- 1School of Biological Sciences, Victoria University of Wellington, Wellington, New Zealand
- 2Department of Marine Biology, Texas A&M University at Galveston, Galveston, TX, United States
- 3Department of Plant Biology, The Carnegie Institution, Stanford, CA, United States
- 4Climate Change Cluster, Faculty of Science, University of Technology Sydney, Broadway, NSW, Australia
- 5Department of Integrative Biology, Oregon State University, Corvallis, OR, United States
The mutualistic cnidarian–dinoflagellate symbiosis underpins the evolutionary success of stony corals and the persistence of coral reefs. However, a molecular understanding of the signalling events that lead to the successful establishment and maintenance of this symbiosis remains unresolved. For example, the phosphatidylinositol (PI) signalling pathway has been implicated during the establishment of multiple mutualistic and parasitic interactions across the kingdoms of life, yet its role within the cnidarian-dinoflagellate symbiosis remains unexplored. Here, we aimed to confirm the presence and assess the specific enzymatic composition of the PI signalling pathway across cnidaria and dinoflagellates by compiling 21 symbiotic anthozoan (corals and sea anemones) and 28 symbiotic dinoflagellate (Symbiodiniaceae) transcriptomic and genomic datasets and querying genes related to this pathway. Presence or absence of PI-kinase and PI-phosphatase orthologs were also compared between a broad sampling of taxonomically related symbiotic and non-symbiotic species. Across the symbiotic anthozoans analysed, there was a complete and highly conserved PI pathway, analogous to the pathway found in model eukaryotes. The Symbiodiniaceae pathway showed similarities to its sister taxon, the Apicomplexa, with the absence of PI 4-phosphatases. However, conversely to Apicomplexa, there was also an expansion of homologs present in the PI5-phosphatase and PI5-kinase groups, with unique Symbiodiniaceae proteins identified that are unknown from non-symbiotic unicellular organisms. Additionally, we aimed to unravel the putative functionalities of the PI signalling pathway in this symbiosis by analysing phosphoinositide (PIP)-binding proteins. Analysis of phosphoinositide (PIP)-binding proteins showed that, on average, 2.23 and 1.29% of the total assemblies of anthozoan and Symbiodiniaceae, respectively, have the potential to bind to PIPs. Enrichment of Gene Ontology (GO) terms associated with predicted PIP-binding proteins within each taxon revealed a broad range of functions, including compelling links to processes putatively involved in symbiosis regulation. This analysis establishes a baseline for current understanding of the PI pathway across anthozoans and Symbiodiniaceae, and thus a framework to target future research.
1. Introduction
Reef-building corals thrive in oligotrophic waters and form the basis of coral reef ecosystems. The success of this highly productive ecosystem relies on the symbiotic relationship formed between the cnidarian host (stony corals) and dinoflagellates of the family Symbiodiniaceae (LaJeunesse et al., 2004, 2018), whereby this symbiosis is underpinned by the mutual exchange of metabolic products. Host corals provide protection from the environment and supply the inorganic compounds needed for photosynthesis, whereas the symbionts provide the host with the majority of its metabolic carbon needs and enable efficient retention and recycling of nutrients (e.g., nitrogen) within the holobiont (Tanaka et al., 2006; Burriesci et al., 2012; Rädecker et al., 2015). Despite their biological and societal significance, coral reefs face unprecedented levels of degradation at a global scale (Frieler et al., 2013; Hughes et al., 2017). In particular, coral-dinoflagellate symbioses are sensitive to elevated temperatures, such that climate-driven ocean warming in the tropics is triggering increasingly frequent episodes of dysbiosis (termed “coral bleaching”) en masse (Frieler et al., 2013; Hughes et al., 2017).
Symbiodiniaceae taxa have evolved diverse physiological thresholds to environmental factors (Abrego et al., 2008; Howells et al., 2012; Hoadley et al., 2021; Nitschke et al., 2022). Certain Symbiodiniaceae species may confer thermal-tolerance to their host when harboured in abundance (Berkelmans and Van Oppen, 2006; Sampayo et al., 2008; Oliver and Palumbi, 2011; Claar et al., 2020), yet the molecular and physiological characteristics that underpin the specificity of symbioses between distinct Symbiodiniaceae taxa and host coral species remain poorly understood. Of particular interest are the molecular-level signalling events that lead to the successful establishment and maintenance of this symbiosis. Discovery-based analyses using diverse “omics” techniques have highlighted specific molecular pathways that likely play a significant role in regulating the symbiotic state (Lehnert et al., 2014; Rosic et al., 2015; Oakley et al., 2016; Bellantuono et al., 2018; Sproles et al., 2018a,b; Rosset et al., 2021), however, the exact functions of these pathways in the maintenance and establishment of the symbiosis are currently unknown.
One mechanism through which cellular messages are conveyed is by the phosphorylation and dephosphorylation of targeted compounds and proteins. This addition and removal of phosphate groups is carried out by the activities of kinase and phosphatase family enzymes, respectively. A key intracellular pathway that is activated through phosphorylation is the phosphatidylinositol (PI) signalling pathway (Figure 1). PI is among the least abundant of the mammalian phospholipids and is produced in the endoplasmic reticulum, before being transported to other cellular locations by phosphoinositide (PIP) transfer proteins (Dickson and Hille, 2019; Nakada-Tsukui et al., 2019). Coordinated activities of diverse PI kinases and phosphatases result in the production of seven distinct PIPs (Nakada-Tsukui et al., 2019) that occupy distinct locations within the cell, each enriched within a particular intracellular membrane (Dickson and Hille, 2019). These PIPs can act by binding directly to target proteins, referred to here as PIP-binding proteins. PIPs can also influence the membrane they embed in, or act as precursors to secondary messengers, known as inositol polyphosphates (IPPs) (Heilmann, 2016). PIPs have a wide variety of roles, regulating key cellular processes by triggering cellular signalling cascades, including those involved in immunity, apoptosis, vesicular trafficking, transmembrane signalling, ion channel regulation, lipid homoeostasis, and organelle identification (Underhill and Ozinsky, 2002; Di Paolo and De Camilli, 2006; Gericke et al., 2006; Thapa et al., 2012; Cernikova et al., 2020).
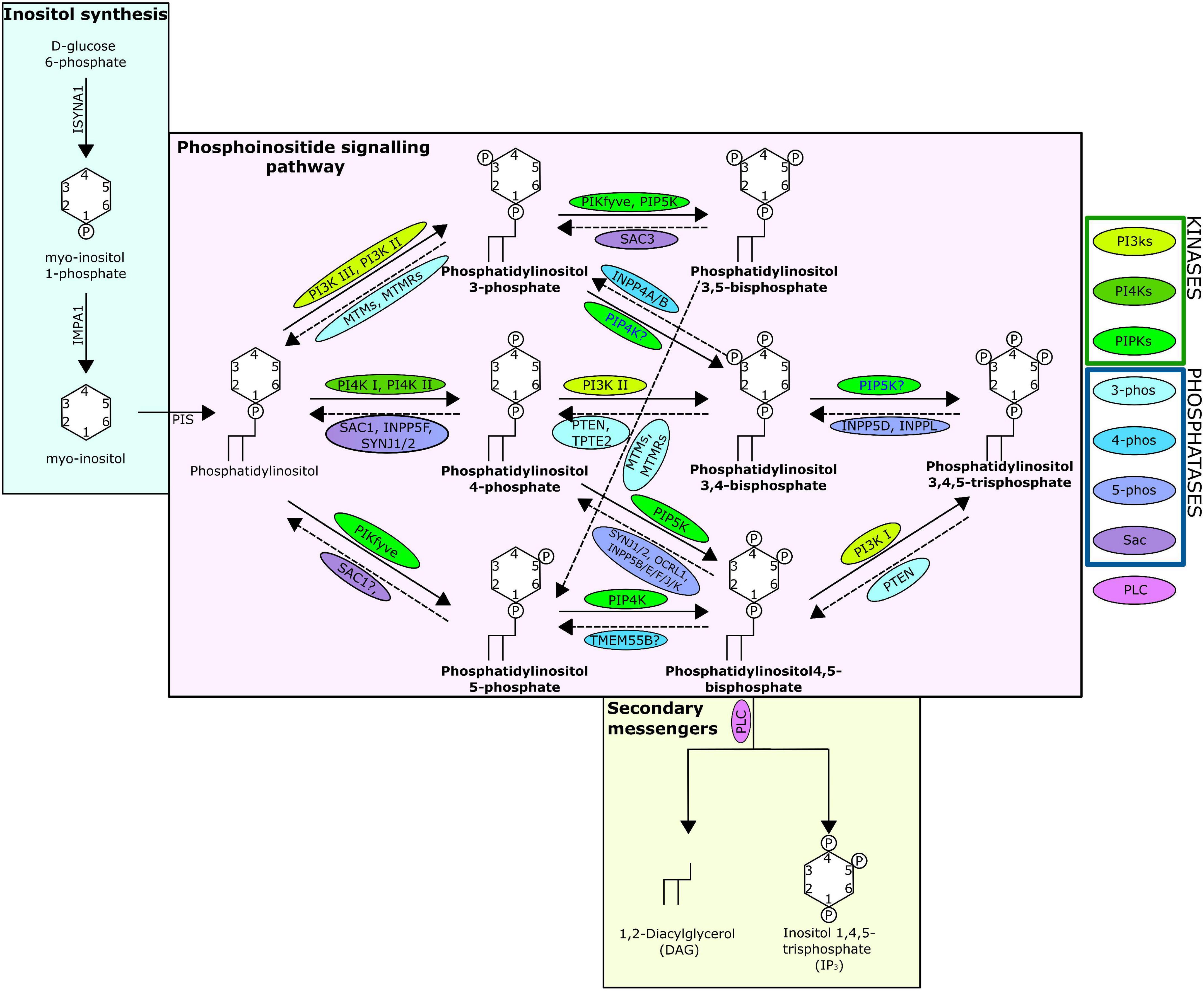
Figure 1. The phosphatidylinositol pathway as known from human studies. Solid arrows indicate kinase action; dashed indicate phosphatase action. Arrows are labelled with the kinases/phosphatases responsible for the reaction, question marks show where there are ambiguities surrounding the reaction, and the proteins are surrounded with a bubble coloured to indicate protein grouping from the key. The seven PIPs are indicated in bold and the structure of the lipids including the location and number of phosphates are shown. The absence of INPP5A is due to evidence suggesting no lipid phosphatase action (Pathway specifics derived from Dickson and Hille, 2019; Nakada-Tsukui et al., 2019).
