- 1Aix-Marseille-University, IRD, MEPHI, Marseille, France
- 2Assistance Publique-Hôpitaux de Marseille, Marseille, France
- 3IHU Méditerranée Infection, Marseille, France
- 4Laboratoire National de Référence des IST/VIH et de la Tuberculose, Niamey, Niger
- 5Institut des Sciences Vétérinaires, Université de Blida 1, Blida, Algeria
Mycobacteria that form the Mycobacterium tuberculosis complex are responsible for deadly tuberculosis in animals and patients. Identification of these pathogens at the species level is of primary importance for treatment and source tracing and currently relies on DNA analysis, including whole genome sequencing (WGS), which requires a whole day. In this study, we report the unprecedented discrimination of M. tuberculosis complex species using matrix-assisted laser desorption ionization-time of flight mass spectrometry (MALDI-TOF-MS), with WGS as the comparative reference standard. In the first step, optimized peptide extraction applied to 36 isolates otherwise identified in five of the 11 M. tuberculosis complex variants by WGS yielded 139 MALDI-TOF spectra, which were used to identify biomarkers of interest that facilitate differentiation between variants. In a second step, 70/80 (88%) other isolates were correctly classified by an algorithm based on specific peaks. This study is the first to report a MALDI-TOF-MS method for discriminating M. tuberculosis complex mycobacteria that is easily implemented in clinical microbiology laboratories.
Introduction
Mycobacteria that form the Mycobacterium tuberculosis complex (MBTC) are responsible for tuberculosis, a deadly infection that is a major public health concern worldwide (Forbes et al., 2018). MBTC is composed of different species that have been recently reclassified as variants of M. tuberculosis: M. tuberculosis var. tuberculosis, M. tuberculosis var. africanum, M. tuberculosis var. bovis, M. tuberculosis var. BCG, M. tuberculosis var. caprae, M. tuberculosis var. microti, M. tuberculosis var. pinnipedii, M. tuberculosis var. canettii, M. tuberculosis var. suricattae, M. tuberculosis var. orygis, and M. tuberculosis var. mungi (Malone and Gordon, 2017; Riojas et al., 2018). In our clinical microbiology practice, however, M. tuberculosis sensu stricto, along with Mycobacterium bovis and its derivative Bacillus Calmette-Guérin (BCG) and Mycobacterium africanum, are the sole MBTC variants that we have encountered for 10 years. For a convenient terminology, we will use the term “species” to distinguish the different variants of the MTBC.
The distinction between M. tuberculosis variants is a concern for the immediate medical management of patients, as, for example, M. bovis and BCG are two species acknowledged to be pyrazinamide-resistant (Wanzala et al., 2019). This distinction is also of concern in terms of tracing the source to prevent additional cases, such as zoonotic M. bovis tuberculosis (Saad et al., 2020).
While real-time PCR detection of MBTC performed directly in clinical samples is now widely used for the routine screening of tuberculosis, this technique does not enable species identification within the complex (Iketleng et al., 2018). In routine clinical microbiology, this identification is achieved by DNA sequencing investigations using commercially available line probe assays (Richter et al., 2003; Pinsky and Banaei, 2008; Shea et al., 2017; Loiseau et al., 2019) and, at best, through whole genome sequencing (WGS) of the colony (Iketleng et al., 2018). These techniques, however, require specific equipment that may not be available at the point-of-care, with results taking a few days to become available.
Matrix-assisted laser desorption ionization-time of flight mass spectrometry (MALDI-TOF-MS) is a widely implemented technique for the routine identification of bacteria in clinical microbiology laboratories. It is a rapid and low-cost method for identifying bacterial species (Seng et al., 2013; Lagier et al., 2017) and is also routinely used for the identification of mycobacteria (Patel, 2015; Alcaide et al., 2018). However, MALDI-TOF-MS suffers from the same limitation as real-time PCR, i.e., the impossibility of differentiating MBTC species based on similar peptide profile matching. Thus, the specific identification of tuberculosis species using this method is currently impossible (O’Connor et al., 2020).
In this study, we investigated the peptidic profile of MBTC isolates to identify relevant biomarkers specific to one or more species that may provide a fast and cost-efficient method of screening. This method is particularly useful for differentiating groups of isolates with similar spectra and is indistinguishable from a non-selective pattern matching method such as biotyping. It relies on the identification of relevant specific peaks in a MALDI-TOF spectral dataset from isolates already identified using another method at the species, subspecies, or variant level to focus on the pattern matching of spectra on these peaks. Previous studies used this approach to distinguish methicillin-resistant Staphylococcus aureus clones from non-resistant clones (Camoez et al., 2016; Wang et al., 2018) or different Streptococcus pneumoniae serotypes (Nakano et al., 2015) directly in MALDI-TOF spectra. Application of this method to distinguish subspecies of mycobacteria has shown its efficiency on the Mycobacterium abscessus complex (Fangous et al., 2014) and on the MBTC in the specific situation of M. bovis from veterinary samples (Bacanelli et al., 2019). Hence, the present study is the first to investigate the differentiation of clinically relevant species in MBTC using MALDI-TOF MS and associated software.
Materials and Methods
Mycobacterial Isolates
One hundred twenty-eight clinical isolates preserved in the Collection de Souches de l’Unité des Rickettsies (CSUR) and cultured in the BSL3 laboratory of the IHU Méditerranée Infection, Marseille, France were included in this study. This collection of isolates has previously been investigated (Bouzid et al., 2018; Tazerart et al., 2021a,b) in part for accurate identification by WGS, and was used here as the reference standard (Saad et al., submitted for publication). In addition, four isolates of reference strains ordered from other collections were added to this study: M. bovis BCG Pasteur 1173P2, M. bovis BCG Tokyo 172, Mycobacterium canettii CIPT 140010059, and Mycobacterium gilvum DSM 45363 (Table 1).
In the first phase, 36 isolates identified by WGS, such as M. tuberculosis, M. africanum, M. bovis, M. bovis BCG, and M. canettii, were defined as reference strains for the identification of potential relevant biomarkers. Sublineages of M. tuberculosis isolates were also investigated, as they present relevant information for diagnosis, notably on antibiotic resistance. These isolates were selected as representative tuberculous mycobacterial species encountered in our clinical experience. Indeed, from 2017 to 2020, WGS lineage identification of 218 MBTC strains yielded 8 (3.7%) as M. bovis, 4 (1.8%) as M. africanum, and 4 (1.8%) as M. bovis BCG. Similarly, in the WHO report on tuberculosis in 2020, M. bovis infections were estimated to represent 1.47% of MTBC clinical cases worldwide. M. canettii strains were added to the work, although no clinical isolate has been encountered in our unit to challenge the method in identifying unusual MTBC species. In addition, 12 non-tuberculous mycobacteria identified using WGS (M. abscessus, Mycobacterium avium, Mycobacterium chelonae, M. gilvum, Mycobacterium persicum, and Mycobacterium xenopi) served as negative controls for the MALDI-TOF Biotyper identification method (Bruker Daltonics, Bremen, Germany). These isolates were selected as representative non-tuberculous mycobacterial species encountered in our clinical experience.
In the second phase, 80 isolates were defined as our test group to evaluate the reliability of discriminating biomarkers identified in the first phase.
