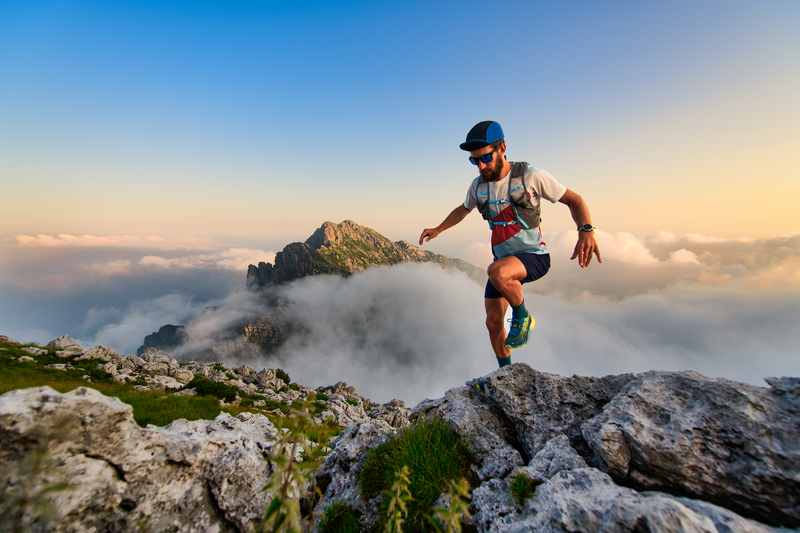
95% of researchers rate our articles as excellent or good
Learn more about the work of our research integrity team to safeguard the quality of each article we publish.
Find out more
ORIGINAL RESEARCH article
Front. Microbiol. , 10 January 2023
Sec. Infectious Agents and Disease
Volume 13 - 2022 | https://doi.org/10.3389/fmicb.2022.1099502
This article is part of the Research Topic Interactions of Microbial Biofilms with Advanced Materials View all 8 articles
Marine bacteria often exist in biofilms as communities attached to surfaces, like plastic. Growing concerns exist regarding marine plastics acting as potential vectors of pathogenic Vibrio, especially in a changing climate. It has been generalized that Vibrio vulnificus and Vibrio parahaemolyticus often attach to plastic surfaces. Different strains of these Vibrios exist having different growth and biofilm-forming properties. This study evaluated how temperature and strain variability affect V. parahaemolyticus and V. vulnificus biofilm formation and characteristics on glass (GL), low-density polyethylene (LDPE), polypropylene (PP), and polystyrene (PS). All strains of both species attached to GL and all plastics at 25, 30, and 35°C. As a species, V. vulnificus produced more biofilm on PS (p ≤ 0.05) compared to GL, and biofilm biomass was enhanced at 25°C compared to 30° (p ≤ 0.01) and 35°C (p ≤ 0.01). However, all individual strains’ biofilm biomass and cell densities varied greatly at all temperatures tested. Comparisons of biofilm-forming strains for each species revealed a positive correlation (r = 0.58) between their dry biomass weight and OD570 values from crystal violet staining, and total dry biofilm biomass for both species was greater (p ≤ 0.01) on plastics compared to GL. It was also found that extracellular polymeric substance (EPS) chemical characteristics were similar on all plastics of both species, with extracellular proteins mainly contributing to the composition of EPS. All strains were hydrophobic at 25, 30, and 35°C, further illustrating both species’ affinity for potential attachment to plastics. Taken together, this study suggests that different strains of V. parahaemolyticus and V. vulnificus can rapidly form biofilms with high cell densities on different plastic types in vitro. However, the biofilm process is highly variable and is species-, strain-specific, and dependent on plastic type, especially under different temperatures.
Vibrio parahaemolyticus and Vibrio vulnificus are two known marine pathogens that naturally exist in the marine environment and can infect both marine animals and humans (Baker-Austin et al., 2018). They are a major concern to human health as they commonly infect humans through consumption of raw seafood (Elmahdi et al., 2018). The Centers for Disease Control and Prevention (CDC) estimates that pathogenic Vibrio cause approximately 80,000 illnesses in the United States each year, with 52,000 of these cases likely being attributed to ingestion of contaminated seafood (Centers for Disease Control and Prevention, 2019). However, the exact number of cases of vibriosis is unknown due to underreporting in clinical settings, as a typical infection can present as symptoms like other common health problems (Baker-Austin et al., 2010; Bell and Bott, 2021). Symptoms of both V. parahaemolyticus and V. vulnificus infections include cramps, nausea, fever, and bloody diarrhea. V. vulnificus skin infections can be more severe and lead to rapid septicemia and death if an open wound encounters salt or brackish water (Centers for Disease Control and Prevention, 2019). Most bacterial diseases in humans are caused by biofilm infections, which are bacteria embedded within a self-secreted matrix that offers protection from the outside environment (Jamal et al., 2018).
Marine bacteria, including potentially pathogenic Vibrio species, often exist in biofilms, where communities of microbes are enclosed in a protective, self-secreted matrix of extracellular polymeric substances (EPS) and attached to a surface or as suspended aggregates (Decho and Gutierrez, 2017). The EPS matrix consists of organic polymers such as polysaccharides, proteins, and eDNA (extracellular DNA), and protects bacteria from environmental stresses like desiccation, changes in temperature and pH, competition and predation, sunlight exposure, and from low nutrient conditions (De Kievit et al., 2001; Stewart and William Costerton, 2001; Donlan, 2002; Zettler et al., 2013; Decho and Gutierrez, 2017; Lami, 2019). This matrix also contributes to enhanced protection of pathogenic strains from antibiotics and enhances virulence (Schroeder et al., 2017). In the past, most studies of bacteria have focused on analyses of individual planktonic cells in the water column. However, many natural marine bacteria, like Vibrio, often exist in biofilm states. Biofilms commonly occur on a variety of substrates in marine environments including animal carapaces, algae, ship hulls, and specifically plastics (Zettler et al., 2013; De Tender et al., 2015; Dang and Lovell, 2016; Lage and Graca, 2016; de Carvalho, 2018). Growth of biofilms can be influenced by environmental factors including temperature.
Temperature is a primary environmental variable that influences Vibrio planktonic and biofilm lifecycles, and contributes greatly to growth and habitat range (Gilbert et al., 2012; Tiruvayipati and Bhassu, 2016; Ward et al., 2017; Hernández-Cabanyero et al., 2020). This presents a possibility that bacterial cells enclosed in the biofilm matrix on plastic surfaces may be responding to environmental changes by exhibiting different growth and activity patterns compared to their planktonic counterparts (Guzmán-Soto et al., 2021). Most cases of vibriosis occur during summer months due to warmer sea surface temperatures in which the bacteria thrive. However, V. parahaemolyticus and V. vulnificus infections are increasing in prevalence due to climate change contributing to rising seawater temperatures and extending the length of time of warm sea surface temperatures (Parry et al., 2007; Whitehead et al., 2009; Baker-Austin et al., 2013, 2016; Vezzulli et al., 2016; Deeb et al., 2018; Centers for Disease Control and Prevention, 2019). Since these two Vibrio species are known to form biofilms and have been shown to be early colonizers of plastic surfaces, it follows that plastics could increase Vibrio exposure to humans (Kesy et al., 2021; Tavelli et al., 2022). Attached biofilms could contribute to higher bacterial concentrations in contaminated seafood, leading to increased levels of bacterial exposure to humans if consumed raw (Keswani et al., 2016; Kesy et al., 2021).
The hydrophobic or hydrophilic nature of the bacterial cell surface also plays a major role in bacteria’s ability to colonize and form biofilms on abiotic surfaces like plastics (Rosenberg, 1984; Reifsteck et al., 1987). More hydrophobic cells adhere more strongly to hydrophobic surfaces like plastic, while more hydrophilic cells adhere more strongly to hydrophilic surfaces like glass (Kochkodan et al., 2008; Giaouris et al., 2009). It is generally accepted that the lifecycles of pathogenic Vibrios, like V. parahaemolyticus and V. vulnificus, include natural environmental and host-associated stages (Kamp et al., 2013; Tiruvayipati and Bhassu, 2016; Ghenem et al., 2017; Hernández-Cabanyero and Amaro, 2020). It has been suggested that within marine environments, exposure to changes in temperature may increase the chances of survival and infectivity of V. parahaemolyticus and V. vulnificus within host-associated stages (Motes et al., 1998; Strom and Paranjpye, 2000; Froelich and Noble, 2016; Sullivan and Neigel, 2018). While studies have identified genotypic and phenotypic traits that allow these bacteria to survive within each environment, the ability to form biofilms on plastics, which could help the bacteria transition between the two environments by ingestion, is not well understood (Reidl and Klose, 2002; Oberbeckmann et al., 2015; Hernández-Cabanyero et al., 2019). There is an underlying knowledge gap regarding hydrophobicity of different strains of V. parahaemolyticus and V. vulnificus and their interactions with different types of plastics.
Bacterial colonization and biofilm development on surfaces involve multiple processes, one of which is material-type surface characteristics (Flemming, 2016). This means that hydrophobicity, hydrophilicity, and chemical composition of a surface like plastic can influence bacterial attachment and development (Nakanishi et al., 2021). There are several major types of plastic, which include polyethylene (PE), polypropylene (PP), and polystyrene (PS). There are increased probabilities that these plastic types end up in marine environments due to their high production and usage (Andrady, 2003, 2011; Brien, 2007; Eriksen et al., 2014; Lusher et al., 2017). Contamination of marine habitats by large pieces of plastics (macroplastics) has raised environmental concerns due to their possible transfer to animals that may coincidently or selectively ingest plastic particles that have been mistaken for food, leading to health complications and death (Gregory, 2009). In addition, plastics poorly degrade in marine environments, and this degradation leads to smaller particulates, deemed “microplastics,” which are classified as plastic particles smaller than 5 mm in size (Arthur et al., 2009; Eriksen et al., 2014; GESAMP, 1997). There are growing concerns that both macro- and micro-plastics can travel large distances and act as transport vectors for attached bacterial pathogens (Zettler et al., 2013; Oberbeckmann et al., 2015; Debroas et al., 2017; Kesy et al., 2019; Bowley et al., 2021).
Microbial communities associate and live on plastic surfaces in the marine environment. These plastic-associated communities have been termed the “Plastisphere,” and have raised serious implications for both marine life and human health (Ward and Kach, 2009; Zettler et al., 2013). Vibrio have been found to be a major community member on marine plastic particles, but Vibrio concentrations on plastic surfaces have appeared lower compared to natural marine particles (Bryant et al., 2016; Amaral-Zettler et al., 2020; Curren et al., 2020). However, since Vibrio biofilms have still been found on numerous macro- and micro-plastic substate surface types in several marine surface waters, this implies that plastic particles could act as transport vectors of potentially pathogenic Vibrio to new areas outside of their native range and to marine animals that may accidently or selectively ingest the biofilm-associated plastic particles coincidently with food particles (Goldstein et al., 2014; Reisser et al., 2014; Kirstein et al., 2016; Viršek et al., 2017; Bowley et al., 2021). In addition, since these bacteria are in close proximity to each other in biofilms on plastics, there is high potential for horizontal transfer of antibiotic-resistance genes, compounding the exposure risk to both marine and human health (Arias-Andres et al., 2018; Laverty et al., 2020).
