- 1School of Environmental Science and Engineering, Zhejiang Gongshang University, Hangzhou, Zhejiang, China
- 2International Science and Technology Cooperation Platform for Low-Carbon Recycling of Waste and Green Development, Zhejiang Gongshang University, Hangzhou, Zhejiang, China
- 3Department of Applied Chemistry, College of Chemistry and Chemical Engineering, Chongqing University of Science and Technology, Chongqing, China
- 4Faculty of Bioengineering, Ghent University, Ghent, Belgium
Remediation of environmental toxic pollutants has attracted extensive attention in recent years. Microbial bioremediation has been an important technology for removing toxic pollutants. However, microbial activity is also susceptible to toxicity stress in the process of intracellular detoxification, which significantly reduces microbial activity. Electroactive microorganisms (EAMs) can detoxify toxic pollutants extracellularly to a certain extent, which is related to their unique extracellular electron transfer (EET) function. In this review, the extracellular and intracellular aspects of the EAMs’ detoxification mechanisms are explored separately. Additionally, various strategies for enhancing the effect of extracellular detoxification are discussed. Finally, future research directions are proposed based on the bottlenecks encountered in the current studies. This review can contribute to the development of toxic pollutants remediation technologies based on EAMs, and provide theoretical and technical support for future practical engineering applications.
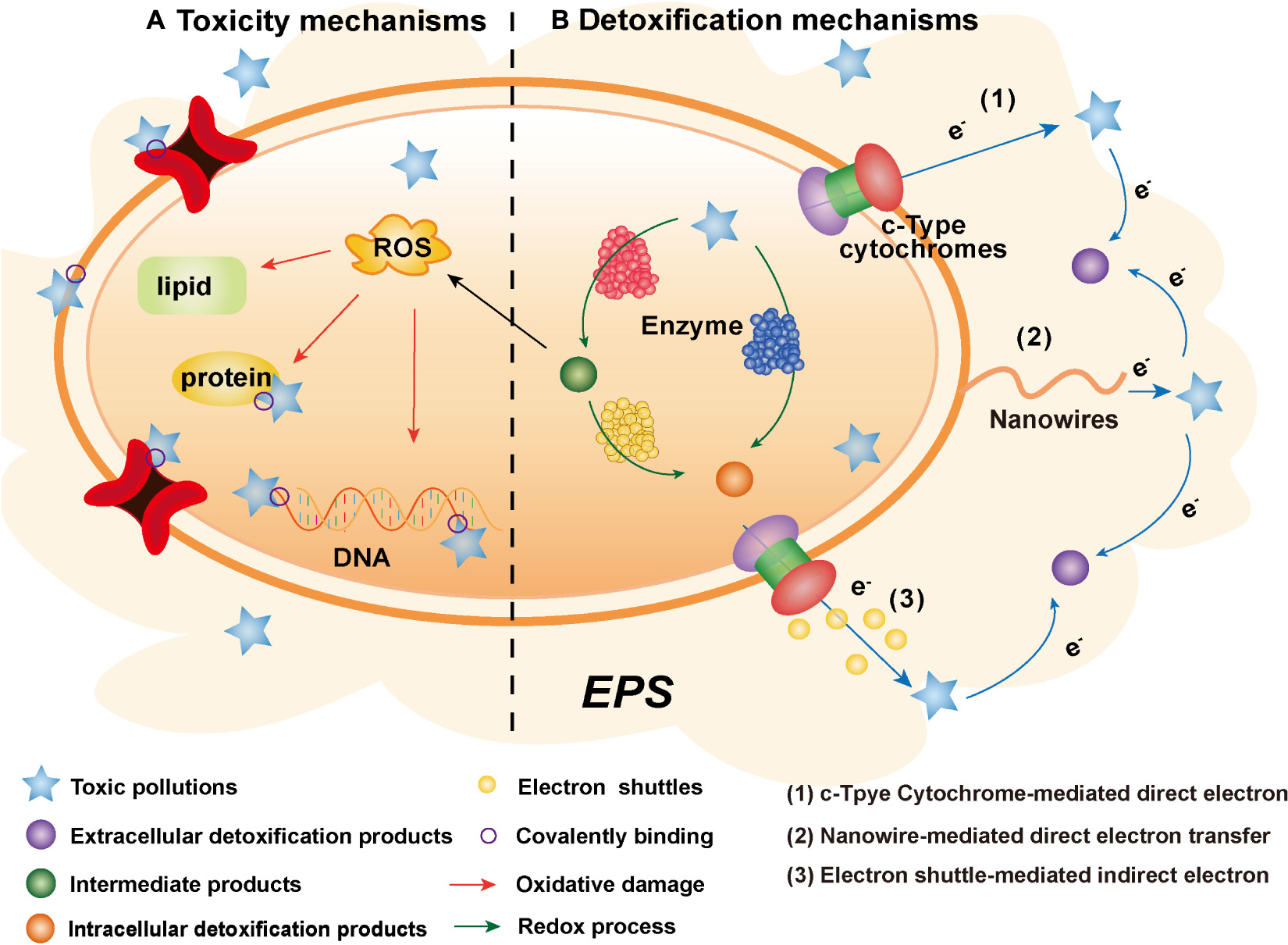
Graphical Abstract. The toxicogenic mechanisms of toxic pollutants and the detoxification mechanisms of electroactive microorganisms.
