- 1Department of Biology, University of North Carolina at Chapel Hill, Chapel Hill, NC, United States
- 2Department of Biology, University of Pennsylvania, Philadelphia, PA, United States
- 3Department of Biology, Western Carolina University, Cullowhee, NC, United States
- 4Environment, Ecology and Energy Program, University of North Carolina, Chapel Hill, NC, United States
The interactions among host-associated microbes and parasites can have clear consequences for disease susceptibility and progression within host individuals. Yet, empirical evidence for how these interactions impact parasite transmission between host individuals remains scarce. We address this scarcity by using a field mesocosm experiment to investigate the interaction between a systemic fungal endophyte, Epichloë coenophiala, and a fungal parasite, Rhizoctonia solani, in leaves of a grass host, tall fescue (Lolium arundinaceum). Specifically, we investigated how this interaction impacted transmission of the parasite under field conditions in replicated experimental host populations. Epichloë-inoculated populations tended to have greater disease prevalence over time, though this difference had weak statistical support. More clearly, Epichloë-inoculated populations experienced higher peak parasite prevalences than Epichloë-free populations. Epichloë conferred a benefit in growth; Epichloë-inoculated populations had greater aboveground biomass than Epichloë-free populations. Using biomass as a proxy, host density was correlated with peak parasite prevalence, but Epichloë still increased peak parasite prevalence after controlling for the effect of biomass. Together, these results suggest that within-host microbial interactions can impact disease at the population level. Further, while Epichloë is clearly a mutualist of tall fescue, it may not be a defensive mutualist in relation to Rhizoctonia solani.
Introduction
Host organisms are commonly infected by defensive symbionts, which can interact with parasites to protect their hosts (reviewed in Clay, 2014; Hopkins et al., 2017). These defensive symbionts can have impacts on disease at the individual and population levels (Hopkins et al., 2017; O’Keeffe et al., 2017). As the ubiquity of diverse within-host microbial communities has come to be realized, a challenge has been to link within- and between-host levels of disease dynamics (Clay et al., 2020). Here, we address this challenge by investigating the within- and between-host impacts of a defensive symbiont of a grass host on the severity and spread of a fungal parasite under field conditions.
Defensive symbionts can dramatically impact the survivorship, growth, and reproduction of parasites infecting the same host individual (Arnold et al., 2003; Costello et al., 2012; Clay, 2014; Santhanam et al., 2015; Hopkins et al., 2017). Within a host, defensive symbionts may prime a host immune response to parasites (Selosse et al., 2014) or directly interfere with an invading parasite (Gerardo and Parker, 2014). For example, systemic fungal endophytes of grasses can produce antimicrobial compounds that may reduce severity of disease caused by a parasite on a plant individual (Clay et al., 1989). Through such mechanisms, defensive symbionts can affect host susceptibility, parasite growth and replication, and subsequent parasite disease severity (Arnold et al., 2003; Oliver et al., 2005; Hussain et al., 2013).
Interactions among coinfecting symbionts within host individuals can have implications that scale up to populations (Cattadori et al., 2008; Telfer et al., 2010). Defensive symbionts can impact the growth and reproduction of parasites within a host, and within-host accumulation is often directly or indirectly linked to between-host transmission (Wintermantel et al., 2008; Tollenaere et al., 2016). Defensive symbionts may therefore be an important driver of epidemiological dynamics, which can have impacts on ecosystem function (Paseka et al., 2020). Yet, how within-host dynamics of defensive symbionts and parasites scale to impacts across host individuals remains an important frontier in disease ecology (Viney and Graham, 2013; Ezenwa and Jolles, 2015; Johnson et al., 2015).
There is a growing literature on how the defensive symbionts and other fungal species that grow within grass hosts interact with foliar fungal parasites to impact disease. Systemic fungal endophytes of grass hosts are considered defensive mutualists under most ecological conditions (Saikkonen et al., 2016). By modulating host defenses and/or producing alkaloids and other diverse toxins, these endophytes can suppress a wide range of fungal parasites under laboratory conditions (Tian et al., 2008; Pańka et al., 2013; Saikkonen et al., 2013; Panaccione et al., 2014; Card et al., 2021). Yet, a series of inoculation experiments under controlled settings found that while the systemic fungal endophyte, Epichloë coenophiala, did not directly impact within-host growth of fungal parasite, Rhizoctonia solani, it did so indirectly when the two species were coinfected with another fungal parasite, Colletotrichum cereale (O’Keeffe et al., 2021b). Further, within-host interactions among R. solani and coinfecting fungal parasites have been shown to scale up to impact transmission of parasites to host individuals (Halliday et al., 2017).
To investigate the impacts of a defensive symbiont on a parasite across levels of ecological organization, we conducted a field mesocosm experiment on a vertically transmitted fungal endophyte, E. coenophiala, the facultative fungal parasite R. solani, and a host grass, tall fescue (Lolium arundinaceum). We established experimental populations of Epichloë-inoculated and Epichloë-free plants in field mesocosms, inoculated them with the parasite, then performed repeated surveys of parasite damage on leaves. We provide evidence that while Epichloë clearly confers a benefit to its host at the host-individual level by increasing host aboveground biomass production, this endophyte can have a contrasting impact on parasite transmission at the host-population level.
Materials and Methods
Study System
Within a grass host, tall fescue (Lolium arundinaceum), we investigated the interaction between two fungal symbionts: the parasite Rhizoctonia solani and the vertically transmitted systemic endophyte Epichloë coenophiala. R. solani is a facultative parasite, as it can persist in the soil as a saprobe. As a necrotrophic parasite, it extracts resources by killing host cells. Rhizoctonia solani is transmitted primarily by hyphae and sclerotia, and symptoms of R. solani on tall fescue can be observed as light brown lesions, of irregular shape, surrounded by dark brown borders. In contrast, E. coenophiala is considered a mutualist under most ecological conditions (Saikkonen et al., 2016) and is vertically transmitted by seed. While E. coenophiala consistently acts as a defensive mutualist with regard to herbivory, E. coenophiala can vary in its impact on parasites (Potter, 1980, 1982; Liu et al., 2006; Saikkonen et al., 2013). Empirical evidence for the direction of the interaction between Epichloë endophytes and Rhizoctonia parasites varies (Pańka et al., 2013; Halliday et al., 2017; O’Keeffe et al., 2021b).
Experimental Design and Setup
We investigated how within-host microbial interactions impact parasite transmission by conducting two field mesocosm experiments. This experiment was conducted at Widener Farm, an old field of the Duke Forest Teaching and Research Laboratory (Orange County, NC, United States), during the summer of 2018. This old field produced row crops until 1996 and has since been mowed to produce hay. During the 2013–2017 growing seasons, surveys of the tall fescue population at this site showed that symptoms from parasite, R. solani, began appearing on leaves in June or July, peaked in prevalence in August and September, and decreased in prevalence over the fall months (Halliday et al., 2017). Because the peak of this parasite epidemic at this site occurred in August in previous years (Halliday et al., 2017), we conducted each experiment during that time period in subsequent years, 2017 and 2018. While the overall design of each experiment was similar in 2017 and 2018, there were a few key differences and notably, parasite transmission was more successful in the 2018 experiment (Supplementary Figure S1). Owing to the relative lack of transmission in 2017 (for example, the peak parasite prevalence in 2018, averaged across all populations, was 739.6% higher than the peak parasite prevalence in 2017), we report the 2017 transmission methods and results in the supplement (Supplementary Methods and Supplementary Table S1), and here in the main text, we report the 2018 methods and results.
