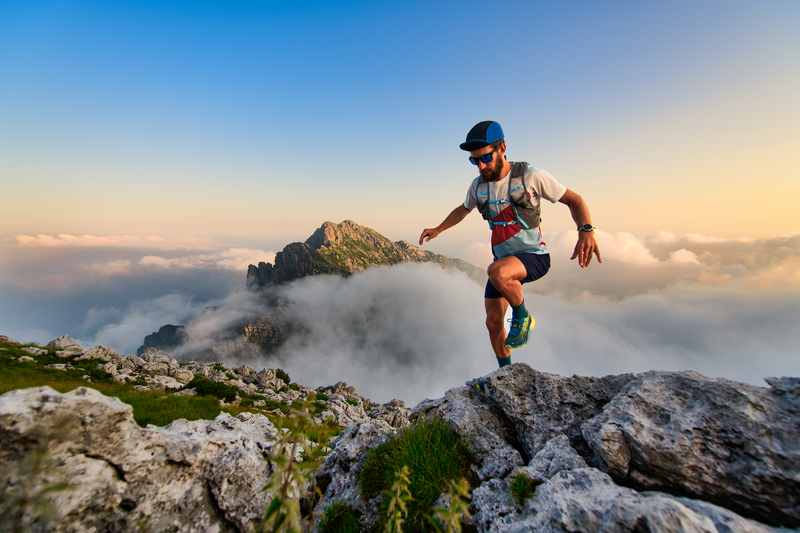
94% of researchers rate our articles as excellent or good
Learn more about the work of our research integrity team to safeguard the quality of each article we publish.
Find out more
ORIGINAL RESEARCH article
Front. Microbiol. , 03 March 2022
Sec. Microbe and Virus Interactions with Plants
Volume 13 - 2022 | https://doi.org/10.3389/fmicb.2022.822682
NADPH oxidase is an enzyme that generates reactive oxygen species from oxygen and NADPH and is highly conserved in eukaryotes. In Fusarium graminearum, a series of different Nox enzymes have been identified. NoxA is involved in sexual development and ascospore production and, like NoxB, also contributes to pathogenicity. Both NoxA and NoxB are regulated by the subunit NoxR, whereas NoxC is usually self-regulated by EF-hand motifs found on the enzyme. In this study, we characterized another NADPH oxidase in F. graminearum, FgNoxD. In the FgNoxD deletion mutant, vegetative growth and conidia production were reduced, while sexual development was totally abolished. The FgNoxD deletion mutant also showed reduced resistance to cell wall perturbing agents; cell membrane inhibitors; and osmotic, fungicide, cold, and extracellular oxidative stress, when compared to the wild type. Moreover, in comparison to the wild type, the FgNoxD deletion mutant exhibited reduced virulence against the host plant. The FgNoxD deletion mutant produced less deoxynivalenol than the wild type, and the Tri5 and Tri6 gene expression was also downregulated. In conclusion, our findings show that FgNoxD is involved in the survival against various stresses, conidiation, sexual development, and virulence, highlighting this enzyme as a new target to control the disease caused by F. graminearum.
Fusarium graminearum is a homothallic ascomycete fungus that causes Fusarium head blight (FHB) in cereal crops worldwide (Leslie and Summerell, 2006). It produces deoxynivalenol (DON) which inhibits protein synthesis by binding to ribosomes, making it toxic to humans and animals (Desjardins and Proctor, 2007; Pestka, 2010; Chong et al., 2020). Despite the major economic and health impacts caused by F. graminearum, sufficient strategies to control FHB have not been developed.
Reactive oxygen species (ROS) are highly reactive chemical molecules that play important roles in cell signaling, cell growth, and homeostasis (Dunand et al., 2007; Veal and Day, 2011). ROS are generated by all aerobic organisms as byproducts of normal metabolism. Excess ROS, such as superoxide, hydrogen peroxide (H2O2), and hydroxyl radicals, can non-specifically and rapidly react with other molecules including lipids, proteins, DNA, and carbohydrates (Gutteridge, 1994; Rodriguez and Redman, 2005). These reactions cause DNA mutation, lipid peroxidation, and protein oxidation, resulting in cellular dysfunction and apoptosis (Aguirre et al., 2005; Halliwell and Gutteridge, 2015).
NADPH oxidases (Nox), a ROS-producing enzyme, is membrane-bound enzyme complex exposed to the extracellular space. This multicomponent Nox enzyme complex was first studied in human phagocytic cells, where it was found that NADPH is used as an electron donor and the electrons are transported through the membrane to convert oxygen to superoxide (Lambeth, 2004). In animal cells, Nox enzymes are linked to cell signaling, cell growth, and cell death (Sumimoto, 2008; Brown and Griendling, 2009). In plant cells, Nox is implicated in the response to abiotic stresses, infection by pathogens, and polarized growth of root hairs. Additionally, Nox also acts as a secondary messenger for speedy transmission over long distance and in local signaling (Torres et al., 2002; Foreman et al., 2003; Suzuki et al., 2011; Lee et al., 2020).
Nox enzyme have also been studied in fungi. Four different fungal Nox enzymes—NoxA (Nox1), NoxB (Nox2), NoxC (Nox3), and NoxD—have been characterized to date. The gh91phox protein homolog enzyme, NoxA, is involved in fruiting body formation in various filamentous fungi, including Aspergillus nidulans, Podospora anserina, and Neurospora crassa (Lara-Ortíz et al., 2003; Malagnac et al., 2004; Cano-Dominguez et al., 2008). Furthermore, NoxA is also related to virulence, formation of sclerotia, and cellulose degradation (Giesbert et al., 2008; Segmuller et al., 2008; Brun et al., 2009; Kim et al., 2011; Yang and Chung, 2012). Another gh91phox protein homolog enzyme, NoxB, is necessary for host penetration in Magnaporthe oryzae and Botrytis cinerea, and ascospore germination in N. crassa and P. anserina (Malagnac et al., 2004; Egan et al., 2007; Cano-Dominguez et al., 2008; Segmuller et al., 2008). Although many fungi, for example, M. oryzae and P. anserine, express the NoxC enzyme, little is known about this enzyme and its function, except for its regulatory subunits (Takemoto et al., 2007).
In phagocytes, gh91phox binds to the p22phox protein, both of which are subunits of flavocytochrome b558, necessary for activation of the Nox enzyme. Moreover, the transmembrane protein also applies to Nox1, Nox3, and Nox4 (Nakano et al., 2008; Zana et al., 2018; Makhezer et al., 2019). In fungi, the functional orthologue of the p22phox protein was first identified in Sordaria macrospora which was named Pro41. In S. macrospora, Pro41 is required for fruiting body maturation (Nowrousian et al., 2007, 2012; Galhano et al., 2017). The membrane protein NoxD is highly homologous to the endoplasmic reticulum (ER) protein Pro41 in several fungi (Nowrousian et al., 2007; Lacaze et al., 2015; Siegmund et al., 2015). In B. cinerea, NoxA and NoxD interact with each other and are involved in pathogenicity, fusion of conidial anastomosis tube, and formation of sclerotia and conidia (Siegmund et al., 2015).