Introduction
Toxic pollutants can be enriched in living organisms through a series of migration and transformation, resulting in carcinogenic, teratogenic, and mutagenic effect. The sources of toxic pollutants are diverse and ubiquitous in the environment (Ma et al., 2016; Zhang et al., 2016; Sun et al., 2022b). Treating toxic pollutants efficiently has always been a focus and a difficult task in the field of environmental remediation.
Microbial remediation is the most commonly used technology to treat toxic pollutants, but toxicity stress causes a bottleneck in the efficient and stable microbial remediation. Microorganisms generally transfer toxic pollutants to cells for metabolism, achieving detoxification by degrading the pollutants. However, degradation and detoxification are sometimes not completely synchronous. Liu et al. (1998) found that although pyridine was degraded by microorganisms, the microbial toxicity increased with the formation of the intermediate methylpyridine in this process, thus inhibiting the activity of microorganisms and even leading to system collapse.
It has been reported that electroactive microorganisms (EAMs) show better activity than that of other microorganisms under toxicity stress (Zheng et al., 2020; Chaudhary et al., 2022). Desulfovibrio, an electroactive sulfate-reducing bacteria, became the dominating bacteria at the genus level and accounted for 32% of the relative abundance after 150 days under the toxicity stress of Cr(VI), despite the fact that it was scarce in the initial inoculum (Qian et al., 2022). The most likely explanation for this phenomenon is that EAMs have a unique metabolic pathway that can perform extracellular electron transfer (EET) with redox active substances to complete a series of metabolic activities (Li and Yu, 2015; Logan et al., 2019). However, the correspondence between the extracellular metabolism and the detoxification ability of EAMs and the related mechanisms still need to be clarified further.
The toxic pollutants remediation technology based on EAMs has the advantages of environmental friendliness and low cost, considered to be the most green and sustainable new environmental pollution treatment method at present (Li and Yu, 2015; Xu et al., 2019). Despite various reviews on the EET mechanism of EAMs (Xiao and Yu, 2020; Xie et al., 2020; Thapa et al., 2022), there haven’t many relevant summaries that are pertinent to the detoxification mechanism of EAMs under pollutants’ toxicity stress. This review summarizes the toxicogenic mechanisms of toxic pollutants to microorganisms, the detoxification mechanisms of EAMs to these pollutants and several strategies to strengthen extracellular detoxification, as well as prospects for possible future research directions based on conclusions regarding the problems encountered in current research.
Toxicogenic mechanisms of toxic pollutants to microorganisms
There are two main toxicogenic mechanisms of toxic pollutants to microorganisms, including the following: on the one hand, the electrophilic groups of toxic pollutants can directly covalently bond with the nucleophilic groups of biological macromolecules (Dudev and Lim, 2014); on the other hand, numerous reactive oxygen species (ROS), which are produced in the oxidation-reduction process of toxic pollutants, oxidize biological macromolecules (Figure 1; Guo et al., 2021). The essence of these two mechanisms is that toxic pollutants with strong electrophilicity directly or indirectly react with biological macromolecules to destroy the structure and function of cells, finally leading to apoptosis (Roy et al., 2005).
Covalent binding to biological macromolecules
In microbial cells, the electrophilic groups of toxic pollutants or their metabolites can covalently bind with nucleophilic groups in biological macromolecules, thus changing the structure and function of biological macromolecules, such as DNA and proteins, and causing a series of harmful biological effects (Jiang et al., 2022).
Because its bases contain many nucleophilic sites, DNA can covalently bind with electrophilic toxic pollutants or their metabolites to form stable complexes (Shibata and Uchida, 2019). For example, heavy metals can covalently bind with the nitrogen atoms located on DNA bases (Tanaka and Ono, 2008); the intermediate product (high-activity electrophilic diol epoxide), which is produced in the intracellular metabolic process of polycyclic aromatic hydrocarbons in microbes, can covalently bind with deoxyguanosine (Vondracek and Machala, 2021). There are two ways to cross-link DNA by toxic pollutants, including intrachain interactions and interchain interactions, and the formation of complexes causes DNA double strands to unwind and bend (Park et al., 2005).
Because of its nucleophilic groups (e.g., amino, sulfhydryl, and carboxyl), proteins can also be covalently modified, resulting in various harmful biological effects (Wells and Winn, 1996). For example, acetaldehyde can covalently bind with various proteins (e.g., albumin, erythrocyte protein, tubulin, lipoprotein, and cytochrome enzymes involved in ethanol metabolism) to form complexes, thus interfering with the physiological functions of proteins and stimulating the immune response of cells (Israel et al., 1986; Smith et al., 1989; Wehr et al., 1993). Moreover, Mauch et al. (1986) proved that the free lysine e-amino group is an important target of proteins that covalently bind with acetaldehyde. In addition, heavy metals can bind with thiol groups that are located on proteins and damage protein folding or the combination of cofactors and enzymes, thus destroying the normal biological activity of proteins (Sharma and Melkania, 2018).