In this study, we examined the effect of temperature on in vitro biofilm production by V. parahaemolyticus and V. vulnificus on different types of plastics, which included low-density polyethylene, polypropylene, and polystyrene. We compared biofilm production of both species, from three strains isolated from different sources (human, animal, and water), a total of six different strains. We hypothesized that all strains from both Vibrio species would produce greater amounts of biofilm on all plastic types compared to a glass (control) due to the increased hydrophobic properties of plastic, which make it a more suitable substrate for colonization. Higher temperatures for V. parahaemolyticus and lower temperatures for V. vulnificus should also lead to increased biofilm formation on plastics due to previous studies that have examined both species’ biofilm production under different temperature conditions. We also postulated that human isolated strains of both species would produce the greatest amount of biofilm on all plastic types compared to animal and seawater isolated strains due to the harsher survival conditions in human hosts compared to the marine environment.
Two clinical and two animal strains were obtained from the American Type Culture Collection (ATCC, Manassas, VA, United States). One seawater strain was gifted from the National Oceanic Atmospheric Administration (NOAA), Charleston, SC, United States, and was originally isolated from the marine environment (methods in Supplementary material, Vickery et al., 2007) in South Carolina, and one other seawater strain for this study was also directly isolated from the marine environment (methods in Supplementary material, Kim et al., 2015) in South Carolina (Table 1). Vibrio parahaemolyticus strains are commonly classified by their species marker (tlh) and capacity to infect humans through production of thermostable direct hemolysin (tdh) or thermostable direct hemolysin-related hemolysin (trh) virulence factors (Honda and Iida, 1993; Broberg et al., 2011). In our study, human isolated strain ATCC17802 contained tlh and trh, mollusk isolated strain ATCC43996 contained tlh and tdh, while the seawater isolated strain vpC12 only contained the species marker tlh. While V. vulnificus strains can also be classified by virulence factors, V. vulnificus can also be classified by 16S rRNA typing, which reveals if they are more clinically (type B, higher possible human infectivity) or more environmentally (type A, higher possible marine vertebrate infectivity) associated. In our study, the human isolated strain ATCC27562 and seawater isolated strain are type B, while eel isolated strain (ATCC33147) is type A.
One clinical, one animal, and one seawater isolated strains of both V. vulnificus and V. parahaemolyticus were tested for biofilm formation at different temperatures on different substrate surfaces. All strains were maintained in 25% (v/v) glycerol at −80°C to be used in further experiments. A single colony of each bacteria was inoculated in 5 ml modified seawater with yeast extract (MSYE, ATCC medium 804, Oliver and Colwell, 1973) broth supplemented with calcium chloride (1.8 g/l), as calcium chloride contributes to biofilm formation (Tischler et al., 2018), and incubated overnight at 35°C with shaking (180 rpm). After incubation, the broth culture was adjusted to 107 cells (OD600) using a SpectraMax M3 plate reader after calibrating the instrument’s absorbance values to cell counts from spread plating (Molecular Devices, San Jose, CA, United States).
Biofilm formation experiments were adapted from Hamanaka et al. (2012) and Valquier-Flynn et al. (2017). Disc coupons (Table 2, BioSurface Technologies, Boseman, MT, United States) were chemically sterilized (70% ethanol for GL and PP, 70% isopropanol for LDPE and PS) for 24 h and were then placed in sterile Petri dishes in a biosafety cabinet until residual alcohol evaporated. Then, the coupons were placed in 24-well sterile non-treated microplates (Costar®, Corning, NY, United States) or sterilized slide coupons (Biosurface Technologies) in sterile Petri dishes (Falcon®, Corning, NY, United States), and then were filled with 990 μl (24-well microplate) or 14.85 ml (Petri dish) of fresh MSYE broth supplemented with calcium chloride medium. The plates were then inoculated with 10 μl of the bacterial cultures for 24 well plates and 150 μl for Petri dishes (107 cells) to achieve a final cell density of 105 cells per well/dish. Then, the 24-well plates were incubated at 25, 30, and 35°C with low shaking (125 rpm) to form biofilms in 24 h, and Petri dishes were incubated at 30°C with low shaking (85 rpm) to form biofilms in 48 h, with spent media in Petri dishes being replaced with 15 ml fresh media after 24 h. Low shaking conditions, instead of static, were chosen to introduce shear stress to the biofilms, to better resemble the marine environment. Borosilicate glass coupons were chosen as the substrate type controls and used as the substrate reference for statistical analyses. Wells/dishes containing MSYE broth supplemented with calcium chloride without inoculation and with coupons were used as blank and group controls. Biofilm biomass on each disc coupon experimental and control group had biological triplicates and each experiment was conducted three times independently. Biofilm biomass on each slide coupon experimental group was pooled from 10 biological replicates one time. All plates/dishes were sealed with Parafilm™ (Bemis, Neenah, WI, United States) to reduce evaporative loss of media.
Biofilms of both V. parahaemolyticus and V. vulnificus were quantified by crystal violet staining according to O’Toole, (2011) and Valquier-Flynn et al. (2017) with some modifications. Following 24-h incubation, planktonic cells were removed from the 24-well microplates before gently washing with 1 × phosphate buffer saline (PBS, Molecular Biologicals International, Irvine, CA, United States) three times. 500 μl of 100% methanol (Sigma-Aldrich) was then added to the plates to fix the biofilms to the glass and plastics and incubated at room temperature for 20 min. Then, the methanol was removed, and residual methanol was allowed to evaporate off disc coupon surfaces. The biofilms were stained with 700 μl of 0.1% (w/v) crystal violet (Sigma-Aldrich) for 15 min at room temperature. The staining solution was removed, and then 1 × PBS was used to remove the non-bound dye four times. The glass and plastic coupons were then transferred to a new 24-well non-treated microplate and the stained and washed biofilms were air-dried overnight. Lastly, 600 μl of 30% acetic acid (Fisher Scientific) was added to dissolve the bound crystal violet and incubated at room temperature for 15 min. Optical densities of each well were measured by absorbance (570 nm) using a SpectraMax M3 plate reader (Molecular Devices). Mean OD570 values were then divided by the surface area (405 mm2) of the disc coupons tested to obtain final biofilm biomass values per mm of the surface type.
Total colony counts were determined from biofilm suspensions according to Portillo et al. (2013) and Bjerkan et al. (2009) with some modifications. Following 24-h incubation, planktonic cells were removed from the 24-well non-treated microplate wells before washing disc coupons with 700 μl 1 × PBS gently, four times. Then, disc coupons were placed individually in 10 ml 1 × PBS in a conical tube (Falcon®) and vortexed using a Vortex Genie 2® (Fisher Scientific) at the highest setting for 1 min. Then, coupons and 1 × PBS solution were individually transferred to borosilicate glass culture tubes (VWR International, Radnor, PA, United States) and placed in a Branson M2800 ultrasonication water bath (Branson Ultrasonics, Brookfield, CT, United States) and sonicated for 5 min at 40 kHz. The coupons and 1 × PBS solution were then transferred back to conical tubes, and vortexed again for 1 min. Then, the biofilm suspension in 1 × PBS was serially diluted in 1X PBS in conical tubes and 10−4 to 10−7 serial dilutions were spread onto prewarmed MSYE supplemented with calcium chloride agar plates. Plates were incubated at 30°C for 20–24 h. The viability of cells was determined in terms of colony-forming units (CFU) per coupon. Biofilm cell densities of each disc coupon experimental and control group had biological triplicates and each experiment was conducted three times independently. Mean CFU values were then log transformed and divided by the surface area (405 mm2) of the disc coupons tested to obtain final CFU values per mm of the surface type.
The strains of both Vibrio species that exhibited the greatest biofilm biomass, on average, combined on all plastic disc surface types were used for measuring cell and EPS concentrations. V. parahaemolyticus strain ATCC17802 (human) and V. vulnificus strain vv155 (seawater) exhibited the greatest mean combined biomass per mm2 of all plastic disc surfaces at 30°C (OD570/405mm2 ~ 4.17E-03). EPS extraction was conducted according to Bramhachari et al. (2007) with some modifications. Following 48-h incubation at 30°C, planktonic cells were removed from Petri dishes before washing slide coupon with 10 ml 0.85% saline gently two times. Then, the slide coupon was placed in 30 ml 0.85% saline in a conical tube and vortexed at highest setting for 1 min for plastics, and low setting for glass. Then, coupon and 0.85% saline solution were transferred to borosilicate glass test tube, and placed in water sonication bath, and sonicated for 5 min at 40 kHz. The coupon and saline solution were then transferred back to conical tube, and vortexed again for one minute. Lastly, the coupon was then scraped on all sides with a cell scraper (Falcon®, Corning, NY, United States), scraper submerged in solution, and coupon was removed. This was repeated 9 more times to pool 10 slide coupons’ total cell and EPS contents in 0.85% saline solution. Then, the 30 ml 0.85% saline biofilm suspension was centrifuged (4000 × g) to pellet cells. Cell pellet was then resuspended in the same solution, centrifuged again, and this process was repeated two more times. Cell pellet was saved at 4°C, while supernatant (EPS solution) was then immediately mixed with 75% total volume cold ethanol (VWR) overnight to precipitate the EPS. Total EPS and ethanol solution were then centrifuged to pellet EPS, the supernatant was removed, and the remaining EPS saved. The cell pellet and crude EPS were then freeze-dried using a FreeZone® 6 system (Labconco, Kansas City, MO, United States) and weighed.
The total carbohydrate content was measured after first dialyzing the EPS solution in SnakeSkin™ membrane with a 10,000 molecular weight cut-off (Fisher Scientific) in a borosilicate glass beaker of deionized water for 24 h at 4°C. Then the EPS solution was mixed with 75% total volume cold ethanol (VWR) overnight to precipitate the EPS. Total EPS and ethanol solution were then centrifuged to pellet EPS, the supernatant was removed, and the remaining EPS was saved. The EPS was then freeze-dried and weighed. This was repeated three times for each plastic type for (1) carbohydrate, (2) protein, and (3) eDNA quantification. (1) Dried crude EPS was prepared and carbohydrate content was quantified according to Dubois et al. (1951) using a Total Carbohydrate Assay Kit with glucose as the calibration standard according to the manufacturer’s instructions (Cell Biolabs, San Diego, CA, United States). The measurement was carried out using absorbance (490 nm; Molecular Devices, San Jose, CA, United States). (2) Dried crude EPS was prepared by using a Compat-Able™ Protein Assay Preparation Reagent kit (Fisher Scientific) according to Jiao et al. (2010) and manufacturer’s instructions. Then, the protein content was measured using a Bradford assay kit with bovine serum albumin (BSA) as the calibration standard according to the manufacturer’s instructions (Fisher Scientific). Absorbance measurements were conducted (595 nm). (3) Dried crude EPS was prepared according to Grande et al. (2015). EPS was resuspended in 1 ml 1X TE buffer (Fisher Scientific) and DNA was quantified using the Invitrogen Quant-iT™ PicoGreen™ dsDNA reagent kit (Molecular Probes, Eugene, OR, United States), with λ-DNA as the calibration standard according to the manufacturer’s instructions. Fluorescence was measured using a SpectraMax M3 plate reader (excit/emiss = 480/520 nm). % EPS by weight was calculated by standardization of each mean concentration of proteins, carbohydrates, and eDNA to μg/ml, then divided by total starting weight of pooled crude EPS from ten samples.