Fusarium graminearum also expresses NoxA, NoxB, NoxC, and NoxD. Study of NoxA and NoxB in F. graminearum indicate that NoxA is involved in perithecia development and ascospore production, and both NoxA and NoxB contribute to virulence but are not associated with mycotoxin synthesis (Wang et al., 2014). NoxC in F. graminearum is typically self-regulated by EF-hand motifs found on the enzyme, whereas NoxA and NoxB are regulated by the regulatory subunit NoxR (Heller and Tudzynski, 2011; Tudzynski et al., 2012; Zhang et al., 2016). Although the Pro41 homolog gene NoxD is also found in F. graminearum, the function of the gene product has not yet been studied. Therefore, in this study, we identified the location and characterized the functions of NoxD in F. graminearum (FgNoxD for F. graminearum NoxD). The study determined the phenotypic changes in conidial germination, vegetative growth, virulence, and mycotoxin synthesis to determine the biological functions of FgNoxD.
Fusarium graminearum wild-type strain GZ3639 (Bowden and Leslie, 1999) and mutants were cultivated in media following the Fusarium laboratory manual (Leslie and Summerell, 2006). Conidia formation was induced in carboxyl methyl cellulose (CMC; Cappellini and Peterson, 1965) or yeast malt agar (YMA) medium (Harris, 2005), and fungal strains were cultivated in complete medium (CM). All strains were stored as agar block in 20% glycerol at −80°C.
Targeted gene deletion and complementation were manipulated according to the split-marker recombination (SMR) strategy (Catlett et al., 2003). For gene deletion, the 5' and 3' flankings of the target gene were amplified from GZ3639. Meanwhile, a hygromycin resistance cassette (HYG) was amplified from pIGPAPA (Horwitz et al., 1999) using primer pairs. The three amplicons were mixed and fused using PCR. The final product for transformation was amplified during the third PCR step using nested primer pairs. To complement the gene deletion, a DNA fragment carrying the open read frame and native promoter of FgNoxD was fused with the geneticin resistance cassette (GEN) and amplified with pII99 through SMR (Namiki et al., 2001). For transformation, protoplasts of GZ3639 were prepared and a previously described method was applied (Kim et al., 2006; Li et al., 2019). In brief, conidia were incubated in 50 ml of YPG (10 g/L of peptone, 3 g/L of yeast extract, and 20 g/L of glucose) with shaking at 200 rpm for 12 h at 25°C. After that, the mycelia were harvested by filtration, then incubated in 35 ml of 1 M NH4Cl containing 15 mg/ml driselase (Sigma-Aldrich) to generate protoplast. The final PCR product, which carried a selectable marker, was incorporated directly into the protoplast. Transformants carrying selectable markers were selected on regeneration medium (1 g/L of casein, 1 g/L of yeast extract, 342 g/L of sucrose, and 15 g/L of micro agar) containing 75 μg/ml hygromycin or 75 μg/ml geneticin. The PCR primers used in this study are listed in Table 1.
To validate the constructed mutant and differently expressed genes involved in DON production, quantitative real-time PCR (qRT-PCR) was performed. The conidia of each strain (1 × 105 conidia/ml) were cultivated in 20 ml of CM at 200 rpm and 25°C for 3 days. Mycelia were harvested and ground using liquid nitrogen before the total RNA of each strain was extracted using the easy-spin Total RNA Extraction Kit (iNtRON Biotechnology, Seongnam, Korea) following the manufacturer’s protocol. Next, cDNA was generated using the First Strand cDNA Synthesis Kit (TOYOBO Co., Osaka, Japan) following the manufacturer’s instructions. The synthesized cDNA of each strain was diluted to 100 ng/μl and 2 μl of cDNA was used for qRT-PCR. The qRT-PCR conditions were 95°C for 5 min, followed by 40 cycles of 95°C for 5 s, 60°C for 10 s, and 72°C for 35 s. Relative gene expression was normalized to that of cyclophilin (CYP; Son et al., 2013).
The GZ3639, FgNoxD deletion mutant (ΔFgNoxD), and complementation (FgNoxD-C) strains were cultivated on potato dextrose agar (PDA), CM, minimal medium (MM), and YMA for 3 days at 25°C, after which the colony diameter of each strain was measured. The aerial mycelia growth of each strain was measured as previously described, with slight modifications (Nguyen et al., 2011). Briefly, each strain was inoculated in CM in a test tube at 25°C for 3 days and aerial mycelial growth was measured. For conidial germination of each strain, conidia (1 × 105 conidia/ml) harvested from CMC were incubated in MM. The number of total conidia and germinated conidia were counted at 4, 8, and 12 h by light microscopy.
Perithecial production was induced as described in a previous study (Min et al., 2010). Each strain was inoculated onto carrot agar medium for 8 days at 25°C in the dark. Thereafter, the mycelia were removed with 1 ml of 2.5% Tween-20 and the plates were incubated under near-ultraviolet light (20 W, 50 lux) for 10 days at 25°C. Perithecia and ascospores were observed and photographed using Moticam Pro S5 Lite camera (Motic, Barcelona, Spain).
The role of FgNoxD in stress response was tested as described in previous studies with slight modifications. For the osmotic stress test, each strain was inoculated in MM supplemented with 1.4 M KCl and NaCl and cultivated for 5 days at 25°C, after which radial growth was measured (Gu et al., 2015). Cold stress tests were performed as previously described (Li et al., 2019). Briefly, conidia (1 × 103 conidia/ml) in distilled water were stored at 4°C for 6 days and 100 μl of each suspension was spread on PDA. The number of surviving spores was counted after 1 day. The cell wall and membrane integrity of each stain were tested on MM supplemented with 60 mg/L Congo red (CR; Sigma-Aldrich), 50 μg/ml calcofluor white (CFW; Sigma-Aldrich), and 0.01% of SDS. The colony diameter of each strain was measured after cultivation at 25°C for 3 days (Ram and Klis, 2006; Schroeder and Ikui, 2019). For the fungicide resistance test, mycelia from each strain were inoculated onto MM supplemented with 0.1–0.5 mg/L prochloraz fungicide and cultivated at 25°C for 5 days (Li et al., 2019). Oxidative stress tests were performed using menadione (Katikireddy et al., 2018; Majiene et al., 2019; Funk et al., 2021) and H2O2. Each strain was inoculated in MM supplemented with 1 or 3 mM menadione and cultivated at 25°C for 3 days. Different concentrations (1, 3, and 5 mM) of H2O2 were added to MM and mycelia plugs of each strain were cultivated at 25°C for 1 day. Then mycelia plug were transmitted to a new CM and cultivated for another 3 days at 25°C to investigate the survival of each strain.
Lipid body staining was performed after treatment with cold stress. Briefly, conidia (1 × 106 conidia/ml) in distilled water were stored at 4°C for 1 day. The conidia were harvested by centrifugation and washed twice with phosphate-buffered saline (PBS). Thereafter, the lipid body in each strain was stained with a Nile Red solution consisting of 0.01 mg/ml Nile Red Oxazone (Sigma-Aldrich; Seong et al., 2008; Jung et al., 2018). The samples were incubated for 15 min at room temperature and washed twice with PBS. Fluorescence emitted by the lipid body was observed using Olympus BX50 microscope (Olympus, Tokyo, Japan).