Accumulation of reactive oxygen species
Reactive oxygen species are oxygen derivatives, such as superoxide anions, hydroxyl radicals, and hydrogen peroxide, which are produced by enzymatic reactions during the normal metabolic activities of microorganisms (Sun et al., 2022a). Under normal physiological conditions, the content of intracellular ROS in microorganisms is dynamically balanced, and a small amount of ROS can be removed by the antioxidant system. However, due to the limited scavenging capacity of antioxidant systems, excessive ROS may damage the activities and functions of various antioxidant-related enzymes (Ballard and Towarnicki, 2020; Sies and Jones, 2020; Sun et al., 2022a). The intake of toxic pollutants (e.g., polycyclic aromatic hydrocarbons and heavy metal ions) can promote the production of ROS (Gao et al., 2019; Vondracek and Machala, 2021). Excessive ROS mainly act on lipids, proteins and DNA in cells, causing oxidative damage and finally affecting the normal physiological and metabolic activities of microorganisms (Tekpli et al., 2011).
When a large amount of ROS accumulates in cells, the rich lipids in the cell membrane are greatly damaged. The peroxidation of lipids may change the physiological characteristics of the membrane and disturb the lipid asymmetry of the membrane, thus changing the fluidity of the membrane. As a result, membrane depolarization and an eventual loss of integrity occurs (Tekpli et al., 2011). With a microbial electron microscope, Morcillo et al. (2016) observed that the structure of the microbiological cell membrane was obviously damaged after exposure to toxic heavy metals. However, damage to the membrane structure not only affects the process of microbiological substrate intake but also promotes the accumulation of toxic pollutants in cells, thus aggravating the toxicity effects on microorganisms (Chen et al., 2020b).
Reactive oxygen species undergo oxidation reactions with intracellular structural and functional proteins, and these reactions include the removal of carboxyl groups, formation of carbonyl groups, and oxidation of sulfhydryl groups (Wells et al., 1997). For example, iron will react with hydrogen peroxide to generate more active ⋅OH (Fenton reaction), leading to the oxidation of amino acids such as histidine, tyrosine, L-cysteine (Yan et al., 2021). The oxidative damage caused by these reactions leads to the crosslinking of proteins, changes in amino acid composition and protein structure, which will change or destroy the function of the protein (Davies et al., 1991).
Reactive oxygen species can also directly destroy the double-stranded structure of DNA through the shear action of oxidation reactions (Radzig et al., 2013). Thiebault et al. (2007) found that a high concentration of U could induce the production of ROS and cause irreversible damage to DNA. Not all ROS cause oxidative damage in the same way, ⋅OH can directly react with DNA, while other less active ROS is through a series of reactions into a higher active ROS such as ⋅OH and ⋅ONOO− playing an oxidizing role. For example, can’t directly lead to DNA damage, but can react with NO to generate ONOOH, resulting in oxidative damage to DNA (van der Vliet et al., 1995).
Detoxification mechanisms of electroactive microorganisms
Since contact with the cell membrane and entry into cells is a prerequisite for the pollutants’ toxicity effects on EAMs, microorganisms generally produce a series of responses in the cell to achieve detoxification under toxicity stress. However, due to the limited capacity of this intracellular detoxification, it is difficult for microorganisms to maintain good activity for a long time under toxic stress. Recent studies have reported that EAMs perform better activity than normal microorganisms under toxicity stress (Zheng et al., 2020; Chaudhary et al., 2022). Unlike normal microorganisms, EAMs can transfer electrons to the extracellular space through their unique EET pathway (Chaudhary et al., 2022). At the same time, toxic pollutants with strong electrophilicity can be used as the final electron acceptors in the process of extracellular respiration due to their good ability to accept electrons (Roy et al., 2005). Therefore, in addition to intracellular detoxification, EAMs can also detoxify toxic pollutants through the EET pathway.
Intracellular detoxification
Under suitable environmental conditions, EAMs can detoxify toxic pollutants by their intracellular metabolic activity, similar to other microorganisms. The intracellular metabolic activity of EAMs also depends on the intracellular electron transfer (IET) pathway, which is mainly composed of three parts, namely, various functional enzymes, quinone pools (e.g., menadione or ubiquinone) and c-type cytochromes (cyt-cs) (Chen and Strous, 2013). Among them, quinone pools can mediate electron transfer between the cytoplasm and periplasm; cyt-cs can play a role in electron transfer among various enzymes (Yang et al., 2020).
