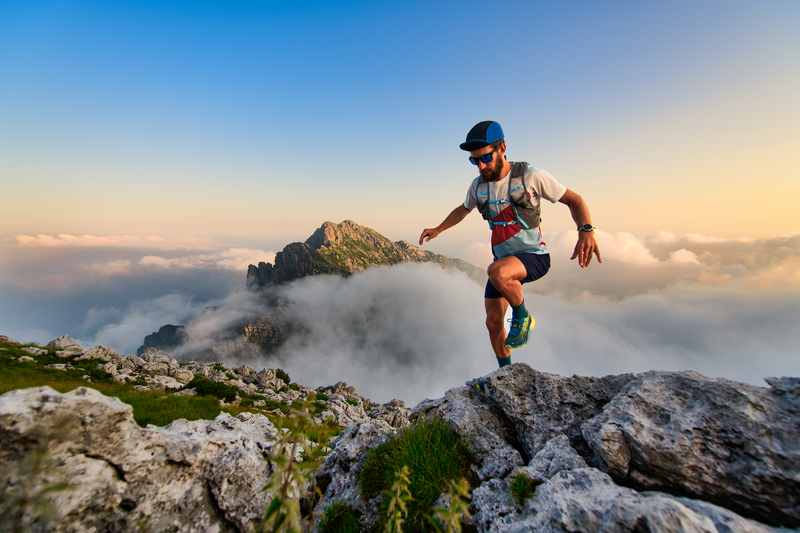
94% of researchers rate our articles as excellent or good
Learn more about the work of our research integrity team to safeguard the quality of each article we publish.
Find out more
ORIGINAL RESEARCH article
Front. Microbiol. , 23 March 2022
Sec. Systems Microbiology
Volume 13 - 2022 | https://doi.org/10.3389/fmicb.2022.835227
The Beet armyworm Spodoptera exigua (Lepidoptera: Noctuidae, Spodoptera) is an important global polyphagous pest. Pathogen infection could destroy the intestinal microbial homeostasis of insects, leading to the death of the host. However, the effect of the host intestinal microbial community on the insecticidal effect of Bacillus thuringiensis is rarely studied. In this study, the genome characteristics of Bt GS57 and the diversity and functions of the gut bacteria in S. exigua are investigated using crystal morphology, biological activity, and Illumina HiSeq high-throughput sequencing. The total size of the Bt GS57 genome is 6.17 Mbp with an average G + C content of 35.66%. Furthermore, the Bt GS57 genome contains six cry genes: cry1Ca, cry1Da, cry2Ab, cry9Ea, cry1Ia, and cry1Aa, and a vegetative insecticidal protein gene vip3Aa. The Bt GS57 strain can produce biconical crystals, mainly expressing 70 kDa and 130 kDa crystal proteins. The LC50 value of the Bt GS57 strain against the S. exigua larvae was 0.339 mg mL–1. Physiological and biochemical reactions showed that Bt GS57 belongs to B.t. var. thuringiensis. In addition, we found that B. thuringiensis can cause a dynamic change in the gut microbiota of S. exigua, with a significant reduction in bacterial diversity and a substantial increase in bacterial load. In turn, loss of gut microbiota significantly decreased the B. thuringiensis susceptibility of S. exigua larvae. Our findings reveal the vital contribution of the gut microbiota in B. thuringiensis-killing activity, providing new insights into the mechanisms of B. thuringiensis pathogenesis in insects.
Bacillus thuringiensis is a rod-shaped and Gram-positive bacterium, which produces a number of parasporal crystal proteins. B. thuringiensis shows different insecticidal activities against insect larvae without toxicity to animals (Nair et al., 2018). Therefore, it has been the most widely used biopesticide for several decades, owing to its toxicity toward a broad range of insect pests, such as Helicoverpa armigera, Spodoptera exigua, and Plutella xylostella (Lone et al., 2017; Su et al., 2017; Baragamaarachchi et al., 2019). Extensive planting of genetically engineered plants to produce Cry toxins has inhibited some major pests, reduced spraying of pesticides, enhanced natural enemy control of pests, and increased planting profit. However, these benefits have been eroded by the development of pest resistance (Gassmann, 2021). This resistance is a significant threat to the sustainability of B. thuringiensis biopesticide, thus reinforcing the need to find new B. thuringiensis strains and search for insect resistance mechanisms.
There are many B. thuringiensis genomes reported due to the rapid development of high-throughput sequencing technology; for instance, the B. thuringiensis Al Hakam, B. thuringiensis YBT-1520, and B. thuringiensis HD73 (Challacombe et al., 2007; Liu et al., 2013; Zhu et al., 2015). Previous reports showed that B. thuringiensis strains have a genome size of 2.4–6.1 Mb and GC content of 32–36% (Carlson and Kolsto, 1993; Li et al., 2015; Wang et al., 2016; Cao et al., 2018). Illumina sequencing is characterized by high-throughput sequencing technology, with the ability to identify insecticidal genes quickly in combination with bioinformatics analysis (Qin et al., 2011), thus providing resources for transgenic and biopesticide research.
Insect gut microbiota has various functions, playing a crucial role in host physiology, especially in the development, metabolism, reproduction, maintenance of the insect immune system, and other life activities (Jones et al., 2013; Ramya et al., 2016; Douglas, 2018). Previous studies found that Enterococcus is beneficial to Spodoptera littoralis by protecting the insect host from pathogenic microorganisms toxins (Shao et al., 2017). Meanwhile, the genus Bacillus has been shown to help insects digest and absorb nutrients by producing lipases, amylases, and proteases (Ramya et al., 2016; Regode et al., 2016; Nguyen et al., 2018). Overall, gut microbiota in insects can affect the reproduction of pathogens and the development of insecticide resistance, which has become the focus of many insect gut microbiology studies. Hilbeck et al. (2018) found that gut bacteria can enhance the insecticidal activity of the Cry toxin protein by causing bacterial septicemia in P. xylostella. Li et al. (2021) found that Bt Cry1Ac toxin interacts with the gut bacteria of P. xylostella to accelerate larval death. Moreover, a pathogenic insect fungus called Beauveria bassiana has been shown to interact with the gut microbiota of mosquitoes, thereby accelerating mosquito mortality (Wei et al., 2017). Therefore, understanding the host gut microbial composition is essential for developing effective entomopathogen-based biological pesticides to manage pests in different environments.