To test how the endophyte affects parasite spread across a host population, we manipulated the presence of E. coenophiala at the level of the host population. We planted a total of 26 populations, and each population was contained within a 45-inch (1.14 meter)-diameter plastic wading pool (Summer Escapes) to limit R. solani inoculum coming from the environment. Each population was randomly assigned one of two treatments: E. coenophiala-inoculated or E. coenophiala-free (herein referred to as Epichloë-inoculated and Epichloë-free). In total, there were 15 Epichloë-inoculated and 11 Epichloë-free populations. Two randomly selected populations in each Epichloë treatment (four total) were not inoculated with the parasite and served as experimental controls.
Each population consisted of 13 plants: one plant in the center of the population (which would ultimately be inoculated with the parasite), and 12 plants surrounding the central plant at distances of 12, 24, and 36 cm away (four plants at each distance; Supplementary Figure S2). The 338 plants within the experiment were propagated from Epichloë-free or Epichloë-inoculated seed produced by Tim Phillips at the University of Kentucky and the Noble Research Institute in Ardmore Oklahoma, respectively. All seed was from variety KY-31. Seed was germinated on 25 June 2018 and grown in a greenhouse for 6 weeks. The greenhouse temperature was kept between 19.7°C and 22.2°C, and light was supplemented between 9 AM and 7 PM if natural light fell below 350 W/m2. All plants except for the central plants were transplanted into the contained field mesocosm experiment on Monday, 6 August 2018, 6 weeks after germination. Each population consisted of plants belonging to the same endophyte category (all Epichloë-inoculated or Epichloë-free) except all central plants were Epichloë-free. Plants were randomly assigned to one of the populations, and locations of the plants within the populations were also randomized. The populations were fully randomized in a 2 × 13 layout, with narrow paths separating populations (Supplementary Figure S2). The plants were given 4 days to acclimate to the field prior to the introduction of the parasite.
The plants that would ultimately be planted in the central position of the populations were transferred to growth chambers on 8 August 2018 and inoculated with an isolate of the parasite that was cultured in 2015 from a leaf lesion on a tall fescue plant in the same field as this experiment. Once in axenic culture, plugs of the leading edge of the culture were stored in mineral oil and potato dextrose broth in a −80°C freezer. We plated these plugs on potato dextrose agar and the resulting growth served as the source of inoculum for this experiment. Inoculum consisted of a 6-mm-diameter plug of potato dextrose agar with the leading edge of the parasite culture placed directly at the base of a leaf. Parasite infection success depends on a humid environment. To maintain moisture at the site of inoculation, a cotton ball wet with sterile water was placed over the inoculum, secured with tin foil and parafilm. The inoculated plants were placed in a growth chamber (Percival PGC-6L (Perry, Iowa)) for 2 days with a 12-h light/12-h dark cycle set at 28°C, and humidity was maintained at approximately 95% with humidifiers (Vicks V5100-N Ultrasonic Humidifier) on each shelf of the growth chambers. In addition to parasite-inoculated plants, four plants were mock-inoculated with plugs of potato dextrose agar without R. solani mycelia. After 2 days, all plants inoculated with R. solani exhibited parasite symptoms and were transplanted into the field mesocosm experiment on 10 August 2018. One mock-inoculated plant was transplanted into each of the four experimental control populations.
Data Collection
Following the placement of the central inoculated plant, twice a week, for 4 weeks, seven random leaves per plant were nondestructively selected and observed for the presence or absence of damage caused by the parasite, as well as any other parasite damage. Specifically, leaves were surveyed 4, 8, 11, 14, 18, 22, 25, and 28 days after parasite inoculation (eight surveys total). Each leaf was surveyed for the presence of any damage caused by parasites, herbivores, or abiotic sources. The symptoms of other fungal parasites of tall fescue in this location caused symptoms that are easily distinguished from those caused by R. solani (O’Keeffe et al., 2021a).
To measure disease severity over time, percent leaf area damaged by the parasite was quantified on individual leaves on one tagged tiller per plant once a week. On each leaf, the initial date of symptomatic infection by the parasite was recorded, and severity was estimated by visually comparing leaves to reference images of leaves of known infection severity (Mitchell et al., 2002, 2003; Halliday et al., 2017). Over the course of the experiment, three severity surveys were conducted (8, 14, and 22 days after parasite inoculation).
At the conclusion of the experiment, we collected and frozen (−20°C freezer) inch-long cross-sections of two tillers per plant to confirm endophyte presence. Endophyte infection was tested via immunoblot (Agrinostics Ltd. Co, Watkinsville, GA, United States). Additionally, aboveground biomass was harvested, dried, and weighed.
Data Analysis
Epichloë-inoculated seed did not always result in endophyte detection in the tillers tested at the end of the experiment. Overall, we detected the endophyte in aboveground tissue of 42% of Epichloë-inoculated plants. This resulted in variation in endophyte prevalence among the Epichloë-inoculated populations (minimum: 15.4%; maximum: 69.2%). We therefore analyzed our data in two separate ways: with endophyte treatment (two levels: Epichloë-free or Epichloë-inoculated) as a predictor, or with endophyte prevalence (continuous variable from 0 to 1) as a predictor.
Control populations (in which the central plant was mock-inoculated) did not exhibit symptoms of the parasite, confirming that mesocosm populations were not infected from environmental sources of inoculum. These control populations were therefore excluded from analyses.
The models based on parasite prevalence reported in the main text only include surveys until each population’s peak parasite prevalence because we were interested in how endophyte presence impacts epidemic spread, which is no longer happening after the peak. We report the results of models based on parasite prevalence with all surveys in the supplement (Supplementary Table S2; Supplementary Figure S3). All analyses were performed in R, version 3.6.1.
Leaves were analyzed as host individuals because each parasite infection is restricted to a single leaf (as done by Halliday et al., 2017; O’Keeffe et al., 2021b). As described in more detail above, we surveyed leaves in two ways; in weekly severity surveys, we estimated the leaf area damaged on a sample of leaves, and in twice weekly prevalence surveys, we sampled leaves for the presence/absence of disease symptoms. To summarize disease progression over time, area under the disease progress stairs (AUDPS) was calculated for each population using the audps function within the agricolae package (version 1.3; de Mendiburu and Yaseen, 2020). AUDPS estimated the integration of the development of disease progress experienced by each population by adding together polygon steps between each time point (Simko and Piepho, 2011). Using data from each of the two sets of surveys, we calculated AUDPS based on two different measures of disease. Specifically, we calculated AUDPS with the average leaf area damaged across all leaves surveyed within a population at each time point, and we separately calculated AUDPS with the prevalence of the parasite at each time point. When calculated with prevalence data, AUDPS was log-transformed to achieve homoscedasticity and normality of residuals. We investigated whether and how endophyte treatment affected these estimates of disease progression over time with a linear model.
To further evaluate the magnitude of epidemics, we investigated measurements of parasite prevalence repeated over time using linear mixed effects models. Data on proportion of leaves infected with the parasite were log-transformed to achieve homoscedasticity and normality of residuals. Using the nlme package (version 3.1) for linear mixed effects models, we modeled parasite prevalence within a population at a given time with a linear mixed effects model that included Epichloë inoculation treatment and a third-order polynomial of days after infection, as well as their interaction, as predictors (Pinheiro et al., 2013). We determined the appropriate polynomial to utilize using AIC. We included random slopes to account for repeated measures of the populations.