Several studies indicate that modification of PI signalling pathway activity plays roles in host-microbe interactions. Parasitic apicomplexans—the sister taxon to Symbiodiniaceae—modify their host’s cells to ensure their own survival, using molecular mechanisms such as the excretion of effector proteins into the host cell to sabotage host signalling and thereby prevent the host from mounting an immune response (Plattner and Soldati-Favre, 2008; Lüder et al., 2009). PI signalling is important in regulating the excretion of proteins that facilitate host cell invasion by Plasmodium falciparum (Ebrahimzadeh et al., 2019). Additionally, apicomplexans of the genus Plasmodium utilise the PI3K/Akt component of the PI pathway to successfully infect the host; inhibition of Plasmodium PI3K (phosphatidylinositol 3-kinase) in this instance, strongly decreases successful infection (Leirião et al., 2005). Also, through the pharmacological inhibition of phosphatidylinositol 4-kinase (PI4K) in Plasmodium, the infection process can be prevented at all stages of the parasitic lifecycle (McNamara et al., 2013). Modification of host cell PI3K activity by Theileria functions to ensure host cell proliferation and parasite survival (Baumgartner et al., 2000). Various bacterial pathogens also target the host PI signalling pathway through the activity of effector proteins to facilitate host cell entry and intracellular survival (Ham et al., 2011).
Multiple transcriptomic and proteomic studies have shown that several proteins involved in the PI signalling pathway are differentially expressed in both cnidarian hosts and Symbiodiniaceae in response to symbiosis (Lehnert et al., 2014; Rosic et al., 2015; Weston et al., 2015; Hillyer et al., 2016; Matthews et al., 2017; Bellantuono et al., 2018; Sproles et al., 2018a; Maor-Landaw et al., 2020). Collectively, this evidence indicates that the regulation of the PI signalling pathway in either or both host and symbiont may be important in the establishment and maintenance of the cnidarian-dinoflagellate symbiosis. However, the exact roles of the PI signalling pathway in the interactions between Symbiodiniaceae and cnidarians, and the effects on the maintenance and regulation of this symbiosis are unknown. Yet, a targeted study of such a specific molecular pathway in non-model organisms is confined by poor gene annotation and resulting lack of complete information on the genes involved.
Here we conduct a comprehensive bioinformatic analysis to identify proteins involved in the PI signalling pathway across multiple anthozoan and Symbiodiniaceae species, providing the first composite analysis of the PI signalling pathway within the cnidarian-dinoflagellate symbiosis. Additionally, we assess functionality of identified PIP-binding proteins to understand what downstream cellular pathways and processes are affected by the activity of the PI signalling pathway across these organisms, and hence potential mechanisms by which the PI pathway regulates the establishment and maintenance of this symbiosis.
2. Materials and methods
2.1. Dataset acquisition and quality assessment
Transcriptomes and genomes of Symbiodiniaceae and symbiotic anthozoan species were obtained from publicly available sources (Symbiodiniaceae—Table 1, anthozoans—Table 2). For anthozoan species, we selected the highest-quality gene sets which also covered a range of hexacorals and Exaiptasia diaphana (n = 21 datasets). Due to the reduced number of available datasets for Symbiodiniaceae, all available assemblies (n = 28) were analysed, regardless of their quality. This spanned six genera, with most covering associations with hexacorals. BUSCO analysis, a metric to measure genome/transcriptome completeness through the identification of near-ubiquitous single-copy orthologs, was used to quantify the quality of these datasets (Manni et al., 2021). The range of BUSCO scores within the cnidarian datasets using the eukaryote database (v5) was between 78 and 93.8% complete, with an average of 87.8% (Figure 2). BUSCO completeness scores of the Symbiodiniaceae datasets using the eukaryote database (v5) ranged from 0.8 to 80.8%, with an average score of 49.2% (Figure 3). There was a particular focus throughout this analysis on the sea anemone Exaiptasia diaphana (“Aiptasia”), a widely used model organism for cnidarian-dinoflagellate symbiosis that can be maintained in an aposymbiotic state and re-colonised with multiple Symbiodiniaceae species (Weis et al., 2008).
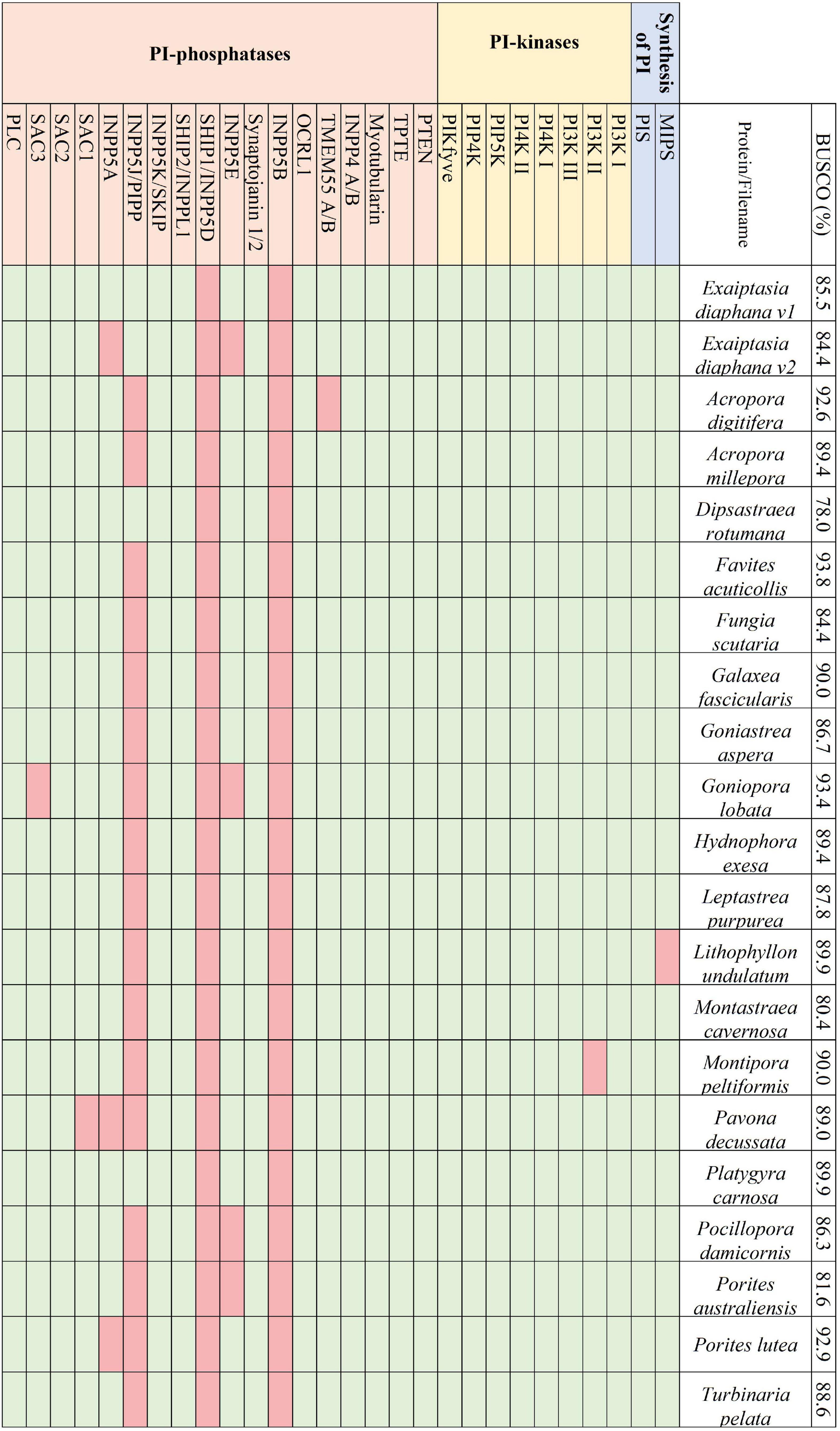
Figure 2. The presence and absence of the proteins constituting the PI pathway across the 21 selected symbiotic anthozoan assemblies and the respective BUSCO scores.
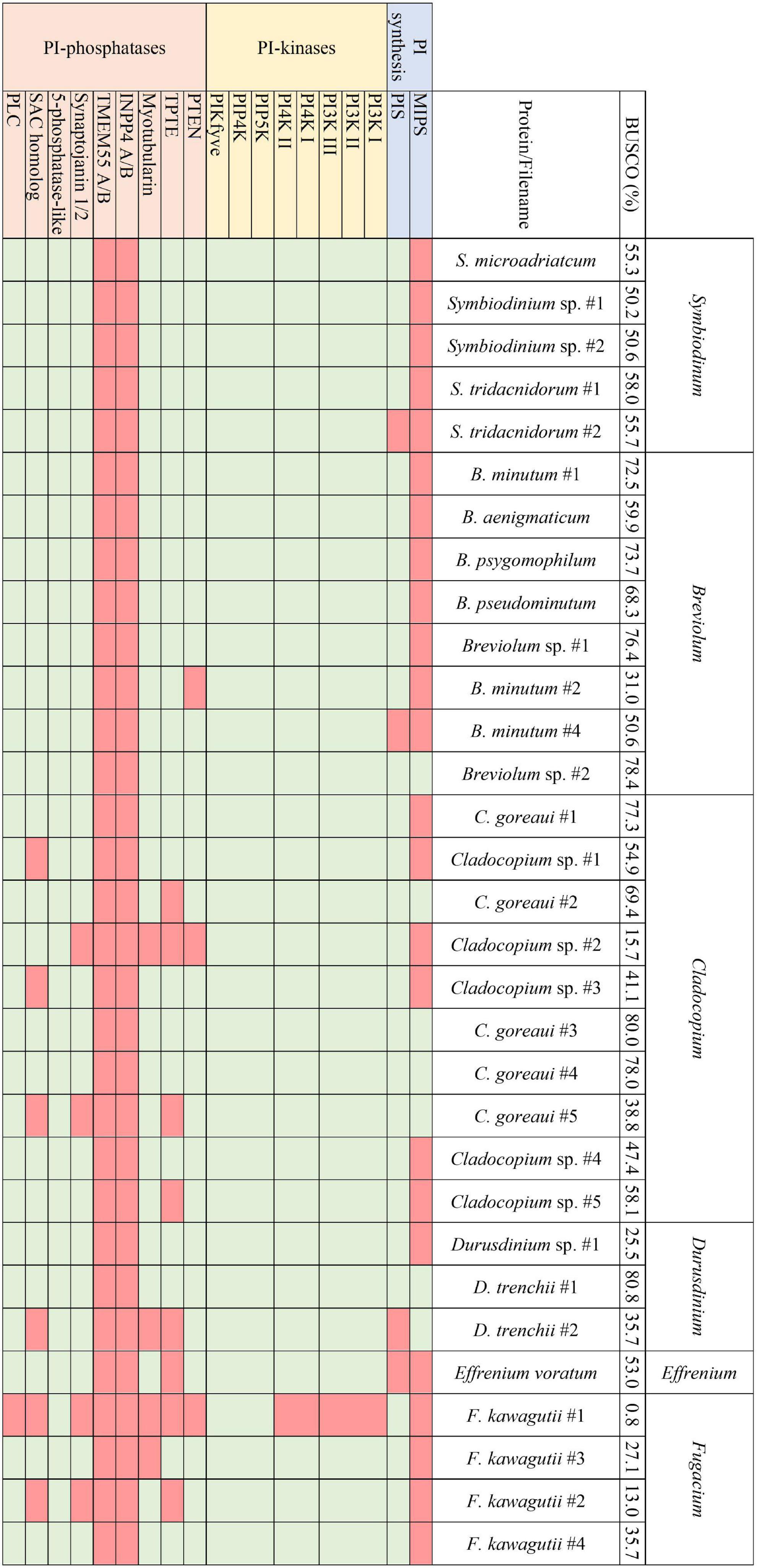
Figure 3. The presence and absence of the proteins constituting the PI pathway across all available Symbiodiniaceae assemblies and the respective BUSCO scores.