Unlike fungi that cause host death mainly by penetrating the host integument and proliferating in the hemolymph, B. thuringiensis primarily interacts with protein receptors on the surface of insect gut cells through toxins, resulting in the formation of pores in the cell membrane and cell death (Mason et al., 2011; Lei et al., 2021). However, the interaction between the intestinal microbiota and the insect biocontrol bacteria B. thuringiensis remains unexplored. Prominent questions include: Can the intestinal microbiota protect the S. exigua from B. thuringiensis infection? Do gut microbes and B. thuringiensis interact, or do they function independently? When B. thuringiensis infects insects, does the intestinal microbiota become virulent? Understanding the tripartite interaction between S. exigua, gut bacteria, and B. thuringiensis biocontrol bacteria may provide new insights into the biocontrol bacteria-insect interactions. In addition, they may help develop new insect control strategies and disease intervention measures.
Spodoptera exigua larvae were reared on an artificial diet under a controlled temperature of 27 ± 1°C, a photoperiod of 16-h light and 8-h dark, and relative humidity of 75 ± 10%, without exposure to any B. thuringiensis toxin. The rearing conditions of axenic S. exigua were the same as described previously (Broderick et al., 2006), except that the neonates were fed with a mixture of antibiotics (rifampicin, penicillin, streptomycin, and gentamicin) at a final concentration of 500 μg mL–1 until the second day of the 4th instar larvae.
The Bt GS57 strain was isolated from soil samples collected in Baoding, Hebei province, China, and identified with high toxicity against S. exigua. The Bt GS57 strain was inoculated in 1/2 LB medium and cultured at 30°C for 46 h until 70–90% of cell crystals were separated. Then, the spore crystal mixture was harvested and stored at −80°C. The spore crystal mixture was diluted to various concentrations (0.05, 0.1, 0.2, 0.4, 0.8, 1.6 mg mL–1) in sterile water and overlaid a 33 mm diameter plastic tube containing artificial diet. The control group was added the sterile water. After drying, the neonates of S. exigua, which had been starved for 2 h before the exposure to B. thuringiensis, were put into the plastic tube and reared under standard culture conditions (Zhang et al., 2017). Bioassays were repeated thrice for each treatment. Larval mortalities were quantified after 3 days, with 50% lethal concentration (LC50) values calculated using Probit analysis (SPSS, Chicago, IL, United States) (Finney, 1971).
The Bt GS57 was inoculated in LB media and kept at 30°C while shaking at 200 rpm. One milliliter of culture was sampled out from the conical flask repeatedly during the incubation period. In addition, the OD600 value was measured to observe the growth state of the cells to obtain microbial growth profiles.
We used a scanning electron microscope (SEM) and SDS-PAGE to characterize the Bt GS57 strain. The Bt GS57 spore-crystal mixtures were washed three times with ice-cold 0.01 M PBS. Then, the cells were harvested and fixed in 2.5% glutaraldehyde in 0.1 M PB for 2 h at 20°C. The samples were dehydrated in an ethanol graded series (30, 50, 70, 80, 90, 95%, and absolute ethanol) for 15 min each, before critical point drying. After fixation and dehydration, the samples were sprayed with gold using an ion sputtering equipment (IXRF, American) with a thickness of about 20–30 nm. Afterward, the material was examined and photographed in a HITACHI SU8100 (HITACHI, Tokyo, Japan) at a voltage of 3 kv. The SDS-PAGE of proteins was performed as described elsewhere (Nair et al., 2018). The molecular mass of proteins was determined using a high range protein molecular weight marker (10–250 kDa) obtained from the pre-stained protein ladder (Thermo Scientific, MA, United States).
The colony shape and the color of the cells were observed by culturing on LB medium, NA medium, beef extract peptone agar medium, dextrose agar medium, and egg yolk agar medium at 30°C for 2–4 days. In addition, the VP reaction of Bt GS57, the production extracellular of amylase, lecithinase, and gelatinase, and the effect on esculin were measured. Finally, acid production was assessed using D-glucose, D-sucrose, D-cellobiose, and salicin media.
The Bt GS57 genome was sequenced using a PacBio RS II platform and Illumina HiSeq 4000 platform at the Beijing Genomics Institute (BGI, Shenzhen, China). PacBio subreads with a lengthless than 1 kb were removed during the analysis. The program Pbdagcon1 was used for self-correction. Draft genomic unitigs, which are uncontested fragments, were assembled against a high-quality corrected circular consensus sequence subreads set (Zuo et al., 2020). To improve the accuracy of the genome sequences, GATK2 and SOAP tool packages (SOAP2, SOAPsnp, SOAPindel) were used to make single-base corrections. To trace the presence of any plasmid, the filtered Illumina reads were mapped using SOAP to the bacterial plasmid database.
The best hit was subjected to Blast alignment tool for function annotation against ten databases: the VFDB (Virulence Factors of Pathogenic Bacteria), ARDB (Antibiotic Resistance Genes Database), CAZy (Carbohydrate-Active enZYmes Database), IPR Swiss-Prot, T3SS (Type III secretion system Effector protein), KEGG (Kyoto Encyclopedia of Genes and Genomes), COG (Clusters of Orthologous Groups), NR (Non-Redundant Protein Database databases), and GO (Gene Ontology). Function annotation was set at an E-value threshold of e < 1e–5.
The 16S rDNA sequences of Bt GS57 were compared and aligned with other Bacillus species using ClustalX. The datasets includes 1,314 sites for 16S rDNA gene. Phylogenetic tree was generated with the MEGAX software, using neighbor-joining (NJ) method with Kimura 2-parameter model, which the gap opening penalty was 15, and gap extension penalty was 6.66 (Saitou and Nei, 1987). Branch supports were evaluated through the ultra-fast bootstrapping method with 1,000 replicates. The strains and their 16S rDNA corresponding to the GenBank accession numbers given below: B. thuringiensis BMB171 (CP001903.1); B. thuringiensis HD521 (CP010106); B. thuringiensis 97-27 (AE017355.1); B. thuringiensis YBT-020 (CP002508.1); B. thuringiensis Al Hakam (CP000485.1); Bacillus cereus ATCC 14579 (AE016877.1); B. cereus E33L (CP000001.1); B. cereus CCM 2010 (NR_115714.1); B. cereus IAM 12605 (NR_115526.1); B. cereus NBRC 15305 (NR_112630.1); B. cereus JCM 2152 (NR_113266.1); Bacillus anthracis SB1 (NR_118379.1); B. anthracis ATCC 14578 (NR_041248.1); B. anthracis SBS1 (NR_118536.1); B. anthracis CDC 684 (CP001215.1); B. anthracis Sterne (AE017225.1); B. anthracis Ames Ancestor (AE017334.2); Bacillus megaterium ATCC 14581 (NR_117473.1); B. megaterium IAM 13418 (NR_043401.1); B. megaterium DSM 32 (NR_118962.1); Bacillus licheniformis ATCC 14580 (NR_074923.1); B. licheniformis DSM 13 (NR_118996.1); Bacillus atrophaeus JCM 9070 (NR_024689.1); B. atrophaeus NBRC 15539 (NR_112723.1); Bacillus cytotoxicus NVH 391-98 (CP000764.1).