Microbial adherence to hydrocarbons was determined using p-xylene according to the MATH test method (Rosenberg, 1984; Kwaszewska et al., 2006; Mizan et al., 2016) with slight modifications. Briefly, overnight cultures of all strains in MSYE broth supplemented with calcium chloride were diluted to 105 cells and then grown at 25, 30, and 35°C for 24 h at 125 rpm. The cells were harvested by centrifugation (4000 × g) for 10 min, washed twice with 1 × PBS, and then resuspended in 1 × PBS to an OD600 ~ 0.3–0.6 (A0). One milliliter of p-xylene (Beantown Chemical, Hudson, NH, United States) was added to a conical tube containing four mL of the adjusted bacterial/PBS suspension and the mixture was then vortexed vigorously at the highest setting for two minutes and incubated for 20 min at room temperature to allow separation of the two phases. The supernatant (aqueous hydrocarbon phase) was then carefully removed using glass Pasteur pipettes and cellular absorbance was measured (OD600) in PBS suspension (A1). Hydrophobicity was calculated as the percentage of planktonic cells partitioning into the hydrocarbon phase. The percentage of p-xylene partitioning was estimated using the following formula: ([A0–A1]/A0) × 100 (Rivas et al., 2008). A mean adherence to p-xylene ≤ 30% indicated that the strains were hydrophilic; values > 30% signified hydrophobic strains. Highly hydrophobic strains exhibited values ≥ 70% (Kwaszewska et al., 2006). Each experimental and control group was completed in biological triplicate and each experiment was conducted independently three times.
The experimental data for biomass CFUs and hydrophobicity were expressed as the mean ± standard deviation. Biomass dry weights from slide coupons were expressed as mean total pooled biomass from 10 biological replicates. Biochemical characteristic weights of EPS were expressed as a percentage of the total pooled EPS weight of plastic type. Two-way analysis of variance (ANOVA) models were calculated using Rstudio software to compare value differences (α = 0.05). Strain, temperature, and surface type were the variables for all models. Glass was selected as the reference surface and 25°C was selected as the reference temperature for all analyses. Also, V. parahaemolyticus strain ATCC17802 was selected as the reference strain for all V. parahaemolyticus strains while V. vulnificus strain ATCC27562 was selected as the reference strain for all V. vulnificus strains. Bonferroni correction was calculated and applied to p-values to control for type 1 error. A t-test (α = 0.05) was calculated for comparison between mean total dry biomass weights between all plastics and glass and a Pearson’s correlation coefficient was calculated for comparison between mean total dry biomass weight and mean biofilm biomass absorbance data using Excel’s data analysis toolpak.
Experiments were conducted to test the effect of temperature (25, 30, and 35°C) on biofilm biomass production and biofilm cell viability on glass (GL), low-density polyethylene (LDPE), polypropylene (PP), and polystyrene (PS) by three different strains of both V. parahaemolyticus and V. vulnificus for 24 h. The vortex/sonication method as described previously was first tested to confirm efficacy of biofilm removal from all substate surfaces while also preserving cell viability (Supplementary Figures S1–S3; Supplementary Tables S14, S15). Raw mean data are presented in Supplementary Tables S1, S6.
The crystal violet staining assay reflects total bacterial biomass formed on the substate surface types. The biofilm removal and colony count assay reflects biofilm cell densities (expressed as colony-forming units, CFUs) on the substate surface types. From these two assays, it was shown that at a species level, both Vibrio parahaemolyticus and V. vulnificus appeared to have greater biofilm biomass and CFU concentrations at all temperatures tested on all combined plastic types compared to GL (Figure 1). V. parahaemolyticus formed greater biofilms and had slightly greater biofilm CFU concentrations at 30 and 35°C on all combined plastic types. V. vulnificus formed greater biofilm biomass at 25°C, but had slightly greater biofilm CFU concentrations at 30 and 35°C. The comparison of biofilm biomass between Vibrio species revealed high biomass variability between substrate surface composition types (glass vs. plastic) at different temperatures, as indicated by high standard deviation bars. Comparison of biofilm biomass between combined species isolated types (human, animal, and water) also revealed high biomass variability between all substrate surfaces at different temperatures (Supplementary Figure S4). However, these high standard deviation bars are due to high variability between species and strain types.
Figure 1. Plastics enhance Vibrio parahaemolyticus and Vibrio vulnificus biofilm formation compared to glass. Effect of temperature (°C) on mean biofilm biomass and colony-forming units (CFUs; means ± SD) by V. parahaemolyticus and V. vulnificus between glass and all plastics after 24 h (means of all biological triplicates and three independent experiments).
Examining biofilm formation on individual plastic types of LDPE, PP, and PS at different temperatures (25, 30, and 35°C) revealed that as a species V. parahaemolyticus appeared to form the greatest biofilms, on average, on LDPE and PP at 30 and 35°C and had higher CFU concentrations across all plastics compared to GL (Figure 2). Vibrio parahaemolyticus biofilm formation on PS was only marginally higher than GL at all temperatures yet still had an overall higher CFU concentration compared to GL. Vibrio parahaemolyticus formed the greatest biofilms across all temperatures on LDPE and PP, which have a specific density lower than seawater (~ 1.02), compared to PS and GL which have a higher specific density than seawater. Comparatively, as a species, V. vulnificus appeared to form greater biofilms, on average, on all plastic types at 25°C. Also, compared to V. parahaemolyticus, V. vulnificus biofilm formation was greatest on PS at 25°C. Vibrio vulnificus formed greater biofilms on LDPE at 35°C compared to 30°C, but this trend was opposite for PS as biofilm formation was greater at 30°C than at 35°C. Vibrio vulnificus biofilm biomass and CFU concentrations on LDPE and PS were also higher than GL across all temperatures. Vibrio vulnificus biofilm formation on PP was only slightly higher than GL at higher temperatures (30 and 35°C), and had lower biofilm biomass at 25°C and lower CFU concentrations on PP at 25 and 30°C compared to GL.
Figure 2. Surface material and temperature influence V. parahaemolyticus and V. vulnificus biofilm formation. Comparison of biofilm biomass and CFUs (means ± SD) by both V. parahaemolyticus and V. vulnificus between substate surface type at different temperatures after 24 h (means of all experiments). * = Significantly greater biofilm biomass compared to GL, ŧ = significantly less overall biofilm biomass compared to 25°C.
ANOVA revealed certain significant differences (α = 0.05) in the amount of biofilm formation on each plastic type compared to glass at the species level (Figure 2; Supplementary Tables S3–S5, S8–S10). Vibrio parahaemolyticus did not produce significantly more biofilm or significantly more CFUs on any plastic surface (p = 0.99) compared to GL. Temperature was also not a significant factor in contributing to V. parahaemolyticus biofilm biomass (30° p = 0.99, 35°C p = 0.99) or CFUs (30°C p = 0.99, 35°C p = 0.99). However, V. vulnificus produced significantly more biofilm, but not CFUs, on PS (p ≤ 0.05) compared to GL. Vibrio vulnificus biofilm biomass production was also significantly enhanced at 25°C compared to 30° (p ≤ 0.01) and 35°C (p ≤ 0.01).
At a strain level, the highest biofilm formation with a mean OD570 per mm2 (OD570/405mm2) of 5.92E-03 was obtained on LDPE and PP by V. parahaemolyticus strain ATCC17802 at 30°C, and the lowest biofilm formation with a mean OD570 per mm2 of 7.41E-05 was obtained on PP by V. parahaemolyticus strain vpC12 at 30°C. This further highlights the variability of biofilm formation between different strains of the same species (Figure 3A; Supplementary Table S1). All strains of both Vibrio species also had high concentrations of biofilm CFUs on GL and the three types of plastic over 24 h and under all temperature conditions. The highest CFU concentration was obtained on LDPE by V. vulnificus strain vv155 at 35°C, while the lowest CFU concentration was obtained on GL by V. vulnificus strain ATCC33147 at 30°C (Figure 3B; Supplementary Table S6). Further comparison of the individual strains revealed significant differences (p ≤ 0.05) in biofilm formation and CFUs between strains on different surfaces and temperatures (Figures 3A,B; Supplementary Tables S1, S6).
Figure 3. Strain type influences V. parahaemolyticus and V. vulnificus colonization and biofilm biomass and cell viability. Comparison of biofilm biomass and CFUs (means ± SD) by V. parahaemolyticus strains (A) and V. vulnificus strains (B) between glass and all plastic types at different temperatures after 24 h (means of all biological triplicates and three independent experiments).* = significantly greater biofilm biomass compared to GL, α = significantly greater CFUs compared to GL, Ŧ = significantly greater overall biofilm biomass compared to 25°C, ŧ = significantly less overall biofilm biomass compared to 25°C, Ć = significantly greater overall CFUs compared to 25°C.
Vibrio parahaemolyticus animal isolate (ATCC43996) and seawater isolate vpC12 produced significantly lower biofilm biomass (p ≤ 0.001) and CFUs (p ≤ 0.01) than human isolate ATCC17802 (Supplementary Tables S2, S7). Human isolated strain ATCC17802 had significantly greater biofilm formation (p ≤ 0.05) and CFU concentrations (p ≤ 0.01) on all plastic surfaces compared to glass. This strain also produced significantly greater biofilms and had greater CFU concentrations at 30 and 35°C (p ≤ 0.05) compared to 25°C. Animal isolated strain ATCC43996 also had significantly greater (p ≤ 0.01) biofilm formation on all plastic surfaces compared to GL. However, elevated temperatures (30 and 35°C) significantly decreased (p ≤ 0.01) the amount of overall biofilm produced by this strain compared to 25°C, but an increase in temperature had no significant effect (30°C, p = 0.2; 35°, p = 0.12) on CFU concentrations. Seawater isolated strain vpC12 did not have significantly greater (p > 0.05) biofilm biomass or CFU concentrations on any plastic surface compared to GL; however, elevated temperature (30°C) did lead to a significant decrease (p ≤ 0.05) in overall biofilm production compared to 25°C.
Vibrio vulnificus animal isolate ATCC33147 had no significant differences in biofilm biomass (p = 0.99) or CFU concentrations (p = 0.99) compared to human isolate ATCC27562 (Supplementary Table S2, S7). However, water isolate vv155 surprisingly produced significantly greater biofilm biomass (p ≤ 0.001) and had significantly higher CFU concentrations (p ≤ 0.05) than ATCC27562. Human isolated strain ATCC27562 did not have significantly greater (p > 0.05) biofilm biomass or CFU concentrations on any plastic surface compared to GL; however, elevated temperature (30 and 35°C) did lead to a significant decrease (p ≤ 0.01) in overall biofilm production. Animal isolated strain ATCC33147 had significantly greater (p ≤ 0.05) biofilm formation on PS compared to GL, and significantly greater (p ≤ 0.05) CFUs on LDPE compared to GL. However, elevated temperature (30 and 35°C) also led to a significant decrease (p ≤ 0.05) in overall biofilm production, but not in CFU concentrations, compared to 25°C. Seawater isolated strain vv155 had significantly greater (p ≤ 0.05) biofilm formation on PS compared to GL, but an increase in temperature had no significant effect (p > 0.05) on overall biofilm biomass and CFU concentrations.