All isolates were cultured on TransBK m4 agar medium (Culture-Top, Marseille, France), a culture medium derived from Middlebrook 7H10 agar medium, at 37°C in a 5% CO2 atmosphere for a minimum of 7 days. In addition, 17 isolates selected from the former collection were cultured on Middlebrook 7H10 agar medium (Becton Dickinson Medical, Le Pont-de-Claix, France) and on Coletsos medium (BioRad, Marnes-la-Coquette, France) at 37°C in a 5% CO2 atmosphere for a minimum of 7 days to validate the applicability of the method regardless of the growth medium used (Table 2).
Total DNA extraction and WGS were performed as previously described (Saad et al., submitted for publication; Saad et al., 2019).
Matrix-Assisted Laser Desorption Ionization-Time of Flight Mass Spectrometry Analysis
Between one and three 1-μl plastic loops harvested from each isolate were mixed with 300 μl of high purity liquid chromatography (HPLC)-grade water in a 1.5-ml tube (Sarstedt, Nümbrecht, Germany). Samples were then heated to 100°C for 30 min to inactivate mycobacteria (Zwadyk et al., 1994). After cooling, 900 μl of ethanol were added, vortexed for 1 min and centrifuged for 2 min at 16,060 × g, and the supernatant was removed. The centrifugation step was repeated twice, and the pellet was dried at room temperature for 1 min. A spatula full of glass beads for cell disruption (diameter: 0.5 mm; Scientific Industries Inc., Bohemia, United States) and 20 μl of acetonitrile were added, and then the samples were stirred using a vortex device for 1 min. Twenty microliters of a solution composed of 70% formic acid were then added and agitated for 5 s. Samples were subsequently agitated using a FastPrep-24™ device (MP Biomedicals, Illkirch-Graffenstaden, Germany, #6004500) for five cycles of 20 s at 4.0 m/s, with a resting time of 5 s between each cycle and then centrifuged for 2 min at 16,060 × g. Four replicates of 1.5 μl of supernatant were deposited on a MALDI target plate (MSP 96 target polished steel, Bruker Daltonics) and dried at room temperature for 1 min. A matrix solution consisting of 1.5 μl of saturated α-cyano 4-hydroxycinnamic acid (CHCA) (Sigma–Aldrich, Taufkirchen, Germany) in trifluoroacetic acid 2.5% /acetonitrile (1:1) was deposited on each spot and dried for 5 min at room temperature. Two spots consisting of 1.5 μl of CHCA matrix alone were incorporated as negative controls, and two spots consisting of 1.5 μl of a 1/10 diluted solution of Bruker bacterial standard test, an Escherichia coli extract spiked with two high-molecular-weight proteins (Bruker Daltonics) mixed with 1.5 μl of CHCA matrix, were deposited on every plate as positive controls. All the solvents used were of MS grade. Mass spectra were obtained using a MicroFlex™ (Bruker Daltonics) MALDI-TOF MS. Spectra were recorded in the mass range from 2,000 to 20,000 Da.
Spectra Processing and Data Analysis
Spectra were identified using Biotyper Compass Explorer v4.1 (Bruker Daltonics). Each spectrum was compared with the Mycobacteria bead method Library v.3.0 (Bruker Daltonics), MALDI Biotyper Compass IVD Library v9.0, and our in-house database consisting of 52 additional references of mycobacteria from MTBC generated by controlling spectral quality, MALDI-TOF identification, and WGS identification of spectra and isolates used for references as recommended by the manufacturer and several publications (Keys et al., 2004; Saleeb et al., 2011; Rodriguez-Temporal et al., 2017). Spectra were then processed with ClinProTools v2.2 build 78 (Bruker Daltonics): spectra preparation included a baseline subtraction by Top-hat with a 10% minimal baseline width, an exclusion of noisy spectra for which the signal-to-noise ratio (S/N) was inferior at 2, and a smoothing using the Savitsky-Golay method with a width of 2.0 m/z for five cycles; finally, all spectra were recalibrated between each other, with an exclusion threshold of 1,000 ppm. Peak picking was then performed on each spectrum with an S/N threshold > 5 and a maximal number of 500 peaks detected. Peaks with similar m/z values were aggregated if they presented a minimal occurrence in a single spectra of 25% with a 500 ppm aggregation width. The intensities of peaks were finally calculated by measuring the areas under peaks with a zero-level integration.
In the first phase, spectra were grouped into different classes according to the identification of their corresponding isolate by WGS. Statistical tests such as t-test/ANOVA (TTA) and Wilcoxon/Kruskal–Wallis test (WKW) were then used and applied to processed spectra to identify potential class-specific peaks, and the Anderson–Darling test (AD) was used to verify the normal distribution of peak intensities in each class. The quick-classifier (QC) algorithm was calculated based on most discriminating peaks presenting the lowest p-value calculated with TTA (PTTA) if possible, i.e., if the intensities of discriminating peaks present a p value from the AD test (PAD) > 0.05, confirming their normal distribution, to classify spectra from test group isolates. Otherwise, QC was calculated from peaks presenting the lowest p values from the WKW test (PWKW).
Results
Speciation of Mycobacteria Based on Database Matching
Matrix-assisted laser desorption ionization-time of flight mass spectrometry spectra presenting a low intensity resulting in a noisy signal were excluded from the study, providing a total of 499 MALDI-TOF-MS spectra for the Mycobacterium species investigated (Table 1), with a mean of 3.90 spectra per isolate. Identification of MTBC isolate spectra by comparison with the selected databases yielded 95.3% correct identifications of Mycobacterium species with a comparison score greater than 2.00, and this value increased to 99.7% using a comparison score greater than 1.80. All spectra from isolates of the reference and test groups presented a first matching hit with the MBTC database. Identifications of negative control isolates are presented in Table 3. While M. abscessus, M. avium, M. gilvum, and M. xenopi presented reliable identification against the Bruker Mycobacteria bead method library, M. chelonae and M. persicum presented incorrect identifications. M. chelonae was incorrectly identified as Mycobacterium salmoniphilum, an M. chelonae-related species (Whipps et al., 2007), and M. persicum presented over 10 spectra with no reliable identification, while six spectra presented the best matching hit for Mycobacterium kansasii (Score: 1.97). This result might be explained by the absence of an M. persicum reference in the mycobacteria bead method library and the genetic similarity of M. persicum and M. kansasii (Jagielski et al., 2019).
