- 1Laboratorio de Microbiología, Departamento de Bioquímica y Biología Molecular, Facultad de Ciencias Químicas y Farmacéuticas, Universidad de Chile, Santiago, Chile
- 2Instituto de Ciencias Biomédicas, Facultad de Medicina y Facultad de Ciencias de la Vida, Universidad Andrés Bello, Santiago, Chile
- 3Escuela de Medicina Veterinaria, Facultad de Ciencias, Universidad Mayor, Santiago, Chile
- 4Escuela de Medicina Veterinaria, Facultad de Ciencias de la Vida, Universidad Andrés Bello, Santiago, Chile
- 5Escuela de Medicina Veterinaria, Facultad de Agronomía e Ingeniería Forestal, Facultad de Ciencias Biológicas y Facultad de Medicina, Pontificia Universidad Católica de Chile, Santiago, Chile
- 6Millennium Initiative on Collaborative Research on Bacterial Resistance (MICROB-R), Santiago, Chile
The Type VI Secretion System (T6SS) is a multiprotein device that has emerged as an important fitness and virulence factor for many Gram-negative bacteria through the injection of effector proteins into prokaryotic or eukaryotic cells via a contractile mechanism. While some effector proteins specifically target bacterial or eukaryotic cells, others can target both types of cells (trans-kingdom effectors). In Salmonella, five T6SS gene clusters have been identified within pathogenicity islands SPI-6, SPI-19, SPI-20, SPI-21, and SPI-22, which are differentially distributed among serotypes. Salmonella enterica serotype Dublin (S. Dublin) is a cattle-adapted pathogen that harbors both T6SSSPI-6 and T6SSSPI-19. Interestingly, while both systems have been linked to virulence and host colonization in S. Dublin, an antibacterial activity has not been detected for T6SSSPI-6 in this serotype. In addition, there is limited information regarding the repertoire of effector proteins encoded within T6SSSPI-6 and T6SSSPI-19 gene clusters in S. Dublin. In the present study, we demonstrate that T6SSSPI-6 and T6SSSPI-19 of S. Dublin CT_02021853 contribute to interbacterial competition. Bioinformatic and comparative genomic analyses allowed us to identify genes encoding three candidate antibacterial effectors located within SPI-6 and two candidate effectors located within SPI-19. Each antibacterial effector gene is located upstream of a gene encoding a hypothetic immunity protein, thus conforming an effector/immunity (E/I) module. Of note, the genes encoding these effectors and immunity proteins are widely distributed in Salmonella genomes, suggesting a relevant role in interbacterial competition and virulence. Finally, we demonstrate that E/I modules SED_RS01930/SED_RS01935 (encoded in SPI-6), SED_RS06235/SED_RS06230, and SED_RS06335/SED_RS06340 (both encoded in SPI-19) contribute to interbacterial competition in S. Dublin CT_02021853.
Introduction
Salmonellosis is a foodborne bacterial disease caused by different serotypes of Salmonella enterica (GBD-2017 et al., 2019). Worldwide, this illness is linked to 95.1 million cases of gastroenteritis per year (GBD-2017 et al., 2019). The genus Salmonella includes more than 2,600 serotypes (also referred to as serovars) distributed between species S. enterica and S. bongori (Issenhuth-Jeanjean et al., 2014), which differ in clinical signs and host range (Uzzau et al., 2000). Salmonella enterica serotype Dublin (S. Dublin) represents a cattle-adapted pathogen that can lead to serious economic problems in bovine production, where it causes a severe systemic disease (Hoelzer et al., 2011; Nielsen et al., 2013). In addition, S. Dublin may constitute a serious risk for public health due to ingestion of contaminated milk by the human population (Small and Sharp, 1979; Fierer, 1983).
The Type VI Secretion System (T6SS) has emerged as an important fitness and virulence factor for many Gram-negative bacteria (Records, 2011; Basler, 2015; Alteri and Mobley, 2016; Cianfanelli et al., 2016; Navarro-Garcia et al., 2019). The T6SS is a molecular nanomachine consisting of three main complexes: a contractile tail, a membrane complex, and a baseplate (Aschtgen et al., 2008; Zoued et al., 2014; Durand et al., 2015; Brunet et al., 2015b; Logger et al., 2016; Nazarov et al., 2018; Rapisarda et al., 2019; Yin et al., 2019). The contractile tail is composed of an internal tube generated by the polymerization of a hexameric protein called Hcp, where a needle-shaped VgrG protein trimer is assembled at the tip. VgrG proteins are often associated with proteins harboring a N-terminal PAAR motif that sharpen the tip (Ballister et al., 2008; Shneider et al., 2013; Brunet et al., 2014, 2015b; Douzi et al., 2014; Zoued et al., 2014; Renault et al., 2018). The internal tube is surrounded by a contractile sheath formed by the polymerization of TssB and TssC subunits (Leiman et al., 2009; Basler, 2015; Durand et al., 2015). Contraction of the sheath provides the energy required for the injection of effector proteins that are confined within the Hcp tube, bound to VgrG and/or associated with PAAR proteins (Silverman et al., 2013). Secreted effectors are delivered fused to VgrG and/or PAAR proteins (evolved or specialized effectors) or through non-covalent interaction with some core components (cargo effectors) (Durand et al., 2014; Whitney et al., 2014; Diniz and Coulthurst, 2015; Ma et al., 2017b; Pissaridou et al., 2018). Notably, both antibacterial and antieukaryotic effector proteins have been identified (Feria and Valvano, 2020; Hernandez et al., 2020), highlighting the role of T6SSs as key players in processes, such as interbacterial competition and host–pathogen interaction (Ma and Mekalanos, 2010; Russell et al., 2012, 2013; Koskiniemi et al., 2013; Miyata et al., 2013; Srikannathasan et al., 2013; Whitney et al., 2013; Egan et al., 2015; Bondage et al., 2016; Flaugnatti et al., 2016; Tang et al., 2018; Ting et al., 2018; Ahmad et al., 2019; Berni et al., 2019; Coulthurst, 2019; Jana et al., 2019; Mariano et al., 2019; Wood et al., 2020). Antibacterial effectors include, among others, those targeting the peptide or glycosidic bonds of peptidoglycan (Ma and Mekalanos, 2010; Russell et al., 2012; Srikannathasan et al., 2013; Whitney et al., 2013; Berni et al., 2019; Wood et al., 2019), or the FtsZ cell division ring (Ting et al., 2018). These antibacterial effectors are usually encoded along with their cognate immunity proteins in bicistronic elements known as effector/immunity (E/I) modules. Immunity proteins bind tightly and specifically to their cognate effector preventing self-intoxication and killing of sibling cells (Russell et al., 2012). Antieukaryotic effectors include, among others, those targeting the actin or microtubule cytoskeleton networks, the endoplasmic reticulum, lipid membranes, and others that activate the AIM2 inflammasome and decrease the levels of reactive oxygen species contributing to survival in macrophages (Pukatzki et al., 2007; Ma et al., 2009; Ma and Mekalanos, 2010; Miyata et al., 2011; Zheng et al., 2011; Durand et al., 2012; Lindgren et al., 2013; Schwarz et al., 2014; Heisler et al., 2015; Sana et al., 2015; Aubert et al., 2016; Jiang et al., 2016; Ray et al., 2017; Dutta et al., 2019; Tan et al., 2019; Wood et al., 2019). In addition, effectors presenting both antibacterial and antieukaryotic activity (defined as trans-kingdom effectors) include, among others, those targeting conserved molecules (e.g., NAD) or macromolecules (e.g., DNA and phospholipids), or those forming pores in biological membranes (Whitney et al., 2015; Tang et al., 2018; Ahmad et al., 2019).
In Salmonella, five T6SS gene clusters have been identified within pathogenicity islands SPI-6, SPI-19, SPI-20, SPI-21, and SPI-22 (Blondel et al., 2009; Fookes et al., 2011). These T6SSs are distributed in four different evolutionary lineages: T6SSSPI-6 belongs to subtype i3, T6SSSPI-19 to subtype i1, T6SSSPI-20 and T6SSSPI-21 to subtype i2, and T6SSSPI-22 to subtype i4a (Bao et al., 2019). In addition to their distinct evolutionary origin, these five T6SS clusters are differentially distributed among distinct serotypes, subspecies, and species of Salmonella (Blondel et al., 2009; Fookes et al., 2011).
Notably, while both T6SSSPI-6 and T6SSSPI-19 have been linked to antibacterial competition, virulence, and host colonization in different Salmonella serotypes (Blondel et al., 2010, 2013; Libby et al., 2010; Wang et al., 2011, 2019; Mulder et al., 2012; Pezoa et al., 2013; Koskiniemi et al., 2014; Brunet et al., 2015a; Sana et al., 2016; Schroll et al., 2019; Sibinelli-Sousa et al., 2020; Xian et al., 2020), there is limited information regarding the effector proteins encoded within the corresponding gene clusters among different Salmonella serotypes. Furthermore, the contribution of both T6SSs to these phenotypes seems to differ between strains within the same serotype (Schroll et al., 2019). This appears to be the case for different S. Dublin strains. While T6SSSPI-19 has been shown to be dispensable for colonization of either chickens or mice by S. Dublin CT_02021853 (Pezoa et al., 2014), it has been shown to be required for efficient intestinal colonization of mice by S. Dublin 2229 (Schroll et al., 2019). Furthermore, it has been reported that T6SSSPI-19 of S. Dublin 2229 contributes to interbacterial competition (Schroll et al., 2019), reminiscent of what has been shown for T6SSSPI-6 of S. Typhimurium (Brunet et al., 2015a; Sana et al., 2016). Interestingly, the contribution of T6SSSPI-6 to interbacterial competition has not been assessed in S. Dublin and there is a lack of information regarding the presence of antibacterial and/or antieukaryotic effector proteins encoded in the SPI-6 and SPI-19 T6SS gene clusters present in this serotype.
In the present study, we evaluated the contribution of T6SSSPI-6 and T6SSSPI-19 to interbacterial competition by S. Dublin CT_02021853 and performed an in silico analysis of both T6SS gene clusters to identify putative effector and cognate immunity proteins. First, we observed that S. Dublin CT_02021853 outcompeted a susceptible Escherichia coli strain in the presence of bile salts, as reported in the case of S. Dublin 2229 (Schroll et al., 2019). In addition, we found that both T6SSSPI-6 and T6SSSPI-19 contribute to interbacterial competition by this strain. Subsequently, a comprehensive bioinformatic analysis identified five high-confidence potential new effector and immunity proteins encoded within SPI-6 and SPI-19 T6SS gene clusters in S. Dublin CT_02021853. The bioinformatic analysis was based on four distinct criteria, including prediction of E/I modules by the Bastion6 prediction pipeline, identification of conserved domains and motifs linked to known T6SS effectors, and remote homology prediction by the HHpred HMM-HMM prediction pipeline. Finally, we confirmed the participation of E/I modules SED_RS01930/SED_RS01935, SED_RS06235/SED_RS06230, and SED_RS06335/SED_RS06340 of S. Dublin CT_02021853 in interbacterial competition.