2.2. Bioinformatic identification of phosphatidylinositol signalling pathway proteins
A literature search was used to identify conserved domains of the PI kinases and phosphatases. The conserved domains were used to search the genomic and transcriptomic resources using the “aphid” R-package (Wilkinson, 2018). Specifically, 17 Hidden Markov Models (HMMs) that provided complete coverage of the PI signalling pathway were used as queries to identify proteins of interest (Table 3). Resulting proteins with a score >20 (an observed cut-off in quality and accuracy below this score) were run through the InterProScan plugin in Geneious (v2019.2.3) to identify additional domains. To verify the presence or absence of PI pathway genes, we also used: (1) a custom BLAST analysis against a database of the 28 Symbiodiniaceae and 21 cnidarian species (E-value cut-off 10–2); (2) motif identification through thorough literature searches; (3) protein alignments using Muscle in Geneious; and (4) similarity scores produced during alignments. Moreover, genes identified above were assigned to gene families, or orthogroups, using OrthoFinder 2.5.2 (Emms and Kelly, 2015). In complex cases, such as in the distinction between phosphatidylinositol 3- and 4-kinases, where the same domain is used to extract distinct target proteins of interest, maximum-likelihood phylogenetic trees were reconstructed using the conserved protein domains with RAxML (v4.0 in Geneious, Protein model: Gamma Blosum 62) (Supplementary Figure 1).
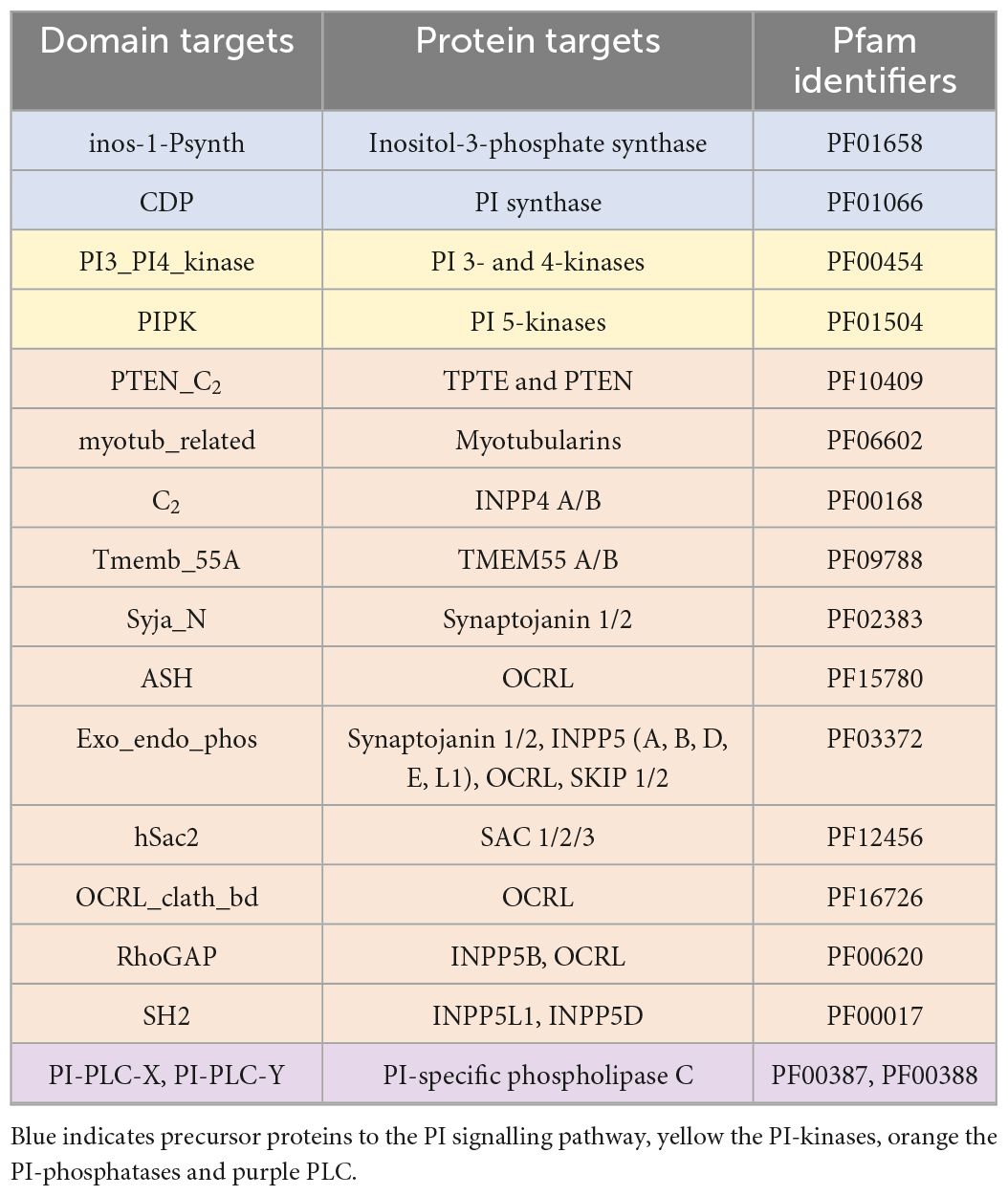
Table 3. The domains targeted to provide complete coverage of the PI pathway, the targeted proteins of these domains, and the Pfam identifiers.
2.3. OrthoFinder analysis
OrthoFinder was used for ortholog assignment of cnidarians and Symbiodiniaceae separately (Emms and Kelly, 2015). Four different analyses were carried out: comparison within the available Symbiodiniaceae datasets; within selected symbiotic cnidarians; within symbiotic and non-symbiotic cnidarians; and within the Symbiodiniaceae plus non-symbiotic unicellular organisms (for symbiotic/non-symbiotic comparison). Protein groups were further delineated by similarity to each other using BLAST, which was especially useful when analysing Symbiodiniaceae datasets, and when attempting to identify differences in the identifiable pathways between symbiotic and non-symbiotic cnidarians/Symbiodiniaceae.
2.4. Bioinformatic analysis of phosphoinositide (PIP)-binding protein functionality
The methods described above were used to identify proteins involved in PIP-binding within datasets of the target species E. diaphana and Breviolum minutum, as well as in datasets of selected species spanning a variety of symbiotic/non-symbiotic symbiont and cnidarian types (Table 4). E. diaphana and B. minutum were utilised due to their common association in nature and the use of them as a model organism (Weis et al., 2008). Previous literature on PIP-binding proteins were used to identify regions of conservation (HMMs) (Cernikova et al., 2019, 2020). Within each of these databases, 14 HMMs were queried. Proteins with at least one of the required PIP-binding domains were included in the gene enrichment analysis (Table 5). The PIP-binding proteins for each species were run through Pannzer2 to ascertain Gene Ontology (GO) terms (Törönen et al., 2018). The “TopGO” R-package (Alexa and Rahnenfuhrer, 2016) was used for the gene enrichment analysis to compare the identified PIP-binding proteins to the entire GO repertoire of biological processes, molecular functions, and cellular compartments within each target species. The GO terms with adjusted p-value < 0.05 were further condensed using Revigo semantic similarity clustering (Supek et al., 2011) (Settings: Small (0.5) similarity, p-value, whole Uniprot database, “SimRel”: a semantic similarity measure). A literature search was then completed to find a link for the significant biological terms to either a mutualistic or parasitic interaction.
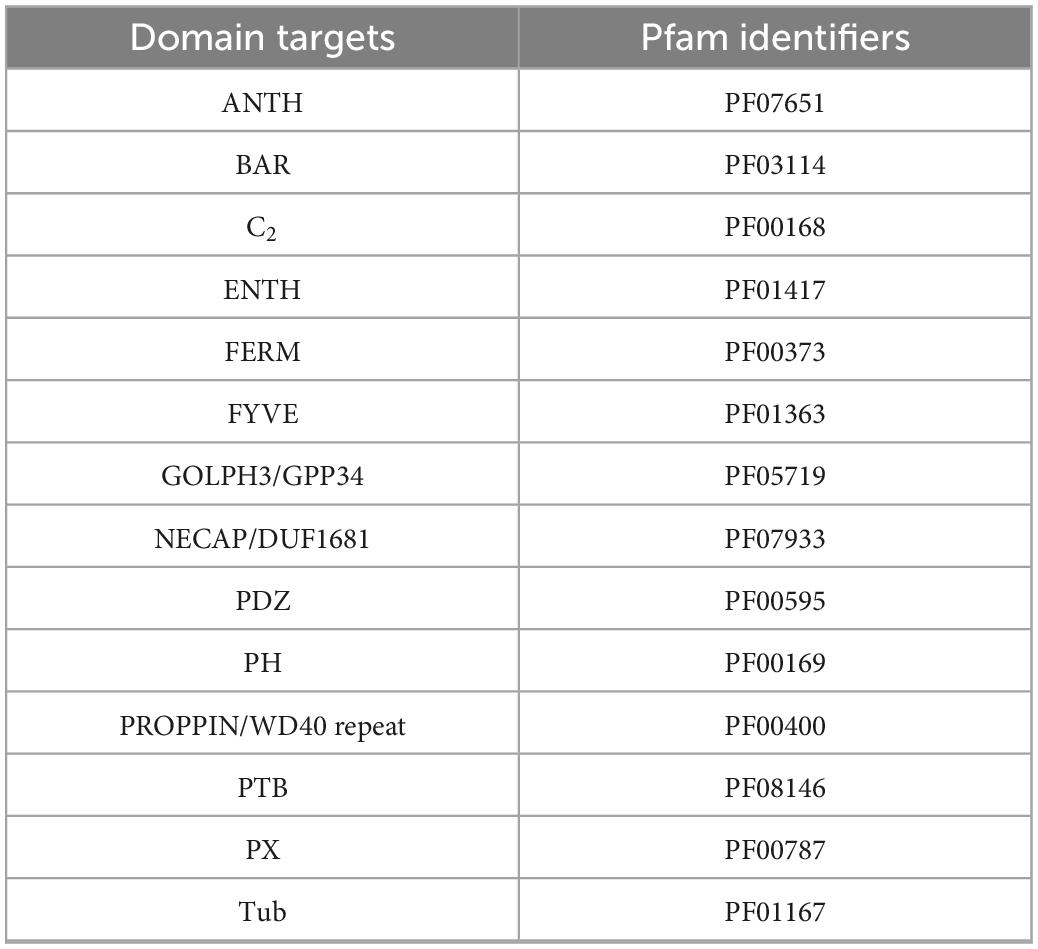
Table 5. The domains targeted to provide complete coverage of the proteins that act upon the PI pathway (PIP-binding proteins).