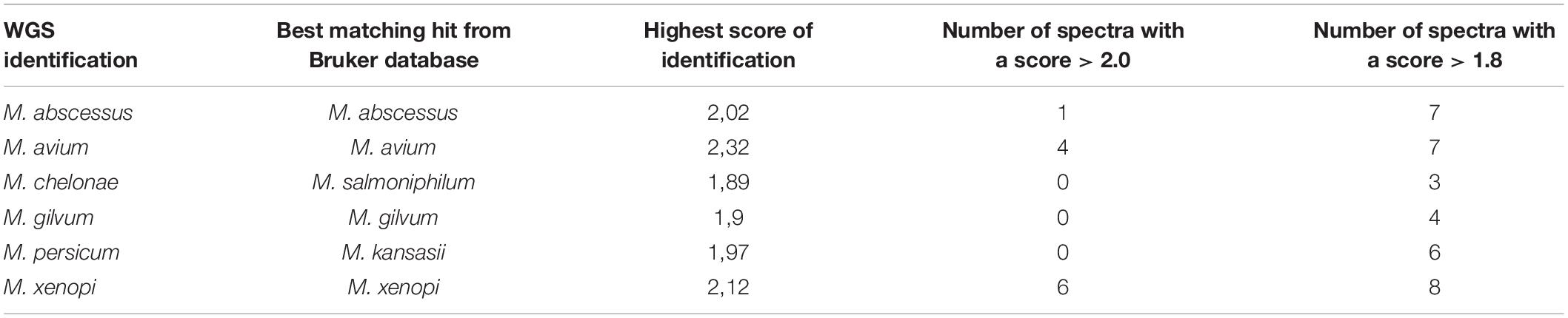
Table 3. Negative control isolate identifications compared with the Bruker Mycobacteria beads method MALDI-TOF library.
Identification of Specific Biomarkers and Classification Method Calculation
Spectra from the reference group were first separated into eight classes corresponding to their WGS identification (M. africanum, M. bovis, M. bovis BCG, M. canettii, M. tuberculosis Lineage 1, M. tuberculosis Lineage 2, M. tuberculosis Lineage 3, and M. tuberculosis Lineage 4). Statistical tests on average spectra from each class identified 84 peaks presenting a PTTA and PWKW value < 0.05. Among these 84 peaks, 11 presented a PAD > 0.05. The QC (QC1) was then calculated for the 23 peaks presenting the lowest PWKW values (Supplementary Table 1). QC1 presented a cross-validation rate of only 48% and a predictive recognition capability of 62%, suggesting that it was not sufficiently reliable to correctly classify spectra among different classes. Evaluation of the classification reliability of QC1 was achieved on spectra from isolates of the test group.
QC1 presented a very low rate of correct classification of 17% for these spectra, confirming that the discriminating power of the classifier was insufficient. Somehow, by considering that the classification of one lineage of M. tuberculosis as another was correct, QC1 presented a correct classification rate of 74% (Table 4). Thus, QC1 was unable to discriminate correctly between different lineages of M. tuberculosis. Moreover, while correct classification rate of spectra from isolates of M. tuberculosis Lineages 2, 3, and 4 improved substantially by implementing this criterion (from 23 to 81, from 15 to 85, and from 16 to 88%, respectively), the correct classification rate of M. tuberculosis Lineage 1 only improved from 20 to 47%, which was still not sufficient to be considered a correct identification rate. Finally, correct classification rates for classes other than M. tuberculosis isolates were very low (M. africanum: 0%; M. bovis: 25%; M. bovis BCG: 13%; M. canettii: 9%). Peaks selected by QC1 were therefore investigated to explain these rates.
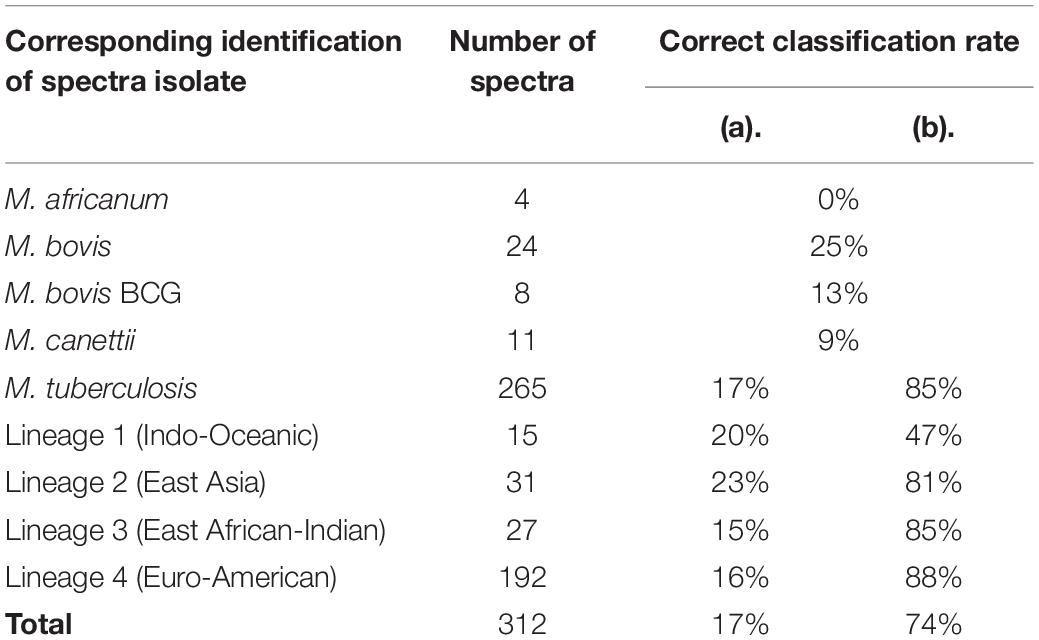
Table 4. Correct classification rate of spectra from test group isolates according to QC1 by considering (a) strictly correct classification or (b) different lineages of M. tuberculosis as one class.
One peak measured at m/z: 3,514 Da presented low PTTA and PW/KW values (both < 1E–6), and the second maximum difference of peak intensity average between classes (DAve) was of particular interest, as it presented an absence/presence discriminatory pattern among classes instead of a difference in the peak intensity ratio (Figure 1). More precisely, the m/z: 3,514 Da peak was present in M. tuberculosis Lineages 2, 3, and 4 (average peak intensities of 20.1, 21.2, and 17.3 a.u., respectively) and absent from M. africanum, M. bovis and M. bovis BCG, M. canettii, and M. tuberculosis Lineage 1 (all average peak intensities < 1.5). This finding might explain the low rate of classification of M. tuberculosis Lineage 1 spectra. Hence, an approach focusing on this biomarker was investigated.
Discriminatory Power of the Identified Biomarker at m/z: 3,514 Da
Spectra from the reference group were divided into two classes according to observations of m/z: 3,514 Da peak intensities: the first class contained spectra from M. africanum, M. bovis and M. bovis BCG, M. canettii, and M. tuberculosis Lineage 1 isolates (n = 76), and the second class contained spectra from M. tuberculosis Lineages 2, 3, and 4 (n = 63). Statistical tests on average spectra from each class identified 37 peaks presenting a PWKW value less than 0.05. Five of these peaks, including the 3,515 Da peak, were selected by the QC algorithm calculated (QC2) as being more relevant for the classification of spectra (Supplementary Table 2 and Figure 2). QC2 presented a cross-validation rate of 99% and a recognition capability of 100%. The QC2 evaluation of the test group yielded an overall correct classification rate of 89%, with correct spectra classification rates of 100% for Class 1 and 86% for Class 2. The relevance of QC2 in terms of the classification of isolates was evaluated, and a classification was considered as determined for each isolate if half or more spectra acquired from this isolate shared the same classification. Based on these criteria, QC2 presented an overall correct isolate classification rate of 87.5% (70/80), divided into 100% correct classification for Class 1 (16/16) and 84% (54/64) for Class 2, with four isolates belonging to this class according to the incorrectly classified WGS identification (5%) and six isolates with an undetermined classification (7.5%) (Table 5). Thus, metrics such as accuracy (Acc), sensitivity (Se), specificity (Sp), and predictive positive value (Vpp) of QC2 were calculated by considering mycobacteria isolates belonging to Class 2 (M. tuberculosis Lineages 2, 3, or 4) that were correctly classified as true positives (TPs) and mycobacteria isolates belonging to Class 1 (M. africanum/M. bovis/M. bovis BCG/M. canetti/M. tuberculosis Lineage 1) that were correctly classified as true negatives (TNs), as mycobacteria isolates that were incorrectly classified as Class 1 as false positives (FPs) and mycobacteria isolates that were incorrectly classified as Class 2 as false negatives (FNs). Acc and Se were reported as ranges by counting undetermined classifications in either TPs or FNs to consider undetermined classification results for Class 1 isolates. Therefore, metrics of QC2 on the confirmation of the belonging of an MBTC isolate as being part of M. tuberculosis Lineages 2, 3, or 4 were as follows: Acc: 87.5–95%; Se: 84–94%; Sp: 100%; and Vpp: 100%.