Materials and Methods
Bacterial Strains and Growth Conditions
The bacterial strains used in this study are listed in Table 1. Bacteria were routinely grown in Luria-Bertani (LB) broth (10 g/L tryptone, 5 g/L yeast extract, 5 g/L NaCl) at 37°C with aeration. LB broth was supplemented with ampicillin (Amp; 100 μg/ml), kanamycin (Kan; 50 μg/ml), chloramphenicol (Cam; 20 μg/ml), or nalidixic acid (Nal; 15 μg/ml), as needed. LB medium was solidified by addition of agar (15 g/L). For interbacterial competition assays bacteria were incubated on MacConkey agar plates at 37°C for 24 h.
Standard DNA Techniques
Plasmid DNA was isolated using the “QIAprep Spin Miniprep Kit” (QIAGEN, MD, United States). PCR products were purified using the “QIAquick PCR Purification Kit” (QIAGEN, MD, United States). DNA samples were analyzed by electrophoresis in 1% agarose gels and were visualized under UV light after RedGel (Biotium, CA, United States) staining. Primers were designed using the “Vector NTI Advance 11.0” software (Invitrogen, CA, United States) and are listed in Table 2. PCR products were amplified in a “MultiGene TC9600-G” thermal cycler (LabNet, NJ, United States). PCR reaction mixes contained 1X buffer, 2 mM MgCl2, 100 nM dNTPs, 100 nM of each primer, 100 ng of template DNA and 0.5–1 U of HiFi DNA pol (KAPA, MA, United States). Standard conditions for amplification were: 2 min at 95°C, followed by 30–35 cycles of 94°C for 45 s, 55°C for 30 s, and 72°C for a suitable time (1 min/kb) according to DNA polymerase processivity, and a final extension step at 72°C for 5 min.
Construction of Salmonella Dublin Mutant Strains
Derivatives of S. Dublin CT_02021853 with deletions of single genes or gene clusters were constructed by the one-step inactivation procedure using the Lambda Red recombination system (Datsenko and Wanner, 2000), with modifications (Santiviago et al., 2009). The oligonucleotides used for mutagenesis (Table 2) were designed with 40 bases at the 5′ ends identical to the ends of the corresponding deletion, and 20 bases at the 3′ ends that anneal with the 5′ or 3′ end of a Cam or Kan resistance cassette flanked by Flp recombinase target (FRT) sites present in plasmids pCLF2 (GenBank accession number HM047089) and pCLF4 (GenBank accession number EU629214.1), respectively. These plasmids were used as templates for the corresponding amplification of PCR products. S. Dublin CT_02021853 carrying the plasmid pKD46, which expresses the Lambda Red recombination system, was grown to an OD600nm of 0.6 at 30°C in LB broth supplemented with Amp and L-arabinose (10 mM). Then, bacteria were made electrocompetent by serial washes with ice-cold, sterile 15% glycerol and transformed by electroporation with 500–600 ng of each PCR product. Transformants were selected on LB agar supplemented with the corresponding antibiotic at 37°C. Correct allelic replacement in each mutant was confirmed by PCR amplification using specific forward primers (Out5) together with reverse primer K1 (that hybridizes within the Kan resistance cassette) or reverse primer C3 (that hybridizes within the Cam resistance cassette; Table 2).
When required, the antibiotic resistance cassette was removed by transforming each mutant with the temperature-sensitive plasmid pCP20, which encodes the Flp recombinase (Cherepanov and Wackernagel, 1995). Transformants were selected at 30°C on LB agar plates containing Amp. A few colonies were streaked two consecutive times at 37°C on LB agar plates and tested for the loss of the antibiotic resistance cassettes and pCP20 by patching them on LB agar plates containing Kan or Cam plus Amp. The absence of the antibiotic resistance cassette was confirmed by PCR amplification using primers flanking the sites of substitution (Table 2). Finally, to differentiate between S. Dublin strains in our interbacterial competition assays, a Kan or Cam resistance cassette was incorporated at a neutral position (i.e., replacing the ORF of phoN gene) in the chromosome of the attacker or the prey strain, respectively. To do this, phage P22 HT 105-1 int-201 was used to transduce mutant alleles ΔphoN::Kan and ΔphoN::Cam into the corresponding mutant strains harboring unmarked deletions of genes encoding effectors or E/I protein pairs.
Interbacterial Competition Assays
Competition assays were performed as described (Ma et al., 2018), with modifications. Briefly, attacker and prey bacteria were grown overnight in LB broth at 37°C. An aliquot (1 ml) of each culture was spun down, and the supernatant was discarded. Each bacterial pellet was washed three times in PBS, adjusted to an OD600nm of 0.5, and mixed at a 1:1 (attacker/prey) ratio. Then, 25 μl of the mixture was incubated at 37°C for 24 h in triplicate on MacConkey agar plates, a condition reported to induce the expression of T6SS gene clusters in Salmonella (Schroll et al., 2019). After incubation, the bacterial mixtures were scraped from the plates and resuspended in 1 ml of PBS, and CFU were determined by plating of serial dilutions on LB agar supplemented with suitable antibiotics. Statistical significance was determined using a one-way ANOVA followed by Tukey’s multiple comparisons test using GraphPad Prism 9.0 software.
Bioinformatics Analyses
To identify putative T6SS effectors encoded within SPI-6 and SPI-19 in S. Dublin CT_02021853, each ORF of both pathogenicity islands was analyzed with the Bastion6 pipeline (Wang et al., 2018) excluding those encoding the 13 structural components of the T6SS. ORFs presenting a Bastion6 score ≥ 0.7 were considered as putative T6SS effectors. Each Bastion6 prediction was also analyzed to determine if it was part of a bicistron encoding a putative immunity protein [i.e., a small protein with potential signal peptides (SignalP) and/or transmembrane domains (TMHMM)] with the Operon-Mapper web server (Taboada et al., 2018). Identification of conserved functional domains and motifs was performed using the PROSITE, NCBI-CDD, Motif-finder, and Pfam databases (Kanehisa et al., 2002; Sigrist et al., 2013; Finn et al., 2014; Lu et al., 2019) with the GenomeNet search engine. An e-value cutoff score of 0.01 was used. Finally, for each putative effector and immunity protein identified, a biochemical functional prediction was performed via HMM homology searches using the HHpred HMM-HMM comparison tool (Zimmermann et al., 2017).
Sequence and Phylogenetic Analysis
Identification of T6SS effector orthologs was carried out using the DNA sequence of each effector/immunity module in BLASTn analyses using publicly available bacterial genome sequences of the NCBI database (January 2021). An 80% identity and 80% sequence coverage threshold were used to select positive matches. Sequence conservation was analyzed by multiple sequence alignments using MAFFT (Katoh et al., 2017) and T-Coffee Expresso (Notredame et al., 2000) and visualized by ESPript 3 (Robert and Gouet, 2014). Comparative genomic analysis of T6SS gene clusters was performed using the multiple aligner Mauve (Darling et al., 2004) and EasyFig v2.2.2 (Sullivan et al., 2011). Nucleotide sequences were analyzed by the sequence visualization and annotation tool Artemis version 18 (Rutherford et al., 2000).
Results and Discussion
The T6SSs Encoded in SPI-6 and SPI-19 Contribute to Interbacterial Competition by S. Dublin CT_02021853
To determine the contribution of T6SSSPI-6 and T6SSSPI-19 to interbacterial competition by S. Dublin CT_02021853, we performed a competition assay in MacConkey agar as it has been shown that bile salts upregulate the expression of T6SS-related genes in S. Typhimurium and S. Dublin strains (Brunet et al., 2015a; Sana et al., 2016; Schroll et al., 2019). We decided to use mutant strains lacking the complete SPI-6 and/or SPI-19 T6SS gene clusters rather than single mutants in key structural components to avoid potential cross-complementation between components of both T6SS gene clusters as reported in other systems (Santos et al., 2020). We observed that the E. coli DH5α prey strain was significantly outcompeted after coincubation with S. Dublin CT_02021853. Of note, the ability of derivative strains ΔT6SSSPI-6 and ΔT6SSSPI-19 to outcompete the E. coli prey strain was similar to the wild-type strain, recovering 1,000-fold lower CFU of E. coli after coincubation (Figure 1). Remarkably, the ability of a ΔT6SSSPI-6 ΔT6SSSPI-19 double mutant to outcompete the E. coli prey strain was 100-fold lower than the wild-type strain and derivative strains ΔT6SSSPI-6 and ΔT6SSSPI-19 (Figure 1). These results indicate that T6SSSPI-6 and T6SSSPI-19 provide a competitive advantage to S. Dublin CT_02021853 over E. coli DH5α. Furthermore, having both T6SS systems does not provide an additive advantage over having only one, revealing a functional redundancy between both systems.
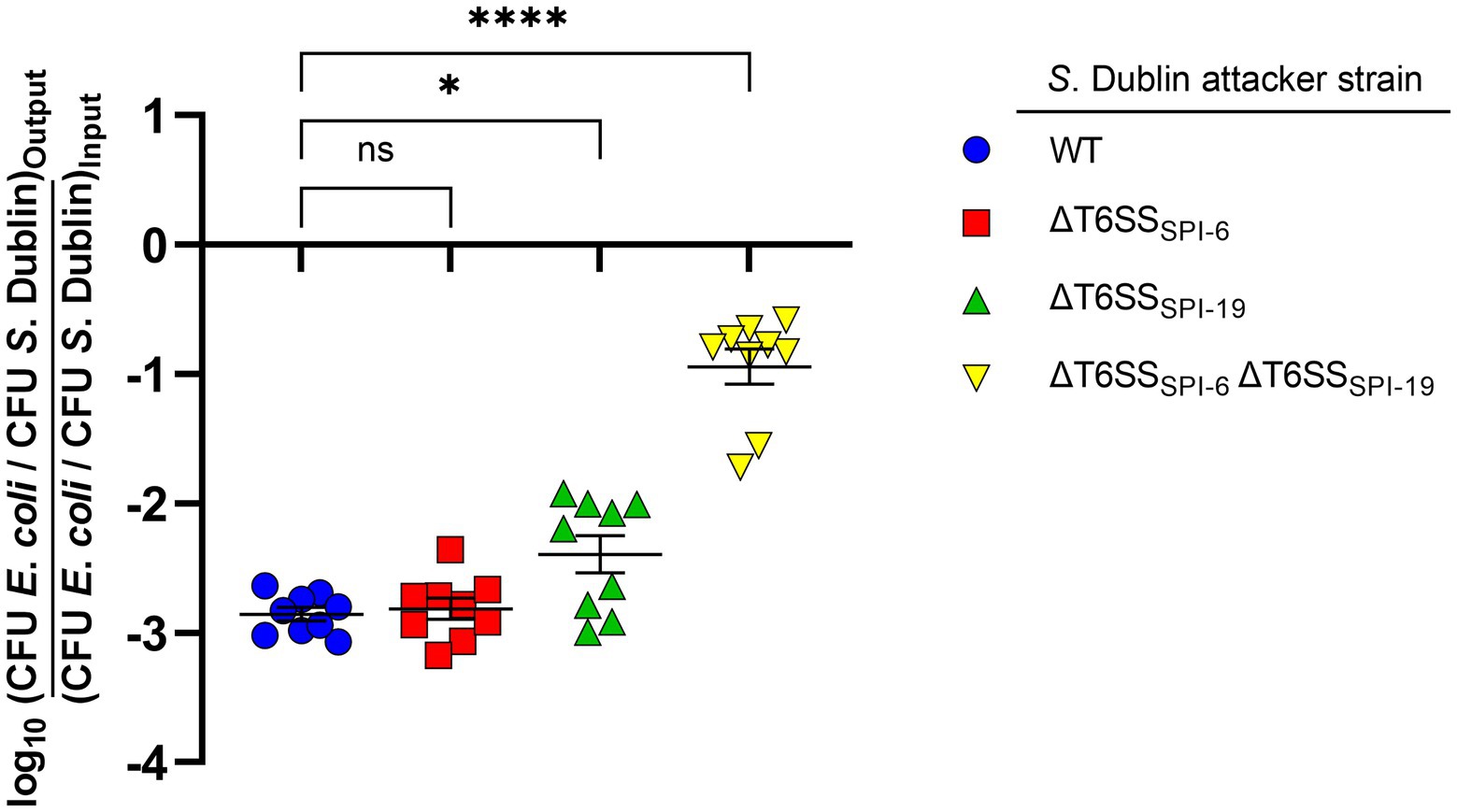
Figure 1. Contribution of T6SSSPI-6 and T6SSSPI-19 to interbacterial competition by S. Dublin strain CT_02021853. Wild-type and mutant strains ΔT6SSSPI-6, ΔT6SSSPI-19, and ΔT6SSSPI-6 ΔT6SSSPI-19 of S. Dublin CT_02021853 were mixed at a ratio of 1:1 (attacker/prey) with Escherichia coli DH5α. Then, 25 μl of the mixture was incubated at 37°C for 24 h in triplicate on MacConkey agar plates. Bacterial counts recovered from each competition assay were calculated by plating serial 10-fold dilutions on LB agar plates with the appropriate antibiotics (Nal in the case of E. coli and Kan or Cam in the case of S. Dublin strains). Data show the ratio of E. coli CFU (prey) to S. Dublin CFU (attacker) normalized to the inoculum ratio and expressed as log10. Error bars indicate standard error. Statistical significance was determined using a one-way ANOVA followed by Tukey’s multiple comparisons test (*p < 0.05; ****p < 0.0001; ns, not significant).