We additionally evaluated whether Epichloë inoculation treatment affected peak parasite prevalence. We quantified peak parasite prevalence as the highest proportion of leaves infected with the parasite at a given time point at the population level. We tested whether the Epichloë inoculation treatment affected peak parasite prevalence with a linear model that included Epichloë inoculation treatment as the predictor.
Under density-dependent transmission, the contact rate between susceptible and infected individuals depends on the host population density; transmission rate therefore increases with density. While host population density (here, the number of leaves in a population) was not measured in the 2018 experiment, aboveground biomass was measured at the conclusion of the experiment. To investigate whether host population density significantly correlated with biomass, we used data from the 2017 field mesocosm experiment in which host density was measured explicitly. We used a ranged major axis regression model (implemented in lmodel2, version 1.7; Legendre and Oksanen, 2018) to investigate whether there was a correlation between total aboveground biomass of plants and the total number of leaves in a given population at the end of the experiment. Both biomass and total leaves were log-transformed. As noted, there was relatively low transmission in the 2017 experiment, but this correlative analysis only considers biomass and number of leaves; it does not incorporate Epichloë treatment or disease data. Based on that correlation, we then used aboveground biomass as a proxy for host density of each population. Specifically, to test whether the effect of the Epichloë inoculation treatment on parasite peak prevalence was due to variation in host density, we added total aboveground biomass to the model as a covariate.
Results
Epichloë-inoculated populations tended to experience more disease than Epichloë-free populations. Epichloë-inoculated populations experienced 8.3% higher disease integrated over time as measured by AUDPS calculated with disease prevalence (Figure 1; Table 1; p = 0.051), with much greater variation evident among Epichloë-free populations. Results when AUDPS was calculated with disease severity were generally consistent, although statistical support was weaker (Supplementary Results; Supplementary Table S3; Supplementary Figures S4, S5). A mixed model of prevalence over time complemented these results, as the Epichloë inoculation treatment tended to increase parasite prevalence over time, though this finding had weak statistical support (Figure 2A; Table 2; p = 0.07). The Epichloë inoculation treatment did clearly increase the peak parasite prevalence. Specifically, the Epichloë-inoculated populations had a 27% higher peak prevalence than Epichloë-free populations, with mean peak parasite prevalences of 0.43 and 0.34, respectively (Figure 2B; Table 3; p = 0.007). Together, these results suggest that while this endophyte may impact spread of a parasite across a population over time, it most clearly leads to higher parasite prevalence at the peak of an epidemic.
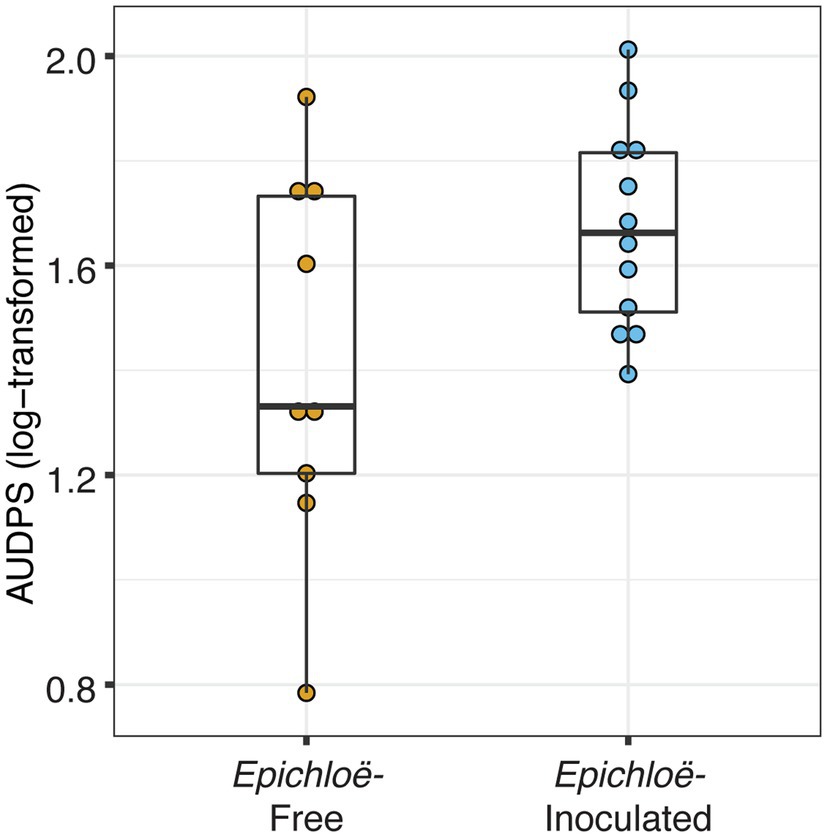
Figure 1. Epichloë-inoculated populations had greater disease prevalence integrated over time. Disease prevalence was integrated over time for each population as log-transformed AUDPS (area under the disease progress stairs). AUDPS was estimated using disease prevalence over the eight surveys until each population’s peak. Boxplots show median, 25th, and 75th percentile, with dots showing data for each population replicate. Whiskers extend to the lowest and highest values no further than ± 1.5 times the inter-quartile range.
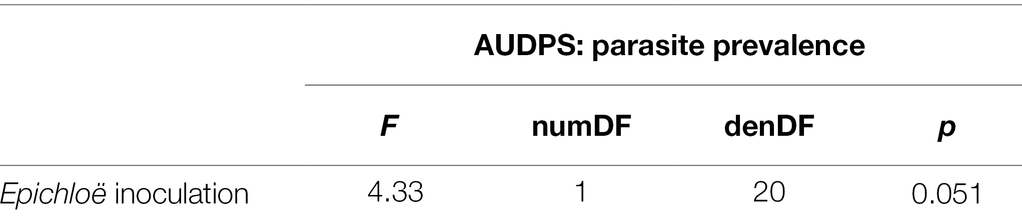
Table 1. Epichloë-inoculated populations tended to have heavier disease prevalence integrated over time as log-transformed AUDPS, though this difference had weak statistical support.