A 300 μLBt GS57 (4 mg mL–1) cell crystal mixture was spread on the diet surface to feed the 4th instar S. exigua larvae. The gut tissue (including contents) of the S. exigua larvae was extracted every 12 h and sequenced by Illumina MiSeq. Alterations of intestinal microbial diversity were analyzed at different time points (0, 12, 24, 48, and 72 h) of feeding Bt GS57. The S. exigua were surface-sterilized by immersion in 75% ethanol for 3 min and then rinsed three times in axenic RNase-free ddH2O. Afterward, they were dissected to separate the gut and the remainder of the body. The DNA was amplified by the 338F/806R primer (Table 1), which targets the V3 and V4 hypervariable region of the bacterial 16S rDNA gene. The 16S rDNA gene was sequenced on the Illumina MiSeq platform by Beijing Biomarker Technologies. The experiment was repeated three times.
After Bt GS57 infection, samples were collected at four time points (0, 24, 48, and 72 h) based on the LC50 value in the 4th instar larvae of S. exigua. The bacterial DNA from S. exigua guts was extracted using TIANamp Genomic DNA Kit (Tiangen, Beijing, China), according to the manufacturer’s instructions. Bacterial quantitation by qRT-PCR was performed on genomic DNA using universal eubacteria primers to amplify the 16S ribosomal RNA (rRNA) fragments (Wei et al., 2017). The Seactin gene was used as an endogenous control (Table 1). Each reaction was performed in triplicate. The data were analyzed using the threshold cycle 2–ΔΔCt method (Livak and Schmittgen, 2001).
To prepare the dissected guts, 4th instar larvae of S. exigua were surface sterilized in 75% ethanol for 3–5 min and then rinsed four times with PBS buffer. The guts were aseptically dissected in a plate containing axenic distilled water using sterilized forceps. It was then transferred to 300 μL axenic distilled water and homogenized for bacterial isolation (Wei et al., 2017). The S. exigua larvae were surface-sterilized, and the perfusion was collected. The gut homogenates were serially diluted 100,000 times with distilled water, plated onto LB agar plates, and then incubated for 2–3 days at 30°C, after which the colony forming units (CFUs) per plate were counted. The gut bacterial genomic DNA was extracted from collected samples using TIANamp Bacteria DNA Kit (Tiangen, Beijing, China), following the manufacturer’s instructions. The isolated bacteria were identified using 16S rRNA gene universal primers 27F/1492R (Table 1). The resulting PCR products were subjected to Sanger sequencing at Huada (Beijing, China). The sequenced bacterial strains were then identified via BLAST search.3 The phylogenetic tree was constructed using the neighbor-joining method using MEGAX software.
The 300 μL Bt GS57 (4 mg mL–1) cell crystal mixture was added to the axenic diet. The axenic and non-axenic group of S. exigua was then compared. B. cereus HB1 and Enterococcus mundtii HB1 were cultured in LB medium at 37°C for 12 h until their OD600 reached 0.8. The bacterial culture was then pelleted by centrifugation (8,000 rpm for 6 min), washed twice in axenic ddH2O, and resuspended in axenic ddH2O to obtain 109 cells per mL. Next, the 300 μL bacterial pellet was mixed with a 300 μL Bt GS57 (4 mg mL–1) cell crystal mixture. Lastly, bacterial suspension was added to an axenic diet, and then the activity was evaluated in sterilized S. exigua.
The growth curve of Bt GS57 showed a 0–4 h delay and 5–20 h logarithmic growth periods. The stable growth had an OD600 between 2.046 and 2.155 (Figure 1A). The Bt GS57 can produce bipyramidal parasporal crystals during the stationary phase of its growth cycle (Figure 1B). The protein pattern of parasporal bodies as determined by SDS-PAGE consisted of two major bands of about 70–130 kDa, consistent with the its parasporal crystal gene (Figure 1C). The LC50 value of the Bt GS57 strain against S. exigua was 0.339 mg mL–1 (Table 2).
Figure 1. General characteristics of Bt GS57. (A) The growth curve of Bt GS57. (B) Scanning electron microscope (SEM) analysis of Bt GS57 spores and parasporal crystals. (C) SDS-PAGE analysis of spore-crystal suspension of Bt GS57: lane M, molecular mass standard; lane 1, Bt GS57.
Colonies of Bt GS57 strain were circular, white-colored, and rough in LB medium, NA medium, Beef extract peptone agar medium, Dextrose agar medium, and Egg yolk agar medium. The Bt GS57 utilized D-sucrose, D-cellobiose, and esculin, producing extracellular amylase, lecithinase, and gelatinase. Hydrolysis study showed that Bt GS57 can hydrolyze starch, gelatin, lecithin, and glycogen. The Bt GS57 does not produce pigments and membranes, and does not use glucose. Results of 10 physiological and biochemical reactions of B.t. var. thuringiensis were consistent; hence, we concluded that the Bt GS57 strain belongs to B.t. var. thuringiensis.
The Bt GS57 genome was sequenced using the Illumina (MiSeq) platform with paired-end reads sequencing. The total size of Bt GS57 genome was 6.17 Mbp with an average G + C content of 35.66%. In total, 6,597 genes, 6,532 coding sequences, 14 complete rRNAs, and 108 tRNAs were identified by PGAP analysis. Among 6,597 genes, 2,664 (40.38%) were smaller than 500 nt, 2,275 (34.49%) were between 500 nt and 1,000 nt, and 1,658 (25.13%) were longer than 1,000 nt, with an average gene length size of 757.66 nt (Supplementary Figure 1).