The SPI-6 of S. Dublin CT_02021853 Encodes 3 Novel Putative T6SS Effector Proteins
S. Dublin CT_02021853 encodes 3 out of 4 antibacterial E/I pairs, as well as the two orphan immunity proteins, previously described in the SPI-6 T6SS gene cluster of S. enterica (Figure 2A): (i) Tae2/TaeI2 E/I pair (SED_RS26190/SED_RS01845) present in different Salmonella serotypes (Russell et al., 2012), (ii) the RHSmain (SED_RS01910) effector protein (Koskiniemi et al., 2014), and (iii) the Tlde1/Tldi1 E/I pair (SED_RS01895/SED_RS01890; Sibinelli-Sousa et al., 2020). In contrast, S. Dublin CT_02021853 does not encode the Tae4/Tai4 and RHSorphan/RhsI E/I pairs described in S. Typhimurium 14028s (Blondel et al., 2009; Koskiniemi et al., 2014; Figure 2A).
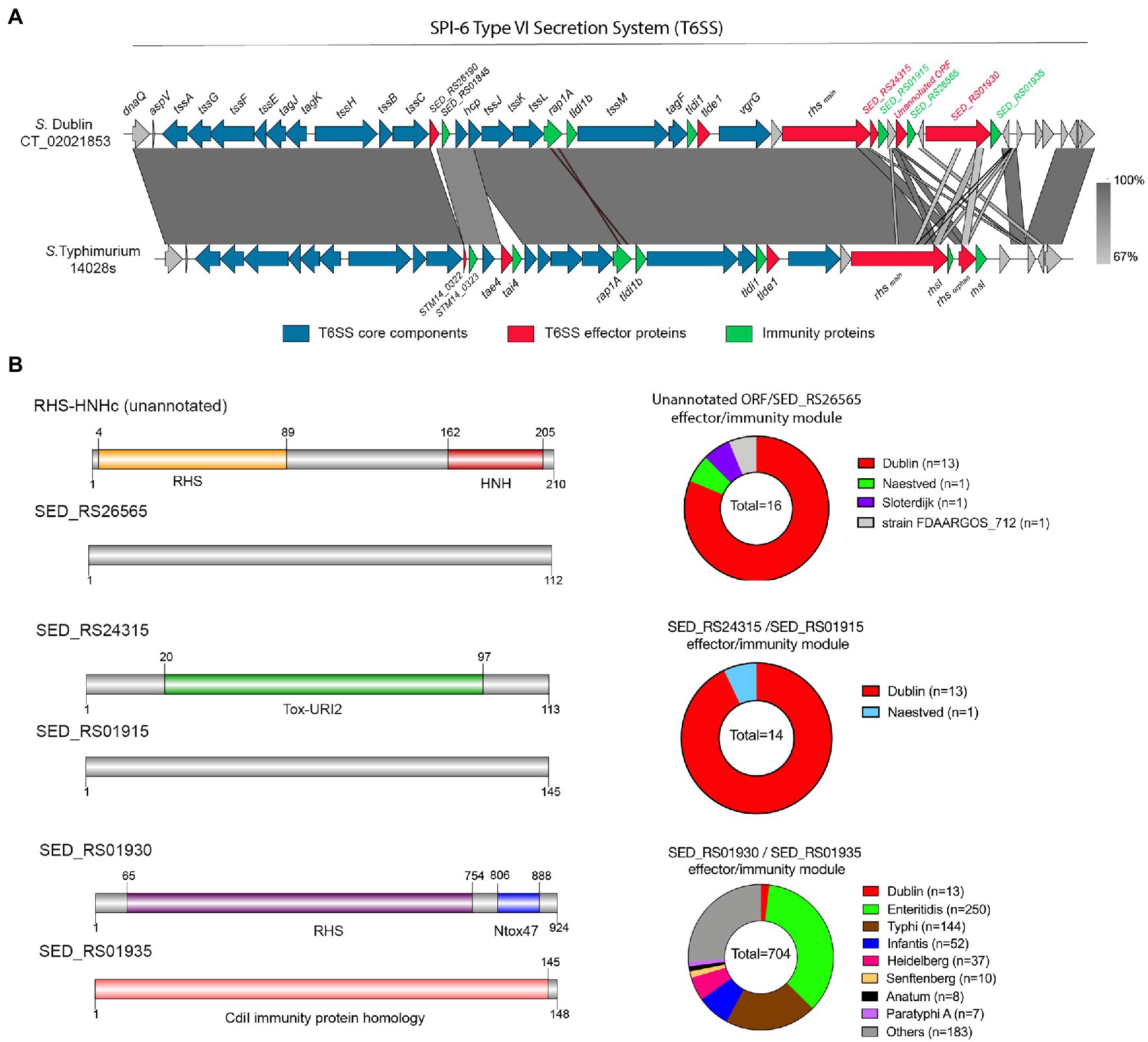
Figure 2. The SPI-6 T6SS gene cluster encodes novel putative Type VI Secretion Systems (T6SS) effector proteins. (A) Comparative genomic analysis of the SPI-6 T6SS cluster of S. Dublin CT_02021853 and S. Typhimurium 14028s. BLASTn sequence alignment was performed and visualized using EasyFig (Sullivan et al., 2011). (B) Schematic representation and distribution among Salmonella genomes of each novel effector and immunity protein identified. Names of genes encoding novel effectors and immunity proteins are highlighted in red and green, respectively. Homologs for each component were identified by BLASTn analyses as described in Materials and Methods.
To gain insight into the T6SS-dependent antibacterial activity of S. Dublin, we performed bioinformatic and comparative genomic analyses to identify potential novel T6SS effector proteins and their cognate immunity proteins. To do this, each ORF encoded within the SPI-6 T6SS gene cluster of S. Dublin CT_02021853 was analyzed based on four criteria, including: (i) analysis by the Bastion6 prediction pipeline (a bioinformatics tool that predicts T6SS effectors based on amino acids sequence profile, evolutionary information, and physicochemical properties); (ii) bioinformatic analysis to identify the presence of putative immunity proteins through detection of signal peptide (SignalP), transmembrane domains (TMHMM), and operon prediction (Operon-mapper; Taboada et al., 2018); (iii) identification of conserved domains and motifs linked to known T6SS effectors (PROSITE, NCBI-CDD, Motif-finder, and Pfam databases); and (iv) functional predictions via HMM homology searches using the HHpred HMM-HMM prediction pipeline (Zimmermann et al., 2017). In addition, we analyzed the SPI-6 T6SS gene cluster to identify potential unannotated ORFs which could encode putative effectors and cognate immunity proteins. Our analysis identified three potential E/I pairs encoded within this T6SS gene cluster of S. Dublin CT_02021853 (Figure 2B; Table 3).
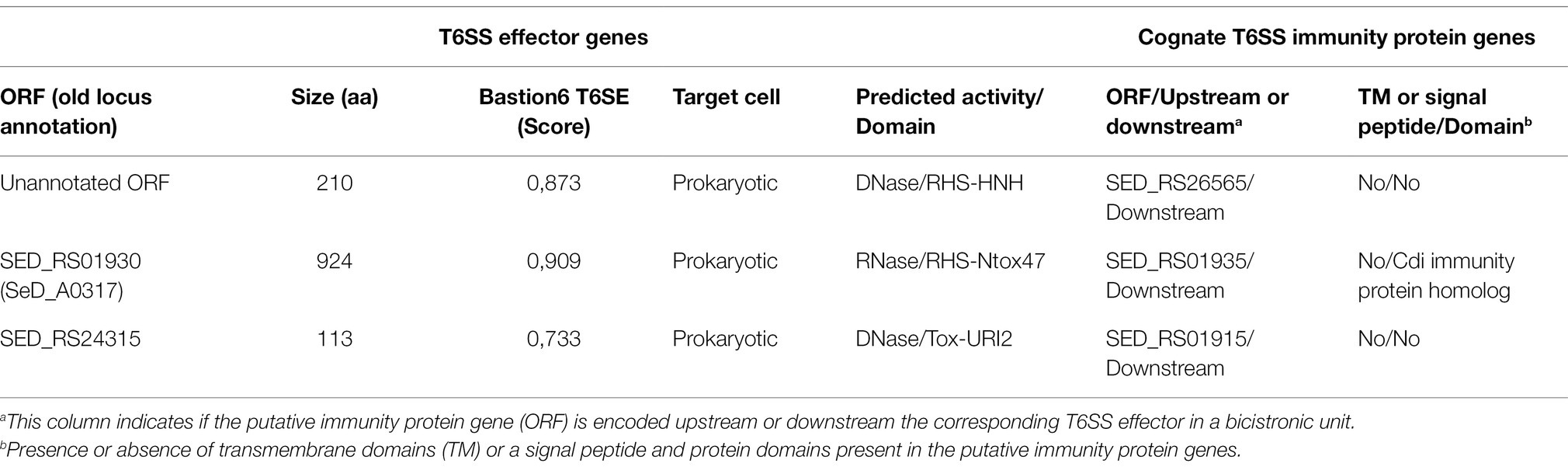
Table 3. Novel predicted T6SS effectors and cognate immunity proteins encoded in SPI-6 of Salmonella Dublin CT_02021853.
First, we identified an unannotated ORF located between the SED_RS24320 and SED_RS24325 ORFs. This novel ORF (Bastion6 score = 0,873) encodes a 210 amino acid protein with a predicted endonuclease RHS-HNHc protein domain (Figure 2B; Table 3). The presence of the HNHc endonuclease domain suggests that this putative protein harbors DNase activity, as described for other antibacterial and antieukaryote T6SS effector proteins (Ma et al., 2017a,b). SED_RS24325 is predicted to be co-transcribed with the downstream ORF SED_RS26565, suggesting that this latter ORF is the cognate immunity protein of the putative novel effector.