Virulence of each strain was evaluated using the wheat cultivar, Geumgangmil, and the rice cultivar DongjinByeo. For the virulence tests on wheat, plant at two different stages were inoculated. Before inoculating each strain on coleoptile, wheat seeds were germinated on moist filter paper at 25°C. Then top 2–3 mm of the coleoptiles were removed and 2 μl of conidia suspension (1 × 106 conidia/ml in 0.01% Tween-20) was inoculated. The coleoptiles were then cultivated in a growth chamber at 25°C with 100% relative humidity and 12 h of light per 24 h. The virulence of each strain was assessed by measuring the length of the lesion on the diseased stem 10 days after inoculation (Wu et al., 2005). For wheat head inoculation, 10 μl of conidia suspension (1 × 106 conidia/ml in 0.01% Tween-20) was inoculated into the center of each spikelet. Spikelet exhibiting FHB symptoms were counted 14 days after inoculation. The rachis of each wheat head were also examined (Lee et al., 2009). For rice head inoculation, rice heads were dipped into suspensions of each strain (1 × 105 conidia/ml in 0.01% Tween-20) for 30 s and individually sealed in plastic bags for 72 h. The infected rice heads were then placed in a greenhouse and rice exhibiting FHB symptoms were counted after inoculation (Jung et al., 2018).
The production of DON was evaluated as described in a previous study (Ponts et al., 2006). Briefly, conidia of each strain were cultivated in 20 ml of GYEP medium (10 g/L glucose, 1 g/L yeast extract, and 1 g/L peptone) supplemented with or without 1 mM of H2O2 for 5 days at 200 rpm and 25°C. DON concentrations were determined using an enzyme-linked immunosorbent assay kit (CUSABIO, College Park, MD, United States) following the manufacturer’s instructions (Yoshizawa et al., 2004; Jung et al., 2018; Xu et al., 2018). Furthermore, mycelia were cultured with same method to detect the transcript levels of Tri5 (Maier et al., 2006) and Tri6 (Nasmith et al., 2011) genes.
Statistical differences of mycelial growth, cold stress, cell wall, and membrane stress tests, and virulence were examined by parametric one-way analysis of variance using R software (version 4.0.2). Additionally, statistical differences in osmotic stress resistance, DON production, and qRT-PCR were examined using t-test.
The gene sequence of FgNoxD (FGSG_01268) was acquired from National Center for Biotechnology Information database1 using the BcNoxD protein sequence of B. cinerea. FgNoxD, which contains 1709 bp with two introns, is predicted to encode a protein with 146 amino acids. Phylogenetic analysis and protein alignment indicated that NoxD was highly conserved in eukaryote (Figure 1A; Supplementary Figure S1).
Figure 1. FgNoxD amino sequence phylogenetic tree and gene manipulation. (A) Phylogenetic analysis of FgNoxD protein. All amino acid sequences were aligned using ClustalW which built-in MEGA X (version 6.0). The phylogenetic tree analyses were performed using the method of Maximum likelihood (ML). (B) Homologous recombination for construction of FgNoxD deletion and complementation constructs. Complementation strain was created using the ΔFgNoxD-3. (C) Relative expression levels of FgNoxD in GZ3639, ΔFgNoxD, and FgNoxD-C. Error bars indicate standard errors from four repeated experiments with three biological replications. Asterisks indicate value of p (NS, no significance; ***p < 0.001) after comparison with Welch’s t-test. HYG, hygromycin resistance cassette and GEN, geneticin resistance cassette.
To characterize the functions of FgNoxD, the FgNoxD gene was replaced with a constitutively expressed HYG cassette via SMR (ΔFgNoxD). To verify whether the observed changes found in the deletion mutant were caused by gene defection, FgNoxD was reintroduced at an alternate site in the deletion mutant (FgNoxD-C; Figure 1B). qRT-PCR showed that the transcripts of FgNoxD were completely abolished in the deletion mutant but was recovered in FgNoxD-C (Figure 1C).
Compared to GZ3639 and FgNoxD-C, ΔFgNoxD showed significantly reduced mycelia growth and aerial hyphae growth (Figures 2A,B). Although the conidial germination and morphology were not significantly different between GZ3639 and ΔFgNoxD, conidia production was significantly reduced in ΔFgNoxD (Table 2). Moreover, ΔFgNoxD completely lost self-fertility and did not form any initial perithecia structures (Figure 2C).
Figure 2. Fungal growth and sexual development GZ3639, ΔFgNoxD, and FgNoxD-C. (A) The growth of GZ3639, ΔFgNoxD-3, ΔFgNoxD-4, and FgNoxD-C on potato dextrose agar (PDA), complete medium (CM), minimal medium (MM), yeast malt agar (YMA) for 3 days at 25°C. Colony diameters of the strains were measured. Error bars represent the SD from five replicates. (B) Aerial hyphae growth of the strains on CM for 3 days. Colony height of the strains was measured. Error bars represent the SD from three replicates. (C) Sexual development induced on carrot agar (CA) for 10 days at near UV. Perithecia formation and ascospores were observed after 10 days. White bar = 10 μm; Black bar = 1 mm. Values with different letters are significantly different according to Tukey’s test ( p < 0.001).
To confirm the role of FgNoxD in resistance to various stresses, a series of abiotic stress resistances were tested. ΔFgNoxD showed significantly reduced resistance to osmotic stress when supplied with 1.4 M KCl or 1.4 M NaCl compared to the wild type (Figure 3A). ΔFgNoxD also showed a significantly lower survival rate under cold condition compared to the wild-type and complemented strains (Figure 3B). In cell wall integrity test, ΔFgNoxD showed a significantly reduced inhibition rate compared to the wild type not only in CR supplemented medium but also in CFW supplemented medium. ΔFgNoxD also displayed reduced resistance to SDS, which disrupts cell membrane integrity (Figure 3C). Compared to the wild type, ΔFgNoxD also showed significantly reduced resistance to prochloraz, a fungicide which target the cell membrane (Figure 3D). In addition, the lipid body of ΔFgNoxD was reduced under cold condition (Figure 4). Treatment with menadione showed no significantly difference between deletion mutant and wild type (Figure 5). When H2O2 was added to the medium, there was no difference between the deletion mutants and the wild type. However, when these strains without mycelium growth (Figure 6A) were transferred to another normal CM, it could be seen that, contrary to the wild type, mycelium growth could not be observed in the deletion mutants, that means H2O2 was lethal to the deletion mutant (Figure 6).