3. Results and discussion
3.1. The phosphatidylinositol pathway in anthozoan datasets
Across the symbiotic anthozoans analysed there was a complete and conserved PI pathway, analogous to that found in multicellular eukaryotic model organisms (Michell, 2011; Balla, 2013; Figure 2). PI3- and PI4- kinases constitute enzymatic families in higher eukaryotes that are subdivided into multiple classes. Members from each group of the kinases as well as members of the 3- and 4-phosphatases were also identified across the surveyed anthozoans. Phylogenetic trees constructed for the PI3- and PI4-kinases identified each kinase subclass, suggesting the conservation of these kinases within Anthozoa (Supplementary Figure 1). Conversely, 5-phosphatases were not conserved throughout the symbiotic anthozoans, with the loss of INPP5B and SHIP1 in all taxa, while others such as SKIP, PIPP, INPP5E and inositol 1,4,5-trisphosphate 5-phosphatase (INPP5A) were absent from various lineages (Figure 2).
We did not identify any direct evidence of altered regulation of anthozoan host PI-kinases and -phosphatases in response to symbiosis in published datasets. Yet, the mechanism of activation of these enzymes—by phosphorylation—means that more commonly used “omics” technologies (transcriptomics, proteomics) may not identify altered activity in the PI signalling pathway. Interestingly, however, sodium and proton myo-inositol co-transporters are upregulated in symbiotic Aiptasia at the transcriptome (Lehnert et al., 2014) and proteome levels (Sproles et al., 2018b). Myo-inositol is the biologically active precursor to the PI pathway that plays a central role in signal transmission (Figure 1). Metabolomic analysis also found the accumulation of inositol in thermally stressed anemones, indicating that its clearance is important for maintaining the cnidarian-dinoflagellate symbiosis (Hillyer et al., 2016). Furthermore, proteomic analysis identified down-regulation of multiple sugar-inositol transporters, the proton myo-inositol co-transporter (HMIT), the myo-inositol/Na + co-transporter (SMIT1), and the myo-/chiro-inositol/glucose transporter (SMIT2) when the host was inoculated with the heat tolerant, but physiologically sub-optimal (Sproles et al., 2018b) symbiont Durusdinium trenchii (Matthews et al., 2017). Taken together, this suggests that the efficient transport of myo-inositol is essential for the establishment and maintenance of an optimal symbiosis.
3.2. The phosphatidylinositol pathway in Symbiodiniaceae datasets
Analogous to the PI pathway found within Apicomplexa (Wengelnik et al., 2018; Cernikova et al., 2019; Nakada-Tsukui et al., 2019; Cestari and Stuart, 2020), Symbiodiniaceae displayed no genes of the 4-phosphatase group (Figure 3). However, conversely to apicomplexans, Symbiodiniaceae showed a greatly increased diversity both of and within the 5-phosphatases, with multiple 5-phosphatase homologs with dual domain structure, identified within the majority of Symbiodiniaceae species (Supplementary Tables 1, 2). Anthozoan genomic and transcriptomic data showed an average of four and three proteins with the PIPK (used for identification of PIP5K proteins) domain, respectively. However, Symbiodiniaceae genomic and transcriptomic datasets both showed an average of 15 proteins containing the PIPK domain, despite the reduced BUSCO scores. As the lowest BUSCO score for anthozoans was 78%, we used that as a threshold to look at comparative numbers of Symbiodiniaceae PIPK domain-containing proteins, and the average proteins further increased to 21. Of particular note was the presence of a dual domain protein within 15 of the Symbiodiniaceae datasets, with both the PIPK domain and also the PI-PLC domain. Searches of the BLAST database showed no results for similar proteins in other species. The range of PI kinases discovered was diverse, with members from each kinase group identified in all but one Symbiodiniaceae species (Figure 3). The exception was “F. kawagutii #1” —a dataset of Fugacium kawagutii—that only returned a 0.8% complete BUSCO score. However, the lack of functional annotation of proteins from other dinoflagellates makes identification difficult. We relied primarily on sequence homology to the characterised 5-phosphatases (n = 10) and other well-annotated results achieved through NCBI BLAST analysis. Caution should be exercised when examining gene absence in this group because of the incompleteness of some of the datasets used (BUSCO scores found in Figure 3). Despite the sparseness of the available datasets, we were able to identify a completely functional PI pathway across the analysed Symbiodiniaceae species.
Transcriptomic analysis of four species spanning a diversity of four Symbiodiniaceae genera [Symbiodinium (ITS strain A2), Breviolum (ITS strain B2), Cladocopium (ITS strain C1), and Durusdinium (ITS strain D1)] revealed that six cellular pathways are common to all four genera, including the PI signalling pathway and the inositol phosphate metabolism pathway, highlighting the potential global significance of these pathways in Symbiodiniaceae (Rosic et al., 2015). Notable genes encoding phosphatidylinositol 4-phosphate 5-kinases (PIP5K) and phosphatidylinositol 4-kinase (PI4K) were identified across all four Symbiodiniaceae species analysed. PIP4K synthesises PI 4-phosphate (PI4P), which is vital for the regulation of chloroplast division (Bovet et al., 2001; Okazaki et al., 2015). The ubiquity of PIP4K across the Symbiodiniaceae datasets analysed here is therefore unsurprising, with exceptions likely reflecting the quality of available resources. Meanwhile, PIP5K is the main route for the production of phosphatidylinositol 4,5-bisphosphate, and has cellular roles in actin dynamics, endocytosis, exocytosis, and focal adhesion assembly (Tan et al., 2015; Nakada-Tsukui et al., 2019). PIP5K also plays an important role in endosomal trafficking, with the specific isoform PIPKIyi2 being localised to recycling endosomes to facilitate transmembrane protein recycling (Thapa et al., 2012). Exocytosis and endocytosis are important functions in signalling processes within symbiosis (Weis, 2008; Oakley et al., 2016; Rosset et al., 2021) and hence, the down-regulation of this protein when the alga is in culture could reflect an increased demand for vesicle transport after colonisation of a host. Meanwhile PIP4K synthesises PI 4-phosphate (PI4P), which is vital for the regulation of chloroplast division (Bovet et al., 2001; Okazaki et al., 2015). The ubiquity of PIP4K and PIP5K across the Symbiodiniaceae datasets analysed here is therefore unsurprising, with exceptions likely reflecting the quality of available resources.
A transcriptomic study of B. minutum demonstrated a significant down-regulation of PIP5K activity when the alga was in culture compared to in hospite, providing further support for the importance of PIP5K in the functionality of symbiosis (Maor-Landaw et al., 2020). A similar transcriptomic analysis explored the differences between the transcriptome of the thermally tolerant D. trenchii when free-living and in hospite in Aiptasia (Bellantuono et al., 2018). Our targeted analysis of the dataset from Bellantuono et al. (2018) revealed that many of the PI pathway-related genes were significantly differentially expressed between the symbiont when in hospite and in culture (Supplementary Figures 2A, B). Notably there was a large downregulation of PIP5K “2” and a large upregulation of PIP5K “9” and “7,” among other types, when the symbiont was in symbiosis compared to free-living. These observations of up-regulated PIP5K expression in symbiosis could reflect an increased demand for vesicle transport in symbiosis. It is also possible that, as observed in other host-microbe interactions (Baumgartner et al., 2000; Drecktrah et al., 2004; Leirião et al., 2005; Ham et al., 2011; Ebrahimzadeh et al., 2019), Symbiodiniaceae modifies PI-kinase expression as a molecular strategy to manipulate host PIP synthesis to facilitate host membrane dynamics and regulate cellular mechanisms essential for symbiosis persistence.
3.3. Ortholog differences between non-symbiotic and symbiotic groups
The range of proteins within the PI pathway was highly conserved between symbiotic and non-symbiotic cnidarians (Tables 1, 2 and Supplementary Table 3). Both coverage of the pathway and number of proteins within each ortholog were similar, with no significant increases or decreases in gene numbers between cnidarians that associate with symbionts (Supplementary Tables 3, 4).
In contrast, differences were observed between the Symbiodiniaceae and non-symbiotic unicellular algal datasets, including presence/absence of ortholog groups and varying numbers of isoforms/duplicates of individual orthogroups between symbiotic and non-symbiotic datasets. One orthogroup of interest was OG0006447, which was absent in all five of the non-symbiotic species, but present across all except one of the Symbiodiniaceae datasets (“F. kawagutii #1” —previously noted to be largely incomplete based on BUSCO analysis). This orthogroup was analysed further using NCBI BLAST, where it matched consistently and with a high sequence conservation to a phosphatidylinositol 5-phosphate 4-kinase, or PIP5K/PIPK type II protein. This protein has been described across model metazoans but has not yet been described within unicellular organisms; indeed some research has suggested that it is not present in unicellular organisms (Mathre et al., 2019; Raghu, 2021). This protein is responsible for a small proportion of the production of PI 4,5-bisphosphate, however, it is predicted that its primary role is the regulation of PI 5-phosphate (PI5P), which acts as its binding substrate (Rameh et al., 1997; Raghu, 2021). This protein is localised to the plasma membrane, vesicular compartments and nucleus, with roles in PI3K-based regulation of receptor-activated signalling, membrane transport, autophagosome regulation, and the control of PI5P levels in response to cues such as UV irradiation and oxidative stress (Keune et al., 2013; Vicinanza et al., 2015; Wang et al., 2019; Raghu, 2021). Interestingly, the loss of this protein in mouse models leads to the hyperactivation of the innate immune system (Shim et al., 2016). The presence of this protein across all but one of the 28 Symbiodiniaceae datasets in our study is noteworthy, and experimental research to elucidate its role in symbiosis would be valuable.