Enzymes play an important role in the IET pathway, and several studies have proven that they mediate nearly all reactions in organisms (Zumstein and Helbling, 2019). In the process of detoxification of toxic pollutants by EAMs, a complete IET pathway often contains a variety of enzymes, such as monooxygenase, dioxygenase, and dehydrogenase, which may be needed in the detoxification of complex organics such as polycyclic aromatic hydrocarbons (PAHs) by EAMs (Ismail et al., 2022). The O2 content in the environment affects the detoxification of toxic pollutants by EAMs (Alegbeleye et al., 2017). The first step in the detoxification of PAHs by EAMs (e.g., Pseudomonas aeruginosa and Ochrobactrum anthropi) under aerobic conditions is to reduce the number of benzene rings, which occurs in two ways: through monooxygenase and dioxygenase (Logan et al., 2019; Ismail et al., 2022). Monooxygenase combines an oxygen atom into PAHs to form oxidized aromatic hydrocarbons, and then hydrates with epoxidase to form trans-dihydrodiol or spontaneously isomerizes to form phenols (Fuchs et al., 2011). Dioxygenase decomposes the aromatic ring to form cis-dihydrodiol, which is then transferred to NAD+ by dehydrogenase while the important intermediate product catechol is metabolized (Alegbeleye et al., 2017). Finally, the hydrolase opens the ring of catechol in the ortho position to form cis, cis-muconic acid or opens the ring by ring transformation to form 2-hydroxymuconic semialdehyde (Seo et al., 2009). The detoxification of PAHs by microorganisms can also occurs under anaerobic conditions through using inorganic salts, such as nitrate and sulfate, and metal ions, such as trivalent iron and high-valent manganese, as electron acceptors for respiration to oxidize PAHs to low molecular weight substances (Nzila, 2018). Similarly, EAMs can detoxify Cr(VI) intracellularly under aerobic and anaerobic conditions. Under aerobic conditions, reductants such as Cr(VI) reductase, dimer glycoprotein, and n-ethylmaleimide reductase can mediate the reduction reaction of Cr(VI). Under anaerobic conditions, water-soluble and fat-soluble reductase such as cytochrome enzyme, hydrogenase, and flavin reductase mediate the reduction of Cr(VI) (Thatoi et al., 2014; He et al., 2020).
Although intracellular detoxification can transform toxic pollutants into low-toxicity substances, sometimes the toxicity effects on EAMs are enhanced in the process. Formation of toxic intermediates (Table 1) and ROS is the reason for increased toxicity. In addition, due to the limited intracellular detoxification, there are still some toxic pollutions can directly cause toxicity effects on EAMs (Rathore et al., 2022). At the same time, the toxicity of these pollutions can attack enzymes as a class of proteins (Feng et al., 2015). Therefore, the intracellular detoxification effect of EAMs can be inhibited under toxic stress. Chang et al. (2016) reported that two homologous strains, Trichoderma asperellum PTN7 and PTN10, exhibited different tolerance and detoxification capacity under the toxicity stress of Cr(VI). This is mainly related to the different reduction capacities of the two strains to extracellular crude enzyme. Due to its superior extracellular reduction ability, PTN10 can alleviate the process of DNA and protein damage caused by ROS produced during the intracellular reduction of Cr(VI), thus showing better toxicity tolerance and biological activity than PTN7 (Xia et al., 2021).
In conclusion, the intracellular metabolism of EAMs can detoxify toxic pollutants, but compared to extracellular detoxification, this method is more negative. Because contact with the cell membrane and entry into cells is a prerequisite for the toxicity effects of toxic pollutants on EAMs, intracellular detoxification cannot fundamentally play a detoxification role, which explains why normal microorganisms are unable to maintain long-term good activity in the environment under toxic stress.
Extracellular detoxification
The extracellular detoxification mechanism of EAMs is mainly to reduce the electrophilicity of toxic pollutants by extracellular reduction, thereby reducing the pollutants’ toxicity effects (Chen et al., 2019; Cui et al., 2021). Nitroaromatic hydrocarbons are common toxic organic pollutants. Due to the strong electrophilicity of the nitro groups located on benzene rings, nitroaromatic hydrocarbons are not easily oxidized by microorganisms but are easily reduced (Tas and Pavlostathis, 2014). It has been reported that EAMs can achieve the extracellular reduction of nitroaromatic hydrocarbons through the EET pathway. For example, Geobacter metallireducens and Shewanella putrefaciens CN32 can transfer the electrons obtained by the oxidation of substrates to nitroaromatic hydrocarbons through extracellular iron oxides, thus promoting the reduction of nitro groups to produce amino aromatic hydrocarbons with low toxicity (Tobler et al., 2007; Luan et al., 2015). In addition, the extracellular reduction of EAMs can also act on toxic inorganic pollutants, such as Cr(VI) (Xia et al., 2021). Because Cr(VI) is reduced to Cr(III) with low electrophilicity through extracellular reduction, its toxicity to EAMs decreased to 1/100 of the original level (Chang et al., 2016). Therefore, the extracellular reduction of toxic pollutants by EAMs is an effective detoxification mechanism (Table 2).