Figure 3. Resistance of GZ3639, ΔFgNoxD, and FgNoxD-C to various stresses. (A) Osmotic stress: strain was cultured in MM supplemented with 1.4 M KCl and NaCl for 5 days. Error bars represent the SE from five replicates. (B) Cold stress: 103 conidia/ml of each strain were stored at 4°C for 6 days in distilled water. One hundred microliter of each conidia suspension was spread onto PDA and the survived spore was counted after 1 day. Error bars represent the SD from five replicates. (C) Cell membrane and cell wall stress test. All strains were cultured in MM without or with 60 mg/L congo red (CR), 50 μg/ml calcofluor white (CFW), 0.01% SDS for 3 days. Error bars represent the SD from five replicates. Values with different letters are significantly different according to Tukey’s test ( p < 0.001). (D) Fungicide test: the strains were inoculated in MM containing different concentration of prochloraz for 5 days at 25°C. This experiment was repeated five times.
Figure 4. Accumulated lipid body in GZ3639, ΔFgNoxD, and FgNoxD-C. Conidia suspension of GZ3639, ΔFgNoxD, and FgNoxD-C (1 × 106 conidia/ml in distilled water) were store at 4°C for 1 day and stained with Nile Red. White arrow depicts accumulated lipid body. Scale bar = 10 μm.
Figure 5. Resistance of GZ3639, ΔFgNoxD, and FgNoxD-C to menadione. Fusarium graminearum strain was cultured on MM supplemented with different concentration of menadione for 3 days at 25°C. This experiment was repeated five times.
Figure 6. The effect of H2O2 on survival of GZ3639, ΔFgNoxD, and FgNoxD-C. (A) All strains were cultured in MM supplemented with different H2O2 concentrations for 1 day. (B) Strains from media containing 3 or 5 mM H2O2 were cultured for 3 days. The red circles indicate areas of growing mycelium.
The virulence of the deletion mutant was reduced compared with that of the wild type. Compared to the wild type, the lesion length on coleoptile was significantly reduced when coleoptile was inoculated with the deletion mutant (Figure 7A). FHB symptom in wheat heads and rachis inoculated with the deletion mutant were also significantly reduced (Figure 7B). The deletion mutant showed significantly reduced disease severity compared to the wild-type and complemented strains (Figure 7C).
Figure 7. Virulence of GZ3639, ΔFgNoxD, and FgNoxD-C. (A) Wheat coleoptiles were inoculated with 2 μl conidial suspension (1 × 106 conidia/ml in 0.01% Tween-20), and lesion length was measured for at least 10 wheat coleoptiles at 10 dpi. This experiment was repeated three times. (B) Flowering wheat heads were inoculated with 10 μl conidial suspension (1 × 106 conidia/ml in 0.01% Tween-20) and observed at 14 dpi. Disease index was determined from the number of symptomatic spikelets per wheat head. At least 14 wheat heads inoculated with F. graminearum strain were examined in addition to the wheat head rachis. (C) Flowering rice heads were dipped into conidial suspension (1 × 105 conidia/ml in 0.01% Tween-20) for 30 s and symptomatic rice grains per rice head were examined at 14 dpi. Disease severity was determined from the number of symptomatic grains per rice head. This experiment was repeated three times. Values with different letters are significantly different according to Tukey’s test ( p < 0.001).
There was no significant difference between the deletion mutant and wild-type strains with respect to DON production when they were cultivated in GYEP medium. However, when the medium was supplemented with H2O2, the deletion mutant showed reduced DON production compared to the wild type (Figure 8A). The transcript levels of both Tri5 and Tri6 showed no significantly differences between ΔFgNoxD and the wild type in GYEP medium but were significantly reduced in ΔFgNoxD compared to the wild type in the medium with H2O2 (Figures 8B,C).
Figure 8. Deoxynivalenol (DON) biosynthesis of GZ3639, ΔFgNoxD, and FgNoxD-C. (A) The DON concentration of F. graminearum strain. Supernatant of each culture was used to analyze DON concentration. (B,C) Relative expression levels of Tri5 and Tri6. GZ3639, ΔFgNoxD and FgNoxD-C were cultivated in GYEP with or without (Mock) 1 mM H2O2 for 5 days. The mycelia of F. graminearum strain was used to measure the transcript expression level. For DON concentration, bars denote SD from three repeated experiments. For qRT-PCR bars denote SE from three repeated experiments with three replications. Asterisks indicate value of p (*p < 0.05, **p < 0.01) after comparison with t-test.
The multicomponent Nox reduces molecular oxygen to superoxides in a stepwise manner, leading to the production of ROS (Lambeth, 2004). In fungi, these multicomponent enzymes are involved in virulence and differentiation (Malagnac et al., 2004; Egan et al., 2007; Takemoto et al., 2007; Giesbert et al., 2008). Moreover, previous studies in B. cinerea revealed that NoxD is involved in vegetative differentiation, colonization of host tissue, and oxidative stress resistance (Siegmund et al., 2015). Similar to previous studies on NoxD, our current study showed that FgNoxD in F. graminearum is involved in normal vegetative growth, virulence, asexual development, and resistance to various stressors.
Sexual development in F. graminearum is a vital factor that leads to its genetic diversity and adaptability in nature (Lee et al., 2011; Ni et al., 2011; Li et al., 2019). Sexual development is also a central strategy to survival in soil or host plant debris in the fields during winter (Guenther and Trail, 2005). Our study showed that ΔFgNoxD completely lost sexual development and also showed reduced resistance to cold stress (Figures 2C, 3B). These results suggest that FgNoxD plays an important role in F. graminearum survival during winter. The accumulated of lipid bodies act as reserves for perithecium development (Guenther et al., 2009; Son et al., 2011). Moreover, lipids are known to be involved in cold tolerance and survival in fungi (Istokovics et al., 1998). Our data also showed that lipid accumulation in ΔFgNoxD was reduced compared to the wild-type strain (Figure 4), which might have resulted in the abolishment of sexual development and reduction in cold stress resistance.
The fungal cell wall is an essential component with great plasticity that plays a vital role in normal cell growth and protection of cells from osmotic stress (Gow et al., 2017; Garcia-Rubio et al., 2019). ΔFgNoxD showed reduced vegetative and aerial hyphae growth (Figures 2A,B) and reduced resistance to osmotic stress compared to the wild type (Figure 3A). ΔFgNoxD was more sensitive to cell wall perturbing factor compared to the wild-type and complemented strains (Figure 3C), leading to the defects in vegetative growth and osmotic stress in the mutant. In addition, these results showed that FgNoxD plays a pivotal role in cell wall integrity in F. graminearum.
The fungal cell membrane is also an important component that is enriched with diverse lipids, such as sphingolipids and sterols (Sant et al., 2016). These lipids regulate fungal pathogenicity through lipid–protein and lipid–lipid interactions (Rella et al., 2016). In our study, ΔFgNoxD showed significantly reduced fungicide resistance compared to the wild type when the medium was supplemented with prochloraz, a fungicide that targets the fungal cell membrane. On the other hand, cell membrane also involved in response to osmotic stress (You et al., 2012; Freitag et al., 2014; Ren et al., 2019). The results showed that ΔFgNoxD was more sensitive to osmotic stress as well as cell membrane inhibitors than the wild-type and complemented strains (Figures 3A,C). Furthermore, lipid accumulation in ΔFgNoxD was significantly reduced (Figure 4). These results suggested that FgNoxD plays an important role for cell membrane integrity, and is tightly linked to virulence in F. graminearum (Figure 7).