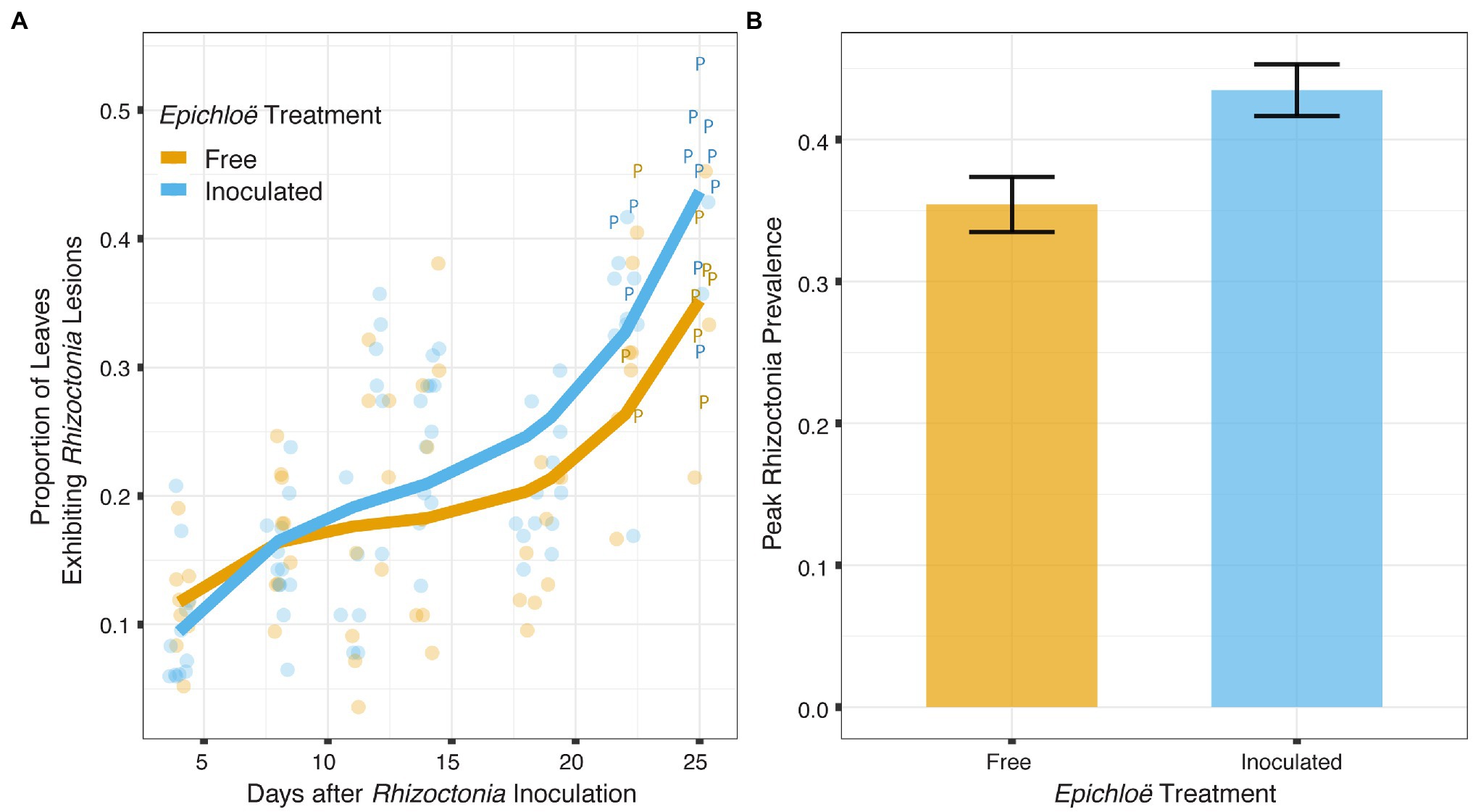
Figure 2. Epichloë-inoculated populations tended to have greater disease prevalence over time, though this difference had weak statistical support in a linear mixed model. More clearly, Epichloë inoculation increased peak parasite prevalence experienced by a population. (A) Bold lines are model-predicted means of parasite prevalence over the course of 4 weeks post-inoculation with the parasite for populations in which Epichloë was inoculated (blue) and populations in which Epichloë was absent (orange). The peak parasite prevalence of each population is indicated by the letter “P.” (B) Epichloë-inoculated populations had 8.6% higher peak parasite prevalence than Epichloë-free populations. Bars indicate mean peak parasite prevalence and error bars are ± 1 SE.
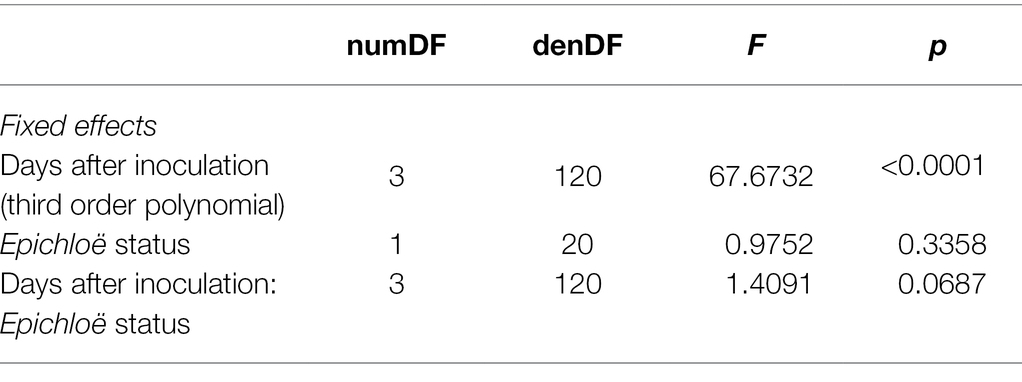
Table 2. Epichloë-inoculated populations tended to have greater disease prevalence tracked over time in a linear mixed model, though this difference had weak statistical support.

Table 3. Epichloë inoculation clearly increased peak parasite prevalence experienced by a population.
In addition to impacting parasite prevalence and disease severity, this endophyte also impacted host population aboveground biomass. Specifically, populations with higher prevalence of Epichloë also had higher aboveground biomass (Figure 3; Table 4; p = 0.047). Motivated by the expectation that transmission of the parasite is density dependent, we investigated whether higher biomass correlated with higher numbers of leaves in a population (i.e., host population density). As counting all leaves was not feasible in the 2018 experiment, we used data from the 2017 experiment, in which we counted all leaves in each population. In the data from 2017, we investigated whether there was a correlation between the aboveground biomass of the plant population measured at the end of the experiment and the total number of leaves surveyed at the end of the experiment. Population-level aboveground biomass and the number of leaves in a population were clearly positively correlated in 2017 (Figure 4; Table 5; Marginal R2 = 0.145; p = 0.045). If we assume that the correlation between population biomass and leaf number from 2017 also held in 2018, then that suggests that in analyzing the 2018 experiment, host population aboveground biomass can be used a proxy for host population density.
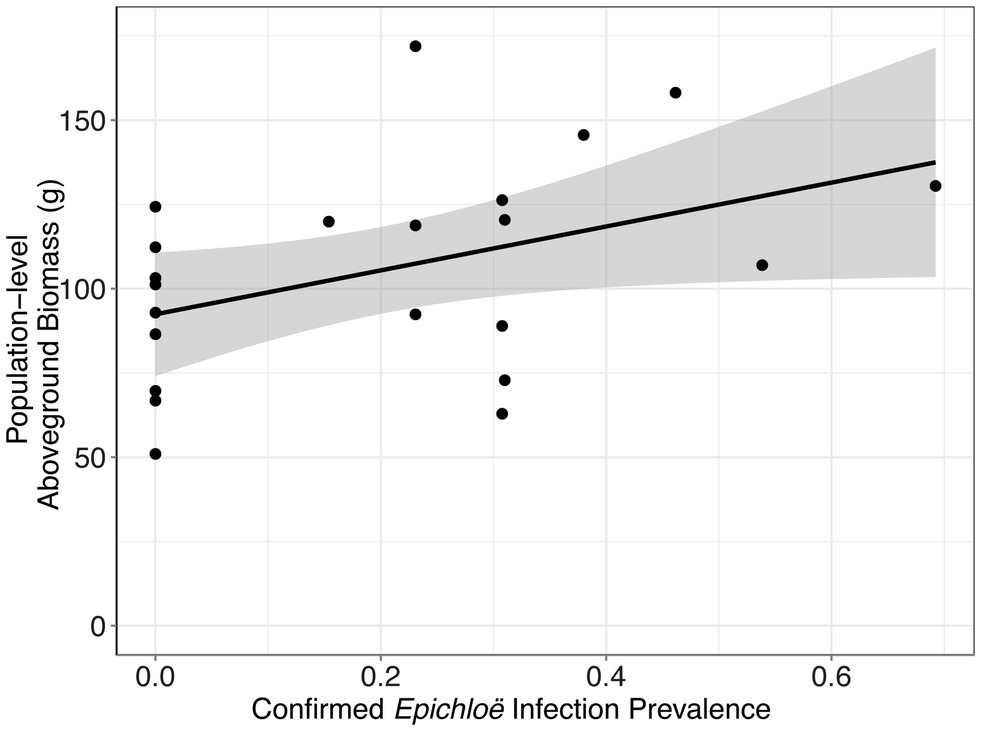
Figure 3. Epichloë infection prevalence was associated with greater host aboveground biomass at the population level. Each point represents a host population, and the bold line represents the best fit linear model. At the population level, confirmed Epichloë prevalence was clearly positively associated with population-level host aboveground biomass at the end of the experiment (p = 0.047).