Comparison of biofilm biomass between combined species isolated sources (human, animal, and water) revealed high biomass and CFU variability between surface types at different temperatures as indicated by high standard deviations (Supplementary Figure S3). While it appeared human isolated strains tended to produce, on average, greater biofilms and CFUs on LDPE and PP at higher temperatures (30 and 35°C), V. parahaemolyticus strain ATCC17802 mainly accounted for this high biofilm mean due to it being the greatest biofilm former at higher temperatures compared to V. vulnificus strain ATCC27562 that formed greater biofilms at 25°C across all surface types (Figures 3A,B; Supplementary Tables S1, S6). The other strain sources (animal and water) of both species formed greater biofilms, on average, at 25°C across all surface types than at higher temperatures (30 and 35°C).
Comparison of biofilm biomass between isolates and plastic surface types revealed differences in mean percent change compared to GL (Table 3). 41/54 total means of biofilm biomass on plastic across all temperatures had a mean positive percent change in biofilm biomass compared to GL. The greatest mean positive percent change compared to GL was observed with strain ATCC17802 on LDPE and PP at 25 and 30°C. Strains vpC12 and ATCC27562 accounted for 9/12 of the negative mean percent changes in biomass across all temperatures, meaning that they formed greater biofilms on GL, on average, compared to plastic in these cases. However, most of these negative percent changes were attributed to LDPE and PP compared to GL, as both strains had a mean positive percent change on PS compared to GL.
Table 3. Summary of biofilm biomass showing percent change (%) between all Vibrio parahaemolyticus and Vibrio vulnificus strains and plastic types at different temperatures compared to glass controls.
Comparison of biofilm CFU concentrations between isolates and plastic surface types at all temperatures tested revealed differences in % change compared to GL (Table 4). 42/54 total means of biofilm CFU concentrations on plastic across all temperatures had a mean positive % change in biofilm CFU concentrations compared to GL. The greatest mean positive % change compared to GL was observed with strain ATCC33137 at 30°C on LDPE. Strains vpC12 and ATCC27562 accounted for 8/12 of the negative mean % changes in biofilm CFUs across all temperatures, meaning that they had greater mean biofilm CFUs on GL compared to specific plastic types in these cases. However, strain vpC12 had a mean positive % change in CFU concentrations at 25°C on all plastic types, and strain ATCC27562 had a mean positive % change in CFU concentrations at 35°C on all plastic types. At 35°C, 5/6 strains had a mean positive % change in biofilm CFUs on all plastic types compared to glass and lower temperatures.
Table 4. Summary of biofilm CFUs showing percent change (%) between all V. parahaemolyticus and V. vulnificus strains and plastic types at different temperatures compared to glass controls.
Across all strains, V. parahaemolyticus strain ATCC17802 and V. vulnificus strain vv155 had the highest mean combined biomass per mm2 of all plastics at 30°C (OD570/405mm2 ~ 4.17E–03). These strains were chosen to be further analyzed for cell and EPS weight and EPS biochemical characterization. Comparison of ATCC17802 and vv155 strains combined total dry biomass on glass compared to plastic revealed significantly greater total dry biomass weights on all plastic types compared to glass (p ≤ 0.01; Table 5). Further comparison revealed a moderately positive Pearson’s correlation coefficient (r = 0.58) between mean total dry biofilm biomass weights (mg) and mean biofilm biomass from crystal violet staining (OD570) of all surfaces of both strains.
Table 5. Estimated pooled cell and crude extracellular polymeric substance mean dry weight per slide coupon at 30°C after 48 h.
Biochemical characterization of both Vibrio species EPS revealed that extracellular proteins were the main component of the EPS, followed by carbohydrates and eDNA on all plastic types (Figure 4; Supplementary Table S11). Vibrio parahaemolyticus extracellular proteins accounted for 75, 77, and 76% of total EPS mass on LDPE, PP, and PS, respectively. V. parahaemolyticus extracellular carbohydrates made up 16, 21, and 18% of total EPS mass on LDPE, PP, and PS, respectively, and eDNA made up ~1% of total EPS mass on each plastic type. V. vulnificus extracellular proteins accounted for 80, 83, and 70% of total EPS mass on LDPE, PP, and PS, respectively. Vibrio vulnificus extracellular carbohydrates accounted for 17, 13, and 26% of total EPS on LDPE, PP, and PS, respectively, and eDNA also made up ~ 1% of total EPS mass on each plastic type.
Figure 4. Proteins are the main component of V. parahaemolyticus and V. vulnificus extracellular polymeric substances on plastics. % extracellular polymeric substance (EPS) by weight of biochemical characteristics of V. parahaemolyticus (ATCC17802) and V. vulnificus (vv155) on all plastic types. % EPS by weight was calculated by standardization of each mean concentration of proteins, carbohydrates, and eDNA to μg/ml, then divided by total starting weight of pooled crude EPS from ten samples.
The MATH method, which is based on the degree of adherence to the hydrocarbon-p-xylene interface, showed that all strains were moderately (values > 30%) to strongly (values ≥ 70%) adhesive to p-xylene, and thus considered hydrophobic, at all temperatures tested (Figure 5). Raw mean hydrophobicity data are presented in Supplementary Table S12 in the Supplementary Data. Most strains (5/6) became slightly less hydrophobic as temperature increased from 25 to 35°C. V. parahaemolyticus strain ATCC43996 was highly hydrophobic at 25 and 30°C while all V. vulnificus strains were highly hydrophobic at 25°C, with strain ATCC33147 also being highly hydrophobic at 30 and 35°C. At a species level, V. vulnificus was, on average, more hydrophobic than V. parahaemolyticus at all temperatures tested, especially at 30 and 35°C (19 and 16% more hydrophobic, respectively; Supplementary Table S13).
Figure 5. Lower temperatures increase Vibrio hydrophobicity. V. parahaemolyticus and V. vulnificus individual strain adherence (means ± SD) to p-xylene (%) at different temperatures (means of all biological triplicates and three independent experiments). Lines designate planktonic cell hydrophobicity from hydrophobic (30% adherence) to highly hydrophobic (70% adherence).
While plastic pollution in the marine environment remains a global concern, their role as substrates for microbial habitats and subsequently vectors for the dispersion of pathogenic or non-pathogenic bacteria must be further evaluated, especially under evolving climate change scenarios (Zettler et al., 2013; Kirstein et al., 2016). Vibrio parahaemolyticus and V. vulnificus are potential pathogenic bacteria that can infect both marine animals and humans. In past decades, Vibrio habitat range has increased and coincided with an increase in plastic production and growth. This expansion of Vibrio coupled with their potential to colonize and live on numerous plastic types will increase the potential risk of both marine animal and human exposure to Vibrio species. To better understand the emerging environmental and public health risks associated with bacterial colonization of plastic particles, studies are needed to determine how this process is affected by different substrate types under different environmental conditions, such as temperature. This study focused on how different bacterial strains from distinct isolation sources of both V. parahaemolyticus and V. vulnificus interact with common marine plastics, such as low-density polyethylene, polypropylene, and polystyrene, under different temperatures.
Bacterial cells have been shown to attach quicker and to grow and develop biofilms more rapidly on hydrophobic surfaces like plastics compared with hydrophilic surfaces like glass (Donlan, 2002). Our study further suggests plastic to be a more favorable substrate on average than glass for both Vibrio species at all temperatures tested under 24 h (Figure 1). Our study also indicates and further strengthens the assumption that Vibrio are early colonizers of plastics, especially LDPE, PP, and PS, as both V. parahaemolyticus and V. vulnificus were able to colonize and develop biofilms on these plastics within 24 h (Harrison et al., 2014; Kesy et al., 2021). Interestingly, most individual isolates besides V. parahaemolyticus ATCC17802 produced greater biofilm formation at lower temperature (25°C) compared to higher temperatures (30 and 35°C). This is in accordance with studies that have reported both V. parahaemolyticus and V. vulnificus biofilm growth in 96-well microplates under different temperature conditions (Han et al., 2016; Çam et al., 2019; Billaud et al., 2022). This suggests that Vibrio may produce greater amounts of biofilm as a survival mechanism in response to lower temperatures in the marine environment. However, when environmental conditions become more suitable and warmer, cells might be dispersing from these biofilms and contributing to higher planktonic cell concentrations (Townsley and Yildiz, 2015; Guilhen et al., 2017; Sheikh et al., 2022). In the context of climate change and public health, warming waters could be contributing to potentially higher exposure risk by this increased Vibrio biofilm dispersal leading to higher planktonic cell concentrations (Deeb et al., 2018).
The genus Vibrio has been reported to have “feast or famine” growth strategies, and the introduction of a new surface into a marine environment may provide a colonization opportunity niche which Vibrio rapidly respond to (Gilbert et al., 2012; Takemura et al., 2014; Westrich et al., 2016). However, while it did appear from our study that specific surface type could influence the colonization and biofilm development over a 24-h period, our study only observed V. parahaemolyticus and V. vulnificus colonization and biofilm development over 24-h on each individual plastic, so this process might be more undirected and driven by the colonization opportunity of a new surface. As there were visually observed differences in substrate flotation behavior and the substrates tested were confirmed to be different in specific density (Table 2), floatation behavior could also influence the adhesion of Vibrio species and, consequently, the production of biofilm on these substrates, especially in the context of in situ marine environments. This is important to note, as many studies have focused mainly on lower specific density plastics on the surface of marine environments as these plastic types are more easily observed, and not on plastics with higher specific density properties or on plastics that have lost buoyancy due to biofouling that are found at greater depths and in sediment (Zettler et al., 2013; Cózar et al., 2014; Van Sebille et al., 2015; Kirstein et al., 2016; Laverty et al., 2020; Delacuvellerie et al., 2022). While these two Vibrio species have been found in the ‘Plastisphere’ on the commonly occurring marine plastics assessed in the present study, studies on other Vibrio species colonization and biofilm development on different plastics, synthetic and organic polymers, and other substrate surfaces are still lacking both in vitro and in vivo.
There is high strain variability within Vibrio species in growth and biofilm formation. Strain variability has not been closely examined in plastic colonization (Whiting and Golden, 2002; Han et al., 2016; Odeyemi and Ahmad, 2017; Song et al., 2017; Çam and Brinkmeyer, 2020). V. parahaemolyticus human isolated strain ATCC17802 had the significantly greatest (p ≤ 0.01) biofilm formation on LDPE and PP compared to GL and compared to the other V. parahaemolyticus strains tested, especially at 30 and 35°C. Song et al. (2017) have also reported that pathogenic strains of V. parahaemolyticus form greater biofilms than non-pathogenic strains. This strain is positive for the trh gene, a known virulence factor, signifying that known V. parahaemolyticus human pathogenic strains can adequately colonize, and have considerable biofilm formation on plastics in a 24-h period, especially in warmer temperatures. Interestingly, without adjusting the CFUs per mm2 of surface type, it was also found that all V. parahaemolyticus isolates’ CFU concentrations on all plastic types had above the threshold dose needed to be infectious in humans (≥ 105 CFUs) at all temperatures tested (Marx et al., 2013).