The 6,497 (98.48% of all distinct sequences) genes matched with the NR database (Supplementary Table 1), 2,674 (40.53%) to Swiss-Prot database (Supplementary Table 2), 3,324 (50.38%) to GO, 4,096 (62.08%) to COG, 3,041 (46.09%) to KEGG, 326 (4.94%) to VFDB, 53 (0.8%) to ARDB, 94 (1.42%) to CAZY, 5,106 (77.39%) to IPR, 755 (11.44%) to T3SS (Supplementary Figure 2). The summary statistics of the BLAST assignment are shown in Table 3 (Supplementary Table 3). GO enrichment of Bt GS57 gene function revealed that these genes include the “cellular component,” “biological process,” and “molecular function” (Supplementary Table 4) (Supplementary Figure 3) (Blake, 2013). To identify the metabolic pathways populated by these genes, 3,041 annotated genes were grouped into six categories: Cellular Processes, Environmental, Genetics, Human Disease, Metabolism, and Organismal System (Supplementary Table 5). The histogram obtained after the KEGG secondary classification statistics of each sample is shown in Supplementary Figure 4.
After assembly, the genome of Bt GS57 consisted of 4 replicons with a circular chromosome of 5,309,747 bp. The GC content of the circular chromosomes was 35.28% (Supplementary Figure 5). The three circular plasmids Bt GS57-1, Bt GS57-2 and Bt GS57-3 with a length of 76,132, 72,996, and 712,902 bp, respectively. The GC contents of the three plasmids ranged from 32.32 to 33.93% (Supplementary Figure 6). Genome annotation results showed that Bt GS57 has insecticidal genes cry1Ca, cry1Da, cry2Ab, cry9Ea, cry1Ia, cry1Aa, and vip3Aa.
A phylogenetic tree was constructed to check whether the chimeric structure of the 16S rDNA gene affects species identification. Twenty-five Bacillus strains and Bt GS57 were chosen for phylogenetic analysis, showing more than 97% sequence similarity based on the basic local alignment search tool. The 16S rDNA sequence from B. cytotoxicus NVH 391-98 168 was selected as an outgroup. The phylogenetic tree illustrated that the Bt GS57 strain is closely related to B. thuringiensis serovar Indiana strain HD521 (Figure 2). However, the bootstraps value of the phylogenetic tree was very low because the 16S rDNA nucleotide sequence divergence of the chosen strains was also low, corroborating the previous study (Li et al., 2015). Ash et al. (1991) showed that 16S rDNA nucleotide sequences among B. anthracis, B. thuringiensis, and B. cereus were highly similar, exhibiting more than 99% sequence similarity.
Figure 2. Phylogenetic tree based on 16S rDNA sequences Bt GS57 and Bacillus cereus sensu lato species group. The strains and their 16S rDNA corresponding to the GenBank accession numbers given below: Bacillus thuringiensis BMB171 (CP001903.1); B. thuringiensis HD521 (CP010106); B. thuringiensis 97-27 (AE017355.1); B. thuringiensis YBT-020 (CP002508.1); B. thuringiensis Al Hakam (CP000485.1); B. cereus ATCC 14579 (AE016877.1); B. cereus E33L (CP000001.1); B. cereus CCM 2010 (NR_115714.1); B. cereus IAM 12605 (NR_115526.1); B. cereus NBRC 15305 (NR_112630.1); B. cereus JCM 2152 (NR_113266.1); Bacillus anthracis SB1 (NR_118379.1); B. anthracis ATCC 14578 (NR_041248.1); B. anthracis SBS1 (NR_118536.1); B. anthracis CDC 684 (CP001215.1); B. anthracis Sterne (AE017225.1); B. anthracis Ames Ancestor (AE017334.2); Bacillus megaterium ATCC 14581 (NR_117473.1); B. megaterium IAM 13418 (NR_043401.1); B. megaterium DSM 32 (NR_118962.1); Bacillus licheniformis ATCC 14580 (NR_074923.1); B. licheniformis DSM 13 (NR_118996.1); Bacillus atrophaeus JCM 9070 (NR_024689.1); B. atrophaeus NBRC 15539 (NR_112723.1); Bacillus cytotoxicus NVH 391-98 (CP000764.1).
Through deep sequencing of the 16S rRNA gene, we further assessed the gut bacteria’s dynamic composition and diversity in the non-infected S. exigua and the Bt GS57-infected S. exigua at 0, 12, 24, 48, and 72 h. In the non-infected S. exigua, the gut bacteria were diverse and dominated by bacteria of four phyla: Firmicutes, Proteobacteria, Bacterioidetes, and Actinobacteria (Figure 3A) (Supplementary Table 6). Firmicutes and Proteobacteria showed a dynamic change over time, possibly because of alterations in S. exigua physiology. The relative expression level of Enterococcus in the Bt GS57 treatment group was highest at 94.07%, and in the CK treatment group was lowest at 92.79% in 72 h (Figure 3B) (Supplementary Table 7).
Figure 3. Bt GS57 infection changes in gut microbiota composition in Spodoptera exigua. (A) Histogram showing temporal changes, at the phylum level, in non-infected (Ctrl; ddH2O treatment as control) and Bt GS57-infected S. exigua (n = 45) over 72 h. (B) Heat map showing temporal changes, at the genus level, in Ctrl and Bt GS57-infected S. exigua. (C) Principal component analysis of microbial communities in different groups from Ctrl and Bt GS57-infected S. exigua.
Compared with non-infected S. exigua, Bt GS57 infection showed a reduced bacterial diversity (Simpson’s evenness, P < 0.05) (Table 4). Firmicutes predominated in Bt GS57-infected S. exigua at 24 h (Figure 3A). Furthermore, the composition and diversity of the gut bacterial population changed markedly in S. exigua after infection by Bt GS57, resulting in an almost exclusive colonization by one genus of Firmicutes–Enterococcus–in the Bt GS57-infected S. exigua (Figure 3B).
Table 4. The diversity index analyzed upon deep sequencing for each sample of gut microbiota of non-infected or Bt GS57-infected Spodoptera exigua at five time points.
The results of principal component analysis (PCA) showed that the gut microbial community composition differed between uninfected S. exigua and Bt GS57-infected S. exigua at 12, 24, and 48 h, while the gut microbial community composition was similar at 72 h (Figure 3C). These results indicate that Bt GS57 infection can alter gut microbial community composition.
Next, we determined whether Bt GS57 infection affected the S. exigua gut microbiota by testing the bacterial loads in the gut of the S. exigua at 24, 48, and 72 h, through topical Bt GS57 infection. Results showed that the gut bacterial load was significantly upregulated in S. exigua following post-infection with Bt GS57 at different time intervals than non-infected controls treated with ddH2O. In addition, the qRT-PCR analysis of gut total bacterial load in infected S. exigua at 24 h showed that the relative expression level of bacterial load increased significantly, reaching a maximum level at 72 h (Figure 4).