The virulence of F. graminearum against the host plant can be ascribed to many factors, including resistance to ROS produced by the host plant and biosynthesis of trichothecenes (Boenisch and Schafer, 2011; Barna et al., 2012; Mentges and Bormann, 2015). ROS is a common by-product of both eukaryotic and prokaryotic organisms (Aguirre et al., 2005). ROS has a well-established damaging effect on cell components and are commonly used in plant defense systems (Jones and Dangl, 2006; Halliwell and Gutteridge, 2015). When plants recognize a pathogen, plant cells are capable of producing a burst of ROS, initially comprising H2O2, which can react with the proteins, DNA, and lipids of the pathogen to accelerate cell death (Sharma and Davis, 1997; O’Brien et al., 2012). Therefore, fungi must deactivate ROS produced by plants for successful plant infections. In our study, ΔFgNoxD showed reduced resistance to oxidative stress compared to the wild-type and complemented strains (Figure 6). The production of DON, which is an important virulence factor in F. graminearum, is triggered by H2O2 (Proctor et al., 1995; Audenaert et al., 2010). The amount of DON production triggered by H2O2 was reduced in ΔFgNoxD compared to the wild type (Figure 8A). Meanwhile, when exposed to H2O2, the expression levels of Tri5 and Tri6 were all significantly decreased in ΔFgNoxD compared to the wild-type and complemented strains (Figures 8B,C). In summary, the reduced virulence of ΔFgNoxD in host plants may be a result of reduced resistance to oxidative stress and DON biosynthesis.
In this study, we identified that FgNoxD plays an important role in the virulence of F. graminearum. The loss of virulence in ΔFgNoxD could be due to reduced mycelia growth, cell wall and membrane integrity, and resistance to ROS. FgNoxD contributed to the spread of the infected F. graminearum throughout the entire spike (Figure 7B). In addition, FgNoxD also plays an important role in sexual development and conidial production (Table 2). Therefore, understanding the role of FgNoxD may provide a new way to control FHB in the field. This study expands our knowledge of the Nox family in F. graminearum, and future studies will allow further dissection the role of FgNoxD and interaction with other Nox family members in F. graminearum.
The original contributions presented in the study are included in the article/Supplementary Material; further inquiries can be directed to the corresponding author.
TL and DK designed the experiments. DK created figures and tables. TL, DK, and JL wrote the manuscript. All authors contributed to the article and approved the submitted version.
This work was supported by the National Research Foundation (2020R1A2C2013617), LG Yonam Foundation of Korea, and Green Fusion Technology Program funded by Ministry of Environment, Republic of Korea.
The authors declare that the research was conducted in the absence of any commercial or financial relationships that could be construed as a potential conflict of interest.
The Supplementary Material for this article can be found online at: https://www.frontiersin.org/articles/10.3389/fmicb.2022.822682/full#supplementary-material
Aguirre, J., Rios-Momberg, M., Hewitt, D., and Hansberg, W. (2005). Reactive oxygen species and development in microbial eukaryotes. Trends Microbiol. 13, 111–118. doi: 10.1016/j.tim.2005.01.007
Audenaert, K., Callewaert, E., Hofte, M., De Saeger, S., and Haesaert, G. (2010). Hydrogen peroxide induced by the fungicide prothioconazole triggers deoxynivalenol (DON) production by Fusarium graminearum. BMC Microbiol. 10:112. doi: 10.1186/1471-2180-10-112
Barna, B., Fodor, J., Harrach, B. D., Pogany, M., and Kiraly, Z. (2012). The Janus face of reactive oxygen species in resistance and susceptibility of plants to necrotrophic and biotrophic pathogens. Plant Physiol. Biochem. 59, 37–43. doi: 10.1016/j.plaphy.2012.01.014
Boenisch, M. J., and Schafer, W. (2011). Fusarium graminearum forms mycotoxin producing infection structures on wheat. BMC Plant Biol. 11:110. doi: 10.1186/1471-2229-11-110
Bowden, R. L., and Leslie, J. F. (1999). Sexual recombination in Gibberella zeae. Phytopathology 89, 182–188. doi: 10.1094/PHYTO.1999.89.2.182
Brown, D. I., and Griendling, K. K. (2009). Nox proteins in signal transduction. Free Radic. Biol. Med. 47, 1239–1253. doi: 10.1016/j.freeradbiomed.2009.07.023
Brun, S., Malagnac, F., Bidard, F., Lalucque, H., and Silar, P. (2009). Functions and regulation of the Nox family in the filamentous fungus Podospora anserina: a new role in cellulose degradation. Mol. Microbiol. 74, 480–496. doi: 10.1111/j.1365-2958.2009.06878.x
Cano-Dominguez, N., Alvarez-Delfin, K., Hansberg, W., and Aguirre, J. (2008). NADPH oxidases NOX-1 and NOX-2 require the regulatory subunit NOR-1 to control cell differentiation and growth in Neurospora crassa. Eukaryot. Cell 7, 1352–1361. doi: 10.1128/EC.00137-08
Cappellini, R., and Peterson, J. (1965). Macroconidium formation in submerged cultures by a nonsporulating strain of Gibberella zeae. Mycologia 57, 962–966. doi: 10.1080/00275514.1965.12018285
Catlett, N. L., Lee, B.-N., Yoder, O., and Turgeon, B. G. (2003). Split-marker recombination for efficient targeted deletion of fungal genes. Fungal Genet. Rep. 50, 9–11. doi: 10.4148/1941-4765.1150
Chong, X., Wang, C., Wang, Y., Wang, Y., Zhang, L., Liang, Y., et al. (2020). The dynamin-like GTPase FgSey1 plays a critical role in fungal development and virulence in Fusarium graminearum. Appl. Environ. Microbiol. 86, e02720–e02819. doi: 10.1128/AEM.02720-19
Desjardins, A. E., and Proctor, R. H. (2007). Molecular biology of Fusarium mycotoxins. Int. J. Food Microbiol. 119, 47–50. doi: 10.1016/j.ijfoodmicro.2007.07.024
Dunand, C., Crevecoeur, M., and Penel, C. (2007). Distribution of superoxide and hydrogen peroxide in Arabidopsis root and their influence on root development: possible interaction with peroxidases. New Phytol. 174, 332–341. doi: 10.1111/j.1469-8137.2007.01995.x
Egan, M. J., Wang, Z. Y., Jones, M. A., Smirnoff, N., and Talbot, N. J. (2007). Generation of reactive oxygen species by fungal NADPH oxidases is required for rice blast disease. Proc. Natl. Acad. Sci. U. S. A. 104, 11772–11777. doi: 10.1073/pnas.0700574104