A second putative effector protein was predicted to be encoded by SED_RS1930. This ORF (Bastion6 score = 0,909) encodes a large 924 amino acid protein with both a RHS and an Ntox47 protein domain, involved in degrading target cell RNA (Zhang et al., 2012). It should be noted that the Ntox47 protein domain of SED_RS1930 has similarity to the Ntox47 domain of RHSorphan protein of S. Typhimurium 14028s (Figure 2B). Since RHS orphan proteins have been linked to recombination events between RHS elements generating RHSmain-orphan chimeras (Koskiniemi et al., 2014), it is plausible that SED_RS1930 was generated through one of such events.
Finally, a third effector protein was predicted to be encoded in SED_RS24315. This ORF (Bastion6 score = 0,733) encodes a small 113 amino acid protein with similarity to bacterial polymorphic toxins in the Tox-URI2 family, a DNAse protein domain that has been linked to RHS-CT proteins exported by the T6SS (Zhang et al., 2012; Jamet and Nassif, 2015; Ma et al., 2017b). SED_RS24315 is predicted to be co-transcribed with SED_RS01915, suggesting that this latter ORF encodes the cognate immunity protein of the putative effector. Interestingly, the ORF just upstream of SED_RS24315 encodes the RHSmain protein (SED_RS01910). Taking into account that there are RHS proteins with C-terminal Tox-URI2 domains (Zhang et al., 2012; Ma et al., 2017b), it is possible that SED_RS01910 and SED_RS24315 were at some point a single ORF that was later split due to the accumulation of nonsense mutations.
Many T6SS-associated RHS proteins have C-terminal endonuclease effector domains, which degrade DNA or RNA in the target cell (Zhang et al., 2012). Interestingly, RHS proteins have YD-peptide repeats, which fold into a large β-cage structure that encapsulates and protects the C-terminal toxin domain and highly increase T6SS secretion efficiency (Donato et al., 2020), which could explain why many T6SS effectors are associated with RHS elements.
The SPI-19 of S. Dublin CT_02021853 Encodes 2 Putative T6SS Effector Proteins
As mentioned, no effector protein has been described to be encoded within the SPI-19 T6SS gene cluster in Salmonella, despite its clear contribution to intestinal colonization and antibacterial activity (Blondel et al., 2010; Pezoa et al., 2014; Schroll et al., 2019; Xian et al., 2020). To identify novel T6SS effector proteins encoded within SPI-19, we performed bioinformatic analyses of each ORF included in this T6SS gene cluster of S. Dublin CT_02021853. In addition, we performed a comparative analysis of this T6SS gene cluster with the SPI-19 T6SS gene cluster of S. Gallinarum SG9, as T6SSSPI-19 activity in this strain causes cytotoxicity to primary macrophages from hens (Schroll et al., 2019).
Our analysis identified two putative T6SS effector proteins encoded in SPI-19 (Figure 3; Table 4), that are conserved in S. Gallinarum SG9 (Figure 3A). The first putative effector corresponds to SED_RS06335 (Bastion6 score = 0,939), an RHS protein that harbors an N-terminal PAAR domain and a C-terminal M91 metallopeptidase domain (Figure 3B). Our analysis showed that SED_RS06335 is part of a bicistronic unit with SED_RS06340. This latter ORF encodes a 110 amino acid protein with a transmembrane domain that may correspond to the cognate immunity protein of SED_RS06335. A comparative analysis with the SPI-19 T6SS gene cluster of S. Gallinarum SG9 revealed the presence of two copies of genes encoding this PAAR-RHS effector protein in this strain (SG9_1071 and SG9_1075; Figure 3A). Interestingly, a M91 metallopeptidase domain is present in the trans-kingdom effector VgrG2b of Pseudomonas aeruginosa (Wood et al., 2019). This M91 domain of VgrG2b has been shown to be involved in the internalization of P. aeruginosa into host cells by interacting with the host gamma-tubulin ring complex (Sana et al., 2015) and in the antibacterial activity of the H2-T6SS (Wood et al., 2019). Our analysis of SED_RS06335 showed the presence of the HEXXH motif typically found in the catalytic site of metallopeptidases (Supplementary Figure S1).
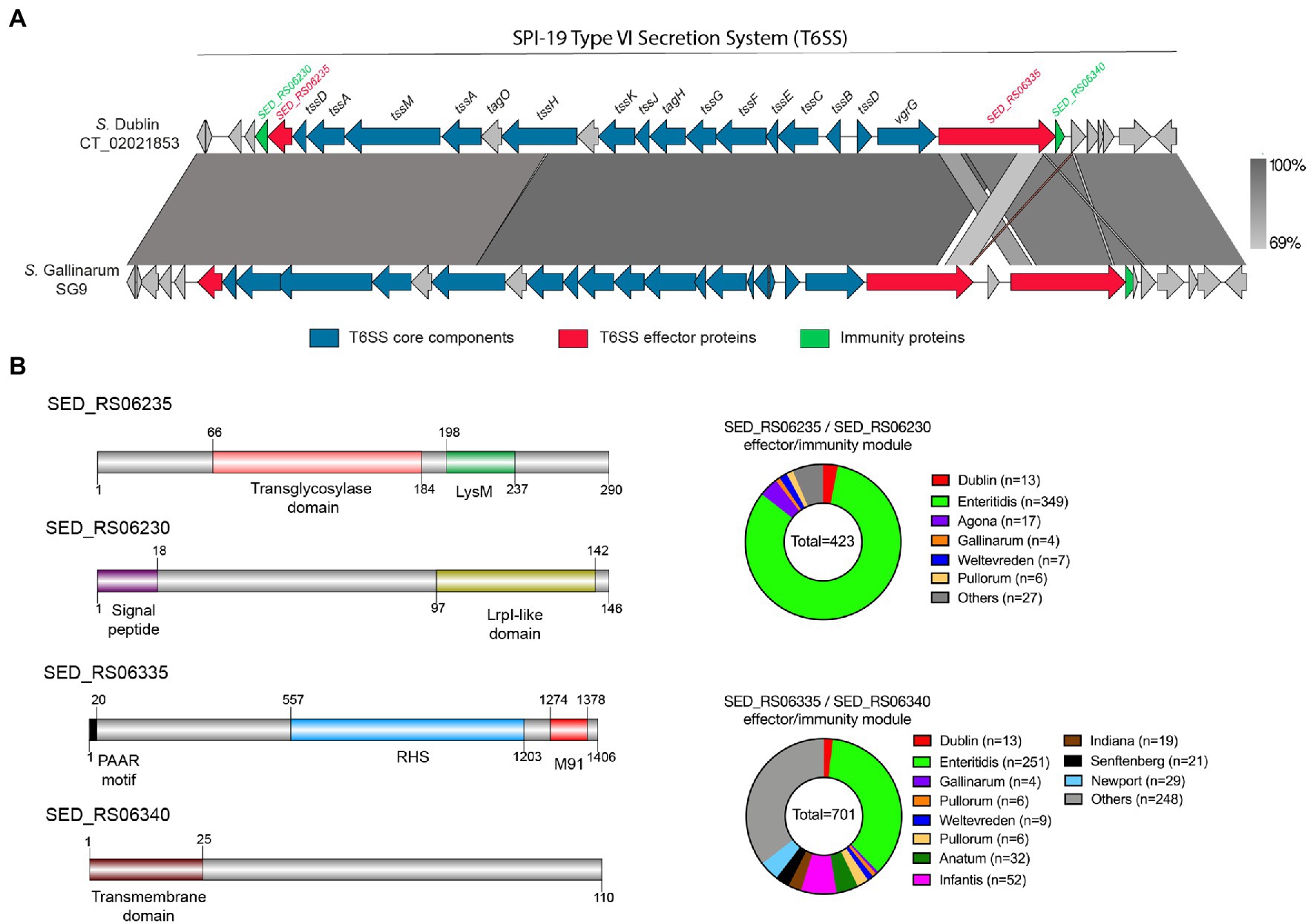
Figure 3. The SPI-19 T6SS gene cluster encodes novel putative T6SS effector proteins. (A) Comparative genomic analysis of the SPI-19 T6SS cluster of S. Dublin CT_02021853 and S. Gallinarum SG9. BLASTn sequence alignment was performed and visualized using EasyFig (Sullivan et al., 2011). (B) Schematic representation and distribution among Salmonella genomes of each novel effector and immunity protein identified. Names of genes encoding novel effectors and immunity proteins are highlighted in red and green, respectively. Homologs for each component were identified by BLASTn analyses as described in Materials and Methods.
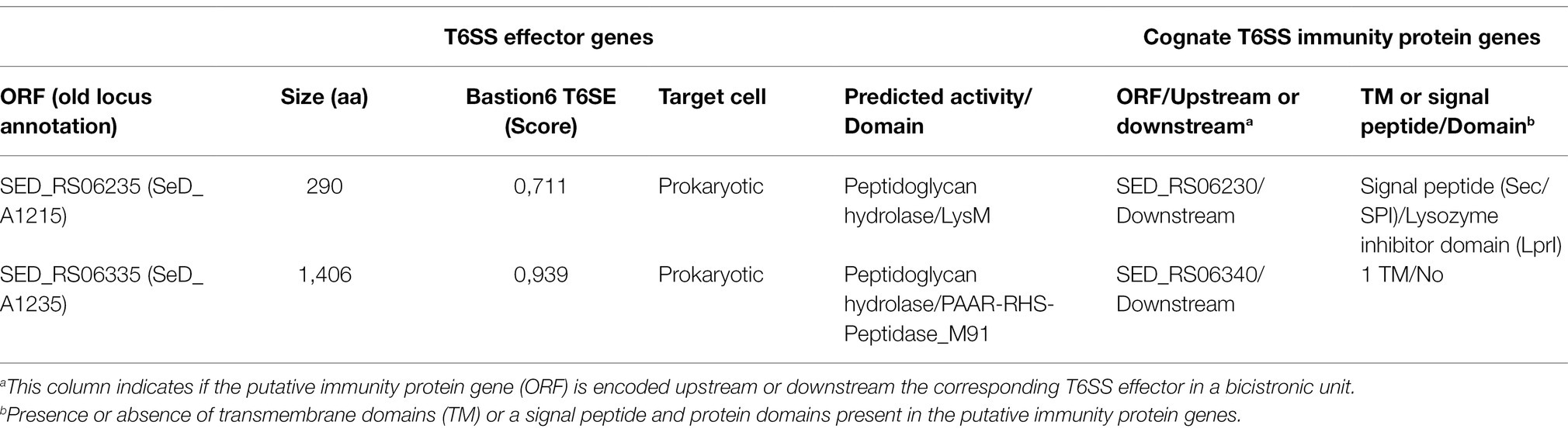
Table 4. Novel predicted T6SS effectors and cognate immunity proteins encoded in SPI-19 of Salmonella Dublin CT_02021853.