Figure 4. Effects of Bt GS57 infection on the gut bacterial load of Spodoptera exigua. The qPCR-based quantification of gut bacterial load in ddH2O and Bt GS57 treatment group of S. exigua (n = 6) at 0, 24, 48, and 72 h. Gene expression of each sample was normalized to that of S. exigua at time 0 (taken as 1). Data are representative of three independent experiments (mean + s.e.m.). The double asterisk indicates the significant difference determined by the student’s t-test, P < 0.01.
To investigate the possible role of the gut microbiota in Bt GS57 in the S. exigua, axenic S. exigua was generated via treatment with oral antibiotics. The efficacy of elimination of gut bacteria was confirmed by plating gut homogenates onto LB agar plates and performing PCR analysis using bacterial 16S rDNA gene universal primers 27F/1492R, which detected unculturable microbes (e.g., obligate anaerobes) (Figures 5A,B and Table 1).
Figure 5. Generation of axenic Spodoptera exigua. (A) The efficacy of eliminating gut cultivable bacteria from non-axenic and axenic S. exigua (n = 3) gut homogenates on LB agar plates. (B) The efficacy of elimination of gut bacteria by performing PCR analysis on non-axenic and axenic S. exigua (n = 3) using 16S rRNA gene universal primers 27F/1492R.
An insect bioassay was conducted using the 4th instar larvae of S. exigua with and without gut microbiota. The biological activity test results of Bt GS57 showed that the mortality rate of axenic insects was significantly lower than that of non-axenic insects, and the mortality rate of non-axenic insects and axenic insects was 76.67 and 56.67% at 72 h, respectively (P < 0.01, t-test) (Figure 6). This result indicates that the gut microbiota accelerates the killing of S. exigua by Bt GS57.
Figure 6. Effect of the gut microbiota on Bt GS57 in Spodoptera exigua. (A) Survival of axenic and non-axenic S. exigua (n = 45) following infection (+Bt GS57) or non-infectioun (−Bt GS57) with Bacillus thuringiensis. (B) The gut culturable bacterial load from axenic-Bt GS57, non-axenic + Bt GS57, and non-infected + Bt GS57 of S. exigua (n = 3) at 0, 24, 48, and 72 h post-Bt GS57 infection. Bacterial load was determined by plating the homogenate of S. exigua guts with 100,000 dilutions on LB agar plates.
Based on 16S rDNA gene sequence analysis, the two predominant cultivable bacteria showed high similarity with E. mundtii 15-1 and B. cereus FDAARGOS_797 and were named E. mundtii HB1 and B. cereus HB1, respectively (Supplementary Figure 7). To determine whether the proliferated E. mundtii HB1 and B. cereus HB1 contributed to the enhanced mortality speed, we reintroduced the overproliferating B. cereus HB1 and E. mundtii HB1 from the gut of Bt GS57-infected S. exigua into the gut of axenic S. exigua. We found that reintroducing B. cereus HB1 restored S. exigua susceptibility to Bt GS57 infection, while the reintroduction of E. mundtii HB1 reduced S. exigua susceptibility to Bt GS57 infection in comparison with ddH2O controls (Figure 7). At the same time, feeding B. cereus HB1 and E. mundtii HB1 alone did not cause the death of S. exigua.
Figure 7. Survival of Spodoptera exigua fed Bacillus cereus and Enterococcus mundtii after infection with Bt GS57. Control S. exigua were fed ddH2O. Bacteria were introduced into the 4th instar larvae of S. exigua gut. Introduction of B. cereus (B. cereus + Bt GS57) significantly increased S. exigua susceptibility to Bt GS57 infection compared with the ddH2O + Bt GS57 treatment [log-rank (Mantel-Cox) test, P < 0.01]. The experiments were performed in three biological replicates. The log-rank test was used to assess the significance of differences between two survival curves using GraphPad Prism software.
Bacillus thuringiensis, as an environmentally friendly microbial pesticide, is already a useful alternative or supplement to synthetic chemical pesticide. Hence, it is a key source of genes for transgenic expression to provide pest resistance in plants (Ren et al., 2021). At present, B. thuringiensis pesticides have been widely used worldwide, accounting for about 90% of the microbial pesticide market in the United States (Chattopadhyay et al., 2004). However, the extensive use of B. thuringiensis insecticides and genetically modified plants has resulted in resistance to a large number of field pests. Furthermore, it has been reported that insects such as P. xylostella, S. exigua, Helicoverpa zea, Spodoptera frugiperda, and Pectinophora gossypiella have high resistance to the insecticidal protein from B. thuringiensis (Hernandez-Martinez et al., 2008; Tabashnik et al., 2008; Bagla, 2010; Storer et al., 2010; Naik et al., 2018; Xiong et al., 2021). Therefore, the search for new high insecticidal B. thuringiensis microbial insecticides, a clear composition of insecticidal genes of B. thuringiensis strain, and the expression of insecticidal proteins are of great significance for further understanding of B. thuringiensis insecticidal activity.
This study sequenced and characterized a novel B. thuringiensis strain isolated from soil. The complete genome of Bt GS57 exhibited some interesting features. Based on the next-generation sequencing of Illumina Hiseq2000 and de novo assembly, we found that Bt GS57 strain contains cry1Ca, cry1Da, cry2Ab, cry9Ea, cry1Ia, cry1Aa, vip3Aa gene, chitinase, and zwittermicin. Using genome sequencing to obtain more comprehensive information provides us with more gene resources for the biological control. It is of great significance in extending the scope of control and delaying the development of insecticide-resistance of the pest.
The gut microbiota contributes to the host’s health, and changes in the composition of the microbiota can cause disease (Pita et al., 2018; Li et al., 2020). The Cry toxin produced by B. thuringiensis creates pores in the membrane of the larval gut cells and disrupts insect gut microbial homeostasis (Portugal et al., 2017; Li et al., 2021). In this study, we evaluated the changes in the bacterial community of S. exigua after B. thuringiensis exposure. The significantly decreased Simpson’s index in B. thuringiensis-treated larvae suggested that they have a lower bacterial species richness than H2O-treated larvae (Table 4). At the phylum level, the results of our study were similar to other reports, namely the dominant bacterial phyla, such as Firmicutes and Proteobacteria (Gao et al., 2020). However, the dominant bacterial Firmicutes significantly increased while the quantity of Proteobacteria significantly reduced after feeding on B. thuringiensis (Figure 3). Therefore, changes in the composition of the gut microbiota caused by B. thuringiensis may adversely affect the physiological function of the insect. In addition, compared with the H2O-treated control group, the total amount of bacteria in the larvae exposed to B. thuringiensis increased significantly, further emphasizing the functional changes of intestinal bacteria after exposure to B. thuringiensis (Figure 6B). The qRT-PCR results showed that the gut bacterial load of S. exigua significantly increased in the Bt GS57 treatment group. Therefore, future studies should focus on the interaction between B. thuringiensis and S. exigua gut bacteria and their functions (Figure 4).