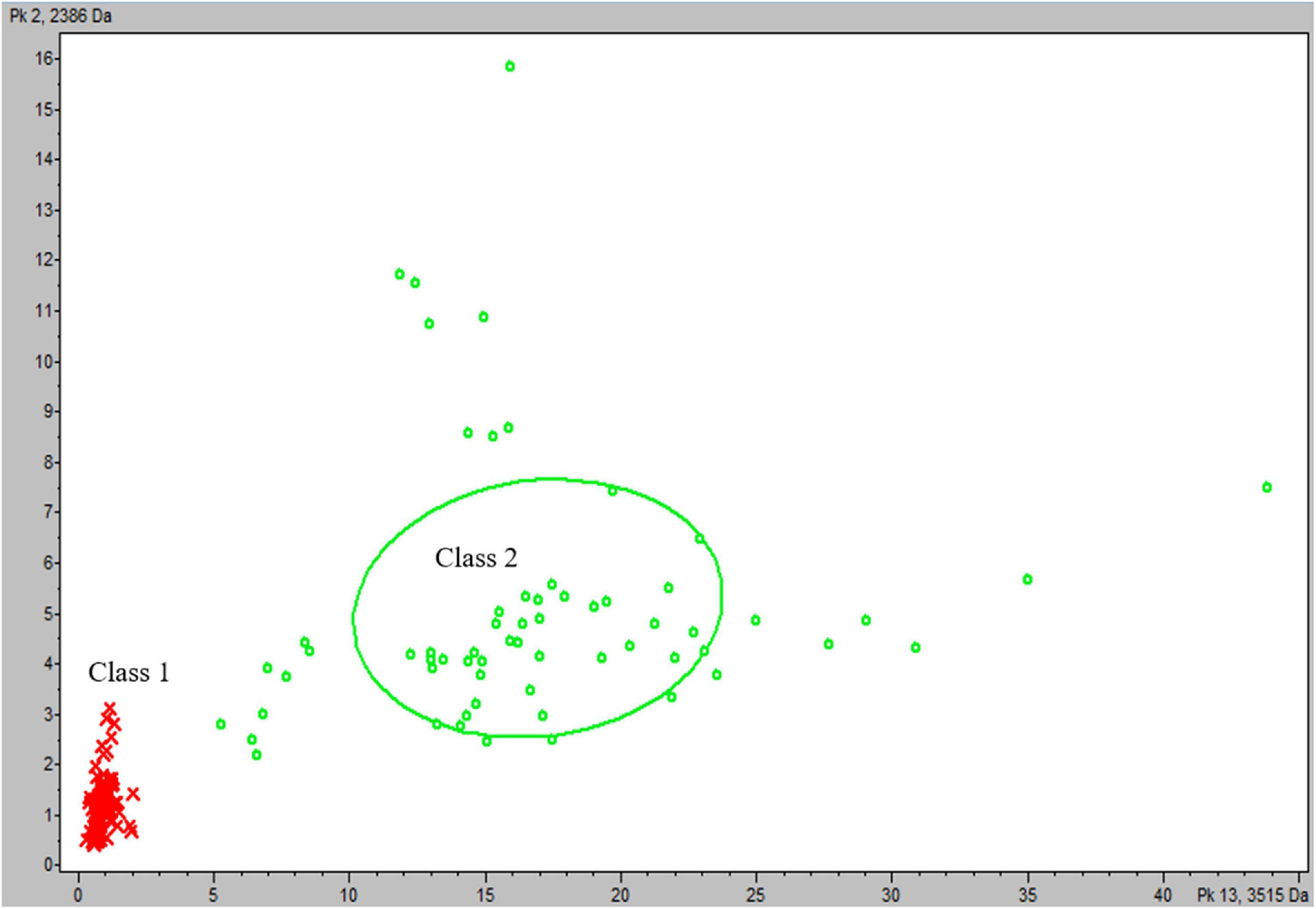
Figure 2. Scatter plot of reference group spectra from classes according to the most discriminating peaks at m/z: 3,515 and 2,386 Da. Class 1 (M. africanum, M. bovis, M. bovis BCG, M. canettii, and M. tuberculosis Lineage 1): red cross and Class 2 (M. tuberculosis Lineages 2, 3, and 4): green circle.
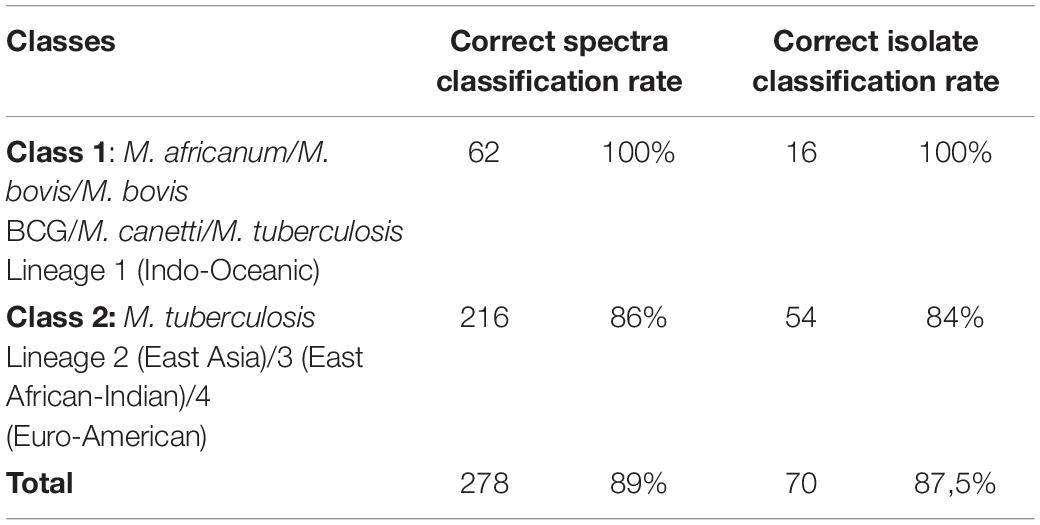
Table 5. Correct classification rates for spectra and isolates from test group isolates according to QC2.