Besides SED_RS06335, our bioinformatic analysis also identified another effector protein encoded within the SPI-19 T6SS gene cluster in S. Dublin CT_02021853. This putative effector protein is encoded in SED_RS06235 (Bastion6 score = 0,711). This ORF encodes a 290 amino acid protein with a predicted LysM domain (IPR018392) at its C-terminus. This domain is present in several T6SS effector proteins and has been linked to peptidoglycan hydrolase activity (Fitzsimons et al., 2018), suggesting that SED_RS06235 is a novel T6SS amidase effector protein with antibacterial activity. Indeed, HHPred analysis revealed the presence of a transglycosylase domain in the N-terminal region of SED_RS06235, which is consistent with the notion that this ORF encodes an antibacterial effector protein. Our analysis also revealed that SED_RS06235 is part of a bicistronic unit along with SED_RS06230. This ORF encodes a 146 amino acid protein with a periplasmic-targeting signal peptide. A sequence analysis revealed that SED_RS06230 harbors the LprI PFAM domain (PF07007). This domain is found in LprI, a Mycobacterium tuberculosis protein which functions as a lysozyme inhibitor (Sethi et al., 2016). This suggests that SED_RS06235/SED_RS06230 corresponds to a LysM/LysMI E/I pair. Interestingly, the LysM effector of S. Enteritidis (SEN1001) has been linked to the ability of the bacteria to colonize the murine host and survive within infected macrophages (Silva et al., 2012). Since SPI-19 has a large internal deletion that inactivated the T6SSSPI-19 in S. Enteritidis, the mechanism involved in these processes remains unknown.
The Identified Putative T6SS Effector and Immunity Proteins Are Widely Distributed in Salmonella Genomes
The identification of five putative T6SS effector proteins encoded within SPI-6 and SPI-19 T6SS gene clusters of S. Dublin CT_02021853 prompted us to investigate the presence and distribution of these proteins in Salmonella. To this end, the sequence of each E/I module identified in this study was used in BLASTn searches using publicly available bacterial genome sequences and the distribution of each effector protein was determined (Figures 2B, 3B; Supplementary Table S1).
The analysis of the three putative effector proteins encoded in SPI-6 showed that only a small number of genomes harbors the unannotated E/I module SED_RS24315/SED_RS01915, and they were almost restricted to S. Dublin strains (Figure 2B). In contrast, the SED_RS01930/SED_RS01935 E/I module was found in the genome of most Salmonella serotypes that harbor SPI-6, including a large number of S. Enteritidis strains (33%). Of note, S. Enteritidis strains harbor an internal deletion within the SPI-6 T6SS gene cluster that is located upstream of the SED_RS01930/SED_RS01935 E/I module (Blondel et al., 2009).
The two putative effector proteins encoded in SPI-19 that we identified were also widely distributed among Salmonella serotypes (Figure 3B). Our analysis showed that the SED_RS06235/SED_RS06230 E/I module is present in the genome of strains of every Salmonella serotype harboring SPI-19. However, the SED_RS06335/SED_RS06340 E/I module was found in a larger number of Salmonella genomes, including those not previously described to carry SPI-19 (Supplementary Table S1). As in the case of the SED_RS01930/SED_RS01935 E/I pair encoded in SPI-6, a large number of S. Enteritidis strains were also found to encode the two T6SSSPI-19 effector proteins identified in this study. Although S. Enteritidis strains harbor large deletions within SPI-6 and SPI-19 that most likely make the corresponding T6SSs non-functional (Blondel et al., 2009), the presence of multiple E/I modules in these clusters suggests that S. Enteritidis retains immunity to many T6SSSPI-6 and T6SSSPI-19 effector proteins.
Contribution of Selected E/I Modules to Interbacterial Competition in S. Dublin CT_02021853
We aimed to evaluate if a selected group of E/I modules identified by our bioinformatic analysis contribute to interbacterial competition in S. Dublin. To this end, we chose E/I modules SED_RS01930/SED_RS01935 (located in SPI-6), SED_RS06235/SED_RS06230, and SED_RS06335/SED_RS06340 (both located in SPI-19) and generated non-polar deletion mutants of either the effector-encoding gene or the whole E/I module in S. Dublin CT_02021853. Next, we performed interbacterial competition assays using different combinations of attacker and prey strains. Noteworthy, in all three E/I modules analyzed we observed that the survival of the mutant lacking the effector-encoding gene (and keeping intact the gene encoding the cognate immunity protein) was not affected after coincubation with the wild-type strain (Figure 4). These results indicate that each immunity protein is able to counteract the toxic activity of the cognate effector protein delivered by the attacker. In contrast, the survival of the mutant lacking the whole E/I module was reduced after coincubation with the wild-type strain. In fact, single mutants lacking E/I module SED_RS01930/SED_RS01935, SED_RS06235/SED_RS06230, and SED_RS06335/SED_RS06340 were recovered 2-, 1.5-, and 2.4-fold less than the wild-type after coincubation, respectively (Figure 4). Finally, the survival of mutants lacking either the effector-encoding gene or the corresponding E/I module were identical when they were coincubated (Figure 4). These results confirm that modules SED_RS01930/SED_RS01935, SED_RS06235/SED_RS06230, and SED_RS06335/SED_RS06340 contribute to interbacterial competition mediated by either T6SSSPI-6 or T6SSSPI-19 in S. Dublin CT_02021853. Also, these results indicate that SED_RS01930, SED_RS06235, and SED_RS06335 encode functional effector proteins, while SED_RS01935, SED_RS06230, and SED_RS06340 encode their cognate immunity proteins. Further experimental evidence is required to fully confirm that each identified effector protein is secreted via T6SSSPI-6 and/or T6SSSPI-19.
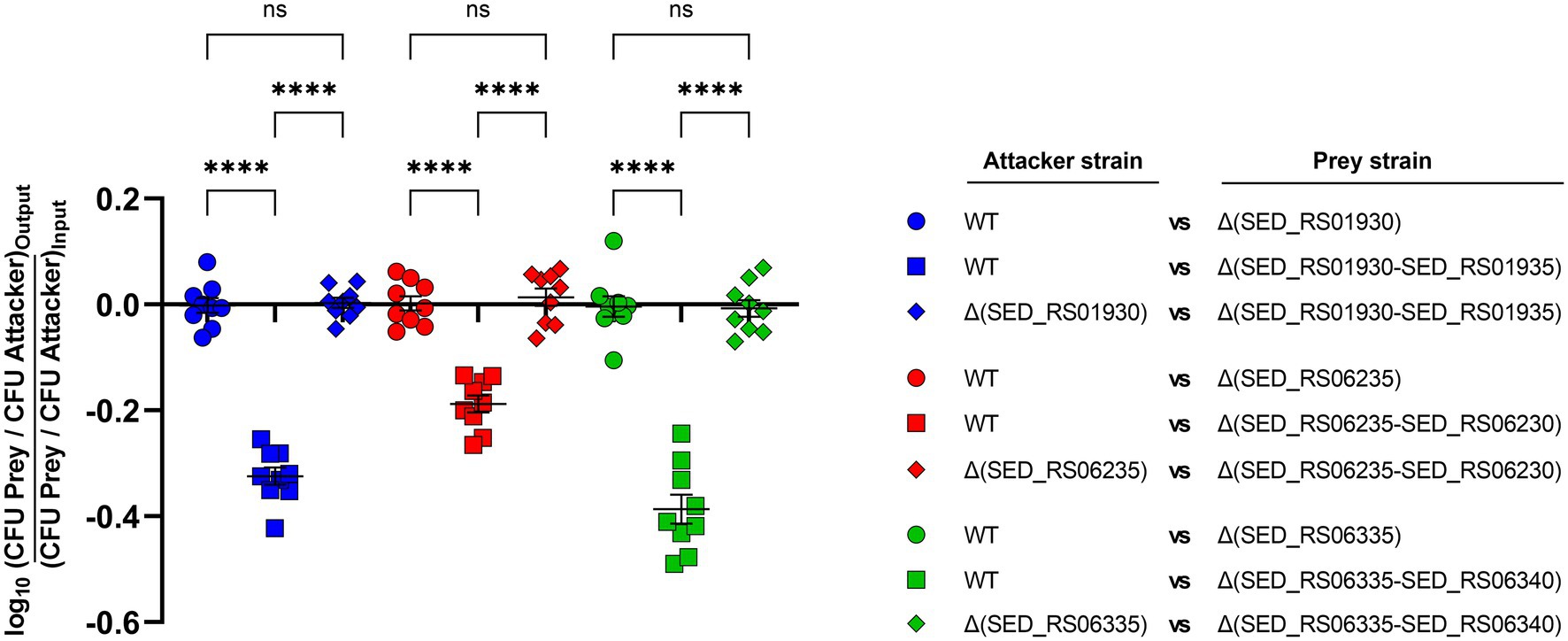
Figure 4. Contribution of selected effectors and effector/immunity protein pairs to interbacterial competition in S. Dublin. Bacterial suspensions of attacker and prey strains adjusted to an OD600nm of 0.5 were mixed at a 1:1 ratio. Then, 25 μl of the mixture was incubated at 37°C for 24 h in triplicate on MacConkey agar plates. Bacterial counts recovered from each competition assay were calculated by plating serial 10-fold dilutions on LB agar plates with the appropriate antibiotics (Kan in the case of the attacker strain and Cam in the case of the prey strain). Data show the prey to attacker CFU ratio normalized to the inoculum and expressed as log10. Error bars indicate standard error. Statistical significance was determined using a one-way ANOVA followed by Tukey’s multiple comparisons test (****p < 0.0001; ns, not significant).
Conclusions
Altogether, our results indicate that both T6SSSPI-6 and T6SSSPI-19 contribute to interbacterial competition by S. Dublin CT_02021853. We identified novel effector proteins and cognate immunity proteins encoded in SPI-6 and SPI-19 T6SS gene clusters and confirmed the contribution of three novel E/I pairs to interbacterial competition in S. Dublin CT_02021853. The biochemical characterization of the putative effector proteins identified is currently undergoing in our laboratory.
The question of why S. Dublin acquired and maintained two distinct and functional T6SSs remains unanswered. We and others have proposed that the presence of multiple T6SSs could contribute to Salmonella fitness in different environments and/or hosts. Thus, the presence of putative antibacterial T6SS effector proteins suggests that S. Dublin may use them to differentially target bacterial cells within the cattle microbiota. Additional studies are required to confirm this hypothesis.
Data Availability Statement
The original contributions presented in the study are included in the article/Supplementary Material; further inquiries can be directed to the corresponding authors.
Author Contributions
FA, CB, CS, and DP: conceptualization, formal analysis, validation, writing-original draft preparation, and writing-review and editing. FA, CB, MB-I, DR, CS, and DP: methodology and investigation. CB, CS, AM-S, and DP: resources, project administration, and funding acquisition. CS and DP: supervision. FA, CB, and DP: visualization. All authors read and approved the final manuscript.
Funding
This work was supported by FONDECYT grant 1181167, ANID Millennium Science Initiative/Millennium Initiative for Collaborative Research on Bacterial Resistance, MICROB-R, NCN17_081. CS was supported by FONDECYT grants 1171844, and 1212075. CB was supported by FONDECYT grant 1201805, ECOS-ANID ECOS200037, and HHMI-Gulbenkian International Research Scholar grant #55008749. FA was supported by CONICYT/ANID fellowship 21191925.
Conflict of Interest
The authors declare that the research was conducted in the absence of any commercial or financial relationships that could be construed as a potential conflict of interest.
Publisher’s Note
All claims expressed in this article are solely those of the authors and do not necessarily represent those of their affiliated organizations, or those of the publisher, the editors and the reviewers. Any product that may be evaluated in this article, or claim that may be made by its manufacturer, is not guaranteed or endorsed by the publisher.