Foreman, J., Demidchik, V., Bothwell, J. H., Mylona, P., Miedema, H., Torres, M. A., et al. (2003). Reactive oxygen species produced by NADPH oxidase regulate plant cell growth. Nature 422, 442–446. doi: 10.1038/nature01485
Freitag, S. I., Wong, J., and Young, P. G. (2014). Genetic and physical interaction of Ssp1 CaMKK and Rad24 14-3-3 during low pH and osmotic stress in fission yeast. Open Biol. 4:130127. doi: 10.1098/rsob.130127
Funk, M. I., Conde, M. A., Piwien-Pilipuk, G., and Uranga, R. M. (2021). Novel antiadipogenic effect of menadione in 3T3-L1 cells. Chem. Biol. Interact. 343:109491. doi: 10.1016/j.cbi.2021.109491
Galhano, R., Illana, A., Ryder, L. S., Rodriguez-Romero, J., Demuez, M., Badaruddin, M., et al. (2017). Tpc1 is an important Zn (II)2Cys6 transcriptional regulator required for polarized growth and virulence in the rice blast fungus. PLoS Pathog. 13:e1006516. doi: 10.1371/journal.ppat.1006516
Garcia-Rubio, R., de Oliveira, H. C., Rivera, J., and Trevijano-Contador, N. (2019). The fungal cell wall: Candida, Cryptococcus, and Aspergillus species. Front. Microbiol. 10:2993. doi: 10.3389/fmicb.2019.02993
Giesbert, S., Schurg, T., Scheele, S., and Tudzynski, P. (2008). The NADPH oxidase Cpnox1 is required for full pathogenicity of the ergot fungus Claviceps purpurea. Mol. Plant Pathol. 9, 317–327. doi: 10.1111/j.1364-3703.2008.00466.x
Gow, N. A. R., Latge, J. P., and Munro, C. A. (2017). The fungal cell wall: structure, biosynthesis, and function. Microbiol. Spectr. 5, 267–292. doi: 10.1128/microbiolspec.FUNK-0035-2016
Gu, Q., Zhang, C., Yu, F., Yin, Y., Shim, W. B., and Ma, Z. (2015). Protein kinase FgSch 9 serves as a mediator of the target of rapamycin and high osmolarity glycerol pathways and regulates multiple stress responses and secondary metabolism in Fusarium graminearum. Environ. Microbiol. 17, 2661–2676. doi: 10.1111/1462-2920.12522
Guenther, J. C., Hallen-Adams, H. E., Bucking, H., Shachar-Hill, Y., and Trail, F. (2009). Triacylglyceride metabolism by Fusarium graminearum during colonization and sexual development on wheat. Mol. Plant-Microbe Interact. 22, 1492–1503. doi: 10.1094/MPMI-22-12-1492
Guenther, J. C., and Trail, F. (2005). The development and differentiation of Gibberella zeae (anamorph: Fusarium graminearum) during colonization of wheat. Mycologia 97, 229–237. doi: 10.1080/15572536.2006.11832856
Gutteridge, J. M. (1994). Antioxidants, nutritional supplements and life-threatening diseases. Br. J. Biomed. Sci. 51, 288–295.
Halliwell, B., and Gutteridge, J. M. (2015). Free Radicals in Biology and Medicine. 5th Edn. Oxford: Oxford Univ. Press.
Harris, S. D. (2005). Morphogenesis in germinating Fusarium graminearum macroconidia. Mycologia 97, 880–887. doi: 10.1080/15572536.2006.11832779
Heller, J., and Tudzynski, P. (2011). Reactive oxygen species in phytopathogenic fungi: signaling, development, and disease. Annu. Rev. Phytopathol. 49, 369–390. doi: 10.1146/annurev-phyto-072910-095355
Horwitz, B. A., Sharon, A., Lu, S.-W., Ritter, V., Sandrock, T. M., Yoder, O., et al. (1999). A G protein alpha subunit from Cochliobolus heterostrophus involved in mating and appressorium formation. Fungal Genet. Biol. 26, 19–32. doi: 10.1006/fgbi.1998.1094
Istokovics, A., Morita, N., Izumi, K., Hoshino, T., Yumoto, I., Sawada, M. T., et al. (1998). Neutral lipids, phospholipids, and a betaine lipid of the snow mold fungus Microdochium nivale. Can. J. Microbiol. 44, 1051–1059. doi: 10.1139/w98-094
Jones, J. D., and Dangl, J. L. (2006). The plant immune system. Nature 444, 323–329. doi: 10.1038/nature05286
Jung, B., Park, J., Kim, N., Li, T., Kim, S., Bartley, L. E., et al. (2018). Cooperative interactions between seed-borne bacterial and air-borne fungal pathogens on rice. Nat. Commun. 9, 1–11. doi: 10.1038/s41467-017-02430-2
Katikireddy, K. R., White, T. L., Miyajima, T., Vasanth, S., Raoof, D., Chen, Y., et al. (2018). NQO1 downregulation potentiates menadione-induced endothelial-mesenchymal transition during rosette formation in Fuchs endothelial corneal dystrophy. Free Radic. Biol. Med. 116, 19–30. doi: 10.1016/j.freeradbiomed.2017.12.036
Kim, H. J., Chen, C., Kabbage, M., and Dickman, M. B. (2011). Identification and characterization of Sclerotinia sclerotiorum NADPH oxidases. Appl. Environ. Microbiol. 77, 7721–7729. doi: 10.1128/AEM.05472-11
Kim, J. E., Jin, J. M., Kim, H., Kim, J. C., Yun, S. H., and Lee, Y. W. (2006). GIP2, a putative transcription factor that regulates the aurofusarin biosynthetic gene cluster in Gibberella zeae. Appl. Environ. Microbiol. 72, 1645–1652. doi: 10.1128/AEM.72.2.1645-1652.2006
Lacaze, I., Lalucque, H., Siegmund, U., Silar, P., and Brun, S. (2015). Identification of NoxD/Pro41 as the homologue of the p22phox NADPH oxidase subunit in fungi. Mol. Microbiol. 95, 1006–1024. doi: 10.1111/mmi.12876
Lambeth, J. D. (2004). NOX enzymes and the biology of reactive oxygen. Nat. Rev. Immunol. 4, 181–189. doi: 10.1038/nri1312
Lara-Ortíz, T., Riveros-Rosas, H., and Aguirre, J. (2003). Reactive oxygen species generated by microbial NADPH oxidase NoxA regulate sexual development in Aspergillus nidulans. Mol. Microbiol. 50, 1241–1255. doi: 10.1046/j.1365-2958.2003.03800.x
Lee, S. H., Han, Y. K., Yun, S. H., and Lee, Y. W. (2009). Roles of the glyoxylate and methylcitrate cycles in sexual development and virulence in the cereal pathogen Gibberella zeae. Eukaryot. Cell 8, 1155–1164. doi: 10.1128/EC.00335-08
Lee, D., Lal, N. K., Lin, Z.-J. D., Ma, S., Liu, J., Castro, B., et al. (2020). Regulation of reactive oxygen species during plant immunity through phosphorylation and ubiquitination of RBOHD. Nat. Commun. 11, 1–16. doi: 10.1038/s41467-020-15601-5
Lee, S., Son, H., Lee, J., Min, K., Choi, G. J., Kim, J. C., et al. (2011). Functional analyses of two acetyl coenzyme A synthetases in the ascomycete Gibberella zeae. Eukaryot. Cell 10, 1043–1052. doi: 10.1128/EC.05071-11
Leslie, J. F., and Summerell, B. A. (2006). The Fusarium Laboratory Manual. Ames, Iowa, USA: Blackwell Publishing.