Evaluation of the Effect of Growth Media
Among 17 isolates cultured in parallel on Middlebrook 7H10 (M7H10) and Coletsos media, 17 and 15 presented satisfying growth after 7 days on M7H10 and Coletsos media, respectively. Indeed, one isolate identified by WGS as M. tuberculosis Lineage 2 (East Asia) and one isolate identified by WGS as M. tuberculosis Lineage 4 (Euro-American) presented insufficient culture on Coletsos medium. One hundred twenty-eight spectra were generated for these isolates using the same experimental procedure as mentioned above. Then, nine spectra were excluded by ClinProTools, as they presented a low signal (S/N < 2). The remaining 119 spectra were classified using the QC2 algorithm. QC2 presented a correct classification accuracy between Class 1 (M. africanum/M. bovis/M. bovis BCG/M. tuberculosis Lineage 1) and Class 2 (M. tuberculosis Lineages 2/3/4) of 94.0% (63/67) for spectra from isolates grown on M7H10 medium and 88.5% (46/52) for spectra from isolates grown on Coletsos medium. In terms of isolates, QC2 presented a correct isolate classification rate of 94.1% (16/17) and one isolate with an undetermined classification after culture on M7H10 growth medium, and 86.7% (13/15) and two isolates with an undetermined classification after culture on Coletsos growth medium (Table 6).
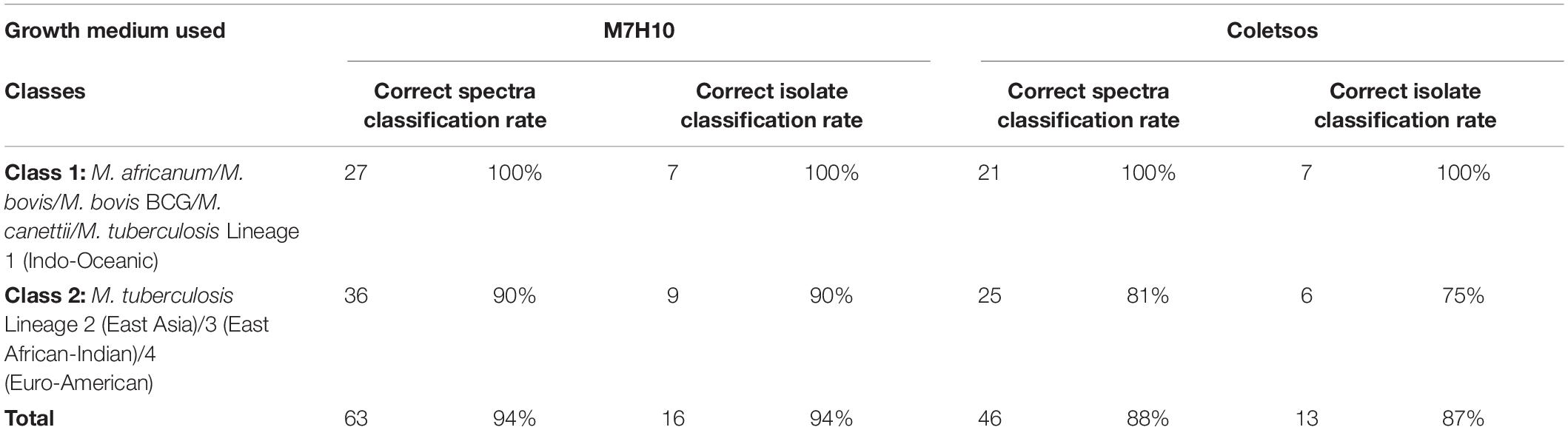
Table 6. Correct classification rates for spectra and isolates from isolates grown on other culture media according to QC2.
As expected from these results, direct observation of the 3,514-Da biomarker using FlexAnalysis software (Bruker Daltonics) resulted in the observation of the presence of a peak at 3,514 Da for spectra from isolates belonging to Class 2 and an absence of this peak for spectra from isolates belonging to Class 1 (Figure 3).

Figure 3. Comparison of MALDI-TOF spectra plots from isolates of Class 1 and 2 grown on different culture media. Spectra of one isolate belonging to Class 1 (M. tuberculosis Lineage 2, red) and one isolate belonging to Class 2 (M. bovis BCG, blue) grown on M4 agar (A), M7H10 (B), and Coletsos (C) media. The x-axis shows the m/z values on a reduced window of 350 Da comprising the specific biomarker of interest at 3,514 Da. The y-axis indicates the intensity of peaks in arbitrary units.
Discussion
In this study, we report the unprecedented discrimination of MBTC species using the MALDI-TOF-MS approach. We showed that MALDI-TOF-MS spectra of mycobacteria can be used as a first-line method for screening isolates in the MBTC, presenting a reasonable sensitivity of 93% (84–94%) and a specificity of 100% for confirming the identification of M. tuberculosis belonging to Lineages 2 (East Asia), 3 (East African-Indian), or 4 (Euro-American). However, the specificity metric was less reliable than the sensitivity, as only 16 of the 80 isolates from the test group were considered negative for this test. Confidence in this metric might be increased by increasing the number of additional members (M. africanum, M. bovis, M. bovis BCG, and M. tuberculosis Lineage 1) of the MTBC in the test group. However, these species are still not easily affordable in large quantities. The American Type Culture Collection (ATCC), for example, currently proposes in its collection a total of 69 M. tuberculosis isolates, including only 34 M. bovis, 2 M. africanum, and no M. canettii.
These results have been consolidated using several controls that include positive (E. coli with a score greater than 2.00) and negative controls (no identification with a score less than 1.60) deposited on each MALDI-TOF-MS target plate; the acquisition of MALDI-TOF-MS spectra at least four times; and the identification of isolates using WGS as the gold standard. Finally, spectra from the reference group were deposited in a publicly available database maintained at our Institute1,2.
The method presented here would be improved by increasing the number of spectra for the reference group to obtain a more accurate classifier algorithm, especially for members of the MBTC rarely encountered in clinical human diagnosis, such as M. africanum and M. canettii, which represented four and six isolates of the entire cohort of 116 isolates between the reference and test groups, respectively. Additional data may achieve classification between variants of the Class 1 group (M. africanum, M. bovis, M. bovis BCG, M. canettii, and M. tuberculosis Lineage 1). Distinction between Lineages 2, 3, and 4 of M. tuberculosis has not been achieved using this method, confirming results reported in a previous study by O’Connor et al. (2020) suggesting that this discrimination was currently impossible with a MALDI-TOF-MS data-based approach. Somehow, the data presented here highlighted a relevant difference between M. tuberculosis Lineage 1 (Indo-Oceanic) and other lineages, as spectra from isolates of this lineage did not present the biomarker investigated. Interestingly, Brosch et al. documented that M. tuberculosis Lineages 2, 3, and 4, which are referred as “modern” strains of M. tuberculosis, differed from M. tuberculosis Lineage 1, which are referred as “ancestral” strains based on the deletion in their genome of the TbD1 region. Loss of TbD1 region is suspected to be responsible for the predominance of these lineages in current tuberculosis epidemiology (Brosch et al., 2002; Bottai et al., 2020). Furthermore, the observation reported here suggested that the 3,514-Da peak was absent in all TbD1-positive MBTC species. The TbD1 locus encompasses the m-pS6/mmpL6 operon, two proteins involved in the trans-inner-membrane transport of lipid residues. These proteins are absent and truncated in M. tuberculosis Lineages 2, 3, and 4, respectively. The 3,514 Da biomarker identified in MALDI-TOF spectra specifically for these lineages might then be hypothetically related to this proteomic difference. Mascot database searches (Matrix Science Inc.) were performed using the following parameters: UniProt database restricted to MBTC taxonomy, with formic acid as the digestion reagent selected and a mass shift tolerance of 2.0 Da on the m/z of 3,514 Da observed for the biomarker. However, this approach did not result in significant hits. Further studies will focus on the identification on the biomarker observed by 2-D electrophoresis gel separation and LC-MS/MS identification (Christner et al., 2014) to confirm the implication of the m-pS6/mmpL6 operon in the spectral differentiation of MBTC species.