Vibrio vulnificus seawater isolated strain vv155 had the highest biofilm formation on all plastics at 25°C and was significantly greater on PS compared to GL and compared to the other V. vulnificus strains tested. While seawater isolates are expected to be strong biofilm producers to survive harsh marine environmental conditions, the result that V. vulnificus human isolate ATCC27562 was not the highest biofilm former was surprising. It was expected that human isolates would have the highest biofilm production between all isolate sources due to being isolated from the more stressful environment of the human host. However, research conducted by Çam and Brinkmeyer (2020) revealed that both clinical and environmental strains of V. vulnificus formed greater biofilms at lower temperatures. While the V. vulnificus human isolated strain ATCC27562 did not have the highest biofilm formation on plastics compared to this V. vulnificus water isolate, it cannot be ruled out that potential human pathogenic strains have higher colonization ability of plastic materials. This is especially apparent as the vv155 strain has a 16S rRNA designated type B genotype, which has a strong association with clinical strains, meaning that it may have a high level of virulence in humans (Nilsson et al., 2003). While type A strains are more environmentally associated, infections in humans from type A have still been reported, and been shown to be more virulent in mice (LD50 = 105–106 CFU) when compared to type B strains (LD50 = 108 CFU; Amaro, 1992; Amaro and Biosca, 1996; Nilsson et al., 2003; Drake et al., 2007; Jones and Oliver, 2009; Çam et al., 2019; Wu et al., 2022). Only the V. vulnificus ATCC33147 type B strain CFUs on LDPE and PS had above the considered threshold LD50 dose of 105–106 CFUs (without adjusting the CFUs per mm2 of surface type) needed to be lethal in animals at all temperatures tested (Amaro and Biosca, 1996; Jeong and Satchell, 2012; Marx et al., 2013).
Biofilm biomass on substrate surfaces consists of the bacteria cells and their self-secreted extracellular polymeric substances (EPS) which are mainly comprised of biopolymers such as polysaccharides, proteins, and extracellular DNA (eDNA; Flemming, 2016; Decho and Gutierrez, 2017). These three major components of the EPS matrix contribute specific roles in biofilm formation, such as attachment and structural integrity (Dragoš and Kovács, 2017). The biofilm component dry mass, biochemical characteristics, and concentrations of EPS vary depending on the bacterial species and the environment in which the biofilm was grown/formed (Vu et al., 2009; Wagner et al., 2009; Villeneuve et al., 2011; Kavita et al., 2013). It is important to note that the dry cell and crude EPS mass and EPS biochemical concentrations obtained in this study might be underestimations of the total amount on the substrates tested, as portions of the weights and concentrations obtained from substrates might be lost during processing, and largely depend on the biofilm removal method, its removal efficiency, and EPS biochemical characterization treatments. Regardless, our study still observed a moderately positive correlation between the mean pooled dry biofilm biomass weight recovered from slide coupons and biofilm biomass from crystal violet staining of disc coupons, strengthening the assumption that crystal violet staining is an accurate method in estimating total biofilm biomass on substrates. Understanding the role of biochemical components in EPS may provide a further understanding of biofilm formation mechanisms of V. parahaemolyticus and V. vulnificus in their attachment to plastic substrates.
The quantitative analysis of the EPS from V. parahaemolyticus and V. vulnificus showed that extracellular proteins were the main component of EPS by mass of the mature biofilms on all plastic types, followed by carbohydrates then eDNA (Figure 4). These results suggested that extracellular proteins and carbohydrates were the main key components of the biofilm matrix of both species on plastics. These results are consistent with Li et al. (2020), which found extracellular proteins and carbohydrates were the main components of mature V. parahaemolyticus biofilms. To the best of our knowledge, this is one of the first studies to quantify and characterize V. vulnificus EPS and its overall biochemical characteristics, especially on plastics, compared to previous studies that focused more on genes that were correlated with biofilm formation (Joseph and Wright, 2004; Grau et al., 2008; Kim et al., 2011; Lee et al., 2013). However, as these three biochemical components did not quite equal 100% of the dry EPS mass of both species across all substrate types, there might be other smaller components that may be part of the EPS like metals, and further analysis is needed to confirm this in addition to identifying specific proteins and carbohydrates that make up both species EPS on plastics (Jiao et al., 2010).
The hydrophobicity of bacteria may differ between the strains of a species and may change in response to changes in environmental conditions (temperature, nutrient availability, etc.), growth phases, and growth state (planktonic vs biofilm; Nwanyanwu et al., 2012). The present results also indicate this, as both V. parahaemolyticus and V. vulnificus strains possess wide differences in their hydrophobicity in the planktonic state at different temperatures. Both Vibrio species were considered hydrophobic, with V. vulnificus being more hydrophobic than V. parahaemolyticus at all temperatures tested, especially at 30 and 35°C (19 and 16% more hydrophobic, respectively) based on their adhesion to p-xylene, a hydrocarbon (Supplementary Table S6). All individual strains were considered hydrophobic at all temperatures tested (Figure 5). Only one V. parahaemolyticus strain (ATCC43996) had strong adhesion to p-xylene and thus was considered highly hydrophobic at 25 and 30°C, while all V. vulnificus strains had strong adhesion to p-xylene at 25°C, and V. vulnificus strain ATCC33147 exhibited strong adhesion to hydrocarbons at all temperatures tested (Figure 5). These results confirm the high variability of the hydrophobicity of Vibrio species and strains’ planktonic cells, and that different temperatures can influence the degree of hydrophobicity (Lee and Yii, 1996; Wong and Chang, 2005; Mizan et al., 2016). The development of specific adaptive mechanisms of Vibrio to the toxicity and low bioavailability of these plastic substrates could contribute to the modification of its cell surface hydrophobicity to permit direct hydrophobic-hydrophobic interactions with these plastic substrates in initial colonization. This could lead to potential biodegradation of plastics as it has been reported that adequate hydrophobic/hydrophilic properties of bacteria can contribute to degradation of hydrocarbons (Krasowska and Sigler, 2014).
Taken together, these results indicate that different strain types of V. parahaemolyticus V. vulnificus can rapidly and adequately form biofilms with high viable cell concentrations on different plastic material types in vitro. However, this colonization process is highly variable and depends on species, strain, and plastic type, especially under different temperatures. Further studies are needed to compare these Vibrio in vitro plastic colonization processes to those found in the natural marine environment. While seawater surface temperature is monitored as it is predictive for Vibrio growth, this monitoring only accounts for planktonic cell growth and biofilms must also be included in monitoring. Seafood is already screened and tested for potential Vibrio contamination, but additional screening for plastic particles in seafood must also be considered as humans are likely to be frequently exposed to plastics particles as they have been found in high concentrations in commercially harvested seafood (Wu et al., 2019; Curren et al., 2020; Nicole, 2021). The present results highlight the ability of Vibrio species to form biofilms on plastics, and may need to be incorporated into forecast models for Vibrio risk to better predict potential human exposure to pathogenic Vibrios, especially under climate change scenarios (Jacobs et al., 2014; Deeb et al., 2018; Ferchichi et al., 2021). Lastly, as both V. parahaemolyticus and V. vulnificus have been demonstrated to rapidly colonize plastics, their ability to utilize and degrade LDPE, PP, and PS also needs to be further explored (Obuekwe et al., 2009; Heipieper et al., 2010; Harrison et al., 2014; Raghul et al., 2014).
The original contributions presented in the study are included in the article/Supplementary material, further inquiries can be directed to the corresponding author.
RL, AD, and RN conceived and designed the study, analyzed the data, corrected the draft, built the final version of the manuscript, and read and approved the submitted version. RL, KCV, AC, KA, and GA performed the lab experiments. LX and GC performed statistical analyses. RL wrote the first draft of the manuscript. All authors contributed to the article and approved the submitted version.
This work has been funded by the NIEHS Center for Oceans and Human Health and Climate Change Interactions at the University of South Carolina (grant #P01ES028942).
We would like to thank the Baruch Marine Research Laboratory for allowing us to stay and take marine water samples for the isolation of the Vibrio parahaemolyticus seawater strain.
The authors declare that the research was conducted in the absence of any commercial or financial relationships that could be construed as a potential conflict of interest.
All claims expressed in this article are solely those of the authors and do not necessarily represent those of their affiliated organizations, or those of the publisher, the editors and the reviewers. Any product that may be evaluated in this article, or claim that may be made by its manufacturer, is not guaranteed or endorsed by the publisher.
The Supplementary material for this article can be found online at: https://www.frontiersin.org/articles/10.3389/fmicb.2022.1099502/full#supplementary-material
Amaral-Zettler, L. A., Zettler, E. R., and Mincer, T. J. (2020). Ecology of the plastisphere. Nat. Rev. Microbiol. 18, 139–151. doi: 10.1038/s41579-019-0308-0
Amaro, C. (1992). Comparative study of phenotypic and virulence properties in Vibrio vulnificus biotype 1 and 2 obtained from a European eel farm experiencing mortalities. Dis. Aquat. Org. 13, 29–35. doi: 10.3354/dao013029
Amaro, C., and Biosca, E. G. (1996). Vibrio vulnificus biotype 2, pathogenic for eels, is also an opportunistic pathogen for humans. Appl. Environ. Microbiol. 62, 1454–1457. doi: 10.1128/aem.62.4.1454-1457.1996
Andrady, A. L. (2011). Microplastics in the marine environment. Marine Pollution Bulletin 62, 1596–1605.
Arias-Andres, M., Klümper, U., Rojas-Jimenez, K., and Grossart, H.-P. (2018). Microplastic pollution increases gene exchange in aquatic ecosystems. Environ. Pollut. 237, 253–261. doi: 10.1016/j.envpol.2018.02.058
Arthur, C., Baker, J., and Bamford, H. (2009). Proceedings of the International Research Workshop on the Occurrence, Effects, and Fate of Microplastic Marine Debris, September 9–11, 2008.