Electroactive microorganisms, as a complex with strong catalysis, promote the extracellular redox process of toxic pollutants to achieve detoxification (Xie et al., 2020). The EET pathway is the key to the extracellular detoxification ability of EAMs, which is composed of a series of redox processes (Figure 2). And the EET pathways of EAMs are mainly composed of cyt-cs, nanowires and small molecule electroactive substances (Zhao et al., 2021).
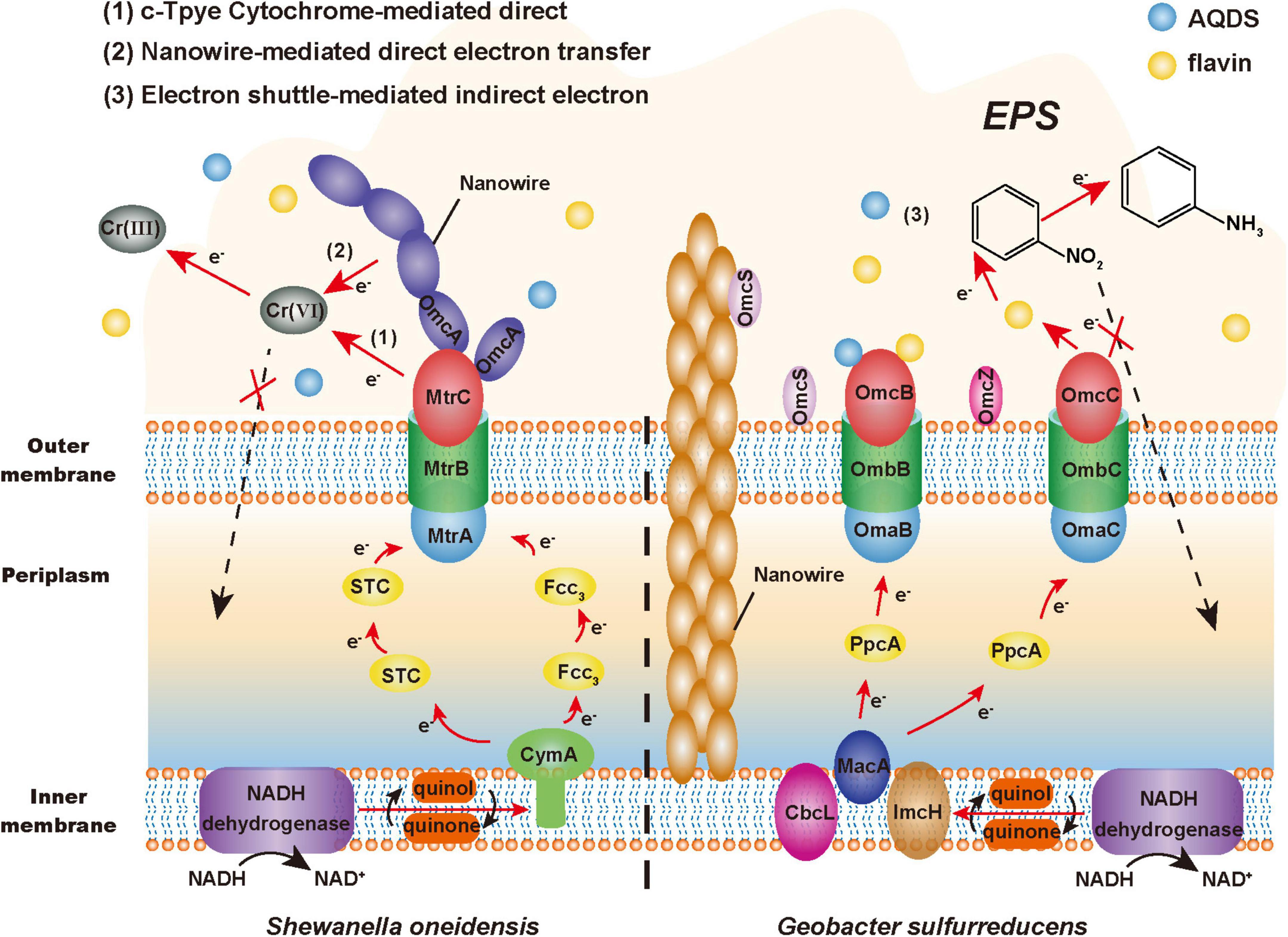
Figure 2. Extracellular detoxification mechanism of electroactive microorganisms (EAMs). The red arrow represents the electron transfer pathway, the black arrow represents the material transformation process, and the dashed arrow represents the non-occurring toxicity effects.