This study does not present results obtained for Mycobacterium caprae, Mycobacterium microti, Mycobacterium orygis, and Mycobacterium pinnipedii, which are rare in human clinical diagnosis, and their epidemiology is considered location-dependent. In fact, a few dozen cases of human M. microti infections have been reported over the last few decades, and cases of human M. caprae infections were evaluated to represent less than 0.3% of human tuberculosis cases in Europe (Panteix et al., 2010; Prodinger et al., 2014). Although these numbers are considered underestimated because M. microti presents slow growth and is often undiagnosed and M. caprae is uneasily distinguished from M. bovis, the occurrence of these species in humans remains scarce compared to the occurrences of MBTC species presented here (M. tuberculosis, M. bovis, M. bovis BCG, and M. africanum), which were estimated at 9.5 million cases in the world in 2020 [World Health Organization [WHO], 2020 TB report]. Ecotypes that have never been reported in humans, such as Mycobacterium mungi and Mycobacterium suricattae, were also not included in this study. Therefore, the method may not be reliable for these species. However, as these variants are genetically closer to M. bovis and M. africanum than M. tuberculosis Lineages 2, 3, or 4 (Brites et al., 2018), the 3,514-Da biomarker might hypothetically be absent from their MALDI-TOF spectra.
The method proposed here relied on the observation of spectra containing or lacking specific biomarkers. This fast and low-cost method might be easily used by other laboratories already equipped with a MALDI-TOF-MS instrument to verify the identification of their isolates for clinical diagnostic purposes and in a retrospective manner for data already acquired on MBTC isolates. As discrimination described here was mostly driven by the absence/presence of the 3,514 Da peak, the direct observation of acquired spectra with software such as FlexAnalysis, which is provided with the MicroFlex device, limits the analytical skills needed for routine application, and the information regarding the absence/presence of biomarker can be obtained within minutes after spectra acquisition. The addition of the assessment of biomarker presence in the workflow of mycobacteria identification is an efficient and cost-reducing screening method, confirming the identification of an inquired isolate as M. tuberculosis or belonging to a less frequent member of the complex, suggesting the value of using an expensive sequencing method for identification purposes. Indeed, MALDI-TOF sample preparation and analysis, even with the addition of a data analysis step, remain cost- and time-limited. The mean consolidated cost of this method for a full target plate of 96 spots after considering all devices used (MALDI-TOF device, MALDI-TOF target plate, heat-block, stirrer, centrifuge, Fastprep device, and BSC), consumables, reagents, and staff occupation time was estimated at $3.93 for the identification of one isolate. Meanwhile, other mycobacteria identification methods costs are estimated at $1–2.3 for the anti-MPB64 assay (Toihir et al., 2011; Kanade et al., 2012) somehow without distinction inside the MBTC. Methods allowing distinction were estimated at $50 for genotyping MTBC (Singh et al., 2013) and $300–500 for WGS (Takiff and Feo, 2015), the latter two requiring several hours of experimentation. The applicability of the method for other laboratories is also consolidated by the correct classification rates obtained on isolates grown on different culture media routinely used in clinical mycobacteriology laboratories and by the observation of the specific biomarker independent of the medium used (94% for M7H10 medium, 87% for Coletsos medium). These results cannot be fully compared to the correct classification rate of 87.5% obtained for isolates grown on TransBK m4 agar medium due to the limited number of isolates used to assess the transposition of the method to other media (17 isolates grown on M7H10/Coletsos medium compared to 80 isolates grown on TransBK m4 agar medium).
Finally, as this work provides proof-of-concept by presenting preliminary results, variations in parameters such as the utilization of isolated growth in broth have not yet been investigated. The consistency of the discrimination described here on data generated on isolate growth in broth is of interest, as liquid medium results in a relatively quicker time of cultivation of mycobacteria than solid medium. A multicentric study performed in several countries, with different growth media (both liquid and solid) and MALDI-TOF devices, would confirm the reproducibility of this method for any routine laboratory. Sufficient data collection generated by this kind of study would be used to refine the model generated by the classifier algorithm and could allow further discrimination inside the MBTC.
The method presented here not only consisted of matching with a database, as reported for most previous studies using MALDI-TOF-MS identification of mycobacteria as a whole and MBTC in particular (Patel, 2015; Alcaide et al., 2018), but was completed with a method of biomarker-based discrimination. We postulate that this screening method is efficient, fast, and inexpensive that refines the identification within the MCTB, presenting a positive predictive value of 100%, indicating that the observation of identified peaks in spectra leads to the confirmation of the identification of isolates as M. tuberculosis.
Data Availability Statement
Publicly available datasets were analyzed in this study. This data can be found here: https://www.mediterranee-infection.com/acces-ressources/donnees-pour-articles/reference-group-spectra_rapid-screening-method-for-identification-in-the-mycobacterium-tuberculosis/.
Author Contributions
SR designed the experiments, analyzed and interpreted the data, and wrote the manuscript. JS and MM performed genomic analyses. ZH and FT collected samples and performed routine analyses. SB analyzed and validated the data, and wrote the manuscript. MD contributed to critically reviewing the manuscript, data interpretation, and validation. All authors declare that they have read and approved the manuscript.
Funding
This work was supported by the French Government under the “Investissements d’Avenir” (Investments for the Future) program managed by the Agence Nationale de la Recherche (ANR, fr: National Agency for Research) (reference: Méditerranée Infection 10-IAHU-03).
Conflict of Interest
MD is a co-founder and shareholder of Culture-Top, a startup whose TransBK m4 agar plate product is cited in this study.
The remaining authors declare that the research was conducted in the absence of any commercial or financial relationships that could be construed as a potential conflict of interest.
Publisher’s Note
All claims expressed in this article are solely those of the authors and do not necessarily represent those of their affiliated organizations, or those of the publisher, the editors and the reviewers. Any product that may be evaluated in this article, or claim that may be made by its manufacturer, is not guaranteed or endorsed by the publisher.
Acknowledgments
We are very grateful to Gilles Liotaud, Simon Pinchemel, and Amaël Fadlane (Assistance Publique-Hôpitaux de Marseille, BSL3 laboratory and Strain Collection of the Rickettsia Unit) for their technical work on mycobacterial culture. Finally, we would like to acknowledge the Head of the Laboratoire National de Référence des IST/VIH et de la Tuberculose, in Niamey, Niger.