Acknowledgments
We thank Andrea Sabag for technical assistance.
Supplementary Material
The Supplementary Material for this article can be found online at: https://www.frontiersin.org/articles/10.3389/fmicb.2022.811932/full#supplementary-material
References
Ahmad, S., Wang, B., Walker, M. D., Tran, H.-K. R., Stogios, P. J., Savchenko, A., et al. (2019). An interbacterial toxin inhibits target cell growth by synthesizing (p)ppApp. Nature 575, 674–678. doi: 10.1038/s41586-019-1735-9
Alteri, C. J., and Mobley, H. L. T. (2016). “The versatile type VI secretion system,” in Virulence Mechanisms of Bacterial Pathogens. (Washington, DC, USA: ASM Press), 337–356.
Aschtgen, M.-S., Bernard, C. S., Bentzmann, S. D., Lloubès, R., and Cascales, E. (2008). SciN is an outer membrane lipoprotein required for type VI secretion in enteroaggregative Escherichia coli. J. Bacteriol. 190, 7523–7531. doi: 10.1128/jb.00945-08
Aubert, D. F., Xu, H., Yang, J., Shi, X., Gao, W., Li, L., et al. (2016). A Burkholderia type VI effector deamidates rho GTPases to activate the pyrin inflammasome and trigger inflammation. Cell Host Microbe 19, 664–674. doi: 10.1016/j.chom.2016.04.004
Ballister, E. R., Lai, A. H., Zuckermann, R. N., Cheng, Y., and Mougous, J. D. (2008). In vitro self-assembly of tailorable nanotubes from a simple protein building block. Proc. Natl. Acad. Sci. U.S.A. 105, 3733–3738. doi: 10.1073/pnas.0712247105
Bao, H., Zhao, J.-H., Zhu, S., Wang, S., Zhang, J., Wang, X.-Y., et al. (2019). Genetic diversity and evolutionary features of type VI secretion systems in Salmonella. Future Microbiol. 14, 139–154. doi: 10.2217/fmb-2018-0260
Basler, M. (2015). Type VI secretion system: secretion by a contractile nanomachine. Philos. Trans. R. Soc. Lond. B Biol. Sci. 370:20150021. doi: 10.1098/rstb.2015.0021
Berni, B., Soscia, C., Djermoun, S., Ize, B., and Bleves, S. (2019). A type VI secretion system trans-kingdom effector is required for the delivery of a novel antibacterial toxin in Pseudomonas aeruginosa. Front. Microbiol. 10:1218. doi: 10.3389/fmicb.2019.01218
Blondel, C. J., Jiménez, J. C., Contreras, I., and Santiviago, C. A. (2009). Comparative genomic analysis uncovers 3 novel loci encoding type six secretion systems differentially distributed in Salmonella serotypes. BMC Genomics 10:354. doi: 10.1186/1471-2164-10-354
Blondel, C. J., Jiménez, J. C., Leiva, L. E., Alvarez, S. A., Pinto, B. I., Contreras, F., et al. (2013). The type VI secretion system encoded in Salmonella pathogenicity island 19 is required for Salmonella enterica serotype Gallinarum survival within infected macrophages. Infect. Immun. 81, 1207–1220. doi: 10.1128/iai.01165-12
Blondel, C. J., Yang, H.-J., Castro, B., Chiang, S., Toro, C. S., Zaldívar, M., et al. (2010). Contribution of the type VI secretion system encoded in SPI-19 to chicken colonization by Salmonella enterica serotypes Gallinarum and Enteritidis. PLoS One 5:e11724. doi: 10.1371/journal.pone.0011724
Bondage, D. D., Lin, J.-S., Ma, L.-S., Kuo, C.-H., and Lai, E.-M. (2016). VgrG C terminus confers the type VI effector transport specificity and is required for binding with PAAR and adaptor–effector complex. Proc. Natl. Acad. Sci. U.S.A. 113, E3931–E3940. doi: 10.1073/pnas.1600428113
Brunet, Y. R., Hénin, J., Celia, H., and Cascales, E. (2014). Type VI secretion and bacteriophage tail tubes share a common assembly pathway. EMBO Rep. 15, 315–321. doi: 10.1002/embr.201337936
Brunet, Y. R., Khodr, A., Logger, L., Aussel, L., Mignot, T., Rimsky, S., et al. (2015a). H-NS silencing of the Salmonella Pathogenicity Island 6-encoded type VI secretion system limits Salmonella enterica serovar Typhimurium interbacterial killing. Infect. Immun. 83, 2738–2750. doi: 10.1128/iai.00198-15
Brunet, Y. R., Zoued, A., Boyer, F., Douzi, B., and Cascales, E. (2015b). The type VI secretion TssEFGK-VgrG phage-like baseplate is recruited to the TssJLM membrane complex via multiple contacts and serves as assembly platform for tail tube/sheath polymerization. PLoS Genet. 11:e1005545. doi: 10.1371/journal.pgen.1005545
Cherepanov, P. P., and Wackernagel, W. (1995). Gene disruption in Escherichia coli: TcR and KmR cassettes with the option of Flp-catalyzed excision of the antibiotic-resistance determinant. Gene 158, 9–14. doi: 10.1016/0378-1119(95)00193-a
Cianfanelli, F. R., Monlezun, L., and Coulthurst, S. J. (2016). Aim, load, fire: the type VI secretion system, a bacterial nanoweapon. Trends Microbiol. 24, 51–62. doi: 10.1016/j.tim.2015.10.005
Coulthurst, S. (2019). The type VI secretion system: a versatile bacterial weapon. Microbiology 165, 503–515. doi: 10.1099/mic.0.000789
Darling, A. C. E., Mau, B., Blattner, F. R., and Perna, N. T. (2004). Mauve: multiple alignment of conserved genomic sequence with rearrangements. Genome Res. 14, 1394–1403. doi: 10.1101/gr.2289704
Datsenko, K. A., and Wanner, B. L. (2000). One-step inactivation of chromosomal genes in Escherichia coli K-12 using PCR products. Proc. Natl. Acad. Sci. U.S.A. 97, 6640–6645. doi: 10.1073/pnas.120163297
Diniz, J. A., and Coulthurst, S. J. (2015). Intraspecies competition in Serratia marcescens is mediated by type VI-secreted Rhs effectors and a conserved effector-associated accessory protein. J. Bacteriol. 197, 2350–2360. doi: 10.1128/jb.00199-15
Donato, S. L., Beck, C. M., Garza-Sánchez, F., Jensen, S. J., Ruhe, Z. C., Cunningham, D. A., et al. (2020). The β-encapsulation cage of rearrangement hotspot (Rhs) effectors is required for type VI secretion. Proc. Natl. Acad. Sci. U.S.A. 117, 33540–33548. doi: 10.1073/pnas.1919350117
Douzi, B., Spinelli, S., Blangy, S., Roussel, A., Durand, E., Brunet, Y. R., et al. (2014). Crystal structure and self-interaction of the type VI secretion tail-tube protein from enteroaggregative Escherichia coli. PLoS One 9:e86918. doi: 10.1371/journal.pone.0086918
Durand, E., Cambillau, C., Cascales, E., and Journet, L. (2014). VgrG, Tae, Tle, and beyond: the versatile arsenal of type VI secretion effectors. Trends Microbiol. 22, 498–507. doi: 10.1016/j.tim.2014.06.004
Durand, E., Derrez, E., Audoly, G., Spinelli, S., Ortiz-Lombardia, M., Raoult, D., et al. (2012). Crystal structure of the VgrG1 actin cross-linking domain of the Vibrio cholerae type VI secretion system. J. Biol. Chem. 287, 38190–38199. doi: 10.1074/jbc.m112.390153
Durand, E., Nguyen, V. S., Zoued, A., Logger, L., Péhau-Arnaudet, G., Aschtgen, M.-S., et al. (2015). Biogenesis and structure of a type VI secretion membrane core complex. Nature 523, 555–560. doi: 10.1038/nature14667
Dutta, P., Jijumon, A. S., Mazumder, M., Dileep, D., Mukhopadhyay, A. K., Gourinath, S., et al. (2019). Presence of actin binding motif in VgrG-1 toxin of Vibrio cholerae reveals the molecular mechanism of actin cross-linking. Int. J. Biol. Macromol. 133, 775–785. doi: 10.1016/j.ijbiomac.2019.04.026
Egan, F., Reen, F. J., and O’Gara, F. (2015). Tle distribution and diversity in metagenomic datasets reveal niche specialization. Environ. Microbiol. Rep. 7, 194–203. doi: 10.1111/1758-2229.12222
Feria, J. M., and Valvano, M. A. (2020). An overview of anti-eukaryotic T6SS effectors. Front. Cell. Infect. Microbiol. 10:584751. doi: 10.3389/fcimb.2020.584751
Fierer, J. (1983). Invasive Salmonella Dublin infections associated with drinking raw milk. West. J. Med. 138, 665–669.