Another orthogroup (OG0015044) predicted to be a PTEN/TPTE2 3-phosphatase homolog showed a similar pattern. Although there were other orthogroups that showed the presence of PTEN/TPTE2 ubiquitously across symbiotic and non-symbiotic unicellular organisms, this separate orthogroup (OG0015044) indicates an expansion of this protein type within the Symbiodiniaceae datasets. Most of the proteins within this orthogroup showed predominantly PTEN hits when run against the NCBI BLAST database. PTEN is a negative regulator of PI3K and has a variety of roles within cells, including survival, proliferation and regulation of cellular architecture, and it is frequently disrupted in cancer (Song et al., 2012). The presence of an expanded PTEN homolog group is observed in the parasitic protist, Entamoeba histolytica, which has six PTEN homologs, while there is only one present in humans (Nakada-Tsukui et al., 2019). The expansion of the PTEN group within Symbiodiniaceae is indicative of an importance of PTEN functionality, given its role in cell survival and proliferation, and it is therefore another good candidate for future functional studies of the cnidarian-dinoflagellate symbiosis.
A few notable Symbiodiniaceae orthogroups (e.g., OG0012793, OG0014270) were also distinct between Symbiodiniaceae and non-symbiotic unicellular organisms; however, the proteins did not BLAST to any known annotations, or recovered only a few hits, with “unnamed” or “hypothetical protein” responses. When analysed using InterPro scan, these orthogroups contained just one domain, PIPK. However, without targeted experimental work, these protein groups cannot be identified beyond the PIPK group in general.
3.4. Identification and predicted function of putative phosphoinositide-binding proteins in the Aiptasia-Breviolum symbiosis
To further understand the downstream functions of the PI pathway and to unravel the potential role in symbiosis, we expanded the analysis to include enrichment of proteins with the capabilities of binding to members of the PI pathway (termed PIP-binding proteins). All 14 identified domains of interest were found in Aiptasia, whereas four domains were absent in Breviolum (ANTH, FERM, GOLPH3, PTB). Within Aiptasia, 913 PIP-binding proteins were identified, which was 3.12% of the total predicted proteins in the genome, resulting in 306 significantly (p < 0.05) enriched GO terms (Supplementary Table 5). Within Symbiodiniaceae (Breviolum sp. B1), 898 PIP-binding proteins were identified, which was 0.91% of the total predicted proteins in the assembly, resulting in 103 significant GO terms (Supplementary Table 6).
Putative functionalities of the PIP-binding proteins identified in Aiptasia and Breviolum that were predicted to be relevant to symbiosis regulation can be found in Figure 4. Of particular interest, there were seven and six significantly enriched GO terms related to vesicle functioning in Breviolum and Aiptasia, respectively. We hypothesise that cellular processes involving vesicles, such as vesicle-mediated transport, play an important role in the functioning of the cnidarian-dinoflagellate symbiosis.
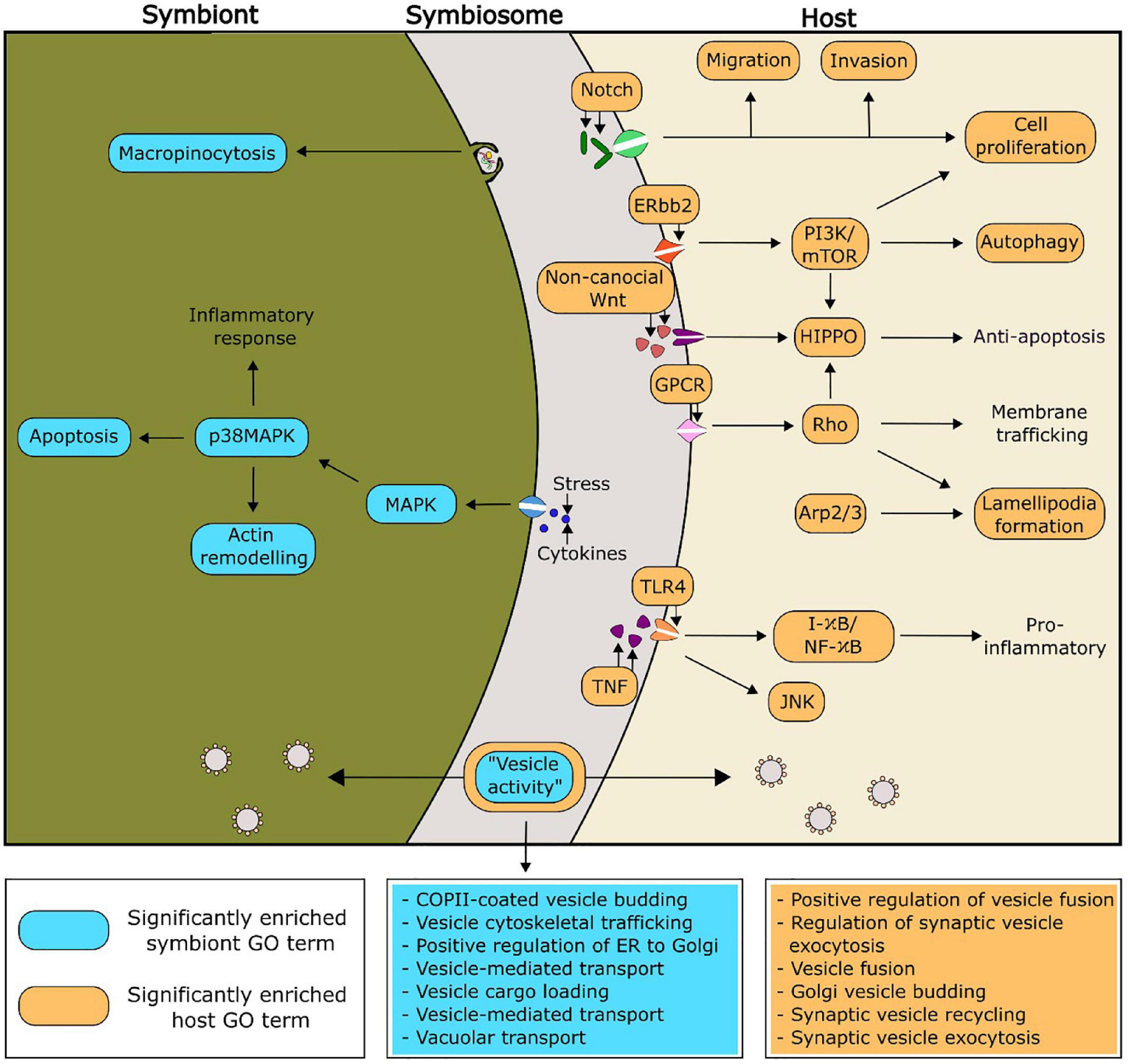
Figure 4. Proposed functionality within the symbiosis of selected significantly enriched functions of the PIP-binding proteins, based on enriched GO functions within the model cnidarian, Aiptasia (orange), and Breviolum minutum (blue). The GO terms related to vesicle activity have been expanded in the two boxes coloured blue and orange, for symbiont and host, respectively.
3.5. Analysis of PIP-binding functionality in additional species of symbiotic anthozoans and Symbiodiniaceae
Enriched GO terms can be found in Supplementary Tables 7–20. The GO term “COPII-coated vesicle budding” was significantly enriched within one of the symbiotic cnidarians (the coral Montipora peltiformis), but more noticeably, all analysed Symbiodiniaceae. One role of COPII vesicles is the transport of proteins from the endoplasmic reticulum, the site of PI synthesis (Agranoff et al., 1958; Sato and Nakano, 2007; Balla, 2013), suggesting an important role of PI in the symbiont. Two GO terms corresponding to immune responses (“innate immune response” and “immune response-activating cell surface receptor”) were found to be significantly enriched across four out of the five Symbiodiniaceae species analysed, whereas these GO terms were not found to be enriched across any of the cnidarians analysed. There is evidence of endosymbiotic Leishmania spp. modulating their host’s immune system through the secretion of exosomes into the host (Silverman and Reiner, 2011). Other research shows Leishmania spp. subverting host immune responses by targeted host pathways such as the PI 3-kinase/Akt signalling cascade to prevent the activation of macrophages (Ruhland et al., 2007; Lambertz et al., 2012). This evidence extends to the cnidarian-dinoflagellate symbiosis, with 16% of differentially expressed genes between symbiotic states being composed predominantly of genes involved in apoptosis and oxidative stress; which are critical functions of immune responses (Kvennefors et al., 2010; Matthews et al., 2017).
Another GO term that regularly appeared as significant is the “positive regulation of TORC1 signalling” in four out of the five analysed Symbiodiniaceae, and “positive regulation of TOR signalling” in three out the five symbiotic cnidarians analysed. TOR (target of rapamycin) signalling activity inhibits autophagy (Juhász et al., 2008), and the presence of significantly enriched GO terms in both the symbiotic cnidarian group and Symbiodiniaceae indicates active inhibition of autophagy across both groups. Transcriptomics supports this finding, with elevated levels of mTORC1 kinase in symbiotic Aiptasia compared to non-symbiotic animals, suggesting a mechanism similar to a feeding response in symbiotic cnidarians (Voss et al., 2019). Up-regulation of TOR-related genes and transcripts during colonisation of plants by mycorrhizae suggests its role in other symbioses (Nanjareddy et al., 2016; Arthikala et al., 2021).
Understanding the implications for the enriched GO terms in the cnidarian-dinoflagellate symbiosis is complex, as just because a function does not appear with a high frequency does not mean its role in the symbiosis is unimportant. Further experimental work, including the inhibition and knock-out of specific genes, will help to elucidate the roles of these highlighted GO terms in the functioning and maintenance of the symbiosis.
4. Conclusion
Our bioinformatic analysis of PI-kinases, PI-phosphatases and PIP-binding proteins is the first to characterise the PI signalling pathway across anthozoans and Symbiodiniaceae. We thereby provide a platform to guide further—more targeted—research. For example, studies will need to apply high-throughput methodologies such as phosphoproteomics and targeted lipidomics to analyse PI pathway activity in cnidarians and Symbiodiniaceae, to ascertain the differences across different experimental treatments, during symbiosis establishment, and across a variety of different cnidarian-dinoflagellate pairings. Such approaches will enable us to begin to unlock the role of the PI signalling pathway in this ecologically important symbiosis. Elucidating how specific molecular processes such as PI signalling regulate the coral-dinoflagellate symbiosis is of high value to identify potential targets to engineer more resilient symbiotic associations [reviewed by Rosset et al., 2021)], a tool that may become necessary in the conservation and restoration of coral reefs.
Data availability statement
The datasets presented in this study can be found in online repositories. The locations and references for the datasets used in this paper are available in Tables 1, 2, 4.