Supplementary Material
The Supplementary Material for this article can be found online at: https://www.frontiersin.org/articles/10.3389/fmicb.2022.753969/full#supplementary-material
Footnotes
- ^ https://www.mediterranee-infection.com
- ^ (https://www.mediterranee-infection.com/accesressources/donnees-pour-articles/reference-group-spectra_rapid-screening-method-for-identification-in-the-mycobacteriumtuberculosis/)
References
Alcaide, F., Amlerová, J., Bou, G., Ceyssens, P. J., Coll, P., Corcoran, D., et al. (2018). How to: identify non-tuberculous Mycobacterium species using MALDI-TOF mass spectrometry. Clin. Microbiol. Infect. 24, 599–603. doi: 10.1016/j.cmi.2017.11.012
Bacanelli, G., Olarte, L. C., Silva, M. R., Rodrigues, R. A., Carneiro, P. A. M., Kaneene, J. B., et al. (2019). Matrix assisted laser desorption ionization-time-of-flight mass spectrometry identification of Mycobacterium bovis in Bovinae. J. Vet. Med. Sci. 81, 1400–1408. doi: 10.1292/jvms.19-0214
Bottai, D., Frigui, W., Sayes, F., Di Luca, M., Spadoni, D., Pawlik, A., et al. (2020). TbD1 deletion as a driver of the evolutionary success of modern epidemic Mycobacterium tuberculosis lineages. Nat. Commun. 11:684. doi: 10.1038/s41467-020-14508-5
Bouzid, F., Astier, H., Osman, D. A., Javelle, E., Hassan, M. O., Simon, F., et al. (2018). Extended spectrum of antibiotic susceptibility for tuberculosis, Djibouti. Int. J. Antimicrob. Agents 51, 235–238. doi: 10.1016/j.ijantimicag.2017.07.007
Brites, D., Loiseau, C., Menardo, F., Borrell, S., Boniotti, M. B., Warren, R., et al. (2018). A new phylogenetic framework for the animal-adapted Mycobacterium tuberculosis complex. Front. Microbiol. 9:2820. doi: 10.3389/fmicb.2018.02820
Brosch, R., Gordon, S. V., Marmiesse, M., Brodin, P., Buchrieser, C., Eiglmeier, K., et al. (2002). A new evolutionary scenario for the Mycobacterium tuberculosis complex. Proc. Natl. Acad. Sci. U.S.A. 99, 3684–3689. doi: 10.1073/pnas.052548299
Camoez, M., Sierra, J. M., Dominguez, M. A., Ferrer-Navarro, M., Vila, J., and Roca, I. (2016). Automated categorization of methicillin-resistant Staphylococcus aureus clinical isolates into different clonal complexes by MALDI-TOF mass spectrometry. Clin. Microbiol. Infect. 22, 161.e1–161.e7. doi: 10.1016/j.cmi.2015.10.009
Christner, M., Trusch, M., Rohde, H., Kwiatkowski, M., Schlüter, H., Wolters, M., et al. (2014). Rapid MALDI-TOF mass spectrometry strain typing during a large outbreak of Shiga-Toxigenic Escherichia coli. PLoS One 9:e101924. doi: 10.1371/journal.pone.0101924
Fangous, M.-S., Mougari, F., Gouriou, S., Calvez, E., Raskine, L., Cambau, E., et al. (2014). Classification algorithm for subspecies identification within the Mycobacterium abscessus species, based on matrix-assisted laser desorption ionization-time of flight mass spectrometry. J. Clin. Microbiol. 52, 3362–3369. doi: 10.1128/JCM.00788-14
Forbes, B. A., Hall, G. S., Miller, M. B., Novak, S. M., Rowlinson, M.-C., Salfinger, M., et al. (2018). Practice guidelines for clinical microbiology laboratories: mycobacteria. Clin. Microbiol. Rev. 31:e00038-17. doi: 10.1128/CMR.00038-17
Iketleng, T., Lessells, R., Dlamini, M. T., Mogashoa, T., Mupfumi, L., Moyo, S., et al. (2018). Mycobacterium tuberculosis next-generation whole genome sequencing: opportunities and challenges. Tuberc. Res. Treat. 2018:1298542. doi: 10.1155/2018/1298542
Jagielski, T., Borówka, P., Bakuła, Z., Lach, J., Marciniak, B., Brzostek, A., et al. (2019). Genomic insights into the Mycobacterium kansasii complex: an update. Front. Microbiol. 10:2918. doi: 10.3389/fmicb.2019.02918
Kanade, S., Nataraj, G., Suryawanshi, R., and Mehta, P. (2012). Utility of MPT 64 antigen detection assay for rapid characterization of mycobacteria in a resource constrained setting. Indian J. Tuberc. 59, 92–96.
Keys, C. J., Dare, D. J., Sutton, H., Wells, G., Lunt, M., McKenna, T., et al. (2004). Compilation of a MALDI-TOF mass spectral database for the rapid screening and characterisation of bacteria implicated in human infectious diseases. Infect Genet. Evol. 4, 221–242. doi: 10.1016/j.meegid.2004.02.004
Lagier, J.-C., Drancourt, M., Charrel, R., Bittar, F., La Scola, B., Ranque, S., et al. (2017). Many more microbes in humans: enlarging the microbiome repertoire. Clin. Infect. Dis. 65, S20–S29. doi: 10.1093/cid/cix404
Loiseau, C., Brites, D., Moser, I., Coll, F., Pourcel, C., Robbe-Austerman, S., et al. (2019). Revised interpretation of the Hain lifescience genotype MTBC To differentiate Mycobacterium canettii and members of the Mycobacterium tuberculosis complex. Antimicrob. Agents Chemother. 63:e000159-19. doi: 10.1128/AAC.00159-19
Malone, K. M., and Gordon, S. V. (2017). Mycobacterium tuberculosis complex members adapted to wild and domestic animals. Adv. Exp. Med. Biol. 1019, 135–154. doi: 10.1007/978-3-319-64371-7_7
Nakano, S., Matsumura, Y., Ito, Y., Fujisawa, T., Chang, B., Suga, S., et al. (2015). Development and evaluation of MALDI-TOF MS-based serotyping for Streptococcus pneumoniae. Eur. J. Clin. Microbiol. Infect. Dis. 34, 2191–2198. doi: 10.1007/s10096-015-2468-9
O’Connor, J. A., Corcoran, G. D., O’Reilly, B., O’Mahony, J., and Lucey, B. (2020). Matrix-assisted laser desorption ionization-time of flight mass spectrometry (MALDI-TOF MS) for investigation of Mycobacterium tuberculosis complex outbreaks: a type dream? J. Clin. Microbiol. 58:e02077-19. doi: 10.1128/JCM.02077-19
Panteix, G., Gutierrez, M. C., Boschiroli, M. L., Rouviere, M., Plaidy, A., Pressac, D., et al. (2010). Pulmonary tuberculosis due to Mycobacterium microti: a study of six recent cases in France. J. Med. Microbiol. 59, 984–989. doi: 10.1099/jmm.0.019372-0
Patel, R. (2015). MALDI-TOF MS for the diagnosis of infectious diseases. Clin. Chem. 61, 100–111. doi: 10.1373/clinchem.2014.221770
Pinsky, B. A., and Banaei, N. (2008). Multiplex real-time PCR assay for rapid identification of Mycobacterium tuberculosis complex members to the species level. J. Clin. Microbiol. 46, 2241–2246. doi: 10.1128/JCM.00347-08