Many studies have shown that gut microbiota can enhance insecticide resistance (Kota et al., 2021; Hu et al., 2022). Inoculation of Spodoptera litura larvae with E. mundtii and Enterococcus casseliflavus significantly reduced susceptibility to methomyl (Hu et al., 2022). In Cletus punctiger, the gut commensal bacterium Burkholderia can degrade organophosphate compounds and remarkably increase the resistance against fenitrothion (Kota et al., 2021). A previous study has suggested that axenic Lepidopteran insects are not sensitive to B. thuringiensis, where inoculation of midgut bacteria can restore B. thuringiensis pathogenicity (Paramasiva et al., 2014). This is supported by previous work of Paramasiva et al. (2014), showing that antibiotics eliminated from gut microflora influenced the toxicity of B. thuringiensis against H. armigera. Wei et al. (2017) also confirmed that B. bassiana interacts with mosquito gut microorganisms to accelerate mosquito death. In this study, we have investigated and evaluated the role of the gut microbiota in the interaction of the Bt GS57 with S. exigua. Our findings indicate that Bt GS57 interacts with the gut microbiota to promote S. exigua death (Figure 6A). Reintroduction of gut B. cereus HB1 and E. mundtii HB1 into axenic S. exigua revealed that B. cereus HB1 can significantly enhance the susceptibility of the S. exigua to Bt GS57, while E. mundtii HB1 can inhibit the vulnerability of the S. exigua to Bt GS57 (Figure 7). Raymond et al. (2008) found that antibiotics secreted by B. cereus can reduce the abundance of symbiotic gut microbiota and promote the infection of B. thuringiensis in the co-infection experiment of B. cereus and B. thuringiensis. However, Shao et al. (2017) pointed out that the E. mundtii, a commensal gut bacteria, produces antimicrobial peptides to kill foreign pathogens effectively, thereby suppressing the mortality of S. exigua. These may result from the interaction between B. thuringiensis and the gut bacteria of S. exigua.
In conclusion, this study carried out a novel analysis of the wild Bt GS57 strain and identified the insecticidal gene at the genomic level. Furthermore, the insect bioassay confirmed that the gut microbiota accelerates killing of S. exigua by Bt GS57. Related research provides reference data for elucidating the functional characteristics of the Bt GS57 strain, further understanding the interaction of B. thuringiensis-insect-microbiota, and exploring its insecticidal potential.
The datasets presented in this study can be found in online repositories. The names of the repository/repositories and accession number(s) can be found in the article/Supplementary Material.
YL, DZ, and WG conceived the research. YL, DZ, HW, and YJ conducted the experiments. DZ and WG contributed the material. ZL, XG, and YB analyzed the data and conducted the statistical analyses. YL wrote the manuscript. WG secured the funding. All authors read and approved the manuscript.
This research was supported by grants from the National Key R&D Program of China (2017YFD0200400), Modern Agro-industry Technology Research System (CARS-13), National Natural Science Foundation of China (31471775), and Foundation of Graduate School of the Chinese Academy of Agricultural Sciences (1610042022005).
The authors declare that the research was conducted in the absence of any commercial or financial relationships that could be construed as a potential conflict of interest.
All claims expressed in this article are solely those of the authors and do not necessarily represent those of their affiliated organizations, or those of the publisher, the editors and the reviewers. Any product that may be evaluated in this article, or claim that may be made by its manufacturer, is not guaranteed or endorsed by the publisher.
We are grateful to all lab members for helpful discussions and suggestions in this work.
The Supplementary Material for this article can be found online at: https://www.frontiersin.org/articles/10.3389/fmicb.2022.835227/full#supplementary-material
Ash, C., Farrow, J. A., Dorsch, M., Stackebrandt, E., and Collins, M. D. (1991). Comparative analysis of Bacillus anthracis, Bacillus cereus, and related species on the basis of reverse transcriptase sequencing of 16S rRNA. Int. J. Syst. Bacteriol. 41, 343–346. doi: 10.1099/00207713-41-3-343
Bagla, P. (2010). India. Hardy cotton-munching pests are latest blow to GM crops. Science 327:1439. doi: 10.1126/science.327.5972.1439
Baragamaarachchi, R. Y., Samarasekera, J. K. R. R., Weerasena, O. V. D. S., Lamour, K., and Jurat-Fuentes, J. L. (2019). Identification of a native Bacillus thuringiensis strain from Sri Lanka active against Dipel-resistant Plutella xylostella. PeerJ. 7:e7535. doi: 10.7717/peerj.7535
Blake, J. A. (2013). Ten quick tips for using the gene ontology. PLoS Comput. Biol. 9:e1003343. doi: 10.1371/journal.pcbi.1003343
Broderick, N. A., Raffa, K. F., and Handelsman, J. (2006). Midgut bacteria required for Bacillus thuringiensis insecticidal activity. Proc. Natl. Acad. Sci. U. S. A. 103, 15196–15199. doi: 10.1073/pnas.0604865103
Cao, Z. L., Tan, T. T., Jiang, K., Mei, S. Q., Hou, X. Y., and Cai, J. (2018). Complete genome sequence of Bacillus thuringiensis L-7601, a wild strain with high production of melanin. J. Biotechnol. 275, 40–43. doi: 10.1016/j.jbiotec.2018.03.020
Carlson, C. R., and Kolsto, A. B. (1993). A complete physical map of a Bacillus thuringiensis chromosome. J. Bacteriol. 175, 1053–1060. doi: 10.1128/jb.175.4.1053-1060.1993