A large number of studies have found that a variety of cyt-cs with different positions and functions are involved in the EET pathway, such as CymA inner membrane protein, Fcc3 and STC periplasmic protein, MtrA and OmcA outer membrane protein in the model bacterium Shewanella oneidensis MR-1 (Zhao et al., 2021); MacA inner membrane protein, PpcA periplasmic protein, OmcS and OmcZ outer membrane protein in the model bacterium G. sulfurreducens (Mehta et al., 2005; Nevin et al., 2009). It has been reported that the cyt-cs of EAMs can reduce U(VI) and Cr(VI) in vitro (Shelobolina et al., 2007; Liu et al., 2016). This series of proteins constitute the electron transfer chain of EAMs, such as the Mtr respiratory pathway of MR-1. Wang et al. found that the reduction ability of the MR-1 mutant with the deficiency of CymA to nitroaromatic hydrocarbons was 64.3% lower than that of wild-type bacteria, which indicated that CymA played an important role in the detoxification ability of MR-1 (Wang et al., 2020). In addition, it is estimated that the periplasmic space (approximately 0.2 fL) of an MR-1-cell contains more than 300,000 hemes from cyt-cs (Sturm et al., 2015). This means that EAMs may have multiple EET pathways. For example, its similar homologous complex MtrDEF can also play a role in compensating for the electron transfer function when MtrABC is blocked (McLean et al., 2008). In general, these cyt-cs constitute a dense electron transfer network, which provides multiple EET pathways for EAMs to reduce and detoxify toxic pollutants extracellularly.
Nanowires, which are conductive appendages and filaments that can transmit electrons extracellularly, can also be used by EAMs to transfer electrons across extended distances (Gorby et al., 2006; Shi et al., 2016). Shewanella can generate nanowires with a length of tens of microns by extending the fusion of MtrC and OmcA to the outside of cells (El-Naggar et al., 2010; Pirbadian et al., 2014). However, the nanowires of Geobacter are pili composed of PilA protein, and their electrical conductivity may come from the aromatic amino acids located on PilA protein or OmcS with electrical conductivity (Vargas et al., 2013; Liu et al., 2019). According to previous research reports, nanowires exhibit a good performance of extracellular catalytic reduction under the stress of heavy metals. G. sulfurreducens can transfer electrons to extracellular U(VI) by conductive pili and then reduce it to insoluble U(IV), which precipitates on the surface of pili to achieve extracellular detoxification (Cologgi et al., 2011; Reguera, 2018). Therefore, this electron transfer mechanism can reduce the harm to microorganisms from toxic pollutants within the conductive range of nanowires and plays a protective role.
Due to the good redox properties, some endogenous (e.g., phenazines, flavins, and quinones) and exogenous [e.g., anthraquinone-2,6-disulfonate (AQDS), cysteine, and sulfur-containing molecules] small-molecule electroactive substances can flexibly mediate and promote the EET process (Huang et al., 2018). These substances are called electron shuttles (Wu et al., 2020). Marsili et al. (2008) found that the efficiency of electron transfer to the electrode of the MR-1 biofilm was reduced by more than 70% after removing flavins. Conversely, the addition of electron shuttles can enhance the detoxification ability of EAMs. Chen et al. found that the addition of AQDS promoted electron transfer between soil microorganisms and iron minerals, thus producing more active Fe(II) to promote the dechlorination of pentachlorophenol (Chen et al., 2020a). Therefore, electron shuttles can make a great contribution to promoting the process in which EAMs transfer electrons extracellularly to toxic pollutants by the EET pathway.
In addition, the extracellular polymeric substances (EPSs) that are tightly attached to the cell membrane surface have a certain auxiliary effect on the extracellular detoxification of EAMs. On the one hand, EPSs can be regarded as a “huge” protective barrier, in which rich functional groups, such as carboxyl, hydroxyl, and sulfonyl groups, actively participate in the binding of toxic pollutants (Maurya et al., 2022). On the other hand, EPSs are important interface mediums for communication and materials exchange between EAMs and the external environment, particularly when used as electron shuttles (e.g., polysaccharide, protein, and humic acid) can promote the EET process (Sedenho et al., 2021). Jiang and Kappler (2008) found that G. sulfurreducens used dissolved humic substances as electron shuttles to transfer electrons to iron oxide at a rate approximately seven times higher than that of direct transfer. Interestingly, the hydrophobicity and permeability of cell membrane will change under toxic stress, which promotes the release of macromolecules such as proteins and polysaccharides, thus increasing the content of EPSs on the surface of EAMs and improving their detoxification effect (Maurya et al., 2022).
In summary, EET plays an important role in the extracellular detoxification of toxic pollutants by EAMs. In terms of EET, the abundant cyt-cs in cells are the main source of the ability of EAMs, constituting various electron transmission pathways and playing a supporting role in the whole EET process. While the endogenous or exogenous electron shuttles and EPSs with good redox activity can also flexibly promote electron transfer (Li et al., 2016).
Strengthening the extracellular detoxification strategies
Compared with extracellular detoxification, intracellular detoxification is a passive response to the stress of toxic pollutants. EAMs can detoxify toxic pollutants in cells, but the effect of toxicity on EAMs cannot be prevented. Therefore, extracellular detoxification under toxicity stress is more beneficial for EAMs to survive in an environment of toxic pollutants. However, the relatively inefficient electron transfer between electron acceptors and donors is the bottleneck that prevents EAMs from using the ability of EET to detoxify toxic pollutants (Wang et al., 2022). Therefore, if the EET ability of EAMs can be improved by some strategies, the extracellular detoxification ability of EAMs to toxic pollutants may be strengthened (Figure 3).