Baker-Austin, C., Oliver, J. D., Alam, M., Ali, A., Waldor, M. K., Qadri, F., et al. (2018). Vibrio spp. infections. Nat. Rev. Dis. Primers. 4, 1–19. doi: 10.1038/s41572-018-0005-8
Baker-Austin, C., Stockley, L., Rangdale, R., and Martinez-Urtaza, J. (2010). Environmental occurrence and clinical impact of Vibrio vulnificus and Vibrio parahaemolyticus: a European perspective. Environ. Microbiol. Rep. 2, 7–18. doi: 10.1111/j.1758-2229.2009.00096.x
Baker-Austin, C., Trinanes, J. A., Salmenlinna, S., Löfdahl, M., Siitonen, A., Taylor, N. G., et al. (2016). Heat wave–associated vibriosis, Sweden and Finland, 2014. Emerg. Infect. Dis. 22, 1216–1220. doi: 10.3201/eid2207.151996
Baker-Austin, C., Trinanes, J. A., Taylor, N. G., Hartnell, R., Siitonen, A., and Martinez-Urtaza, J. (2013). Emerging vibrio risk at high latitudes in response to ocean warming. Nat. Clim. Chang. 3, 73–77. doi: 10.1038/nclimate1628
Bell, A., and Bott, M. (2021). Vibriosis:: what you and your patients need to know. Dela. J. Public Health 7, 14–21. doi: 10.32481/djph.2021.01.005
Billaud, M., Seneca, F., Tambutté, E., and Czerucka, D. (2022). An increase of seawater temperature upregulates the expression of Vibrio parahaemolyticus virulence factors implicated in adhesion and biofilm formation. Front. Microbiol. 13. doi: 10.3389/fmicb.2022.840628
Bjerkan, G., Witsø, E., and Bergh, K. (2009). Sonication is superior to scraping for retrieval of bacteria in biofilm on titanium and steel surfaces in vitro. Acta Orthop. 80, 245–250. doi: 10.3109/17453670902947457
Bowley, J., Baker-Austin, C., Porter, A., Hartnell, R., and Lewis, C. (2021). Oceanic hitchhikers–assessing pathogen risks from marine microplastic. Trends Microbiol. 29, 107–116. doi: 10.1016/j.tim.2020.06.011
Bramhachari, P., Kishor, P. K., Ramadevi, R., Rao, B. R., and Dubey, S. K. (2007). Isolation and characterization of mucous exopolysaccharide (EPS) produced by Vibrio furnissii strain VB0S3. J. Microbiol. Biotechnol. 17, 44–51.
Broberg, C. A., Calder, T. J., and Orth, K. (2011). Vibrio parahaemolyticus cell biology and pathogenicity determinants. Microbes Infect. 13, 992–1001. doi: 10.1016/j.micinf.2011.06.013
Bryant, J. A., Clemente, T. M., Viviani, D. A., Fong, A. A., Thomas, K. A., Kemp, P., et al. (2016). Diversity and Activity of Communities Inhabiting Plastic Debris in the North Pacific Gyre. mSystems 1, e00024–e00016.
Çam, S., and Brinkmeyer, R. (2020). The effects of temperature, pH, and iron on biofilm formation by clinical versus environmental strains of Vibrio vulnificus. Folia Microbiol. 65, 557–566. doi: 10.1007/s12223-019-00761-9
Çam, S., Brinkmeyer, R., and Schwarz, J. R. (2019). Quantitative PCR enumeration of vcgC and 16S rRNA type a and B genes as virulence indicators for environmental and clinical strains of Vibrio vulnificus in Galveston Bay oysters. Can. J. Microbiol. 65, 613–621. doi: 10.1139/cjm-2018-0399
Centers for Disease Control and Prevention (2019). Vibrio species causing vibriosis. 2019-03-05 [2020-08-28]. https://www.cdc.gov/vibrio/index.html
Cózar, A., Echevarría, F., González-Gordillo, J. I., Irigoien, X., Úbeda, B., Hernández-León, S., et al. (2014). Plastic debris in the open ocean. Proc. Natl. Acad. Sci. 111, 10239–10244. doi: 10.1073/pnas.1314705111
Curren, E., Leaw, C. P., Lim, P. T., and Leong, S. C. Y. (2020). Evidence of marine microplastics in commercially harvested seafood. Front. Bioeng. Biotechnol. 8:562760. doi: 10.3389/fbioe.2020.562760
Dang, H., and Lovell, C. R. (2016). Microbial surface colonization and biofilm development in marine environments. Microbiol. Mol. Biol. Rev. 80, 91–138. doi: 10.1128/MMBR.00037-15
de Carvalho, C. C. C. R. (2018). Marine biofilms: a successful microbial strategy with economic implications. Front. Mar. Sci. 5. doi: 10.3389/fmars.2018.00126
De Kievit, T. R., Gillis, R., Marx, S., Brown, C., and Iglewski, B. H. (2001). Quorum-sensing genes in Pseudomonas aeruginosa biofilms: their role and expression patterns. Appl. Environ. Microbiol. 67, 1865–1873. doi: 10.1128/AEM.67.4.1865-1873.2001
De Tender, C. A., Devriese, L. I., Haegeman, A., Maes, S., Ruttink, T., and Dawyndt, P. (2015). Bacterial community profiling of plastic litter in the Belgian part of the North Sea. Environ. Sci. Technol. 49, 9629–9638. doi: 10.1021/acs.est.5b01093
Debroas, D., Mone, A., and Ter Halle, A. (2017). Plastics in the North Atlantic garbage patch: a boat-microbe for hitchhikers and plastic degraders. Sci. Total Environ. 599-600, 1222–1232. doi: 10.1016/j.scitotenv.2017.05.059
Decho, A. W., and Gutierrez, T. (2017). Microbial extracellular polymeric substances (EPSs) in ocean systems. Front. Microbiol. 8. doi: 10.3389/fmicb.2017.00922
Deeb, R., Tufford, D., Scott, G. I., Moore, J. G., and Dow, K. (2018). Impact of climate change on Vibrio vulnificus abundance and exposure risk. Estuaries Coasts 41, 2289–2303. doi: 10.1007/s12237-018-0424-5
Delacuvellerie, A., Géron, A., Gobert, S., and Wattiez, R. (2022). New insights into the functioning and structure of the PE and PP plastispheres from the Mediterranean Sea. Environ. Pollut. 295:118678. doi: 10.1016/j.envpol.2021.118678
Donlan, R. M. (2002). Biofilms: microbial life on surfaces. Emerg. Infect. Dis. 8, 881–890. doi: 10.3201/eid0809.020063
Dragoš, A., and Kovács, Á. T. (2017). The peculiar functions of the bacterial extracellular matrix. Trends Microbiol. 25, 257–266. doi: 10.1016/j.tim.2016.12.010
Drake, S. L., DePaola, A., and Jaykus, L.-A. (2007). An overview of Vibrio vulnificus and Vibrio parahaemolyticus. Compr. Rev. Food Sci. Food Saf. 6, 120–144. doi: 10.1111/j.1541-4337.2007.00022.x
Dubois, M., Gilles, K., Hamilton, J., Rebers, P., and Smith, F. (1951). A colorimetric method for the determination of sugars. Nature 168:167. doi: 10.1038/168167a0
Elmahdi, S., Parveen, S., Ossai, S., DaSilva, L. V., Jahncke, M., Bowers, J., et al. (2018). Vibrio parahaemolyticus and Vibrio vulnificus recovered from oysters during an oyster relay study. Appl. Environ. Microbiol. 84, e01790–e01717. doi: 10.1016/j.fm.2016.02.008
Eriksen, M., Lebreton, L., Carson, H., Thiel, M., Moore, C., Borerro, J., et al. (2014). Plastic pollution in the world's oceans. PLoS One 9:e111913. doi: 10.1371/journal.pone.0111913
Ferchichi, H., St-Hilaire, A., Ouarda, T. B. M. J., and Lévesque, B. (2021). Impact of the future coastal water temperature scenarios on the risk of potential growth of pathogenic vibrio marine bacteria. Estuar. Coast. Shelf Sci. 250:107094. doi: 10.1016/j.ecss.2020.107094
Froelich, B. A., and Noble, R. T. (2016). Vibrio bacteria in raw oysters: managing risks to human health. Philos. Trans. R. Soc. London B Biol. Sci. 371:20150209. doi: 10.1098/rstb.2015.0209
GESAMP (1997). “Towards safe and effective use of chemicals in coastal aquaculture.” Joint Group of Experts on the Scientific Aspects of Marine Environmental Protection Reports and Studies.
Ghenem, L., Elhadi, N., Alzahrani, F., and Nishibuchi, M. (2017). Vibrio parahaemolyticus: a review on distribution, pathogenesis, virulence determinants and epidemiology. Saudi J. Med. Med. Sci. 5, 93–103. doi: 10.4103/sjmms.sjmms_30_17
Giaouris, E., Chapot-Chartier, M.-P., and Briandet, R. (2009). Surface physicochemical analysis of natural Lactococcus lactis strains reveals the existence of hydrophobic and low charged strains with altered adhesive properties. Int. J. Food Microbiol. 131, 2–9. doi: 10.1016/j.ijfoodmicro.2008.09.006
Gilbert, J. A., Steele, J. A., Caporaso, J. G., Steinbrück, L., Reeder, J., Temperton, B., et al. (2012). Defining seasonal marine microbial community dynamics. ISME J. 6, 298–308. doi: 10.1038/ismej.2011.107
Goldstein, M. C., Carson, H. S., and Eriksen, M. (2014). Relationship of diversity and habitat area in North Pacific plastic-associated rafting communities. Mar. Biol. 161, 1441–1453. doi: 10.1007/s00227-014-2432-8
Grande, R., Di Marcantonio, M. C., Robuffo, I., Pompilio, A., Celia, C., Di Marzio, L., et al. (2015). Helicobacter pylori ATCC 43629/NCTC 11639 outer membrane vesicles (OMVs) from biofilm and planktonic phase associated with extracellular DNA (eDNA). Front. Microbiol. 6. doi: 10.3389/fmicb.2015.01369
Grau, B. L., Henk, M. C., Garrison, K. L., Olivier, B. J., Schulz, R. M., O'Reilly, K. L., et al. (2008). Further characterization of Vibrio vulnificus rugose variants and identification of a capsular and rugose exopolysaccharide gene cluster. Infect. Immun. 76, 1485–1497. doi: 10.1128/IAI.01289-07
Gregory, M. R. (2009). Environmental implications of plastic debris in marine settings--entanglement, ingestion, smothering, hangers-on, hitch-hiking and alien invasions. Philos. Trans. R. Soc. Lond. Ser. B Biol. Sci. 364, 2013–2025. doi: 10.1098/rstb.2008.0265
Guilhen, C., Forestier, C., and Balestrino, D. (2017). Biofilm dispersal: multiple elaborate strategies for dissemination of bacteria with unique properties. Mol. Microbiol. 105, 188–210. doi: 10.1111/mmi.13698
Guzmán-Soto, I., McTiernan, C., Gonzalez-Gomez, M., Ross, A., Gupta, K., Suuronen, E. J., et al. (2021). Mimicking biofilm formation and development: recent progress in in vitro and in vivo biofilm models. iScience 24:102443. doi: 10.1016/j.isci.2021.102443
Hamanaka, D., Onishi, M., Genkawa, T., Tanaka, F., and Uchino, T. (2012). Effects of temperature and nutrient concentration on the structural characteristics and removal of vegetable-associated pseudomonas biofilm. Food Control 24, 165–170. doi: 10.1016/j.foodcont.2011.09.021
Han, N., Mizan, M., Jahid, I., and Ha, S.-D. (2016). Biofilm formation by Vibrio parahaemolyticus on food and food contact surfaces increases with rise in temperature. Food Control 70, 161–166. doi: 10.1016/j.foodcont.2016.05.054
Harrison, J. P., Schratzberger, M., Sapp, M., and Osborn, A. M. (2014). Rapid bacterial colonization of low-density polyethylene microplastics in coastal sediment microcosms. BMC Microbiol. 14:232. doi: 10.1186/s12866-014-0232-4
Heipieper, H. J., Cornelissen, S., and Pepi, M. (2010). “Surface properties and cellular energetics of bacteria in response to the presence of hydrocarbons” in Handbook of Hydrocarbon and Lipid Microbiology. ed. K. N. Timmis (Berlin, Heidelberg: Springer Berlin Heidelberg), 1615–1624.