Author contributions
IA, SD, AG, DS, VW, CO, and SR conceptualized the project. IA performed the analysis and wrote the manuscript. SK and LG assisted in data analysis. SD, AG, DS, VW, SR, CO, and SK contributed to the revisions. All authors read and approved the final manuscript.
Funding
Financial support for this research was provided by the Marsden Fund of the Royal Society Te Apārangi (Grant No. 19-VUW-086 to SD, VW, DS, AG, and CO), the Swiss National Science Foundation (Grant No. P400PB_183935 to SR), and Victoria University of Wellington Doctoral Scholarships to IA and LG. Contributions from DS were further supported by an Australian Research Council (ARC) Discovery grant (DP200100091).
Conflict of interest
The authors declare that the research was conducted in the absence of any commercial or financial relationships that could be construed as a potential conflict of interest.
Publisher’s note
All claims expressed in this article are solely those of the authors and do not necessarily represent those of their affiliated organizations, or those of the publisher, the editors and the reviewers. Any product that may be evaluated in this article, or claim that may be made by its manufacturer, is not guaranteed or endorsed by the publisher.
Supplementary material
The Supplementary Material for this article can be found online at: https://www.frontiersin.org/articles/10.3389/fmicb.2022.1094255/full#supplementary-material
Abbreviations
BUSCO, benchmarking universal single copy orthologs; GO, gene ontology; GPI, glycosylphosphatidylinositol; HMMs, hidden Markov models; INPP5A, type I inositol 1,4,5-trisphosphate 5-phosphatase; INPP5B, type II inositol 1,4,5-trisphosphate 5-phosphatase; INPP5E, inositol polyphosphate-5-phosphatase E; IPP, inositol polyphosphate; ITS, internal transcribed spacer; PI, phosphatidylinositol; PI3K, phosphatidylinositol 3-kinase; PI4K, phosphatidylinositol 4-kinase; PI4P, phosphatidylinositol 4-phosphate; PI5P, phosphatidylinositol 5-phosphate; PIP, phosphoinositide; PIP5K, phosphatidylinositol 4-phosphate 5-kinases; PIPP, inositol-polyphosphate 5-phosphatase; SHIP1, SH-2 containing inositol 5′ polyphosphatase 1; SKIP, inositol-polyphosphate 5-phosphatase; TOR, target of rapamycin.
References
Abrego, D., Ulstrup, K. E., Willis, B. L., and Van Oppen, M. J. H. (2008). Species-specific interactions between algal endosymbionts and coral hosts define their bleaching response to heat and light stress. Proc. R. Soc. B Biol. Sci. 275, 2273–2282. doi: 10.1098/rspb.2008.0180
Agranoff, B. W., Bradley, R. M., and Brady, R. O. (1958). The enzymatic synthesis of inositol phosphatide. J. Biol. Chem. 233, 1077–1083. doi: 10.1016/s0021-9258(19)77342-6
Aranda, M., Li, Y., Liew, Y. J., Baumgarten, S., Simakov, O., Wilson, M. C., et al. (2016). Genomes of coral dinoflagellate symbionts highlight evolutionary adaptations conducive to a symbiotic lifestyle. Sci. Rep. 6, 1–15. doi: 10.1038/srep39734
Arriola, M. B., Velmurugan, N., Zhang, Y., Plunkett, M. H., Hondzo, H., and Barney, B. M. (2018). Genome sequences of Chlorella sorokiniana UTEX 1602 and Micractinium conductrix SAG 241.80: Implications to maltose excretion by a green alga. Plant J. 93, 566–586. doi: 10.1111/tpj.13789
Arthikala, M. K., Nanjareddy, K., Blanco, L., Alvarado-Affantranger, X., and Lara, M. (2021). Target of rapamycin, PvTOR, is a key regulator of arbuscule development during mycorrhizal symbiosis in Phaseolus. Sci. Rep. 11, 1–14. doi: 10.1038/s41598-021-90288-2
Balla, T. (2013). Phosphoinositides: Tiny lipids with giant impact on cell regulation. Physiol. Rev. 93, 1019–1137. doi: 10.1152/physrev.00028.2012
Bateman, A., Martin, M. J., Orchard, S., Magrane, M., Agivetova, R., Ahmad, S., et al. (2021). UniProt: The universal protein knowledgebase in 2021. Nucleic Acids Res. 49, D480–D489. doi: 10.1093/nar/gkaa1100
Baumgarten, S., Simakov, O., Esherick, L. Y., Liew, Y. J., Lehnert, E. M., Michell, C. T., et al. (2015). The genome of Aiptasia, a sea anemone model for coral symbiosis. Proc. Natl. Acad. Sci. U.S.A. 112, 11893–11898. doi: 10.1073/pnas.1513318112
Baumgartner, M., Chaussepied, M., Moreau, M. F., Werling, D., Davis, W. C., Garcia, A., et al. (2000). Constitutive P13-K activity is essential for proliferation, but not survival, of Theileria parva-transformed B cells. Cell. Microbiol. 2, 329–339. doi: 10.1046/j.1462-5822.2000.00062.x
Bayer, T., Aranda, M., Sunagawa, S., Yum, L. K., DeSalvo, M. K., Lindquist, E., et al. (2012). Symbiodinium transcriptomes: Genome insights into the dinoflagellate symbionts of reef-building corals. PLoS One 7:e35269. doi: 10.1371/journal.pone.0035269
Bellantuono, A. J., Dougan, K., Granados-Cifuentes, C., and Rodriguez-Lanetty, M. (2018). Transcriptome landscape of a thermal-tolerant coral endosymbiont reveals molecular signatures of symbiosis and dysbiosis. bioRxiv [Preprint]. 1–82. doi: 10.1101/508184
Berkelmans, R., and Van Oppen, M. J. H. (2006). The role of zooxanthellae in the thermal tolerance of corals: A “nugget of hope” for coral reefs in an era of climate change. Proc. R. Soc. B Biol. Sci. 273, 2305–2312. doi: 10.1098/rspb.2006.3567
Bhattacharya, D., Agrawal, S., Aranda, M., Baumgarten, S., Belcaid, M., Drake, J. L., et al. (2016). Comparative genomics explains the evolutionary success of reef-forming corals. Elife 5, 1–26. doi: 10.7554/eLife.13288
Bovet, L., Müller, M. O., and Siegenthaler, P. A. (2001). Three distinct lipid kinase activities are present in spinach chloroplast envelope membranes: Phosphatidylinositol phosphorylation is sensitive to wortmannin and not dependent on chloroplast ATP. Biochem. Biophys. Res. Commun. 289, 269–275. doi: 10.1006/bbrc.2001.5969
Burriesci, M. S., Raab, T. K., and Pringle, J. R. (2012). Evidence that glucose is the major transferred metabolite in dinoflagellate-cnidarian symbiosis. J. Exp. Biol. 215, 3467–3477. doi: 10.1242/jeb.070946
Camp, E. F., Kahlke, T., Signal, B., Oakley, C. A., Lutz, A., Davy, S. K., et al. (2022). Proteome metabolome and transcriptome data for three Symbiodiniaceae under ambient and heat stress conditions. Sci. Data 9, 1–10. doi: 10.1038/s41597-022-01258-w
Cernikova, L., Faso, C., and Hehl, A. B. (2019). Roles of Phosphoinositides and Their binding proteins in parasitic protozoa. Trends Parasitol. 35, 996–1008. doi: 10.1016/j.pt.2019.08.008
Cernikova, L., Faso, C., and Hehl, A. B. (2020). Phosphoinositide-binding proteins mark, shape and functionally modulate highly-diverged endocytic compartments in the parasitic protist Giardia lamblia. PLoS Pathog. 16:e1008317. doi: 10.1371/journal.ppat.1008317
Cestari, I., and Stuart, K. (2020). The phosphoinositide regulatory network in trypanosoma brucei: Implications for cell-wide regulation in eukaryotes. PLoS Negl. Trop. Dis. 14:e0008689. doi: 10.1371/journal.pntd.0008689
Claar, D. C., Starko, S., Tietjen, K. L., Epstein, H. E., Cunning, R., Cobb, K. M., et al. (2020). Dynamic symbioses reveal pathways to coral survival through prolonged heatwaves. Nat. Commun. 11, 1–10. doi: 10.1038/s41467-020-19169-y
Cunning, R., Bay, R. A., Gillette, P., Baker, A. C., and Traylor-Knowles, N. (2018). Comparative analysis of the Pocillopora damicornis genome highlights role of immune system in coral evolution. Sci. Rep. 8, 1–10. doi: 10.1038/s41598-018-34459-8
Di Paolo, G., and De Camilli, P. (2006). Phosphoinositides in cell regulation and membrane dynamics. Nature 443, 651–657. doi: 10.1038/nature05185
Dickson, E. J., and Hille, B. (2019). Understanding phosphoinositides: Rare, dynamic, and essential membrane phospholipids. Biochem. J. 476, 1–23. doi: 10.1042/BCJ20180022
Drecktrah, D., Knodler, L. A., and Steele-Mortimer, O. (2004). Modulation and utilization of host cell phosphoinositides by Salmonella spp. Infect. Immun. 72, 4331–4335. doi: 10.1128/IAI.72.8.4331-4335.2004
Ebrahimzadeh, Z., Mukherjee, A., Crochetière, M., Sergerie, A., Amiar, S., Thompson, L. A., et al. (2019). A pan-apicomplexan phosphoinositide-binding protein acts in malarial microneme exocytosis. EMBO Rep. 20, 1–15. doi: 10.15252/embr.201847102
Emms, D. M., and Kelly, S. (2015). OrthoFinder: Solving fundamental biases in whole genome comparisons dramatically improves orthogroup inference accuracy. Genome Biol. 16, 1–14. doi: 10.1186/s13059-015-0721-2
Frieler, K., Meinshausen, M., Golly, A., Mengel, M., Lebek, K., Donner, S. D., et al. (2013). Limiting global warming to 2C is unlikely to save most coral reefs. Nat. Clim. Chang. 3, 165–170. doi: 10.1038/nclimate1674
Gericke, A., Munson, M., and Ross, A. H. (2006). Regulation of the PTEN phosphatase. Gene 374, 1–9. doi: 10.1016/j.gene.2006.02.024
González-Pech, R. A., Ragan, M. A., and Chan, C. X. (2017). Signatures of adaptation and symbiosis in genomes and transcriptomes of Symbiodinium. Sci. Rep. 7, 1–10. doi: 10.1038/s41598-017-15029-w