Li, T. Y., Jung, B., Park, S. Y., and Lee, J. (2019). Survival factor gene FgSvf1 is required for normal growth and stress resistance in Fusarium graminearum. Plant Pathol. J. 35, 393–405. doi: 10.5423/PPJ.OA.03.2019.0070
Maier, F. J., Miedaner, T., Hadeler, B., Felk, A., Salomon, S., Lemmens, M., et al. (2006). Involvement of trichothecenes in fusarioses of wheat, barley and maize evaluated by gene disruption of the trichodiene synthase (Tri5) gene in three field isolates of different chemotype and virulence. Mol. Plant Pathol. 7, 449–461. doi: 10.1111/j.1364-3703.2006.00351.x
Majiene, D., Kuseliauskyte, J., Stimbirys, A., and Jekabsone, A. (2019). Comparison of the effect of native 1,4-naphthoquinones plumbagin, menadione, and lawsone on viability, redox status, and mitochondrial functions of C6 Glioblastoma cells. Nutrients 11:1294. doi: 10.3390/nu11061294
Makhezer, N., Ben Khemis, M., Liu, D., Khichane, Y., Marzaioli, V., Tlili, A., et al. (2019). NOX1-derived ROS drive the expression of Lipocalin-2 in colonic epithelial cells in inflammatory conditions. Mucosal Immunol. 12, 117–131. doi: 10.1038/s41385-018-0086-4
Malagnac, F., Lalucque, H., Lepere, G., and Silar, P. (2004). Two NADPH oxidase isoforms are required for sexual reproduction and ascospore germination in the filamentous fungus Podospora anserina. Fungal Genet. Biol. 41, 982–997. doi: 10.1016/j.fgb.2004.07.008
Mentges, M., and Bormann, J. (2015). Real-time imaging of hydrogen peroxide dynamics in vegetative and pathogenic hyphae of Fusarium graminearum. Sci. Rep. 5:14980. doi: 10.1038/srep14980
Min, K., Lee, J., Kim, J.-C., Kim, S. G., Kim, Y. H., Vogel, S., et al. (2010). A novel gene, ROA, is required for normal morphogenesis and discharge of ascospores in Gibberella zeae. Eukaryot. Cell 9, 1495–1503. doi: 10.1128/EC.00083-10
Nakano, Y., Longo-Guess, C. M., Bergstrom, D. E., Nauseef, W. M., Joness, S. M., and Banfi, B. (2008). Mutation of the Cyba gene encoding p22phox causes vestibular and immune defects in mice. J. Clin. Invest. 118, 1176–1185. doi: 10.1172/JCI33835
Namiki, F., Matsunaga, M., Okuda, M., Inoue, I., Nishi, K., Fujita, Y., et al. (2001). Mutation of an arginine biosynthesis gene causes reduced pathogenicity in Fusarium oxysporum f. sp. melonis. Mol. Plant-Microbe Interact. 14, 580–584. doi: 10.1094/MPMI.2001.14.4.580
Nasmith, C. G., Walkowiak, S., Wang, L., Leung, W. W., Gong, Y., Johnston, A., et al. (2011). Tri6 is a global transcription regulator in the phytopathogen Fusarium graminearum. PLoS Pathog. 7:e1002266. doi: 10.1371/journal.ppat.1002266
Nguyen, L. N., Bormann, J., Le, G. T. T., Stärkel, C., Olsson, S., Nosanchuk, J. D., et al. (2011). Autophagy-related lipase FgATG15 of Fusarium graminearum is important for lipid turnover and plant infection. Fungal Genet. Biol. 48, 217–224. doi: 10.1016/j.fgb.2010.11.004
Ni, M., Feretzaki, M., Sun, S., Wang, X., and Heitman, J. (2011). Sex in fungi. Annu. Rev. Genet. 45, 405–430. doi: 10.1146/annurev-genet-110410-132536
Nowrousian, M., Frank, S., Koers, S., Strauch, P., Weitner, T., Ringelberg, C., et al. (2007). The novel ER membrane protein PRO41 is essential for sexual development in the filamentous fungus Sordaria macrospora. Mol. Microbiol. 64, 923–937. doi: 10.1111/j.1365-2958.2007.05694.x
Nowrousian, M., Teichert, I., Masloff, S., and Kuck, U. (2012). Whole-genome sequencing of Sordaria macrospora mutants identifies developmental genes. G3 2, 261–270. doi: 10.1534/g3.111.001479
O’Brien, J. A., Daudi, A., Butt, V. S., and Bolwell, G. P. (2012). Reactive oxygen species and their role in plant defence and cell wall metabolism. Planta 236, 765–779. doi: 10.1007/s00425-012-1696-9
Pestka, J. J. (2010). Deoxynivalenol: mechanisms of action, human exposure, and toxicological relevance. Arch. Toxicol. 84, 663–679. doi: 10.1007/s00204-010-0579-8
Ponts, N., Pinson-Gadais, L., Verdal-Bonnin, M. N., Barreau, C., and Richard-Forget, F. (2006). Accumulation of deoxynivalenol and its 15-acetylated form is significantly modulated by oxidative stress in liquid cultures of Fusarium graminearum. FEMS Microbiol. Lett. 258, 102–107. doi: 10.1111/j.1574-6968.2006.00200.x
Proctor, R. H., Hohn, T. M., and McCormick, S. P. (1995). Reduced virulence of Gibberella zeae caused by disruption of a trichothecene toxin biosynthetic gene. Mol. Plant-Microbe Interact. 8, 593–601. doi: 10.1094/MPMI-8-0593
Ram, A. F. J., and Klis, F. M. (2006). Identification of fungal cell wall mutants using susceptibility assays based on Calcofluor white and Congo red. Nat. Protoc. 1, 2253–2256. doi: 10.1038/nprot.2006.397
Rella, A., Farnoud, A. M., and Del Poeta, M. (2016). Plasma membrane lipids and their role in fungal virulence. Prog. Lipid Res. 61, 63–72. doi: 10.1016/j.plipres.2015.11.003
Ren, W., Liu, N., Yang, Y., Yang, Q., Chen, C., Gao, Q. J. F., et al. (2019). The sensor proteins BcSho1 and BcSln1 are involved in, though not essential to, vegetative differentiation, pathogenicity and osmotic stress tolerance in Botrytis cinerea. Front. Microbiol. 10:328. doi: 10.3389/fmicb.2019.00328
Rodriguez, R., and Redman, R. (2005). Balancing the generation and elimination of reactive oxygen species. Proc. Natl. Acad. Sci. U. S. A. 102, 3175–3176. doi: 10.1073/pnas.0500367102
Sant, D. G., Tupe, S. G., Ramana, C. V., and Deshpande, M. V. (2016). Fungal cell membrane-promising drug target for antifungal therapy. J. Appl. Microbiol. 121, 1498–1510. doi: 10.1111/jam.13301
Schroeder, L., and Ikui, A. E. (2019). Tryptophan confers resistance to SDS-associated cell membrane stress in Saccharomyces cerevisiae. PLoS One 14:e0199484. doi: 10.1371/journal.pone.0199484
Segmuller, N., Kokkelink, L., Giesbert, S., Odinius, D., van Kan, J., and Tudzynski, P. (2008). NADPH oxidases are involved in differentiation and pathogenicity in Botrytis cinerea. Mol. Plant-Microbe Interact. 21, 808–819. doi: 10.1094/MPMI-21-6-0808