Hernández-Cabanyero, C., and Amaro, C. (2020). Phylogeny and life cycle of the zoonotic pathogen Vibrio vulnificus. Environ. Microbiol. 22, 4133–4148. doi: 10.1111/1462-2920.15137
Hernández-Cabanyero, C., Lee, C. T., Tolosa-Enguis, V., Sanjuán, E., Pajuelo, D., Reyes-López, F., et al. (2019). Adaptation to host in Vibrio vulnificus, a zoonotic pathogen that causes septicemia in fish and humans. Environ. Microbiol. 21, 3118–3139. doi: 10.1111/1462-2920.14714
Hernández-Cabanyero, C., Sanjuán, E., Fouz, B., Pajuelo, D., Vallejos-Vidal, E., Reyes-López, F. E., et al. (2020). The effect of the environmental temperature on the adaptation to host in the zoonotic pathogen Vibrio vulnificus. Front. Microbiol. 11:489. doi: 10.3389/fmicb.2020.00489
Honda, T., and Iida, T. (1993). The pathogenicity of Vibrio parahaemolyticus and the role of the thermostable direct haemolysin and related haemolysins. Rev. Med. Microbiol. 4, 106–113. doi: 10.1097/00013542-199304000-00006
Jacobs, J. M., Rhodes, M., Brown, C. W., Hood, R. R., Leight, A., Long, W., et al. (2014). Modeling and forecasting the distribution of Vibrio vulnificus in Chesapeake Bay. J. Appl. Microbiol. 117, 1312–1327. doi: 10.1111/jam.12624
Jamal, M., Ahmad, W., Andleeb, S., Jalil, F., Imran, M., Nawaz, M. A., et al. (2018). Bacterial biofilm and associated infections. Journal of the Chinese Medical Association 81, 7–11.
Jeong, H.-G., and Satchell, K. J. F. (2012). Additive function of Vibrio vulnificus MARTXVv and VvhA cytolysins promotes rapid growth and epithelial tissue necrosis during intestinal infection. PLoS Pathog. 8:e1002581. doi: 10.1371/journal.ppat.1002581
Jiao, Y., Cody, G. D., Harding, A. K., Wilmes, P., Schrenk, M., Wheeler, K. E., et al. (2010). Characterization of extracellular polymeric substances from acidophilic microbial biofilms. Appl. Environ. Microbiol. 76, 2916–2922. doi: 10.1128/AEM.02289-09
Jones, M. K., and Oliver, J. D. (2009). Vibrio vulnificus: disease and pathogenesis. Infect. Immun. 77, 1723–1733. doi: 10.1128/IAI.01046-08
Joseph, L. A., and Wright, A. C. (2004). Expression of Vibrio vulnificus capsular polysaccharide inhibits biofilm formation. J. Bacteriol. 186, 889–893. doi: 10.1128/JB.186.3.889-893.2004
Kamp, H. D., Patimalla-Dipali, B., Lazinski, D. W., Wallace-Gadsden, F., and Camilli, A. (2013). Gene fitness landscapes of vibrio cholerae at important stages of its life cycle. PLoS Pathogens 9:e1003800. doi: 10.1371/journal.ppat.1003800
Kavita, K., Mishra, A., and Jha, B. (2013). Extracellular polymeric substances from two biofilm forming Vibrio species: characterization and applications. Carbohydr. Polym. 94, 882–888. doi: 10.1016/j.carbpol.2013.02.010
Keswani, A., Oliver, D. M., Gutierrez, T., and Quilliam, R. S. (2016). Microbial hitchhikers on marine plastic debris: human exposure risks at bathing waters and beach environments. Mar. Environ. Res. 118, 10–19. doi: 10.1016/j.marenvres.2016.04.006
Kesy, K., Labrenz, M., Scales, B. S., Kreikemeyer, B., and Oberbeckmann, S. (2021). Vibrio colonization is highly dynamic in early microplastic-associated biofilms as well as on field-collected microplastics. Microorganisms 9:76. doi: 10.3390/microorganisms9010076
Kesy, K., Oberbeckmann, S., Kreikemeyer, B., and Labrenz, M. (2019). Spatial environmental heterogeneity determines young biofilm assemblages on microplastics in Baltic Sea Mesocosms. Front. Microbiol. 10. doi: 10.3389/fmicb.2019.01665
Kim, M., Park, J.-M., Um, H.-J., Lee, K.-H., Kim, H., Min, J., et al. (2011). The antifouling potentiality of galactosamine characterized from Vibrio vulnificus exopolysaccharide. Biofouling 27, 851–857. doi: 10.1080/08927014.2011.605521
Kim, H.-J., Ryu, J.-O., Lee, S.-Y., Kim, E.-S., and Kim, H.-Y. (2015). Multiplex PCR for detection of the vibrio genus and five pathogenic Vibrio species with primer sets designed using comparative genomics. BMC Microbiol. 15:239. doi: 10.1186/s12866-015-0577-3
Kirstein, I. V., Kirmizi, S., Wichels, A., Garin-Fernandez, A., Erler, R., Löder, M., et al. (2016). Dangerous hitchhikers? Evidence for potentially pathogenic Vibrio spp. on microplastic particles. Mar. Environ. Res. 120, 1–8. doi: 10.1016/j.marenvres.2016.07.004
Kochkodan, V., Tsarenko, S., Potapchenko, N., Kosinova, V., and Goncharuk, V. (2008). Adhesion of microorganisms to polymer membranes: a photobactericidal effect of surface treatment with TiO2. Desalination 220, 380–385. doi: 10.1016/j.desal.2007.01.042
Krasowska, A., and Sigler, K. (2014). How microorganisms use hydrophobicity and what does this mean for human needs? Front. Cell. Infect. Microbiol. 4:112. doi: 10.3389/fcimb.2014.00112
Kwaszewska, A. K., Brewczynska, A., and Szewczyk, E. M. (2006). Hydrophobicity and biofilm formation of lipophilic skin corynebacteria. Pol. J. Microbiol. 55, 189–193.
Lage, O., and Graca, a. P. (2016). “Biofilms: an extra coat on macroalgae,” in Algae - Organisms for Imminent Biotechnology. eds. N. Thajuddin and D. Dhanasekaran (IntechOpen).
Lami, R. (2019). “Chapter 3–quorum sensing in marine biofilms and environments” in Quorum Sensing. ed. G. Tommonaro (Cambridge, MA: Academic Press), 55–96.
Laverty, A. L., Primpke, S., Lorenz, C., Gerdts, G., and Dobbs, F. C. (2020). Bacterial biofilms colonizing plastics in estuarine waters, with an emphasis on Vibrio spp. and their antibacterial resistance. PLoS One 15:e0237704. doi: 10.1371/journal.pone.0237704
Lee, K.-J., Kim, J.-A., Hwang, W., Park, S.-J., and Lee, K.-H. (2013). Role of capsular polysaccharide (CPS) in biofilm formation and regulation of CPS production by quorum-sensing in Vibrio vulnificus. Mol. Microbiol. 90, 841–857. doi: 10.1111/mmi.12401
Lee, K. K., and Yii, K. C. (1996). A comparison of three methods for assaying hydrophobicity of pathogenic Vibrios. Letters in applied microbiology 23, 343–346.
Li, W., Wang, J. J., Qian, H., Tan, L., Zhang, Z., Liu, H., et al. (2020). Insights into the role of extracellular DNA and extracellular proteins in biofilm formation of Vibrio parahaemolyticus. Front. Microbiol. 11. doi: 10.3389/fmicb.2020.00813
Lusher, A. P., Hollman,, and Mendoza, J. (2017). Microplastics in fisheries and aquaculture: Status of knowledge on their occurrence and implications for aquatic organisms and food safety.
Marx, J., Hockberger, R., and Walls, R. (2013). Rosen's Emergency Medicine-concepts and Clinical Practice E-book: 2-volume Set. Amsterdam: Elsevier Health Sciences.
Mizan, M. F. R., Jahid, I. K., Kim, M., Lee, K.-H., Kim, T. J., and Ha, S.-D. (2016). Variability in biofilm formation correlates with hydrophobicity and quorum sensing among Vibrio parahaemolyticus isolates from food contact surfaces and the distribution of the genes involved in biofilm formation. Biofouling 32, 497–509. doi: 10.1080/08927014.2016.1149571
Motes, M., DePaola, A., Cook, D., Veazey, J., Hunsucker, J., Garthright, W., et al. (1998). Influence of water temperature and salinity on Vibrio vulnificus in northern gulf and Atlantic coast oysters (Crassostrea virginica). Appl. Environ. Microbiol. 64, 1459–1465. doi: 10.1128/AEM.64.4.1459-1465.1998
Nakanishi, E. Y., Palacios, J. H., Godbout, S., and Fournel, S. (2021). Interaction between biofilm formation, surface material and cleanability considering different materials used in pig facilities—an overview. Sustainability 13:5836. doi: 10.3390/su13115836
Nicole, W. (2021). Microplastics in seafood: how much are people eating? Environ. Health Perspect. 129:034001. doi: 10.1289/EHP8936
Nilsson, W. B., Paranjype, R. N., DePaola, A., and Strom, M. S. (2003). Sequence polymorphism of the 16S rRNA gene of Vibrio vulnificus is a possible indicator of strain virulence. J. Clin. Microbiol. 41, 442–446. doi: 10.1128/JCM.41.1.442-446.2003
Nwanyanwu, C., Alisi, C., Nweke, C., and Orji, J. (2012). Cell surface properties of phenol-utilizing bacteria isolated from petroleum refinery wastewater. J. Res. Biol. 2, 383–391.
Oberbeckmann, S., Lder, M. G. J., and Labrenz, M. (2015). Marine microplastic-associated biofilms a review. Environ. Chem. 12, 551–562. doi: 10.1071/EN15069
Obuekwe, C. O., Al-Jadi, Z. K., and Al-Saleh, E. S. (2009). Hydrocarbon degradation in relation to cell-surface hydrophobicity among bacterial hydrocarbon degraders from petroleum-contaminated Kuwait desert environment. Int. Biodeterior. Biodegradation 63, 273–279. doi: 10.1016/j.ibiod.2008.10.004
Odeyemi, O. A., and Ahmad, A. (2017). Population dynamics, antibiotics resistance and biofilm formation of Aeromonas and Vibrio species isolated from aquatic sources in northern Malaysia. Microb. Pathog. 103, 178–185. doi: 10.1016/j.micpath.2017.01.007
Oliver, J. D., and Colwell, R. R. (1973). Extractable lipids of gram-negative marine bacteria: phospholipid composition. J. Bacteriol. 114, 897–908. doi: 10.1128/jb.114.3.897-908.1973
O’Toole, G. A. (2011). Microtiter dish biofilm formation assay. J. Vis. Exp. 47:2437. doi: 10.3791/2437
Parry, M. L., Canziani, O. F., Palutikof, J. P., Van Der Linden, P. J., and Hanson, C. E. (2007). “IPCC, 2007: climate change 2007: impacts, adaptation and vulnerability. Contribution of working group II to the fourth assessment report of the intergovernmental panel on climate change.” Cambridge University Press, Cambridge, UK.