Finn, R. D., Bateman, A., Clements, J., Coggill, P., Eberhardt, R. Y., Eddy, S. R., et al. (2014). Pfam: the protein families database. Nucleic Acids Res. 42, D222–D230. doi: 10.1093/nar/gkt1223
Fitzsimons, T. C., Lewis, J. M., Wright, A., Kleifeld, O., Schittenhelm, R. B., Powell, D., et al. (2018). Identification of novel Acinetobacter baumannii type VI secretion system antibacterial effector and immunity pairs. Infect. Immun. 86, e00297–e00318. doi: 10.1128/iai.00297-18
Flaugnatti, N., Le, T. T. H., Canaan, S., Aschtgen, M., Nguyen, V. S., Blangy, S., et al. (2016). A phospholipase A1 antibacterial type VI secretion effector interacts directly with the C-terminal domain of the VgrG spike protein for delivery. Mol. Microbiol. 99, 1099–1118. doi: 10.1111/mmi.13292
Fookes, M., Schroeder, G. N., Langridge, G. C., Blondel, C. J., Mammina, C., Connor, T. R., et al. (2011). Salmonella bongori provides insights into the evolution of the salmonellae. PLoS Pathog. 7:e1002191. doi: 10.1371/journal.ppat.1002191
GBD-2017, Stanaway, J. D., Parisi, A., Sarkar, K., Blacker, B. F., Reiner, R. C., et al. (2019). The global burden of non-typhoidal Salmonella invasive disease: a systematic analysis for the global burden of disease study 2017. Lancet Infect. Dis. 19, 1312–1324. doi: 10.1016/s1473-3099(19)30418-9
Heisler, D. B., Kudryashova, E., Grinevich, D. O., Suarez, C., Winkelman, J. D., Birukov, K. G., et al. (2015). ACD toxin–produced actin oligomers poison formin-controlled actin polymerization. Science 349, 535–539. doi: 10.1126/science.aab4090
Hernandez, R. E., Gallegos-Monterrosa, R., and Coulthurst, S. J. (2020). Type VI secretion system effector proteins: effective weapons for bacterial competitiveness. Cell. Microbiol. 22:e13241. doi: 10.1111/cmi.13241
Hoelzer, K., Switt, A. I. M., and Wiedmann, M. (2011). Animal contact as a source of human non-typhoidal salmonellosis. Vet. Res. 42:34. doi: 10.1186/1297-9716-42-34
Issenhuth-Jeanjean, S., Roggentin, P., Mikoleit, M., Guibourdenche, M., de Pinna, E., Nair, S., et al. (2014). Supplement 2008–2010 (no. 48) to the White–Kauffmann–Le Minor scheme. Res. Microbiol. 165, 526–530. doi: 10.1016/j.resmic.2014.07.004
Jamet, A., and Nassif, X. (2015). New players in the toxin field: polymorphic toxin systems in bacteria. mBio 6, e00285–e00315. doi: 10.1128/mbio.00285-15
Jana, B., Fridman, C. M., Bosis, E., and Salomon, D. (2019). A modular effector with a DNase domain and a marker for T6SS substrates. Nat. Commun. 10:3595. doi: 10.1038/s41467-019-11546-6
Jiang, F., Wang, X., Wang, B., Chen, L., Zhao, Z., Waterfield, N. R., et al. (2016). The Pseudomonas aeruginosa type VI secretion PGAP1-like effector induces host autophagy by activating endoplasmic reticulum stress. Cell Rep. 16, 1502–1509. doi: 10.1016/j.celrep.2016.07.012
Kanehisa, M., Goto, S., Kawashima, S., and Nakaya, A. (2002). The KEGG databases at GenomeNet. Nucleic Acids Res. 30, 42–46. doi: 10.1093/nar/30.1.42
Katoh, K., Rozewicki, J., and Yamada, K. D. (2017). MAFFT online service: multiple sequence alignment, interactive sequence choice and visualization. Brief. Bioinform. 20, 1160–1166. doi: 10.1093/bib/bbx108
Koskiniemi, S., Garza-Sánchez, F., Sandegren, L., Webb, J. S., Braaten, B. A., Poole, S. J., et al. (2014). Selection of orphan Rhs toxin expression in evolved Salmonella enterica serovar Typhimurium. PLoS Genet. 10:e1004255. doi: 10.1371/journal.pgen.1004255
Koskiniemi, S., Lamoureux, J. G., Nikolakakis, K. C., t’Kint de Roodenbeke, C., Kaplan, M. D., Low, D. A., et al. (2013). Rhs proteins from diverse bacteria mediate intercellular competition. Proc. Natl. Acad. Sci. U.S.A. 110, 7032–7037. doi: 10.1073/pnas.1300627110
Leiman, P. G., Basler, M., Ramagopal, U. A., Bonanno, J. B., Sauder, J. M., Pukatzki, S., et al. (2009). Type VI secretion apparatus and phage tail-associated protein complexes share a common evolutionary origin. Proc. Natl. Acad. Sci. U.S.A. 106, 4154–4159. doi: 10.1073/pnas.0813360106
Libby, S. J., Brehm, M. A., Greiner, D. L., Shultz, L. D., McClelland, M., Smith, K. D., et al. (2010). Humanized nonobese diabetic-scid IL2rγnull mice are susceptible to lethal Salmonella Typhi infection. Proc. Natl. Acad. Sci. U.S.A. 107, 15589–15594. doi: 10.1073/pnas.1005566107
Lindgren, M., Bröms, J. E., Meyer, L., Golovliov, I., and Sjöstedt, A. (2013). The Francisella tularensis LVS ΔpdpC mutant exhibits a unique phenotype during intracellular infection. BMC Microbiol. 13:20. doi: 10.1186/1471-2180-13-20
Logger, L., Aschtgen, M.-S., Guérin, M., Cascales, E., and Durand, E. (2016). Molecular dissection of the interface between the type VI secretion TssM cytoplasmic domain and the TssG baseplate component. J. Mol. Biol. 428, 4424–4437. doi: 10.1016/j.jmb.2016.08.032
Lu, S., Wang, J., Chitsaz, F., Derbyshire, M. K., Geer, R. C., Gonzales, N. R., et al. (2019). CDD/SPARCLE: the conserved domain database in 2020. Nucleic Acids Res. 48, D265–D268. doi: 10.1093/nar/gkz991
Ma, A. T., McAuley, S., Pukatzki, S., and Mekalanos, J. J. (2009). Translocation of a Vibrio cholerae type VI secretion effector requires bacterial endocytosis by host cells. Cell Host Microbe 5, 234–243. doi: 10.1016/j.chom.2009.02.005
Ma, A. T., and Mekalanos, J. J. (2010). In vivo actin cross-linking induced by Vibrio cholerae type VI secretion system is associated with intestinal inflammation. Proc. Natl. Acad. Sci. U.S.A. 107, 4365–4370. doi: 10.1073/pnas.0915156107
Ma, J., Pan, Z., Huang, J., Sun, M., Lu, C., and Yao, H. (2017a). The Hcp proteins fused with diverse extended-toxin domains represent a novel pattern of antibacterial effectors in type VI secretion systems. Virulence 8, 1189–1202. doi: 10.1080/21505594.2017.1279374
Ma, J., Sun, M., Dong, W., Pan, Z., Lu, C., and Yao, H. (2017b). PAAR-Rhs proteins harbor various C-terminal toxins to diversify the antibacterial pathways of type VI secretion systems. Environ. Microbiol. 19, 345–360. doi: 10.1111/1462-2920.13621
Ma, J., Sun, M., Pan, Z., Lu, C., and Yao, H. (2018). Diverse toxic effectors are harbored by vgrG islands for interbacterial antagonism in type VI secretion system. Biochim. Biophys. Acta Gen. Subj. 1862, 1635–1643. doi: 10.1016/j.bbagen.2018.04.010
Mariano, G., Trunk, K., Williams, D. J., Monlezun, L., Strahl, H., Pitt, S. J., et al. (2019). A family of type VI secretion system effector proteins that form ion-selective pores. Nat. Commun. 10:5484. doi: 10.1038/s41467-019-13439-0
Miyata, S. T., Kitaoka, M., Brooks, T. M., McAuley, S. B., and Pukatzki, S. (2011). Vibrio cholerae requires the type VI secretion system virulence factor VasX to kill Dictyostelium discoideum. Infect. Immun. 79, 2941–2949. doi: 10.1128/iai.01266-10
Miyata, S. T., Unterweger, D., Rudko, S. P., and Pukatzki, S. (2013). Dual expression profile of type VI secretion system immunity genes protects pandemic Vibrio cholerae. PLoS Pathog. 9:e1003752. doi: 10.1371/journal.ppat.1003752
Mulder, D. T., Cooper, C. A., and Coombes, B. K. (2012). Type VI secretion system-associated gene clusters contribute to pathogenesis of Salmonella enterica serovar Typhimurium. Infect. Immun. 80, 1996–2007. doi: 10.1128/iai.06205-11
Navarro-Garcia, F., Ruiz-Perez, F., Cataldi, Á., and Larzábal, M. (2019). Type VI secretion system in pathogenic Escherichia coli: structure, role in virulence, and acquisition. Front. Microbiol. 10:1965. doi: 10.3389/fmicb.2019.01965
Nazarov, S., Schneider, J. P., Brackmann, M., Goldie, K. N., Stahlberg, H., and Basler, M. (2018). Cryo-EM reconstruction of type VI secretion system baseplate and sheath distal end. EMBO J. 37:e97103. doi: 10.15252/embj.201797103
Nielsen, T. D., Kudahl, A. B., Østergaard, S., and Nielsen, L. R. (2013). Gross margin losses due to Salmonella Dublin infection in Danish dairy cattle herds estimated by simulation modelling. Prev. Vet. Med. 111, 51–62. doi: 10.1016/j.prevetmed.2013.03.011
Notredame, C., Higgins, D. G., and Heringa, J. (2000). T-coffee: a novel method for fast and accurate multiple sequence alignment. J. Mol. Biol. 302, 205–217. doi: 10.1006/jmbi.2000.4042
Pezoa, D., Blondel, C. J., Silva, C. A., Yang, H.-J., Andrews-Polymenis, H., Santiviago, C. A., et al. (2014). Only one of the two type VI secretion systems encoded in the Salmonella enterica serotype Dublin genome is involved in colonization of the avian and murine hosts. Vet. Res. 45:2. doi: 10.1186/1297-9716-45-2
Pezoa, D., Yang, H.-J., Blondel, C. J., Santiviago, C. A., Andrews-Polymenis, H. L., and Contreras, I. (2013). The type VI secretion system encoded in SPI-6 plays a role in gastrointestinal colonization and systemic spread of Salmonella enterica serovar Typhimurium in the chicken. PLoS One 8:e63917. doi: 10.1371/journal.pone.0063917
Pissaridou, P., Allsopp, L. P., Wettstadt, S., Howard, S. A., Mavridou, D. A. I., and Filloux, A. (2018). The Pseudomonas aeruginosa T6SS-VgrG1b spike is topped by a PAAR protein eliciting DNA damage to bacterial competitors. Proc. Natl. Acad. Sci. U.S.A. 115, 12519–12524. doi: 10.1073/pnas.1814181115
Pukatzki, S., Ma, A. T., Revel, A. T., Sturtevant, D., and Mekalanos, J. J. (2007). Type VI secretion system translocates a phage tail spike-like protein into target cells where it cross-links actin. Proc. Natl. Acad. Sci. U.S.A. 104, 15508–15513. doi: 10.1073/pnas.0706532104
Rapisarda, C., Cherrak, Y., Kooger, R., Schmidt, V., Pellarin, R., Logger, L., et al. (2019). In situ and high-resolution cryo-EM structure of a bacterial type VI secretion system membrane complex. EMBO J. 38:e100886. doi: 10.15252/embj.2018100886
Ray, A., Schwartz, N., de Santos, M. S., Zhang, J., Orth, K., and Salomon, D. (2017). Type VI secretion system MIX-effectors carry both antibacterial and anti-eukaryotic activities. EMBO Rep. 18, 1978–1990. doi: 10.15252/embr.201744226
Records, A. R. (2011). The type VI secretion system: a multipurpose delivery system with a phage-Like machinery. Mol. Plant-Microbe Interact. 24, 751–757. doi: 10.1094/mpmi-11-10-0262
Renault, M. G., Beas, J. Z., Douzi, B., Chabalier, M., Zoued, A., Brunet, Y. R., et al. (2018). The gp27-like hub of VgrG serves as adaptor to promote Hcp tube assembly. J. Mol. Biol. 430, 3143–3156. doi: 10.1016/j.jmb.2018.07.018
Robert, X., and Gouet, P. (2014). Deciphering key features in protein structures with the new ENDscript server. Nucleic Acids Res. 42, W320–W324. doi: 10.1093/nar/gku316