Table 4. Epichloë prevalence was associated with greater final aboveground biomass of the host population.
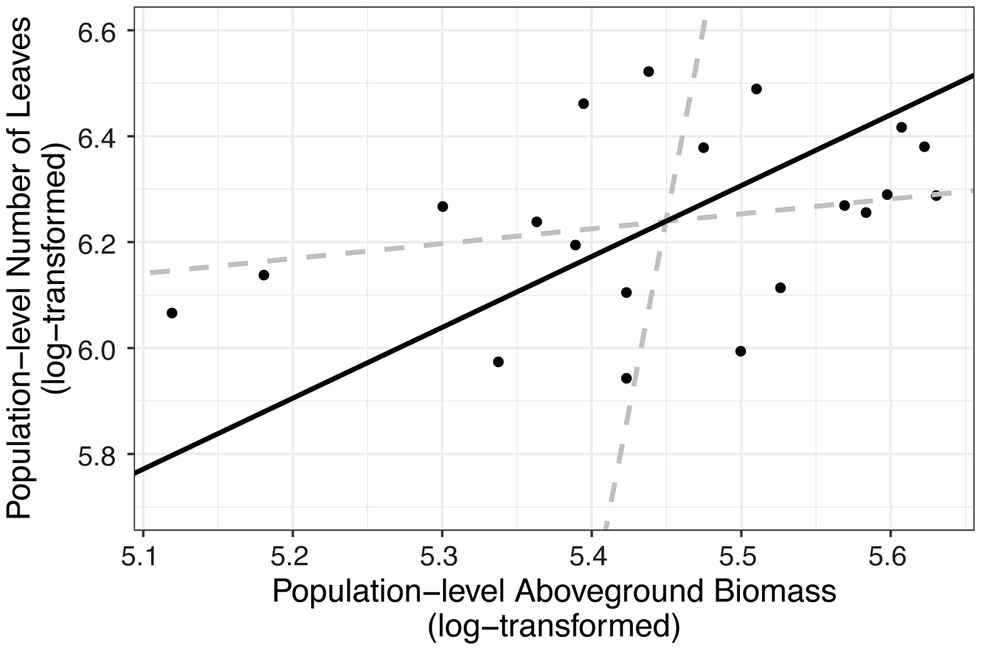
Figure 4. Number of leaves in a population was correlated with dry aboveground biomass of a population. We summed the total number of leaves and the biomass (in grams) of each population (the level at which other analyses were performed). Each point represents a host population in the 2017 experiment. The black solid line indicates ranged major axis fit, and the gray dashed lines indicate 95% CIs. The total number of leaves (log-transformed) at the end of the experiment was correlated with log-transformed dry aboveground biomass (R2 = 0.145, permutation test, p = 0.045).

Table 5. Aboveground biomass was correlated with number of leaves at the population level (permutation test of ranged major axis regression).
We then investigated whether higher levels of disease experienced by Epichloë-inoculated populations were driven by higher host densities. Based on the analysis of data from 2017, we used population-level aboveground biomass as a proxy for host population density and tested whether biomass accounted for the effect of Epichloë treatment on peak prevalence. Biomass and Epichloë treatment explained approximately 56% of variance in peak parasite prevalence. Biomass was positively correlated with peak parasite prevalence (p = 0.006), and independent of this association (interaction p = 0.87), Epichloë inoculation increased peak prevalence (p = 0.01; Figure 5; Table 6). While biomass did contribute to peak parasite prevalence, it did not account for the effect of the Epichloë treatment.
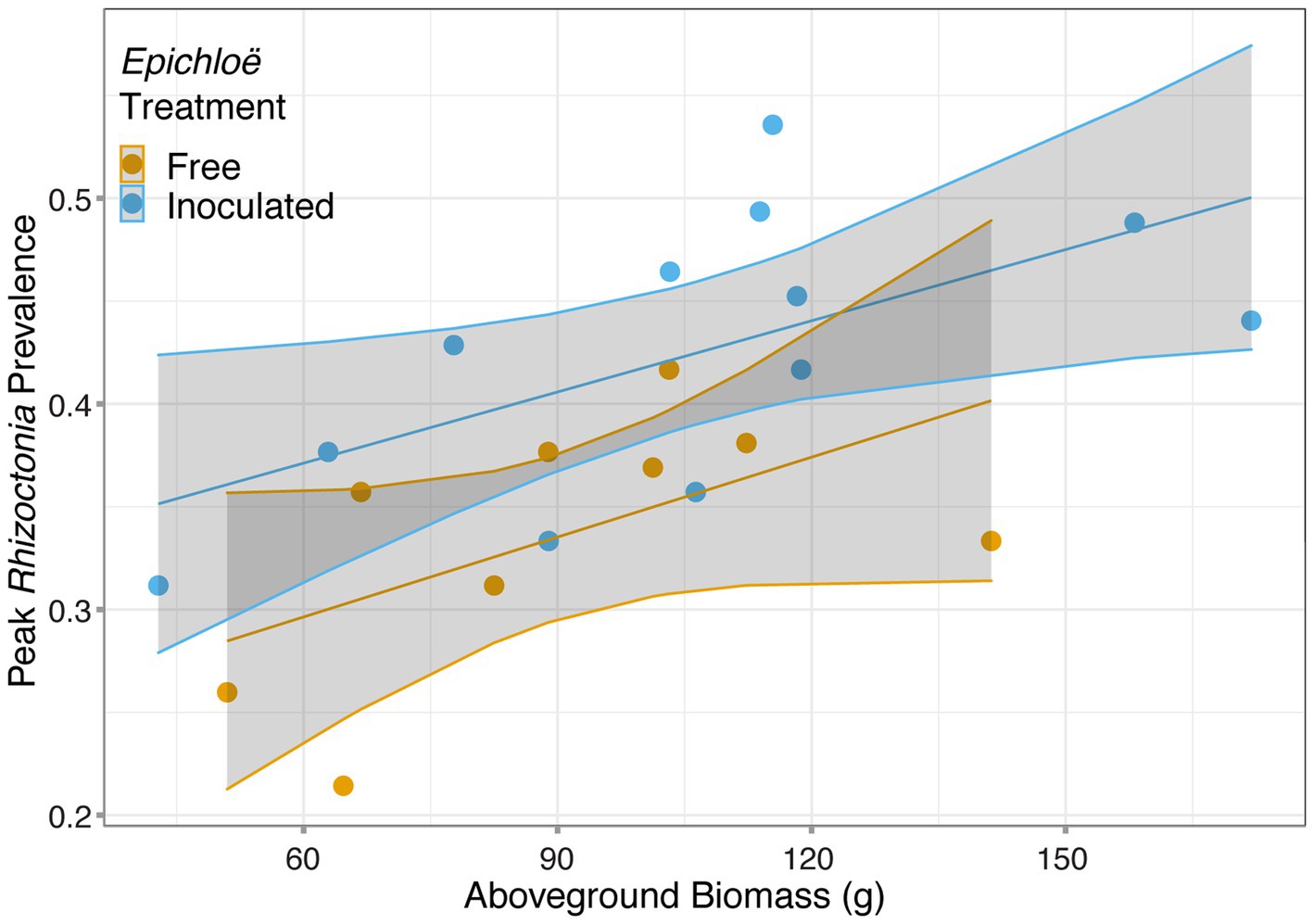
Figure 5. Peak Rhizoctonia prevalence was correlated with dry aboveground biomass. Each population (represented with a point) was either inoculated with Epichloë (blue) or free of Epichloë (orange). Biomass predicted peak parasite prevalence (p = 0.006), and independent of this effect, Epichloë inoculation increased peak prevalence by 0.07 (p = 0.01).
