimicrobial activity against food-borne pathogenic microorganisms. J. Biotechnol. 238, 52–55. doi: 10.1016/j.jbiotec.2016.09.012
Lim, J. H., Yoon, S. M., Tan, P. L., Yang, S., Kim, S. H., and Park, H. J. (2018). Probiotic Properties of Lactobacillus plantarum LRCC5193, a Plant-Origin Lactic Acid Bacterium Isolated from Kimchi and Its Use in Chocolates. J. Food Sci. 83, 2802–2811. doi: 10.1111/1750-3841.14364
Lönnqvist, S., Junker, J. P. E., Sedell, M., Nyman, E., and Kratz, G. (2019). Tracking keratinocytes and melanocytes using carboxyfluorescein hydroxysuccinimidyl ester staining. PLoS One 14:e0221878. doi: 10.1371/journal.pone.0221878
Monteagudo-Mera, A., Rastall, R. A., Gibson, G. R., Charalampopoulos, D., and Chatzifragkou, A. (2019). Adhesion mechanisms mediated by probiotics and prebiotics and their potential impact on human health. Appl. Microbiol. Biotechnol. 103, 6463–6472. doi: 10.1007/s00253-019-09978-7
Nami, Y., Vaseghi Bakhshayesh, R., Mohammadzadeh Jalaly, H., Lotfi, H., Eslami, S., and Hejazi, M. A. (2019). Probiotic Properties of Enterococcus Isolated From Artisanal Dairy Products. Front. Microbiol. 10:300. doi: 10.3389/fmicb.2019.00300
Papadimitriou, K., Zoumpopoulou, G., Foligne, B., Alexandraki, V., Kazou, M., Pot, B., et al. (2015). Discovering probiotic microorganisms: in vitro, in vivo, genetic and omics approaches. Front. Microbiol. 6:58. doi: 10.3389/fmicb.2015.00058
Pence, M. A., and Liesman, R. (2020). “Chapter 55 - Clinical microbiology,” in Contemporary Practice in Clinical Chemistry (Fourth Edition), eds W. Clarke and M. A. Marzinke (Cambridge: Academic Press), 985–1006.
Prins, W. A., Botha, M., Botes, M., De Kwaadsteniet, M., Endo, A., and Dicks, L. M. (2010). Lactobacillus plantarum 24, isolated from the marula fruit (Sclerocarya birrea), has probiotic properties and harbors genes encoding the production of three bacteriocins. Curr. Microbiol. 61, 584–589. doi: 10.1007/s00284-010-9656-8
Pumriw, S., Luang-In, V., and Samappito, W. (2021). Screening of Probiotic Lactic Acid Bacteria Isolated from Fermented Pak-Sian for Use as a Starter Culture. Curr. Microbiol. 78, 2695–2707. doi: 10.1007/s00284-021-02521-w
Sauvageau, J., Ryan, J., Lagutin, K., Sims, I. M., Stocker, B. L., and Timmer, M. S. (2012). Isolation and structural characterisation of the major glycolipids from Lactobacillus plantarum. Carbohydr. Res. 357, 151–156. doi: 10.1016/j.carres.2012.05.011
Seddik, H. A., Bendali, F., Gancel, F., Fliss, I., Spano, G., and Drider, D. (2017). Lactobacillus plantarum and Its Probiotic and Food Potentialities. Probiot. Antimicrob. Proteins 9, 111–122. doi: 10.1007/s12602-017-9264-z
Spivey, M. A., Dunn-Horrocks, S. L., and Duong, T. (2014). Epithelial cell adhesion and gastrointestinal colonization of Lactobacillus in poultry. Poult. Sci. 93, 2910–2919. doi: 10.3382/ps.2014-04076
Tarrah, A., Dos Santos, Cruz, B. C., Sousa Dias, R., Da Silva, Duarte, V., et al. (2021). Lactobacillus paracasei DTA81, a cholesterol-lowering strain having immunomodulatory activity, reveals gut microbiota regulation capability in BALB/c mice receiving high-fat diet. J. Appl. Microbiol. 131, 1942–1957. doi: 10.1111/jam.15058