Challacombe, J. F., Altherr, M. R., Xie, G., Bhotika, S. S., Brown, N., Bruce, D., et al. (2007). The complete genome sequence of Bacillus thuringiensis Al Hakam. J. Bacteriol. 189, 3680–3681. doi: 10.1128/JB.00241-07
Chattopadhyay, A., Bhatnagar, N. B., and Bhatnagar, R. (2004). Bacterial insecticidal toxins. Crit. Rev. Microbiol. 30, 33–54. doi: 10.1080/10408410490270712
Douglas, A. E. (2018). The Drosophila model for microbiome research. Lab. Anim. 47, 157–164. doi: 10.1038/s41684-018-0065-0
Gao, X., Li, W., Luo, J., Zhang, L., Ji, J., Zhu, X., et al. (2020). DNA sequencing reveals bacterial communities in midgut and other parts of the larvae of Spodoptera exigua Hubner (Lepidoptera: noctuidae). FEMS Microbiol. Lett. 367:fnaa002. doi: 10.1093/femsle/fnaa002
Gassmann, A. J. (2021). Resistance to bt maize by western corn rootworm: effects of pest biology, the Pest-Crop interaction and the agricultural landscape on resistance. Insects 12:136. doi: 10.3390/insects12020136
Hernandez-Martinez, P., Ferre, J., and Escriche, B. (2008). Susceptibility of Spodoptera exigua to 9 toxins from Bacillus thuringiensis. J. Invertebr. Pathol. 97, 245–250. doi: 10.1016/j.jip.2007.11.001
Hilbeck, A., Defarge, N., Bohn, T., Krautter, M., Conradin, C., Amiel, C., et al. (2018). Impact of Antibiotics on Efficacy of Cry Toxins Produced in Two Different Genetically Modified Bt Maize Varieties in Two Lepidopteran Herbivore Species, Ostrinia nubilalis and Spodoptera littoralis. Toxins 10:489. doi: 10.3390/toxins10120489
Hu, L., Sun, Z., Xu, C., Wang, J., Mallik, A. U., Gu, C., et al. (2022). High nitrogen in maize enriches gut microbiota conferring insecticide tolerance in lepidopteran pest Spodoptera litura. Iscience 25:103726. doi: 10.1016/j.isci.2021.103726
Jones, R. M., Luo, L., Ardita, C. S., Richardson, A. N., Kwon, Y. M., Mercante, J. W., et al. (2013). Symbiotic lactobacilli stimulate gut epithelial proliferation via Nox-mediated generation of reactive oxygen species. EMBO J. 32, 3017–3028. doi: 10.1038/emboj.2013.224
Kota, I., Seonghan, J., Hideomi, I., and Yoshitomo, K. (2021). Insecticide resistance governed by gut symbiosis in a rice pest, Cletus punctiger, under laboratory conditions. Biol Lett. 17:20200780. doi: 10.1098/rsbl.2020.0780
Lei, Y., Hussain, A., Guan, Z., Wang, D., Jaleel, W., Lyu, L., et al. (2021). Unraveling the Mode of Action of Cordyceps fumosorosea: potential Biocontrol Agent against Plutella xylostella (Lepidoptera: plutellidae). Insects 12:179. doi: 10.3390/insects12020179
Li, F., Li, M., Mao, T., Wang, H., Chen, J., Lu, Z., et al. (2020). Effects of phoxim exposure on gut microbial composition in the silkworm, Bombyx mori. Ecotox. Environ. Safe. 189:110011. doi: 10.1016/j.ecoenv.2019.110011
Li, Q., Xu, L. Z., Zou, T., Ai, P., Huang, G. H., Li, P., et al. (2015). Complete genome sequence of Bacillus thuringiensis strain HD521. Stand. Genomic Sci. 10:62. doi: 10.1186/s40793-015-0058-1
Li, S., Xu, X., De Mandal, S., Shakeel, M., Hua, Y., Shoukat, R. F., et al. (2021). Gut microbiota mediate Plutella xylostella susceptibility to Bt Cry1Ac protoxin is associated with host immune response. Environ. Pollut. 271:116271. doi: 10.1016/j.envpol.2020.116271
Liu, G., Song, L., Shu, C., Wang, P., Deng, C., Peng, Q., et al. (2013). Complete genome sequence of Bacillus thuringiensis subsp. kurstaki strain HD73. Genome Announc. 1:e0008013. doi: 10.1128/genomeA.00080-13
Livak, K. J., and Schmittgen, T. D. (2001). Analysis of relative gene expression data using real-time quantitative PCR and the 2(-Delta Delta C(T)) Method. Methods 25, 402–408. doi: 10.1006/meth.2001.1262
Lone, S. A., Malik, A., and Padaria, J. C. (2017). Selection and characterization of Bacillus thuringiensis strains from northwestern Himalayas toxic against Helicoverpa armigera. Microbiologyopen 6:e00484. doi: 10.1002/mbo3.484
Mason, K. L., Stepien, T. A., Blum, J. E., Holt, J. F., Labbe, N. H., Rush, J. S., et al. (2011). From commensal to pathogen: translocation of Enterococcus faecalis from the midgut to the hemocoel of Manduca sexta. Mbio 2, e00065–11. doi: 10.1128/mBio.00065-11
Naik, V. C., Kumbhare, S., Kranthi, S., Satija, U., and Kranthi, K. R. (2018). Field-evolved resistance of pink bollworm, Pectinophora gossypiella (Saunders) (Lepidoptera: gelechiidae), to transgenic Bacillus thuringiensis (Bt) cotton expressing crystal 1Ac (Cry1Ac) and Cry2Ab in India. Pest Manag. Sci. 74, 2544–2554. doi: 10.1002/ps.5038
Nair, K., Al-Thani, R., Al-Thani, D., Al-Yafei, F., Ahmed, T., and Jaoua, S. (2018). Diversity of Bacillus thuringiensis Strains From Qatar as Shown by Crystal Morphology, δ-Endotoxins and Cry Gene Content. Front. Microbiol. 9:708. doi: 10.3389/fmicb.2018.00708
Nguyen, H. T., Nguyen, T. T., Pham, H., Nguyen, Q., Tran, M. T., Nguyen, A. H., et al. (2018). Fate of carotenoid-producing Bacillus aquimaris SH6 colour spores in shrimp gut and their dose-dependent probiotic activities. PLoS One 13:e209341. doi: 10.1371/journal.pone.0209341
Paramasiva, I., Sharma, H. C., and Krishnayya, P. V. (2014). Antibiotics influence the toxicity of the delta endotoxins of Bacillus thuringiensis towards the cotton bollworm, Helicoverpa armigera. BMC Microbiol. 14:200. doi: 10.1186/1471-2180-14-200
Pita, L., Rix, L., Slaby, B. M., Franke, A., and Hentschel, U. (2018). The sponge holobiont in a changing ocean: from microbes to ecosystems. Microbiome 6:46. doi: 10.1186/s40168-018-0428-1