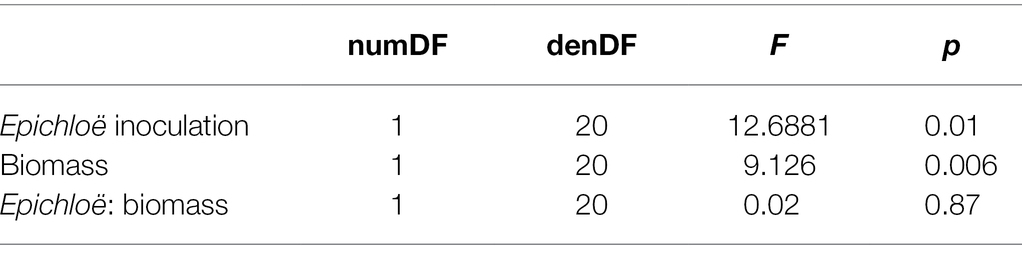
Table 6. There was a clear effect of Epichloë inoculation on peak prevalence, even after accounting for the association of biomass with prevalence.
Discussion
Our study provides experimental evidence that population-level disease dynamics can be impacted by the presence of a mutualist. Specifically, we investigated how parasite spread through a host population under field conditions responds to the presence of a mutualistic systemic endophyte under field conditions. We found that populations of tall fescue inoculated with the endophyte, E. coenophiala, counterintuitively experienced a higher peak prevalence of parasite, R. solani, over the course of the experimental epidemic.
The mutualistic relationship between cool-season grasses and vertically transmitted systemic fungal endophytes related to Epichloë has been studied extensively. While Epichloë endophyte infection has been shown to benefit host plants by increasing resistance to herbivores and seed predators, as well as providing protection against abiotic stressors (Saikkonen et al., 1998; Kauppinen et al., 2016), evidence for defending against infectious disease is less consistent (Potter, 1980, 1982; Liu et al., 2006; Saikkonen et al., 2013). In this experiment, we found that infection with E. coenophiala resulted in an increase in aboveground biomass, but there was no support for E. coenophiala limiting disease progression. Epichloë-inoculated populations actually experienced R. solani epidemics with higher peak prevalences. Our results are consistent with studies reporting no effect (Burpee and Bouton, 1993; Halliday et al., 2017; O’Keeffe et al., 2021b) or a facilitative effect of the endophyte on a parasite (Wäli et al., 2006; Halliday et al., 2017). Vertically transmitted fungal endophytes can impact fungal parasites via resource competition and changes in host immunity, which depends on parasite-feeding strategies (Saikkonen et al., 2013). While we expected that R. solani would be inhibited by E. coenophiala given previous findings that E. coenophiala most often suppressed disease caused by a relative of R. solani (Pańka et al., 2013), the direction of the effect of these endophytes on parasites likely depends on host genotype and environmental conditions (Krauss et al., 2007; Pańka et al., 2013). Further experimentation is needed to determine the mechanism underlying the potential facilitation of R. solani by E. coenophiala.
Epichloë-inoculated populations tended to have greater disease prevalence over time, though this difference had weak statistical support. More clearly, Epichloë inoculation increased peak parasite prevalence experienced by a population. We hypothesized that under density-dependent transmission, the benefit to the host individual of increased aboveground biomass, which in this case, correlated with host density (here, number of leaves), may result in a higher contact rate between hosts and consequently higher parasite peak prevalence. While biomass and peak parasite prevalence were significantly positively correlated, consistent with density dependent transmission, our results suggest that Epichloë impacted peak parasite prevalence beyond effects of biomass. One possible explanation is that Epichloë infection may have altered the growth of Rhizoctonia or other processes within host individuals that scaled up to the observed effect on peak prevalence in the host population. Alternatively, given that there is evidence that parasites can impact their host’s biomass (Cordovez et al., 2017; Preston and Sauer, 2020), directly accounting for host density in models (rather than with biomass as a proxy) may more completely account for the impact of Epichloë on peak parasite prevalence.
There is growing evidence that the direction and magnitude of the consequences of within-host interactions are strongly affected by environmental context (Leung et al., 2018; Tracy et al., 2018). Our study provides a contribution to this understanding. By interrogating the impacts of an interaction between a systemic endophyte and parasite on parasite transmission at the population level, we expanded upon previous work which found no support for this hypothesized mutualist limiting parasite growth under controlled settings (O’Keeffe et al., 2021b). Foliar fungal parasites have been studied extensively and can serve as a suitable model system to investigate microbiome/parasite interactions under field settings. Here, we show that field mesocosm experiments offer the ability to investigate the effect of within-host microbial interactions on parasite spread.
Within-host microbial interactions can influence natural epidemics in complex ways (Mordecai et al., 2015; Clay et al., 2019). Across hosts, we found that populations inoculated with a mutualist counterintuitively experienced higher peak prevalence of this parasite. These results suggest that within-host interactions among parasites and non-pathogenic microbes can impact epidemic dynamics, and we propose that field mesocosm experiments can yield important insight into disease dynamics across populations under field settings.
Data Availability Statement
The original contributions presented in the study are publicly available. This data can be found here: https://github.com/krokeeffe12/OKeeffe_fieldmesocosm.
Author Contributions
KO, BW, and CM contributed to the conception and design of the study. KO and BW collected data. KO analyzed the data and wrote the first draft of the manuscript. All authors contributed to the article and approved the submitted version.
Funding
This work was supported by the NSF-USDA joint program in Ecology and Evolution of Infectious Diseases (USDA-NIFA AFRI grant 2016-67013-25762). KO was supported by a graduate research fellowship from the National Science Foundation.
Conflict of Interest
The authors declare that the research was conducted in the absence of any commercial or financial relationships that could be construed as a potential conflict of interest.