Trukhachev, V. I., Chmykhalo, V. K., Belanova, A. A., Beseda, D. K., Chikindas, M. L., Bren, A. B., et al. (2021). Probiotic biomarkers and models upside down: From humans to animals. Vet. Microbiol. 261:109156. doi: 10.1016/j.vetmic.2021.109156
Van Zyl, W. F., Deane, S. M., and Dicks, L. M. T. (2018). In vivo bioluminescence imaging of the spatial and temporal colonization of Lactobacillus plantarum 423 and enterococcus mundtii ST4SA in the intestinal tract of mice. BMC Microbiol. 18:171. doi: 10.1186/s12866-018-1315-4
Vinderola, C. G., Medici, M., and Perdigón, G. (2004). Relationship between interaction sites in the gut, hydrophobicity, mucosal immunomodulating capacities and cell wall protein profiles in indigenous and exogenous bacteria. J. Appl. Microbiol. 96, 230–243. doi: 10.1046/j.1365-2672.2004.02158.x
Wang, W., He, J., Pan, D., Wu, Z., Guo, Y., Zeng, X., et al. (2018). Metabolomics analysis of Lactobacillus plantarum ATCC 14917 adhesion activity under initial acid and alkali stress. PLoS One 13:e0196231. doi: 10.1371/journal.pone.0196231
Xing, Z., Tang, W., Yang, Y., Geng, W., Rehman, R. U., and Wang, Y. (2018). Colonization and Gut Flora Modulation of Lactobacillus kefiranofaciens ZW3 in the Intestinal Tract of Mice. Probiot. Antimicrob. Proteins 10, 374–382. doi: 10.1007/s12602-017-9288-4
Zheng, J., Wittouck, S., Salvetti, E., Franz, C., Harris, H. M. B., Mattarelli, P., et al. (2020). A taxonomic note on the genus Lactobacillus: Description of 23 novel genera, emended description of the genus Lactobacillus Beijerinck 1901, and union of Lactobacillaceae and Leuconostocaceae. Int. J. Syst. Evol. Microbiol. 70, 2782–2858. doi: 10.1099/ijsem.0.004107
Keywords: Lactiplantibacillus plantarum, adherence, persistence, CFDA/SE, colonization
Citation: Zhao X, Zhao C, Yang L, Jiang L, Zhang J, Yu X, Chen G, Zhu H, Tang W, Li Y, Wei M, Zhang X and Jia H (2022) Spatial and Temporal Persistence of Fluorescent Lactiplantibacillus plantarum RS-09 in Intestinal Tract. Front. Microbiol. 13:843650. doi: 10.3389/fmicb.2022.843650
Received: 26 December 2021; Accepted: 07 March 2022;
Published: 30 March 2022.
Edited by:
Giovanna Suzzi, University of Teramo, ItalyReviewed by:
Guangqiang Wang, University of Shanghai for Science and Technology, ChinaHuaxi Yi, Ocean University of China, China
Copyright © 2022 Zhao, Zhao, Yang, Jiang, Zhang, Yu, Chen, Zhu, Tang, Li, Wei, Zhang and Jia. This is an open-access article distributed under the terms of the Creative Commons Attribution License (CC BY). The use, distribution or reproduction in other forums is permitted, provided the original author(s) and the copyright owner(s) are credited and that the original publication in this journal is cited, in accordance with accepted academic practice. No use, distribution or reproduction is permitted which does not comply with these terms.
*Correspondence: Linlin Jiang, bGlubGluamlhbmcxOTg2QDE2My5jb20=; Xingxiao Zhang, emhhbmd4aW5neGlhbzIwMTdAMTYzLmNvbQ==; Hong Jia, aG9uZ2ppYTIwMjExMkAxNjMuY29t
†These authors have contributed equally to this work and share first authorship