Enhanced electron transfer pathway
The EET pathway of EAMs is mainly composed of cyt-cs, small-molecule electroactive substances, and nanowires. Among them, cyt-cs play a supporting role in the whole EET process (Chiranjeevi and Patil, 2020); nanowires play an important role in the long-distance EET of EAMs (Lovley, 2011); small molecule electroactive substances can be used as electron shuttles to flexibly transfer electrons (Watanabe et al., 2009). At the same time, it has been found that some nano scale artificial conductive materials can also promote the EET process of EAMs (Zhao et al., 2021). Therefore, increasing the content of cyt-cs, nanowires, small-molecule electroactive substances and artificial conductive materials may be a strategy to promote the EET process of EAMs and strengthen their detoxification ability.
Studies have proven that biosynthesis can regulate the expression of related genes to promote the synthesis of cyt-cs that are related to the EET pathway in EAMs, thus strengthening their detoxification ability (Rosenbaum and Henrich, 2014). Li Y. et al. (2020) induced the overexpression of cyt-cs (e.g., MtrA, MtrB, MtrD, and OmcA) genes of S. xiamenensis by graphene oxide, thereby increasing the ability to reduce Cr(VI) by 2.7 times. In addition, some researchers have promoted the EET ability of other EAMs by the heterologous expression of cyt-cs genes with good EET performance. For example, Jensen et al. (2010) reconstituted MtrA, MtrB, and MtrC on the EET pathway of S. oneidensis MR-1 into Escherichia coli, thereby making the ability to reduce metal ions and their oxides of engineered strains eight times and four times higher than that of the parent wild-type, respectively.
Increasing the content of electron shuttles in the EET pathway can also improve the detoxification ability of EAMs (Liu et al., 2018). On the one hand, add exogenous electron shuttles directly. For example, Kang et al. (2022) found that under the condition of Fe(III) reduction, the addition of sucrose and NH4+ enhanced the reduction ability of EAMs to perfluoroalkyl substances in sediments, while the system with added NH4+ had the best enhancement effect, and the remaining perfluoroalkyl substances in this system decreased by 30.2% compared with that of the control group after 5 days. On the other hand, promote the generation of endogenous electron shuttles by regulating gene expression. Yang et al. (2022) heterologously expressed the riboflavin synthesis gene cluster ribADEHC of Bacillus subtilis in S. carassii-D5, thus the riboflavin yield and the reduction efficiency of methyl orange were 4.7 times and 1.3 times that of the wild type, respectively.
It has been reported that promoting the synthesis of nanowires can strengthen the EET ability of EAMs. For example, Leang et al. (2013) knocked out the PilZ protein coding gene GSU1240 in the G. sulfurreducens genome to make engineered strains produce more nanowires, while the maximum current density increased by 1.5 times. However, at present, few studies have reported that this strategy can improve the detoxification ability of EAMs. As nanowires play an important role in the long-distance and interspecific electron transfer process of EAMs, it is speculated that this strategy may help to strengthen the detoxification ability of EAMs to extracellular toxic pollutants and promote the synergistic detoxification among EAMs.
Recent studies have found that carbon nanotubes with good biocompatibility and excellent electrical conductivity can promote the redox process of cyt-cs (Zhang et al., 2018a). To explore whether carbon nanotubes can strengthen the detoxification ability of EAMs, Yan et al. (2013) found that most nitrobenzene was reduced extracellularly by adding 0.5% (w/v) carbon nanotubes to the cell-immobilized alginate beads, and the reduction efficiency of nitrobenzene increased by 74%. In addition, it was observed that the strain showed better activity under toxicity stress. It is speculated that the reason why CNTs can change the electron transfer mechanism of EAMs is as follows: (1) CNTs are rich in redox-active sites that are conducive to high conductivity, thus accelerating EET (Tasis et al., 2006); (2) CNTs can absorb a variety of organic compounds (Chen et al., 2007; Zhang et al., 2009), thus allowing greater mass diffusion and enhancing reaction kinetics. Additionally, whether other nanomaterials with similar physical and chemical properties to carbon nanotubes have this function is worthy of further study (Guo et al., 2015; Liu et al., 2015; Huang et al., 2017).
Increasing the content of electronic carrier
NAD (H/+) in EAMs is an important carrier of electrons in the EET pathway, and it is also among the key limiting factors of its detoxification ability (Li et al., 2018a). Therefore, regulating the synthesis of intracellular NAD (H/+) may solve the problem involving the low electron transfer efficiency in the EET pathway by promoting the generation of electrons, thus strengthening the detoxification ability of EAMs (Yong et al., 2014). Yong et al. (2014) significantly increased the availability of NAD (H/+) by overexpressing the NAD synthase gene nadE, thereby making the output power of the engineered strain 3 times higher than that of the wild-type strain. Additionally, several studies have applied the strategy of modular synthesis biology, such as Li et al. (2018a) redirected the synthesis of NAD+ by using three modules (ab initio, remediation, and general biosynthesis) of S. oneidensis MR-1, thereby increasing the content of NAD (H/+) in cells and increasing the number of electrons entering the EET pathway. Similarly, whether this strategy can strengthen the detoxification ability of EAMs needs further exploration.