Publisher’s Note
All claims expressed in this article are solely those of the authors and do not necessarily represent those of their affiliated organizations, or those of the publisher, the editors and the reviewers. Any product that may be evaluated in this article, or claim that may be made by its manufacturer, is not guaranteed or endorsed by the publisher.
Acknowledgments
We thank Marc Cubeta for helpful conversations about the biology of Rhizoctonia, James Umbanhowar for help with statistics, and Fletcher Halliday, Ignazio Carbone, Corbin Jones, and Elizabeth Shank for helpful comments on experimental design and analyses. We also thank Brooklynn Joyner, Charlie Muirhead, Storm Crews, and Julie Long for help in the field and Tim Phillips and Rebecca McCulley for the tall fescue seed.
Supplementary Material
The Supplementary Material for this article can be found online at: https://www.frontiersin.org/articles/10.3389/fmicb.2022.824211/full#supplementary-material
References
Arnold, A. E., Mejía, L. C., Kyllo, D., Rojas, E. I., Maynard, Z., Robbins, N., et al. (2003). Fungal endophytes limit pathogen damage in a tropical tree. Proc. Natl. Acad. Sci. U. S. A. 100, 15649–15654. doi: 10.1073/pnas.2533483100
Burpee, L. L., and Bouton, J. H. (1993). Effect of eradication of the endophyte Acremonium coenophialum on epidemics of Rhizoctonia blight in tall fescue. Plant Dis. 77, 157–159.
Card, S. D., Bastías, D. A., and Caradus, J. R. (2021). Antagonism to plant pathogens by Epichloë fungal Endophytes: a review. Plan. Theory 10. doi: 10.3390/plants10101997
Cattadori, I. M., Boag, B., and Hudson, P. J. (2008). Parasite co-infection and interaction as drivers of host heterogeneity. Int. J. Parasitol. 38, 371–380. doi: 10.1016/j.ijpara.2007.08.004
Clay, K. (2014). Defensive symbiosis: a microbial perspective. Funct. Ecol. 28, 293–298. doi: 10.1111/1365-2435.12258
Clay, K., Cheplick, G. P., and Marks, S. (1989). Impact of the fungus Balansia henningsiana on Panicum agrostoides: frequency of infection, plant growth and reproduction, and resistance to pests. Oecologia 80, 374–380. doi: 10.1007/BF00379039
Clay, P. A., Cortez, M. H., Duffy, M. A., and Rudolf, V. H. W. (2019). Priority effects within coinfected hosts can drive unexpected population-scale patterns of parasite prevalence. Oikos 128, 571–583. doi: 10.1111/oik.05937
Clay, P. A., Duffy, M. A., and Rudolf, V. H. W. (2020). Within-host priority effects and epidemic timing determine outbreak severity in co-infected populations. Proc. R. Soc. B Biol. Sci. 287:20200046. doi: 10.1098/rspb.2020.0046
Cordovez, V., Mommer, L., Moisan, K., Lucas-Barbosa, D., Pierik, R., Mumm, R., et al. (2017). Plant phenotypic and transcriptional changes induced by volatiles from the fungal root pathogen Rhizoctonia solani. Front. Plant Sci. 8:1262. doi: 10.3389/fpls.2017.01262
Costello, E. K., Stagaman, K., Dethlefsen, L., Bohannan, B. J. M., and Relman, D. A. (2012). The application of ecological theory toward an understanding of the human microbiome. Science 336, 1255–1262. doi: 10.1126/science.1224203
de Mendiburu, F., and Yaseen, M. (2020). Agricolae: Statistical Procedures for Agricultural Research. R package version 1.4.0, Available at: https://myaseen208.github.io/agricolae/
Ezenwa, V. O., and Jolles, A. E. (2015). Opposite effects of anthelmintic treatment on microbial infection at individual versus population scales. Science 347, 175–177. doi: 10.1126/science.1261714
Gerardo, N. M., and Parker, B. J. (2014). Mechanisms of symbiont-conferred protection against natural enemies: an ecological and evolutionary framework. Curr. Opin. Insect. Sci. 4, 8–14. doi: 10.1016/j.cois.2014.08.002
Halliday, F. W., Umbanhowar, J., and Mitchell, C. E. (2017). Interactions among symbionts operate across scales to influence parasite epidemics. Ecol. Lett. 20, 1285–1294. doi: 10.1111/ele.12825
Hopkins, S. R., Wojdak, J. M., and Belden, L. K. (2017). Defensive symbionts mediate host-parasite interactions at multiple scales. Trends Parasitol. 33, 53–64. doi: 10.1016/j.pt.2016.10.003
Hussain, M., Lu, G., Torres, S., Edmonds, J. H., Kay, B. H., Khromykh, A. A., et al. (2013). Effect of Wolbachia on replication of West Nile virus in a mosquito cell line and adult mosquitoes. J. Virol. 87, 851–858. doi: 10.1128/JVI.01837-12
Johnson, P. T. J., de Roode, J. C., and Fenton, A. (2015). Why infectious disease research needs community ecology. Science 349, 1259504-1–1259504-9. doi: 10.1126/science.1259504
Kauppinen, M., Saikkonen, K., Helander, M., Pirttilä, A. M., and Wäli, P. R. (2016). Epichloë grass endophytes in sustainable agriculture. Nat. Plants 2:15224. doi: 10.1038/nplants.2015.224
Krauss, J., Harri, S. A., Bush, L., Power, S. A., and Muller, C. B. (2007). “Fungal grass endophytes, grass cultivars, nitrogen deposition and the associations with colonizing insects,” in NZGA: Research and Practice Series. eds. A. J. Popay and E. R. Thom (Christchurch, NZ: New Zealand Grassland Association). 13, 53–57.
Legendre, P., and Oksanen, M. J. (2018). Package ‘lmodel2’. Available at: https://CRAN.R-Project.Org/Package=Lmodel2 (Accessed November 1, 2021).