Ham, H., Sreelatha, A., and Orth, K. (2011). Manipulation of host membranes by bacterial effectors. Nat. Rev. Microbiol. 9, 635–646. doi: 10.1038/nrmicro2602
Heilmann, I. (2016). Phosphoinositide signaling in plant development. Development 143, 2044–2055. doi: 10.1242/dev.136432
Hillyer, K. E., Tumanov, S., Villas-Bôas, S., and Davy, S. K. (2016). Metabolite profiling of symbiont and host during thermal stress and bleaching in a model cnidarian-dinoflagellate symbiosis. J. Exp. Biol. 219, 516–527. doi: 10.1242/jeb.128660
Hoadley, K. D., Pettay, D. T., Lewis, A., Wham, D., Grasso, C., Smith, R., et al. (2021). Different functional traits among closely related algal symbionts dictate stress endurance for vital Indo-Pacific reef-building corals. Glob. Chang. Biol. 27, 5295–5309. doi: 10.1111/gcb.15799
Howells, E. J., Beltran, V. H., Larsen, N. W., Bay, L. K., Willis, B. L., and Van Oppen, M. J. H. (2012). Coral thermal tolerance shaped by local adaptation of photosymbionts. Nat. Clim. Chang. 2, 116–120. doi: 10.1038/nclimate1330
Hughes, T. P., Kerry, J. T., Álvarez-Noriega, M., Álvarez-Romero, J. G., Anderson, K. D., Baird, A. H., et al. (2017). Global warming and recurrent mass bleaching of corals. Nature 543, 373–377. doi: 10.1038/nature21707
Juhász, G., Hill, J. H., Yan, Y., Sass, M., Baehrecke, E. H., Backer, J. M., et al. (2008). The class III PI(3)K Vps34 promotes autophagy and endocytosis but not TOR signaling in Drosophila. J. Cell Biol. 181, 655–666. doi: 10.1083/jcb.200712051
Keeling, P. J., Burki, F., Wilcox, H. M., Allam, B., Allen, E. E., Amaral-Zettler, L. A., et al. (2014). The marine microbial eukaryote transcriptome sequencing project (MMETSP): Illuminating the functional diversity of eukaryotic life in the oceans through transcriptome sequencing. PLoS Biol. 12:e1001889. doi: 10.1371/journal.pbio.1001889
Keune, W. J., Jones, D. R., and Divecha, N. (2013). PtdIns5P and Pin1 in oxidative stress signaling. Adv. Biol. Regul. 53, 179–189. doi: 10.1016/j.jbior.2013.02.001
Kvennefors, E. C. E., Leggat, W., Kerr, C. C., Ainsworth, T. D., Hoegh-Guldberg, O., and Barnes, A. C. (2010). Analysis of evolutionarily conserved innate immune components in coral links immunity and symbiosis. Dev. Comp. Immunol. 34, 1219–1229. doi: 10.1016/j.dci.2010.06.016
Ladner, J. T., Barshis, D. J., and Palumbi, S. R. (2012). Protein evolution in two co-occurring types of Symbiodinium: An exploration into the genetic basis of thermal tolerance in Symbiodinium clade D. BMC Evol. Biol. 12:217. doi: 10.1186/1471-2148-12-217
LaJeunesse, T. C., Parkinson, J. E., Gabrielson, P. W., Jeong, H. J., Reimer, J. D., Voolstra, C. R., et al. (2018). Systematic Revision of Symbiodiniaceae Highlights the Antiquity and Diversity of Coral Endosymbionts. Curr. Biol. 28, 2570–2580.e6. doi: 10.1016/j.cub.2018.07.008.
LaJeunesse, T. C., Thornhill, D. J., Cox, E. F., Stanton, F. G., Fitt, W. K., and Schmidt, G. W. (2004). High diversity and host specificity observed among symbiotic dinoflagellates in reef coral communities from Hawaii. Coral Reefs 23, 596–603. doi: 10.1007/s00338-004-0428-4
Lambertz, U., Silverman, J. M., Nandan, D., McMaster, W. R., Clos, J., Foster, L. J., et al. (2012). Secreted virulence factors and immune evasion in visceral leishmaniasis. J. Leukoc. Biol. 91, 887–899. doi: 10.1189/jlb.0611326
Lehnert, E. M., Mouchka, M. E., Burriesci, M. S., Gallo, N. D., Schwarz, J. A., and Pringle, J. R. (2014). Extensive differences in gene expression between symbiotic and aposymbiotic cnidarians. G3 (Bethesda) 4, 277–295. doi: 10.1534/g3.113.009084
Leirião, P., Albuquerque, S. S., Corso, S., van Gemert, G. J., Sauerwein, R. W., Rodriguez, A., et al. (2005). HGF/MET signalling protects Plasmodium-infected host cells from apoptosis. Cell. Microbiol. 7, 603–609. doi: 10.1111/j.1462-5822.2004.00490.x
Levin, R. A., Beltran, V. H., Hill, R., Kjelleberg, S., McDougald, D., Steinberg, P. D., et al. (2016). Sex, scavengers, and chaperones: Transcriptome secrets of divergent Symbiodinium thermal tolerances. Mol. Biol. Evol. 33, 2201–2215. doi: 10.1093/molbev/msw119
Li, T., Yu, L., Song, B., Song, Y., Li, L., Lin, X., et al. (2020). Genome improvement and core gene set refinement of Fugacium kawagutii. Microorganisms 8, 1–14. doi: 10.3390/microorganisms8010102
Lin, S., Cheng, S., Song, B., Zhong, X., Lin, X., Li, W., et al. (2015). The Symbiodinium kawagutii genome illuminates dinoflagellate gene expression and coral symbiosis. Science 350, 691–694. doi: 10.1126/science.aad0408
Liu, H., Stephens, T. G., González-Pech, R. A., Beltran, V. H., Lapeyre, B., Bongaerts, P., et al. (2018). Symbiodinium genomes reveal adaptive evolution of functions related to coral-dinoflagellate symbiosis. Commun. Biol. 1:95. doi: 10.1038/s42003-018-0098-3
Lüder, C. G. K., Stanway, R. R., Chaussepied, M., Langsley, G., and Heussler, V. T. (2009). Intracellular survival of apicomplexan parasites and host cell modification. Int. J. Parasitol. 39, 163–173. doi: 10.1016/j.ijpara.2008.09.013
Manni, M., Berkeley, M. R., Seppey, M., Simão, F. A., and Zdobnov, E. M. (2021). BUSCO update: Novel and streamlined workflows along with broader and deeper phylogenetic coverage for scoring of eukaryotic, prokaryotic, and viral genomes. Mol. Biol. Evol. 38, 4647–4654. doi: 10.1093/molbev/msab199
Maor-Landaw, K., van Oppen, M. J. H., and McFadden, G. I. (2020). Symbiotic lifestyle triggers drastic changes in the gene expression of the algal endosymbiont Breviolum minutum (Symbiodiniaceae). Ecol. Evol. 10, 451–466. doi: 10.1002/ece3.5910
Mathre, S., Balasankara Reddy, K., Ramya, V., Krishnan, H., Ghosh, A., and Raghu, P. (2019). Functional analysis of the biochemical activity of mammalian phosphatidylinositol 5 phosphate 4-kinase enzymes. Biosci. Rep. 39, 1–12. doi: 10.1042/BSR20182210
Matthews, J. L., Crowder, C. M., Oakley, C. A., Lutz, A., Roessner, U., Meyer, E., et al. (2017). Optimal nutrient exchange and immune responses operate in partner specificity in the cnidarian-dinoflagellate symbiosis. Proc. Natl. Acad. Sci. U.S.A. 114, 13194–13199. doi: 10.1073/pnas.1710733114
McNamara, C. W., Lee, M. C. S., Lim, C. S., Lim, S. H., Roland, J., Nagle, A., et al. (2013). Targeting Plasmodium PI(4)K to eliminate malaria. Nature 504, 248–253. doi: 10.1038/nature12782
Michell, R. H. (2011). Inositol and its derivatives: Their evolution and functions. Adv. Enzyme Regul. 51, 84–90. doi: 10.1016/j.advenzreg.2010.10.002
Moustafa, A., Evans, A. N., Kulis, D. M., Hackett, J. D., Erdner, D. L., Anderson, D. M., et al. (2010). Transcriptome profiling of a toxic dinoflagellate reveals a gene-rich protist and a potential impact on gene expression due to bacterial presence. PLoS One 5:e9688. doi: 10.1371/journal.pone.0009688
Murray, S., Jørgensen, M. F., Daugbjerg, N., and Rhodes, L. (2004). Amphidinium revisited. II. Resolving species boundaries in the Amphidinium operculatum species complex (Dinophyceae), including the descriptions of Amphidinium trulla sp nov. and Amphidinium gibbosum. Comb. Nov. J. Phycol. 40, 366–382. doi: 10.1046/j.1529-8817.2004.03132.x
Nakada-Tsukui, K., Watanabe, N., Maehama, T., and Nozaki, T. (2019). Phosphatidylinositol kinases and Phosphatases in Entamoeba histolytica. Front. Cell. Infect. Microbiol. 9:150. doi: 10.3389/fcimb.2019.00150
Nanjareddy, K., Blanco, L., Arthikala, M. K., Alvarado-Affantranger, X., Quinto, C., Sánchez, F., et al. (2016). A legume TOR protein kinase regulates Rhizobium symbiosis and is essential for infection and nodule development. Plant Physiol. 172, 2002–2020. doi: 10.1104/pp.16.00844
Nitschke, M. R., Rosset, S. L., Oakley, C. A., Gardner, S. G., Camp, E. F., Suggett, D. J., et al. (2022). The diversity and ecology of Symbiodiniaceae: A traits-based review, 1st Edn. Amsterdam: Elsevier Ltd. doi: 10.1016/bs.amb.2022.07.001
Oakley, C. A., Ameismeier, M. F., Peng, L., Weis, V. M., Grossman, A. R., and Davy, S. K. (2016). Symbiosis induces widespread changes in the proteome of the model cnidarian Aiptasia. Cell. Microbiol. 18, 1009–1023. doi: 10.1111/cmi.12564
Okazaki, K., Miyagishima, S. Y., and Wada, H. (2015). Phosphatidylinositol 4-phosphate negatively regulates chloroplast division in arabidopsis. Plant Cell 27, 663–674. doi: 10.1105/tpc.115.136234
Oliver, T. A., and Palumbi, S. R. (2011). Many corals host thermally resistant symbionts in high-temperature habitat. Coral Reefs 30, 241–250. doi: 10.1007/s00338-010-0696-0
Parkinson, J. E., Baumgarten, S., Michell, C. T., Baums, I. B., LaJeunesse, T. C., and Voolstra, C. R. (2016). Gene expression variation resolves species and individual strains among coral-associated dinoflagellates within the genus Symbiodinium. Genome Biol. Evol. 8, 665–680. doi: 10.1093/gbe/evw019