Prodinger, W. M., Indra, A., Koksalan, O. K., Kilicaslan, Z., and Richter, E. (2014). Mycobacterium caprae infection in humans. Expert Rev. Anti. Infect. Ther. 12, 1501–1513. doi: 10.1586/14787210.2014.974560
Richter, E., Weizenegger, M., Rüsch-Gerdes, S., and Niemann, S. (2003). Evaluation of genotype MTBC assay for differentiation of clinical Mycobacterium tuberculosis complex isolates. J. Clin. Microbiol. 41, 2672–2675. doi: 10.1128/JCM.41.6.2672-2675.2003
Riojas, M. A., McGough, K. J., Rider-Riojas, C. J., Rastogi, N., and Hazbón, M. H. (2018). Phylogenomic analysis of the species of the Mycobacterium tuberculosis complex demonstrates that Mycobacterium africanum, Mycobacterium bovis, Mycobacterium caprae, Mycobacterium microti and Mycobacterium pinnipedii are later heterotypic synonyms of Mycobacterium tuberculosis. Int. J. Syst. Evol. Microbiol. 68, 324–332. doi: 10.1099/ijsem.0.002507
Rodriguez-Temporal, D., Perez-Risco, D., Struzka, E. A., Mas, M., and Alcaide, F. (2017). Impact of updating the MALDI-TOF MS database on the identification of nontuberculous mycobacteria. J Mass Spectrom. 52, 597–602. doi: 10.1002/jms.3944
Saad, J., Baron, S., Lagier, J.-C., Drancourt, M., and Gautret, P. (2020). Mycobacterium bovis pulmonary tuberculosis after ritual sheep sacrifice in Tunisia. Emerg. Infect. Dis. 26, 1605–1607. doi: 10.3201/eid2607.191597
Saad, J., Phelippeau, M., Khoder, M., Lévy, M., Musso, D., and Drancourt, M. (2019). “Mycobacterium mephinesia”, a Mycobacterium terrae complex species of clinical interest isolated in French Polynesia. Sci. Rep. 9:11169. doi: 10.1038/s41598-019-47674-8
Saleeb, P. G., Drake, S. K., Murray, P. R., and Zelazny, A. M. (2011). Identification of mycobacteria in solid-culture media by matrix-assisted laser desorption ionization-time of flight mass spectrometry. J. Clin. Microbiol. 49, 1790–1794. doi: 10.1128/JCM.02135-10
Seng, P., Abat, C., Rolain, J. M., Colson, P., Lagier, J.-C., Gouriet, F., et al. (2013). Identification of rare pathogenic bacteria in a clinical microbiology laboratory: impact of matrix-assisted laser desorption ionization-time of flight mass spectrometry. J. Clin. Microbiol. 51, 2182–2194. doi: 10.1128/JCM.00492-13
Shea, J., Halse, T. A., Lapierre, P., Shudt, M., Kohlerschmidt, D., Van Roey, P., et al. (2017). Comprehensive whole-genome sequencing and reporting of drug resistance profiles on clinical cases of Mycobacterium tuberculosis in New York State. J. Clin. Microbiol. 55, 1871–1882. doi: 10.1128/JCM.00298-17
Singh, A. K., Maurya, A. K., Umrao, J., Kant, S., Kushwaha, R. A., Nag, V. L., et al. (2013). Role of genotype(®) Mycobacterium common mycobacteria/additional species assay for rapid differentiation between Mycobacterium tuberculosis complex and different species of non-tuberculous mycobacteria. J. Lab. Physicians 5, 83–89. doi: 10.4103/0974-2727.119847
Takiff, H. E., and Feo, O. (2015). Clinical value of whole-genome sequencing of Mycobacterium tuberculosis. Lancet Infect. Dis. 15, 1077–1090. doi: 10.1016/S1473-3099(15)00071-7
Tazerart, F., Saad, J., Niar, A., Sahraoui, N., and Drancourt, M. (2021a). Mycobacterium bovis Pulmonary tuberculosis, Algeria. Emerg. Infect. Dis. 27, 972–974. doi: 10.3201/eid2703.191823
Tazerart, F., Saad, J., Sahraoui, N., Yala, D., Niar, A., and Drancourt, M. (2021b). Whole genome sequence analysis of Mycobacterium bovis cattle isolates, Algeria. Pathogens 10:802. doi: 10.3390/pathogens10070802
Toihir, A. H., Rasolofo, V., Andrianarisoa, S. H., Ranjalahy, G. M., and Ramarokoto, H. (2011). Validation of an immunochromatographic assay kit for the identification of the Mycobacterium tuberculosis complex. Mem. Inst. Oswaldo Cruz. 106, 777–780. doi: 10.1590/s0074-02762011000600022
Wang, H.-Y., Lien, F., Liu, T.-P., Chen, C.-H., Chen, C.-J., and Lu, J.-J. (2018). Application of a MALDI-TOF analysis platform (ClinProTools) for rapid and preliminary report of MRSA sequence types in Taiwan. PeerJ 6:e5784. doi: 10.7717/peerj.5784
Wanzala, S. I., Nakavuma, J., Travis, D., Kia, P., Ogwang, S., Waters, W. R., et al. (2019). Retrospective analysis of archived pyrazinamide resistant Mycobacterium tuberculosis complex isolates from Uganda-evidence of interspecies transmission. Microorganisms 7:221.
Whipps, C. M., Butler, W. R., Pourahmad, F., Watral, V. G., and Kent, M. L. (2007). Molecular systematics support the revival of Mycobacterium salmoniphilum (ex Ross 1960) sp. nov., nom. rev., a species closely related to Mycobacterium chelonae. Int. J. Syst. Evol. Microbiol. 57, 2525–2531. doi: 10.1099/ijs.0.64841-0
World Health Organization [WHO] (2020). Global Tuberculosis Report. Available online at: https://www.who.int/teams/global-tuberculosis-programme/tb-reports (accessed September 29, 2021).
Keywords: Mycobacterium, Mycobacterium tuberculosis, tuberculosis, MALDI-TOF mass spectrometry, whole genome sequencing, identification
Citation: Robinne S, Saad J, Morsli M, Hamidou ZH, Tazerart F, Drancourt M and Baron SA (2022) Rapid Identification of Mycobacterium tuberculosis Complex Using Mass Spectrometry: A Proof of Concept. Front. Microbiol. 13:753969. doi: 10.3389/fmicb.2022.753969
Received: 10 August 2021; Accepted: 15 February 2022;
Published: 31 March 2022.
Edited by:
Carlos Alberto Moreira-Filho, University of São Paulo, BrazilReviewed by:
Oana Dumitrescu, Hospices Civils de Lyon, FranceWilly Ssengooba, Makerere University, Uganda
Copyright © 2022 Robinne, Saad, Morsli, Hamidou, Tazerart, Drancourt and Baron. This is an open-access article distributed under the terms of the Creative Commons Attribution License (CC BY). The use, distribution or reproduction in other forums is permitted, provided the original author(s) and the copyright owner(s) are credited and that the original publication in this journal is cited, in accordance with accepted academic practice. No use, distribution or reproduction is permitted which does not comply with these terms.
*Correspondence: Sophie Alexandra Baron, sophie.baron@ap-hm.fr