Portugal, L., Muñóz-Garay, C., Martínez De Castro, D. L., Soberón, M., and Bravo, A. (2017). Toxicity of Cry1A toxins from Bacillus thuringiensis to CF1 cells does not involve activation of adenylate cyclase/PKA signaling pathway. Insect Biochem. Molec. 80, 21–31. doi: 10.1016/j.ibmb.2016.11.004
Qin, N., Li, D., and Yang, R. (2011). [Next-generation sequencing technologies and the application in microbiology–a review]. Acta Microbiol. Sin. 51, 445–457. doi: 10.13343/j.cnki.wsxb.2011.04.013
Ramya, S. L., Venkatesan, T., Srinivasa, M. K., Jalali, S. K., and Verghese, A. (2016). Detection of carboxylesterase and esterase activity in culturable gut bacterial flora isolated from diamondback moth, Plutella xylostella (Linnaeus), from India and its possible role in indoxacarb degradation. Braz. J. Microbiol. 47, 327–336. doi: 10.1016/j.bjm.2016.01.012
Raymond, B., Lijek, R. S., Griffiths, R. I., and Bonsall, M. B. (2008). Ecological consequences of ingestion of Bacillus cereus on Bacillus thuringiensis infections and on the gut flora of a lepidopteran host. J. Invertebr. Pathol. 99, 103–111. doi: 10.1016/j.jip.2008.04.007
Regode, V., Kuruba, S., Mohammad, A. S., and Sharma, H. C. (2016). Isolation and Characterization of Gut Bacterial Proteases Involved in Inducing Pathogenicity of Bacillus thuringiensis Toxin in Cotton Bollworm, Helicoverpa armigera. Front. Microbiol. 7:1567. doi: 10.3389/fmicb.2016.01567
Ren, Y., Zhou, X., Dong, Y., Zhang, J., Wang, J., and Yang, M. (2021). Exogenous Gene Expression and Insect Resistance in Dual Bt Toxin Populus x euramericana ‘Neva’ Transgenic Plants. Front. Plant Sci. 12:660226. doi: 10.3389/fpls.2021.660226
Saitou, N., and Nei, M. (1987). The neighbor-joining method: a new method for reconstructing phylogenetic trees. MoI Bio Evo. 4, 406–425. doi: 10.1093/oxfordjournals.molbev.a040454
Shao, Y., Chen, B., Sun, C., Ishida, K., Hertweck, C., and Boland, W. (2017). Symbiont-Derived Antimicrobials Contribute to the Control of the Lepidopteran Gut Microbiota. Cell Chem Biol. 24, 66–75. doi: 10.1016/j.chembiol.2016.11.015
Storer, N. P., Babcock, J. M., Schlenz, M., Meade, T., Thompson, G. D., Bing, J. W., et al. (2010). Discovery and characterization of field resistance to Bt maize: spodoptera frugiperda (Lepidoptera: noctuidae) in Puerto Rico. J. Econ. Entomol. 103, 1031–1038. doi: 10.1603/ec10040
Su, H., Zou, J., Zhou, Q., Yu, Q., Yang, Y., and Yang, Y. (2017). Better cold tolerance of Bt-resistant Spodoptera exigua strain and the corresponding cold-tolerant mechanism. Pestic. Biochem. Phys. 140, 51–57. doi: 10.1016/j.pestbp.2017.06.003
Tabashnik, B. E., Gassmann, A. J., Crowder, D. W., and Carriere, Y. (2008). Insect resistance to Bt crops: evidence versus theory. Nat. Biotechnol. 26, 199–202. doi: 10.1038/nbt1382
Wang, Y., Fu, J., Zhu, Q., Zhu, L., Zheng, J., Liu, H., et al. (2016). Complete genome sequence of Bacillus thuringiensis serovar alesti BGSC 4C1, a typical strain with toxicity to Lepidoptera insects. J. Biotechnol. 239, 61–64. doi: 10.1016/j.jbiotec.2016.09.015
Wei, G., Lai, Y., Wang, G., Chen, H., Li, F., and Wang, S. (2017). Insect pathogenic fungus interacts with the gut microbiota to accelerate mosquito mortality. Proc. Natl. Acad. Sci. U. S. A. 114, 5994–5999. doi: 10.1073/pnas.1703546114
Xiong, L., Liu, Z., Shen, L., Xie, C., Ye, M., Li, Z., et al. (2021). A Novel Reference for Bt-Resistance Mechanism in Plutella xylostella Based on Analysis of the Midgut Transcriptomes. Insects 12:1091. doi: 10.3390/insects12121091
Zhang, Y., Zhao, D., Yan, X., Guo, W., Bao, Y., Wang, W., et al. (2017). Identification and Characterization of Hyphantria cunea Aminopeptidase N as a Binding Protein of Bacillus thuringiensis Cry1Ab35 Toxin. Int. J. Mol. Sci. 18:2575. doi: 10.3390/ijms18122575
Zhu, L., Peng, D., Wang, Y., Ye, W., Zheng, J., Zhao, C., et al. (2015). Genomic and transcriptomic insights into the efficient entomopathogenicity of Bacillus thuringiensis. Sci Rep. 5:14129. doi: 10.1038/srep14129
Keywords: Bt GS57, genomic feature, Spodoptera exigua, gut microbiota, diversity, dysbiosis
Citation: Li Y, Zhao D, Wu H, Ji Y, Liu Z, Guo X, Guo W and Bi Y (2022) Bt GS57 Interaction With Gut Microbiota Accelerates Spodoptera exigua Mortality. Front. Microbiol. 13:835227. doi: 10.3389/fmicb.2022.835227
Received: 15 December 2021; Accepted: 14 February 2022;
Published: 23 March 2022.
Edited by:
F. L. Consoli, University of São Paulo, BrazilReviewed by:
Antonio Nascimento, University of São Paulo, BrazilCopyright © 2022 Li, Zhao, Wu, Ji, Liu, Guo, Guo and Bi. This is an open-access article distributed under the terms of the Creative Commons Attribution License (CC BY). The use, distribution or reproduction in other forums is permitted, provided the original author(s) and the copyright owner(s) are credited and that the original publication in this journal is cited, in accordance with accepted academic practice. No use, distribution or reproduction is permitted which does not comply with these terms.
*Correspondence: Wei Guo, Z3Vvd2VpMDVAY2Fhcy5jbg==
†These authors have contributed equally to this work
Disclaimer: All claims expressed in this article are solely those of the authors and do not necessarily represent those of their affiliated organizations, or those of the publisher, the editors and the reviewers. Any product that may be evaluated in this article or claim that may be made by its manufacturer is not guaranteed or endorsed by the publisher.
Research integrity at Frontiers
Learn more about the work of our research integrity team to safeguard the quality of each article we publish.