Plattner, F., and Soldati-Favre, D. (2008). Hijacking of host cellular functions by the Apicomplexa. Annu. Rev. Microbiol. 62, 471–487. doi: 10.1146/annurev.micro.62.081307.162802
Rädecker, N., Pogoreutz, C., Voolstra, C. R., Wiedenmann, J., and Wild, C. (2015). Nitrogen cycling in corals: The key to understanding holobiont functioning? Trends Microbiol. 23, 490–497. doi: 10.1016/j.tim.2015.03.008
Raghu, P. (2021). Emerging cell biological functions of phosphatidylinositol 5 phosphate 4 kinase. Curr. Opin. Cell Biol. 71, 15–20. doi: 10.1016/j.ceb.2021.01.012
Rameh, L. E., Tolias, K. F., Duckworth, B. C., and Cantley, L. C. (1997). A new pathway for synthesis of phosphatidylinositol-4,5-bisphosphate. Nature 390, 192–196. doi: 10.1038/36621
Rosic, N., Ling, E. Y. S., Chan, C. K. K., Lee, H. C., Kaniewska, P., Edwards, D., et al. (2015). Unfolding the secrets of coral-Algal symbiosis. ISME J. 9, 844–856. doi: 10.1038/ismej.2014.182
Rosset, S. L., Oakley, C. A., Ferrier-Pagès, C., Suggett, D. J., Weis, V. M., and Davy, S. K. (2021). The molecular language of the cnidarian–dinoflagellate symbiosis. Trends Microbiol. 29, 320–333. doi: 10.1016/j.tim.2020.08.005
Ruhland, A., Leal, N., and Kima, P. E. (2007). Leishmania promastigotes activate PI3K/Akt signalling to confer host cell resistance to apoptosis. Cell. Microbiol. 9, 84–96. doi: 10.1111/j.1462-5822.2006.00769.x
Sampayo, E. M., Ridgway, T., Bongaerts, P., and Hoegh-Guldberg, O. (2008). Bleaching susceptibility and mortality of corals are determined by fine-scale differences in symbiont type. Proc. Natl. Acad. Sci. U.S.A. 105, 10444–10449. doi: 10.1073/pnas.0708049105
Sato, K., and Nakano, A. (2007). Mechanisms of COPII vesicle formation and protein sorting. FEBS Lett. 581, 2076–2082. doi: 10.1016/j.febslet.2007.01.091
Shim, H., Wu, C., Ramsamooj, S., Bosch, K. N., Chen, Z., Emerling, B. M., et al. (2016). Deletion of the gene Pip4k2c, a novel phosphatidylinositol kinase, results in hyperactivation of the immune system. Proc. Natl. Acad. Sci. U.S.A. 113, 7596–7601. doi: 10.1073/pnas.1600934113
Shoguchi, E., Beedessee, G., Tada, I., Hisata, K., Kawashima, T., Takeuchi, T., et al. (2018). Two divergent Symbiodinium genomes reveal conservation of a gene cluster for sunscreen biosynthesis and recently lost genes. BMC Genomics 19:458. doi: 10.1186/s12864-018-4857-9
Shoguchi, E., Shinzato, C., Kawashima, T., Gyoja, F., Mungpakdee, S., Koyanagi, R., et al. (2013). Draft assembly of the Symbiodinium minutum nuclear genome reveals dinoflagellate gene structure. Curr. Biol. 23, 1399–1408. doi: 10.1016/j.cub.2013.05.062
Silverman, J. M., and Reiner, N. E. (2011). Leishmania exosomes deliver preemptive strikes to create an environment permissive for early infection. Front. Cell. Infect. Microbiol. 1:26. doi: 10.3389/fcimb.2011.00026
Song, M. S., Salmena, L., and Pandolfi, P. P. (2012). The functions and regulation of the PTEN tumour suppressor. Nat. Rev. Mol. Cell Biol. 13, 283–296. doi: 10.1038/nrm3330
Sproles, A. E., Oakley, C. A., Matthews, J. L., Peng, L., Owen, J. G., Grossman, A. R., et al. (2018b). Proteomics quantifies protein expression changes in a model cnidarian colonised by a thermally tolerant but suboptimal symbiont. ISME J. 13, 2334–2345. doi: 10.1038/s41396-019-0437-5
Sproles, A. E., Kirk, N. L., Kitchen, S. A., Oakley, C. A., Grossman, A. R., Weis, V. M., et al. (2018a). Phylogenetic characterization of transporter proteins in the cnidarian-dinoflagellate symbiosis. Mol. Phylogenet. Evol. 120, 307–320. doi: 10.1016/j.ympev.2017.12.007
Supek, F., Bošnjak, M., Škunca, N., and Šmuc, T. (2011). Revigo summarizes and visualizes long lists of gene ontology terms. PLoS One 6:e21800. doi: 10.1371/journal.pone.0021800
Tan, X., Thapa, N., Choi, S., and Anderson, R. A. (2015). Emerging roles of PtdIns(4,5)P2 - beyond the plasma membrane. J. Cell Sci. 128, 4047–4056. doi: 10.1242/jcs.175208
Tanaka, Y., Miyajima, T., Koike, I., Hayashibara, T., and Ogawa, H. (2006). Translocation and conservation of organic nitrogen within the coral-zooxanthella symbiotic system of Acropora pulchra, as demonstrated by dual isotope-labeling techniques. J. Exp. Mar. Bio. Ecol. 336, 110–119. doi: 10.1016/j.jembe.2006.04.011
Thapa, N., Sun, Y., Schramp, M., Choi, S., Ling, K., and Anderson, R. A. (2012). Phosphoinositide signaling regulates the exocyst complex and polarized integrin trafficking in directionally migrating cells. Dev. Cell 22, 116–130. doi: 10.1016/j.devcel.2011.10.030
Törönen, P., Medlar, A., and Holm, L. (2018). PANNZER2: A rapid functional annotation web server. Nucleic Acids Res. 46, W84–W88. doi: 10.1093/nar/gky350
Underhill, D. M., and Ozinsky, A. (2002). Phagocytosis of microbes: Complexity in action. Annu. Rev. Immunol. 20, 825–852. doi: 10.1146/annurev.immunol.20.103001.114744
Vicinanza, M., Korolchuk, V. I., Ashkenazi, A., Puri, C., Menzies, F. M., Clarke, J. H., et al. (2015). PI(5)P regulates autophagosome biogenesis. Mol. Cell 57, 219–234. doi: 10.1016/j.molcel.2014.12.007
Voolstra, C. R., Miller, D. J., Ragan, M. A., Hoffmann, A. A., Hoegh-Guldberg, O., Bourne, D. G., et al. (2015). The ReFuGe 2020 Consortium-using “omics” approaches to explore the adaptability and resilience of coral holobionts to environmental change. Front. Mar. Sci. 2:68. doi: 10.3389/fmars.2015.00068
Voss, P., Gornik, S., Jacobovitz, M., Rupp, S., Dörr, M., Maegele, I., et al. (2019). Nutrient-dependent mTORC1 signaling in coral-algal symbiosis. bioRxiv [Preprint]. 1–24. doi: 10.1101/723312
Wang, D. G., Paddock, M. N., Lundquist, M. R., Sun, J. Y., Mashadova, O., Amadiume, S., et al. (2019). PIP4Ks suppress insulin signaling through a catalytic-independent mechanism. Cell Rep. 27, 1991–2001.e5. doi: 10.1016/j.celrep.2019.04.070
Weis, V. M. (2008). Cellular mechanisms of cnidarian bleaching: Stress causes the collapse of symbiosis. J. Exp. Biol. 211, 3059–3066. doi: 10.1242/jeb.009597
Weis, V. M., Davy, S. K., Hoegh-Guldberg, O., Rodriguez-Lanetty, M., and Pringle, J. R. (2008). Cell biology in model systems as the key to understanding corals. Trends Ecol. Evol. 23, 369–376. doi: 10.1016/j.tree.2008.03.004
Wengelnik, K., Daher, W., and Lebrun, M. (2018). Phosphoinositides and their functions in apicomplexan parasites. Int. J. Parasitol. 48, 493–504. doi: 10.1016/j.ijpara.2018.01.009
Weston, A. J., Dunlap, W. C., Beltran, V. H., Starcevic, A., Hranueli, D., Ward, M., et al. (2015). Proteomics links the redox state to calcium signaling during bleaching of the scleractinian coral acropora microphthalma on exposure to high solar irradiance and thermal stress. Mol. Cell. Proteomics 14, 585–595. doi: 10.1074/mcp.M114.043125
Wilding, C. S., Fletcher, N., Smith, E. K., Prentis, P., Weedall, G. D., and Stewart, Z. (2020). The genome of the sea anemone Actinia equina (L.): Meiotic toolkit genes and the question of sexual reproduction. Mar. Genomics 53:100753. doi: 10.1016/j.margen.2020.100753
Wilkinson, S. (2018). The “aphid” package for analysis with profile hidden Markov models. R package version 1.1.0.
Xiang, T., Nelson, W., Rodriguez, J., Tolleter, D., and Grossman, A. R. (2015). Symbiodinium transcriptome and global responses of cells to immediate changes in light intensity when grown under autotrophic or mixotrophic conditions. Plant J. 82, 67–80. doi: 10.1111/tpj.12789
Keywords: phosphatidylinositol, symbiosis, coral, inositol, Symbiodiniaceae, bioinformatics, reef, signalling
Citation: Ashley IA, Kitchen SA, Gorman LM, Grossman AR, Oakley CA, Suggett DJ, Weis VM, Rosset SL and Davy SK (2023) Genomic conservation and putative downstream functionality of the phosphatidylinositol signalling pathway in the cnidarian-dinoflagellate symbiosis. Front. Microbiol. 13:1094255. doi: 10.3389/fmicb.2022.1094255
Received: 09 November 2022; Accepted: 28 December 2022;
Published: 26 January 2023.
Edited by:
Crisalejandra Rivera-Perez, Centro de Investigación Biológica del Noroeste (CIBNOR), MexicoReviewed by:
Cesar Cardona-Felix, Instituto Politécnico Nacional (IPN), MexicoMaria-Cristina Lopez-Mendez, Misantla Higher Technological Institute (ITSM), Mexico
Betzabe Lopez, University of Sonora, Mexico
Copyright © 2023 Ashley, Kitchen, Gorman, Grossman, Oakley, Suggett, Weis, Rosset and Davy. This is an open-access article distributed under the terms of the Creative Commons Attribution License (CC BY). The use, distribution or reproduction in other forums is permitted, provided the original author(s) and the copyright owner(s) are credited and that the original publication in this journal is cited, in accordance with accepted academic practice. No use, distribution or reproduction is permitted which does not comply with these terms.
*Correspondence: Simon K. Davy, c2ltb24uZGF2eUB2dXcuYWMubno=