Leung, J. M., Budischak, S. A., Chung The, H., Hansen, C., Bowcutt, R., Neill, R., et al. (2018). Rapid environmental effects on gut nematode susceptibility in rewilded mice. PLoS Biol. 16:e2004108. doi: 10.1371/journal.pbio.2004108
Liu, Y., Kang, Z., and Buchenauer, H. (2006). Ultrastructural and immunocytochemical studies on effects of barley yellow dwarf virus–infection on Fusarium head blight, caused by Fusarium graminearum, in wheat plants. J. Phytopathol. 154, 6–15. doi: 10.1111/j.1439-0434.2005.01048.x
Mitchell, C. E., Tilman, D., and Groth, J. V. (2002). Effects of grassland plant species diversity, abundance, and composition on foliar fungal disease. Ecology 83, 1713–1726. doi: 10.1890/0012-9658
Mitchell, C. E., Tilman, D., and Groth, J. V. (2003). Effects of elevated CO2, nitrogen deposition, and decreased species diversity on foliar fungal plant disease. Glob. Chang. Biol. 9, 438–451. doi: 10.1046/j.1365-2486.2003.00602.x
Mordecai, E. A., Gross, K., and Mitchell, C. E. (2015). Within-host niche differences and fitness trade-offs promote coexistence of plant viruses. Am. Nat. 187, E13–E26. doi: 10.1086/684114
O’Keeffe, K. R., Carbone, I., Jones, C. D., and Mitchell, C. E. (2017). Plastic potential: how the phenotypes and adaptations of pathogens are influenced by microbial interactions within plants. Curr. Opin. Plant Biol. 38, 78–83. doi: 10.1016/j.pbi.2017.04.014
O’Keeffe, K. R., Halliday, F. W., Jones, C. D., Carbone, I., and Mitchell, C. E. (2021a). Parasites, niche modification and the host microbiome: a field survey of multiple parasites. Mol. Ecol. 30, 2404–2416. doi: 10.1111/mec.15892
O’Keeffe, K. R., Simha, A., and Mitchell, C. E. (2021b). Indirect interactions among co-infecting parasites and a microbial mutualist impact disease progression. Proc. R. Soc. B Biol. Sci. 288:20211313. doi: 10.1098/rspb.2021.1313
Oliver, K. M., Moran, N. A., and Hunter, M. S. (2005). Variation in resistance to parasitism in aphids is due to symbionts not host genotype. Proc. Natl. Acad. Sci. U. S. A. 102, 12795–12800. doi: 10.1073/pnas.0506131102
Panaccione, D. G., Beaulieu, W. T., and Cook, D. (2014). Bioactive alkaloids in vertically transmitted fungal endophytes. Funct. Ecol. 28, 299–314. doi: 10.1111/1365-2435.12076
Pańka, D., West, C. P., Guerber, C. A., and Richardson, M. D. (2013). Susceptibility of tall fescue to Rhizoctonia zeae infection as affected by endophyte symbiosis. Ann. Appl. Biol. 163, 257–268. doi: 10.1111/aab.12051
Paseka, R. E., White, L. A., Van de Waal, D. B., Strauss, A. T., González, A. L., Everett, R. A., et al. (2020). Disease-mediated ecosystem services: pathogens, plants, and people. Trends Ecol. Evol. 35, 731–743. doi: 10.1016/j.tree.2020.04.003
Pinheiro, J., Bates, D., DebRoy, S., Sarkar, D., and Team, R. C. (2013). nlme: Linear and Nonlinear Mixed Effects Models. R Package Version, 3, 111.
Potter, L. R. (1980). The effects of barley yellow dwarf virus and powdery mildew in oats and barley with single and dual infections. Ann. Appl. Biol. 94, 11–17. doi: 10.1111/j.1744-7348.1980.tb03891.x
Potter, L. R. (1982). Interaction between barley yellow dwarf virus and rust in wheat, barley and oats, and the effects on grain yield and quality. Ann. Appl. Biol. 100, 321–329. doi: 10.1111/j.1744-7348.1982.tb01945.x
Preston, D. L., and Sauer, E. L. (2020). Infection pathology and competition mediate host biomass overcompensation from disease. Ecology 101:e03000. doi: 10.1002/ecy.3000
Saikkonen, K., Faeth, S. H., Helander, M., and Sullivan, T. J. (1998). Fungal endophytes: a continuum of interactions with host plants. Annu. Rev. Ecol. Syst. 29, 319–343. doi: 10.1146/annurev.ecolsys.29.1.319
Saikkonen, K., Gundel, P. E., and Helander, M. (2013). Chemical ecology mediated by fungal endophytes in grasses. J. Chem. Ecol. 39, 962–968. doi: 10.1007/s10886-013-0310-3
Saikkonen, K., Young, C. A., Helander, M., and Schardl, C. L. (2016). Endophytic Epichloë species and their grass hosts: from evolution to applications. Plant Mol. Biol. 90, 665–675. doi: 10.1007/s11103-015-0399-6
Santhanam, R., Luu, V. T., Weinhold, A., Goldberg, J., Oh, Y., and Baldwin, I. T. (2015). Native root-associated bacteria rescue a plant from a sudden-wilt disease that emerged during continuous cropping. Proc. Natl. Acad. Sci. 112, E5013–E5020. doi: 10.1073/pnas.1505765112
Selosse, M.-A., Bessis, A., and Pozo, M. J. (2014). Microbial priming of plant and animal immunity: symbionts as developmental signals. Trends Microbiol. 22, 607–613. doi: 10.1016/j.tim.2014.07.003
Simko, I., and Piepho, H.-P. (2011). The area under the disease progress stairs: calculation, advantage, and application. Phytopathology 102, 381–389. doi: 10.1094/PHYTO-07-11-0216
Telfer, S., Lambin, X., Birtles, R., Beldomenico, P., Burthe, S., Paterson, S., et al. (2010). Species interactions in a parasite community drive infection risk in a wildlife population. Science 330, 243–246. doi: 10.1126/science.1190333
Tian, P., Nan, Z., Li, C., and Spangenberg, G. (2008). Effect of the endophyte Neotyphodium lolii on susceptibility and host physiological response of perennial ryegrass to fungal pathogens. Eur. J. Plant Pathol. 122, 593–602. doi: 10.1007/s10658-008-9329-7
Tollenaere, C., Susi, H., and Laine, A. (2016). Evolutionary and epidemiological implications of multiple infection in plants. Trends Plant Sci. 21, 80–90. doi: 10.1016/j.tplants.2015.10.014
Tracy, A. M., Weil, E., and Harvell, C. D. (2018). Octocoral co-infection as a balance between host immunity and host environment. Oecologia 186, 743–753. doi: 10.1007/s00442-017-4051-9
Viney, M. E., and Graham, A. L. (2013). “Chapter Five: Patterns and processes in parasite co-infection,” in D. B. T.-A. Vol. 82. ed. P. Rollinson (Academic Press), 321–369. doi: 10.1016/B978-0-12-407706-5.00005-8
Wäli, P. R., Helander, M., Nissinen, O., and Saikkonen, K. (2006). Susceptibility of endophyte-infected grasses to winter pathogens (snow molds). Can. J. Bot. 84, 1043–1051. doi: 10.1139/b06-075
Wintermantel, W. M., Cortez, A. A., Anchieta, A. G., Gulati-Sakhuja, A., and Hladky, L. L. (2008). Co-infection by two criniviruses alters accumulation of each virus in a host-specific manner and influences efficiency of virus transmission. Phytopathology 98, 1340–1345. doi: 10.1094/PHYTO-98-12-1340
Keywords: within-host microbial interactions, species interactions, co-infection, mesocosm experiment, transmission, disease ecology, plant parasites
Citation: O’Keeffe KR, Wheeler BT and Mitchell CE (2022) A Microbial Mutualist Within Host Individuals Increases Parasite Transmission Between Host Individuals: Evidence From a Field Mesocosm Experiment. Front. Microbiol. 13:824211. doi: 10.3389/fmicb.2022.824211
Edited by:
Posy Elizabeth Busby, Oregon State University, United StatesReviewed by:
Laura Ellen Rose, Heinrich Heine University of Düsseldorf, GermanyGeorgia C. Drew, University of Oxford, United Kingdom
Copyright © 2022 O’Keeffe, Wheeler and Mitchell. This is an open-access article distributed under the terms of the Creative Commons Attribution License (CC BY). The use, distribution or reproduction in other forums is permitted, provided the original author(s) and the copyright owner(s) are credited and that the original publication in this journal is cited, in accordance with accepted academic practice. No use, distribution or reproduction is permitted which does not comply with these terms.
*Correspondence: Kayleigh R. O’Keeffe, a29rZWVmZmUxMkBnbWFpbC5jb20=