Russell, A. B., LeRoux, M., Hathazi, K., Agnello, D. M., Ishikawa, T., Wiggins, P. A., et al. (2013). Diverse type VI secretion phospholipases are functionally plastic antibacterial effectors. Nature 496, 508–512. doi: 10.1038/nature12074
Russell, A. B., Singh, P., Brittnacher, M., Bui, N. K., Hood, R. D., Carl, M. A., et al. (2012). A widespread bacterial type VI secretion effector superfamily identified using a heuristic approach. Cell Host Microbe 11, 538–549. doi: 10.1016/j.chom.2012.04.007
Rutherford, K., Parkhill, J., Crook, J., Horsnell, T., Rice, P., Rajandream, M.-A., et al. (2000). Artemis: sequence visualization and annotation. Bioinformatics 16, 944–945. doi: 10.1093/bioinformatics/16.10.944
Sana, T. G., Baumann, C., Merdes, A., Soscia, C., Rattei, T., Hachani, A., et al. (2015). Internalization of Pseudomonas aeruginosa strain PAO1 into epithelial cells is promoted by interaction of a T6SS effector with the microtubule network. mBio 6, e00712–e00715. doi: 10.1128/mbio.00712-15
Sana, T. G., Flaugnatti, N., Lugo, K. A., Lam, L. H., Jacobson, A., Baylot, V., et al. (2016). Salmonella Typhimurium utilizes a T6SS-mediated antibacterial weapon to establish in the host gut. Proc. Natl. Acad. Sci. U.S.A. 113, E5044–E5051. doi: 10.1073/pnas.1608858113
Santiviago, C. A., Reynolds, M. M., Porwollik, S., Choi, S.-H., Long, F., Andrews-Polymenis, H. L., et al. (2009). Analysis of pools of targeted Salmonella deletion mutants identifies novel genes affecting fitness during competitive infection in mice. PLoS Pathog. 5:e1000477. doi: 10.1371/journal.ppat.1000477
Santos, M. N. M., Cho, S.-T., Wu, C.-F., Chang, C.-J., Kuo, C.-H., and Lai, E.-M. (2020). Redundancy and specificity of type VI secretion vgrG loci in antibacterial activity of Agrobacterium tumefaciens 1D1609 strain. Front. Microbiol. 10:3004. doi: 10.3389/fmicb.2019.03004
Schroll, C., Huang, K., Ahmed, S., Kristensen, B. M., Pors, S. E., Jelsbak, L., et al. (2019). The SPI-19 encoded type-six secretion-systems (T6SS) of Salmonella enterica serovars Gallinarum and Dublin play different roles during infection. Vet. Microbiol. 230, 23–31. doi: 10.1016/j.vetmic.2019.01.006
Schwarz, S., Singh, P., Robertson, J. D., LeRoux, M., Skerrett, S. J., Goodlett, D. R., et al. (2014). VgrG-5 is a Burkholderia type VI secretion system-exported protein required for multinucleated giant cell formation and virulence. Infect. Immun. 82, 1445–1452. doi: 10.1128/iai.01368-13
Sethi, D., Mahajan, S., Singh, C., Lama, A., Hade, M. D., Gupta, P., et al. (2016). Lipoprotein LprI of Mycobacterium tuberculosis acts as a lysozyme inhibitor. J. Biol. Chem. 291, 2938–2953. doi: 10.1074/jbc.m115.662593
Shneider, M. M., Buth, S. A., Ho, B. T., Basler, M., Mekalanos, J. J., and Leiman, P. G. (2013). PAAR-repeat proteins sharpen and diversify the type VI secretion system spike. Nature 500, 350–353. doi: 10.1038/nature12453
Sibinelli-Sousa, S., Hespanhol, J. T., Nicastro, G. G., Matsuyama, B. Y., Mesnage, S., Patel, A., et al. (2020). A family of T6SS antibacterial effectors related to l,d-transpeptidases targets the peptidoglycan. Cell Rep. 31:107813. doi: 10.1016/j.celrep.2020.107813
Sigrist, C. J. A., de Castro, E., Cerutti, L., Cuche, B. A., Hulo, N., Bridge, A., et al. (2013). New and continuing developments at PROSITE. Nucleic Acids Res. 41, D344–D347. doi: 10.1093/nar/gks1067
Silva, C. A., Blondel, C. J., Quezada, C. P., Porwollik, S., Andrews-Polymenis, H. L., Toro, C. S., et al. (2012). Infection of mice by Salmonella enterica serovar Enteritidis involves additional genes that are absent in the genome of serovar Typhimurium. Infect. Immun. 80, 839–849. doi: 10.1128/iai.05497-11
Silverman, J. M., Agnello, D. M., Zheng, H., Andrews, B. T., Li, M., Catalano, C. E., et al. (2013). Haemolysin coregulated protein is an exported receptor and chaperone of type VI secretion substrates. Mol. Cell 51, 584–593. doi: 10.1016/j.molcel.2013.07.025
Small, R. G., and Sharp, J. C. M. (1979). A milk-borne outbreak due to Salmonella Dublin. J. Hyg. 82, 95–100. doi: 10.1017/s0022172400025511
Srikannathasan, V., English, G., Bui, N. K., Trunk, K., O’Rourke, P. E. F., Rao, V. A., et al. (2013). Structural basis for type VI secreted peptidoglycan dl-endopeptidase function, specificity and neutralization in Serratia marcescens. Acta Crystallogr. D Biol. Crystallogr. 69, 2468–2482. doi: 10.1107/s0907444913022725
Sullivan, M. J., Petty, N. K., and Beatson, S. A. (2011). Easyfig: a genome comparison visualizer. Bioinformatics 27, 1009–1010. doi: 10.1093/bioinformatics/btr039
Taboada, B., Estrada, K., Ciria, R., and Merino, E. (2018). Operon-mapper: a web server for precise operon identification in bacterial and archaeal genomes. Bioinformatics 34, 4118–4120. doi: 10.1093/bioinformatics/bty496
Tan, J., Yang, D., Wang, Z., Zheng, X., Zhang, Y., and Liu, Q. (2019). EvpP inhibits neutrophils recruitment via Jnk-caspy inflammasome signaling in vivo. Fish Shellfish Immunol. 92, 851–860. doi: 10.1016/j.fsi.2019.05.051
Tang, J. Y., Bullen, N. P., Ahmad, S., and Whitney, J. C. (2018). Diverse NADase effector families mediate interbacterial antagonism via the type VI secretion system. J. Biol. Chem. 293, 1504–1514. doi: 10.1074/jbc.ra117.000178
Ting, S.-Y., Bosch, D. E., Mangiameli, S. M., Radey, M. C., Huang, S., Park, Y.-J., et al. (2018). Bifunctional immunity proteins protect bacteria against FtsZ-targeting ADP-Ribosylating toxins. Cell 175, 1380.e14–1392.e14. doi: 10.1016/j.cell.2018.09.037
Uzzau, S., Brown, D. J., Wallis, T., Rubino, S., Leori, G., Bernard, S., et al. (2000). Host adapted serotypes of Salmonella enterica. Epidemiol. Infect. 125, 229–255. doi: 10.1017/s0950268899004379
Wang, M., Luo, Z., Du, H., Xu, S., Ni, B., Zhang, H., et al. (2011). Molecular characterization of a functional type VI secretion system in Salmonella enterica serovar Typhi. Curr. Microbiol. 63, 22–31. doi: 10.1007/s00284-011-9935-z
Wang, J., Yang, B., Leier, A., Marquez-Lago, T. T., Hayashida, M., Rocker, A., et al. (2018). Bastion6: a bioinformatics approach for accurate prediction of type VI secreted effectors. Bioinformatics 34, 2546–2555. doi: 10.1093/bioinformatics/bty155
Wang, S., Yang, D., Wu, X., Yi, Z., Wang, Y., Xin, S., et al. (2019). The ferric uptake regulator represses type VI secretion system function by binding directly to the clpV promoter in Salmonella enterica serovar Typhimurium. Infect. Immun. 87, e00562–19. doi: 10.1128/iai.00562-19
Whitney, J. C., Beck, C. M., Goo, Y. A., Russell, A. B., Harding, B. N., Leon, J. A. D., et al. (2014). Genetically distinct pathways guide effector export through the type VI secretion system. Mol. Microbiol. 92, 529–542. doi: 10.1111/mmi.12571
Whitney, J. C., Chou, S., Russell, A. B., Biboy, J., Gardiner, T. E., Ferrin, M. A., et al. (2013). Identification, structure, and function of a novel type VI secretion peptidoglycan glycoside hydrolase effector-immunity pair. J. Biol. Chem. 288, 26616–26624. doi: 10.1074/jbc.m113.488320
Whitney, J. C., Quentin, D., Sawai, S., LeRoux, M., Harding, B. N., Ledvina, H. E., et al. (2015). An interbacterial NAD(P)+ glycohydrolase toxin requires elongation factor Tu for delivery to target cells. Cell 163, 607–619. doi: 10.1016/j.cell.2015.09.027
Wood, T. E., Aksoy, E., and Hachani, A. (2020). From welfare to warfare: the arbitration of host-microbiota interplay by the type VI secretion system. Front. Cell. Infect. Microbiol. 10:587948. doi: 10.3389/fcimb.2020.587948
Wood, T. E., Howard, S. A., Förster, A., Nolan, L. M., Manoli, E., Bullen, N. P., et al. (2019). The Pseudomonas aeruginosa T6SS delivers a periplasmic toxin that disrupts bacterial cell morphology. Cell Rep. 29, 187.e7–201.e7. doi: 10.1016/j.celrep.2019.08.094
Xian, H., Yuan, Y., Yin, C., Wang, Z., Ji, R., Chu, C., et al. (2020). The SPI-19 encoded T6SS is required for Salmonella Pullorum survival within avian macrophages and initial colonization in chicken dependent on inhibition of host immune response. Vet. Microbiol. 250:108867. doi: 10.1016/j.vetmic.2020.108867
Yin, M., Yan, Z., and Li, X. (2019). Architecture of type VI secretion system membrane core complex. Cell Res. 29, 251–253. doi: 10.1038/s41422-018-0130-7
Zhang, D., de Souza, R. F., Anantharaman, V., Iyer, L. M., and Aravind, L. (2012). Polymorphic toxin systems: comprehensive characterization of trafficking modes, processing, mechanisms of action, immunity and ecology using comparative genomics. Biol. Direct 7:18. doi: 10.1186/1745-6150-7-18
Zheng, J., Ho, B., and Mekalanos, J. J. (2011). Genetic analysis of anti-amoebae and anti-bacterial activities of the type VI secretion system in Vibrio cholerae. PLoS One 6:e23876. doi: 10.1371/journal.pone.0023876
Zimmermann, L., Stephens, A., Nam, S.-Z., Rau, D., Kübler, J., Lozajic, M., et al. (2017). A completely reimplemented MPI bioinformatics toolkit with a new HHpred server at its core. J. Mol. Biol. 430, 2237–2243. doi: 10.1016/j.jmb.2017.12.007
Keywords: Salmonella Dublin, interbacterial competition, T6SS, effector, immunity protein
Citation: Amaya FA, Blondel CJ, Barros-Infante MF, Rivera D, Moreno-Switt AI, Santiviago CA and Pezoa D (2022) Identification of Type VI Secretion Systems Effector Proteins That Contribute to Interbacterial Competition in Salmonella Dublin. Front. Microbiol. 13:811932. doi: 10.3389/fmicb.2022.811932
Edited by:
Eric Cascales, Aix-Marseille Université, FranceReviewed by:
Qiyao Wang, East China University of Science and Technology, ChinaCasey Fowler, University of Alberta, Canada
Copyright © 2022 Amaya, Blondel, Barros-Infante, Rivera, Moreno-Switt, Santiviago and Pezoa. This is an open-access article distributed under the terms of the Creative Commons Attribution License (CC BY). The use, distribution or reproduction in other forums is permitted, provided the original author(s) and the copyright owner(s) are credited and that the original publication in this journal is cited, in accordance with accepted academic practice. No use, distribution or reproduction is permitted which does not comply with these terms.
*Correspondence: Carlos A. Santiviago, Y3NhbnRpdmlhZ29AY2lxLnVjaGlsZS5jbA== David Pezoa, ZGF2aWQucGV6b2FAdW1heW9yLmNs
†These authors have contributed equally to this work and share first authorship