Seong, K. Y., Zhao, X., Xu, J. R., Guldener, U., and Kistler, H. C. (2008). Conidial germination in the filamentous fungus Fusarium graminearum. Fungal Genet. Biol. 45, 389–399. doi: 10.1016/j.fgb.2007.09.002
Sharma, Y. K., and Davis, K. R. (1997). The effects of ozone on antioxidant responses in plants. Free Radic. Biol. Med. 23, 480–488. doi: 10.1016/S0891-5849(97)00108-1
Siegmund, U., Marschall, R., and Tudzynski, P. (2015). BcNoxD, a putative ER protein, is a new component of the NADPH oxidase complex in Botrytis cinerea. Mol. Microbiol. 95, 988–1005. doi: 10.1111/mmi.12869
Son, H., Lee, J., Park, A. R., and Lee, Y. W. (2011). ATP citrate lyase is required for normal sexual and asexual development in Gibberella zeae. Fungal Genet. Biol. 48, 408–417. doi: 10.1016/j.fgb.2011.01.002
Son, M., Lee, K. M., Yu, J., Kang, M., Park, J. M., Kwon, S. J., et al. (2013). The HEX1 gene of Fusarium graminearum is required for fungal asexual reproduction and pathogenesis and for efficient viral RNA accumulation of Fusarium graminearum virus 1. J. Virol. 87, 10356–10367. doi: 10.1128/JVI.01026-13
Sumimoto, H. (2008). Structure, regulation and evolution of Nox-family NADPH oxidases that produce reactive oxygen species. FEBS J. 275, 3249–3277. doi: 10.1111/j.1742-4658.2008.06488.x
Suzuki, N., Miller, G., Morales, J., Shulaev, V., Torres, M. A., and Mittler, R. (2011). Respiratory burst oxidases: the engines of ROS signaling. Curr. Opin. Plant Biol. 14, 691–699. doi: 10.1016/j.pbi.2011.07.014
Takemoto, D., Tanaka, A., and Scott, B. (2007). NADPH oxidases in fungi: diverse roles of reactive oxygen species in fungal cellular differentiation. Fungal Genet. Biol. 44, 1065–1076. doi: 10.1016/j.fgb.2007.04.011
Torres, M. A., Dangl, J. L., and Jones, J. D. (2002). Arabidopsis gp91phox homologues AtrbohD and AtrbohF are required for accumulation of reactive oxygen intermediates in the plant defense response. Proc. Natl. Acad. Sci. U. S. A. 99, 517–522. doi: 10.1073/pnas.012452499
Tudzynski, P., Heller, J., and Siegmund, U. (2012). Reactive oxygen species generation in fungal development and pathogenesis. Curr. Opin. Microbiol. 15, 653–659. doi: 10.1016/j.mib.2012.10.002
Veal, E., and Day, A. (2011). Hydrogen peroxide as a signaling molecule. Antioxid. Redox Signal. 15, 147–151. doi: 10.1089/ars.2011.3968
Wang, L., Mogg, C., Walkowiak, S., Joshi, M., and Subramaniam, R. (2014). Characterization of NADPH oxidase genes NoxA and NoxB in Fusarium graminearum. Can. J. Plant Pathol. 36, 12–21. doi: 10.1080/07060661.2013.868370
Wu, A. B., Li, H. P., Zhao, C. S., and Liao, Y. C. (2005). Comparative pathogenicity of Fusarium graminearum isolates from China revealed by wheat coleoptile and floret inoculations. Mycopathologia 160, 75–83. doi: 10.1007/s11046-005-1153-4
Xu, H., Dong, Y., Guo, J., Jiang, X., Liu, J., Xu, S., et al. (2018). Monoclonal antibody production and the development of an indirect competitive enzyme-linked immunosorbent assay for screening T-2 toxin in milk. Toxicon 156, 1–6. doi: 10.1016/j.toxicon.2018.10.307
Yang, S. L., and Chung, K. R. (2012). The NADPH oxidase-mediated production of hydrogen peroxide (H2O2) and resistance to oxidative stress in the necrotrophic pathogen Alternaria alternata of citrus. Mol. Plant Pathol. 13, 900–914. doi: 10.1111/j.1364-3703.2012.00799.x
Yoshizawa, T., Kohno, H., Ikeda, K., Shinoda, T., Yokohama, H., Morita, K., et al. (2004). A practical method for measuring deoxynivalenol, nivalenol and T-2+HT-2 toxin in foods by an enzyme-linked immunosorbent assay using monoclonal antibodies. Biosci. Biotechnol. Biochem. 68, 2076–2085. doi: 10.1271/bbb.68.2076
You, T., Ingram, P., Jacobsen, M. D., Cook, E., McDonagh, A., Thorne, T., et al. (2012). A systems biology analysis of long and short-term memories of osmotic stress adaptation in fungi. BMC Res. Notes 5:258. doi: 10.1186/1756-0500-5-258
Zana, M., Péterfi, Z., Kovács, H. A., Tóth, Z. E., Enyedi, B., Morel, F., et al. (2018). Interaction between p22phox and Nox4 in the endoplasmic reticulum suggests a unique mechanism of NADPH oxidase complex formation. Free Radic. Biol. Med. 116, 41–49. doi: 10.1016/j.freeradbiomed.2017.12.031
Keywords: Fusarium graminearum, NADPH oxidase, virulence, sexual development, stress
Citation: Li T, Kim D and Lee J (2022) NADPH Oxidase Gene, FgNoxD, Plays a Critical Role in Development and Virulence in Fusarium graminearum. Front. Microbiol. 13:822682. doi: 10.3389/fmicb.2022.822682
Received: 26 November 2021; Accepted: 01 February 2022;
Published: 03 March 2022.
Edited by:
Ajar Nath Yadav, Eternal University, IndiaReviewed by:
Gopal Subramaniam, Agriculture and Agri-Food Canada (AAFC), CanadaCopyright © 2022 Li, Kim and Lee. This is an open-access article distributed under the terms of the Creative Commons Attribution License (CC BY). The use, distribution or reproduction in other forums is permitted, provided the original author(s) and the copyright owner(s) are credited and that the original publication in this journal is cited, in accordance with accepted academic practice. No use, distribution or reproduction is permitted which does not comply with these terms.
*Correspondence: Jungkwan Lee, anVuZ2xlQGRhdS5hYy5rcg==
Disclaimer: All claims expressed in this article are solely those of the authors and do not necessarily represent those of their affiliated organizations, or those of the publisher, the editors and the reviewers. Any product that may be evaluated in this article or claim that may be made by its manufacturer is not guaranteed or endorsed by the publisher.
Research integrity at Frontiers
Learn more about the work of our research integrity team to safeguard the quality of each article we publish.