Portillo, M. E., Salvadó, M., Trampuz, A., Plasencia, V., Rodriguez-Villasante, M., Sorli, L., et al. (2013). Sonication versus vortexing of implants for diagnosis of prosthetic joint infection. J. Clin. Microbiol. 51, 591–594. doi: 10.1128/JCM.02482-12
Raghul, S., Bhat, S., Chandrasekaran, M., Francis, V., and Thachil, E. (2014). Biodegradation of polyvinyl alcohol-low linear density polyethylene-blended plastic film by consortium of marine benthic Vibrios. Int. J. Environ. Sci. Technol. 11, 1827–1834. doi: 10.1007/s13762-013-0335-8
Reidl, J., and Klose, K. E. (2002). Vibrio cholerae and cholera: out of the water and into the host. FEMS Microbiol. Rev. 26, 125–139. doi: 10.1111/j.1574-6976.2002.tb00605.x
Reifsteck, F., Wee, S., and Wilkinson, B. (1987). Hydrophobicity—hydrophilicity of staphylococci. J. Med. Microbiol. 24, 65–73. doi: 10.1099/00222615-24-1-65
Reisser, J., Shaw, J., Hallegraeff, G., Proietti, M., Barnes, D. K. A., Thums, M., et al. (2014). Millimeter-sized marine plastics: a new pelagic habitat for microorganisms and invertebrates. PLoS One 9:e100289. doi: 10.1371/journal.pone.0100289
Rivas, L., Fegan, N., and Dykes, G. A. (2008). Expression and putative roles in attachment of outer membrane proteins of Escherichia coli O157 from planktonic and sessile culture. Foodborne Pathogens & Disease 5, 155–164.
Rosenberg, M. (1984). Bacterial adherence to hydrocarbons: a useful technique for studying cell surface hydrophobicity. FEMS Microbiol. Lett. 22, 289–295. doi: 10.1111/j.1574-6968.1984.tb00743.x
Schroeder, M., Brooks, B. D., and Brooks, A. E. (2017). The complex relationship between virulence and antibiotic resistance. Genes 8:39. doi: 10.3390/genes8010039
Sheikh, H. I., Najiah, M., Fadhlina, A., Laith, A. A., Nor, M. M., Jalal, K. C. A., et al. (2022). temperature upshift mostly but not always enhances the growth of vibrio species: a systematic review. Front. Marine Sci. 9. doi: 10.3389/fmars.2022.959830
Song, X., Ma, Y., Fu, J., Zhao, A., Guo, Z., Malakar, P. K., et al. (2017). Effect of temperature on pathogenic and non-pathogenic Vibrio parahaemolyticus biofilm formation. Food Control 73, 485–491. doi: 10.1016/j.foodcont.2016.08.041
Stewart, P. S., and William Costerton, J. (2001). Antibiotic resistance of bacteria in biofilms. Lancet 358, 135–138. doi: 10.1016/S0140-6736(01)05321-1
Strom, M. S., and Paranjpye, R. N. (2000). Epidemiology and pathogenesis of Vibrio vulnificus. Microbes Infect. 2, 177–188. doi: 10.1016/S1286-4579(00)00270-7
Sullivan, T. J., and Neigel, J. E. (2018). Effects of temperature and salinity on prevalence and intensity of infection of blue crabs, Callinectes sapidus, by Vibrio cholerae, V. parahaemolyticus, and V. vulnificus in Louisiana. J. Invertebr. Pathol. 151, 82–90. doi: 10.1016/j.jip.2017.11.004
Takemura, A. F., Chien, D. M., and Polz, M. F. (2014). Associations and dynamics of Vibrionaceae in the environment, from the genus to the population level. Front. Microbiol. 5:38. doi: 10.3389/fmicb.2014.00038
Tavelli, R., Callens, M., Grootaert, C., Abdallah, M. F., and Rajkovic, A. (2022). Foodborne pathogens in the plastisphere: can microplastics in the food chain threaten microbial food safety? Trends Food Sci. Technol. 129, 1–10. doi: 10.1016/j.tifs.2022.08.021
Tiruvayipati, S., and Bhassu, S. (2016). Host, pathogen and the environment: the case of Macrobrachium rosenbergii, Vibrio parahaemolyticus and magnesium. Gut Pathogens 8:15. doi: 10.1186/s13099-016-0097-1
Tischler, A. H., Lie, L., Thompson, C. M., and Visick, K. L. (2018). Discovery of calcium as a biofilm-promoting signal for Vibrio fischeri reveals new phenotypes and underlying regulatory complexity. J. Bacteriol. 200, e00016–e00018. doi: 10.1128/JB.00016-18
Townsley, L., and Yildiz, F. H. (2015). Temperature affects c-di-GMP signalling and biofilm formation in vibrio cholerae. Environ. Microbiol. 17, 4290–4305. doi: 10.1111/1462-2920.12799
Valquier-Flynn, H., Wilson, C. L., Holmes, A. E., and Wentworth, C. D. (2017). Growth rate of Pseudomonas aeruginosa biofilms on slippery butyl methacrylate-co-ethylene Dimethacrylate (BMA-EDMA), glass and polycarbonate surfaces. J. Biotechnol. Biomater. 7:274. doi: 10.4172/2155-952X.1000274
Van Sebille, E., Wilcox, C., Lebreton, L., Maximenko, N., Hardesty, B. D., Van Franeker, J. A., et al. (2015). A global inventory of small floating plastic debris. Environ. Res. Lett. 10:124006. doi: 10.1088/1748-9326/10/12/124006
Vezzulli, L., Grande, C., Reid, P. C., Hélaouët, P., Edwards, M., Höfle, M. G., et al. (2016). Climate influence on vibrio and associated human diseases during the past half-century in the coastal North Atlantic. Proc. Natl. Acad. Sci. 113, E5062–E5071. doi: 10.1073/pnas.1609157113
Vickery, M. C., Nilsson, W. B., Strom, M. S., Nordstrom, J. L., and DePaola, A. (2007). A real-time PCR assay for the rapid determination of 16S rRNA genotype in Vibrio vulnificus. J. Microbiol. Methods 68, 376–384. doi: 10.1016/j.mimet.2006.02.018
Villeneuve, A., Bouchez, A., and Montuelle, B. (2011). In situ interactions between the effects of season, current velocity and pollution on a river biofilm. Freshw. Biol. 56, 2245–2259. doi: 10.1111/j.1365-2427.2011.02649.x
Viršek, M. K., Lovšin, M. N., Koren, Š., Kržan, A., and Peterlin, M. (2017). Microplastics as a vector for the transport of the bacterial fish pathogen species Aeromonas salmonicida. Mar. Pollut. Bull. 125, 301–309. doi: 10.1016/j.marpolbul.2017.08.024
Vu, B., Chen, M., Crawford, R. J., and Ivanova, E. P. (2009). Bacterial extracellular polysaccharides involved in biofilm formation. Molecules 14, 2535–2554. doi: 10.3390/molecules14072535
Wagner, M., Ivleva, N. P., Haisch, C., Niessner, R., and Horn, H. (2009). Combined use of confocal laser scanning microscopy (CLSM) and Raman microscopy (RM): investigations on EPS–matrix. Water Res. 43, 63–76. doi: 10.1016/j.watres.2008.10.034
Ward, J. E., and Kach, D. J. (2009). Marine aggregates facilitate ingestion of nanoparticles by suspension-feeding bivalves. Mar. Environ. Res. 68, 137–142. doi: 10.1016/j.marenvres.2009.05.002
Ward, C. S., Yung, C.-M., Davis, K. M., Blinebry, S. K., Williams, T. C., Johnson, Z. I., et al. (2017). Annual community patterns are driven by seasonal switching between closely related marine bacteria. ISME J. 11, 1412–1422. doi: 10.1038/ismej.2017.4
Westrich, J. R., Ebling, A. M., Landing, W. M., Joyner, J. L., Kemp, K. M., Griffin, D. W., et al. (2016). Saharan dust nutrients promote vibrio bloom formation in marine surface waters. Proc. Natl. Acad. Sci. 113, 5964–5969. doi: 10.1073/pnas.1518080113
Whitehead, P. G., Wilby, R. L., Battarbee, R. W., Kernan, M., and Wade, A. J. (2009). A review of the potential impacts of climate change on surface water quality. Hydrol. Sci. J. 54, 101–123. doi: 10.1623/hysj.54.1.101
Whiting, R. C., and Golden, M. H. (2002). Variation among Escherichia coli O157:H7 strains relative to their growth, survival, thermal inactivation, and toxin production in broth. Int. J. Food Microbiol. 75, 127–133. doi: 10.1016/S0168-1605(02)00003-X
Wong, H.-C., and Chang, C.-N. (2005). Hydrophobicity, cell adherence, cytotoxicity, and Enterotoxigenicity of starved Vibrio parahaemolyticus. J. Food Prot. 68, 154–156. doi: 10.4315/0362-028X-68.1.154
Wu, W., Jing, Z., Yu, X., Yang, Q., Sun, J., Liu, C., et al. (2019). Recent advances in screening aquatic products for Vibrio spp. TrAC Trends Anal. Chem. 111, 239–251. doi: 10.1016/j.trac.2018.11.043
Wu, Z., Wu, Y., Gao, H., He, X., Yao, Q., Yang, Z., et al. (2022). Identification and whole-genome sequencing analysis of Vibrio vulnificus strains causing pearl gentian grouper disease in China. BMC Microbiol. 22:200. doi: 10.1186/s12866-022-02610-1
Keywords: Vibrio vulnificus, Vibrio parahaemolyticus, biofilms, strain variability, extracellular polymeric substances, plastics, climate change
Citation: Leighton RE, Correa Vélez KE, Xiong L, Creech AG, Amirichetty KP, Anderson GK, Cai G, Norman RS and Decho AW (2023) Vibrio parahaemolyticus and Vibrio vulnificus in vitro colonization on plastics influenced by temperature and strain variability. Front. Microbiol. 13:1099502. doi: 10.3389/fmicb.2022.1099502
Received: 15 November 2022; Accepted: 16 December 2022;
Published: 10 January 2023.
Edited by:
Gururaja Perumal Pazhani, SRM Institute of Science and Technology, IndiaReviewed by:
Jessica L Jones, United States Food and Drug Administration, United StatesCopyright © 2023 Leighton, Correa Vélez, Xiong, Creech, Amirichetty, Anderson, Cai, Norman and Decho. This is an open-access article distributed under the terms of the Creative Commons Attribution License (CC BY). The use, distribution or reproduction in other forums is permitted, provided the original author(s) and the copyright owner(s) are credited and that the original publication in this journal is cited, in accordance with accepted academic practice. No use, distribution or reproduction is permitted which does not comply with these terms.
*Correspondence: Alan W. Decho, ✉ QXdkZWNob0BtYWlsYm94LnNjLmVkdQ==
Disclaimer: All claims expressed in this article are solely those of the authors and do not necessarily represent those of their affiliated organizations, or those of the publisher, the editors and the reviewers. Any product that may be evaluated in this article or claim that may be made by its manufacturer is not guaranteed or endorsed by the publisher.
Research integrity at Frontiers
Learn more about the work of our research integrity team to safeguard the quality of each article we publish.