Regulating the expression of signaling molecules
Intracellular signaling molecules can influence the growth and metabolic activity of EAMs by regulating the expression of related genes, such as the second messenger or global regulator, which can regulate its EET pathway (Camilli and Bassler, 2006). Therefore, whether the detoxification ability of EAMs can be strengthened by regulating the expression of intracellular signaling molecules has attracted the attention of many researchers. Cheng et al. (2020) heterologously expressed the adenylate cyclase-encoding gene from Beggiatoa sp. PS in S. oneidensis MR-1 to increase intracellular second messenger cyclic adenosine 3’, 5’-monophosphate (cAMP) content, thereby upregulating the expression level of the coding genes of the cyt-cs and flavin synthesis pathways, resulting in the reduction rate of Cr(VI) being increased by three times higher. Additionally, several studies have found that the EET efficiency of EAMs can also be improved by regulating intracellular global regulatory factors. For example, Luo et al. (2018) introduced the global regulatory factor IrrE of Deinococcus radiodurans into P. aeruginosa PAO1, thereby promoting the secretion of more phenazine compounds and increasing the maximum power density of the system by 70%. Therefore, regulating the expression of intracellular signaling molecules is a feasible strategy to strengthen the detoxification ability of EAMs.
Conclusion and outlook
Toxicity stress has a strong detrimental impact on microbial activity during the bioremediation of toxic pollutants. Under toxicity stress, EAMs exhibit more steady activity than ordinary microorganisms, which is mostly due to EAMs’ unique and outstanding detoxifying capabilities. However, the detoxification mechanisms of EAMs are still lack relevant comprehensive review. This review summarizes the toxicogenic pathways of toxic pollutants to microorganisms, the detoxification mechanisms of EAMs and important strategies to strengthen extracellular detoxification:
1) The main toxicogenic mechanisms of toxic pollutants include direct covalent binding of toxic pollutants’ electrophilic groups to nucleophilic groups of biological macromolecules, as well as the promotion of ROS generation causing oxidative damage to cells.
2) Under toxicity stress, the detoxification mechanisms of EAMs to toxic pollutants are included intracellular detoxification and extracellular detoxification. The excellent detoxification ability of EAMs comes from its extracellular detoxification mechanism, which can extracellularly reduce electrophilic toxic pollutants through EET pathway.
3) The extracellular detoxification capacity of EAMs can be further improved by enhancing the electron transport pathway, regulating the synthesis of NAD (H/+) and modulating signaling molecule expression.
However, the study on detoxification of EAMs under toxicity stress is still in the initial stage, with most studies focusing on a single pollutant and rarely on the combined effects of pollutants. Therefore, comprehensive researches into the detoxification process of EAMs on composite contaminants are required for improved applicability in practical engineering. At the same time, it is unknown whether the metabolic activities of other microorganisms coexisting with EAMs in practical applications will assist or interfere EAM detoxification. Furthermore, many strategies have been demonstrated to enhance the ability EET, but some of them have not been applied to enhance their detoxification ability under toxicity stress, thus the EET ability of EAMs and the mechanism of enhancing their detoxification ability remain to be further explored. Further clarification of these understandings may help us to develop EAMs-based toxic pollutants remediation technologies, as well as providing a more complete theoretical basis for future practical engineering applications.
Author contributions
HF: funding acquisition, conceptualization, writing—review and editing, and supervision. LX: investigation, visualization, formal analysis, and writing—original draft. RC: conceptualization, methodology, and writing—review and editing. XM: formal analysis and writing—review and editing. HQ: formal analysis and writing—polishing and editing. NZ: investigation and writing—review and editing. YD: writing—review and editing. DW: supervision. All authors contributed to the article and approved the submitted version.
Funding
This work was supported by the National Natural Science Foundation of China (51978615) and the Zhejiang Provincial Ten Thousand Talent Program (2021R52055).
Conflict of interest
The authors declare that the research was conducted in the absence of any commercial or financial relationships that could be construed as a potential conflict of interest.
Publisher’s note
All claims expressed in this article are solely those of the authors and do not necessarily represent those of their affiliated organizations, or those of the publisher, the editors and the reviewers. Any product that may be evaluated in this article, or claim that may be made by its manufacturer, is not guaranteed or endorsed by the publisher.
References
Alegbeleye, O. O., Opeolu, B. O., and Jackson, V. A. (2017). Polycyclic aromatic hydrocarbons: A critical review of environmental occurrence and bioremediation. Environ. Manage. 60, 758–783. doi: 10.1007/s00267-017-0896-2
Alfryyan, N., Kordy, M. G. M., Abdel-Gabbar, M., Soliman, H. A., and Shaban, M. (2022). Characterization of the biosynthesized intracellular and extracellular plasmonic silver nanoparticles using Bacillus cereus and their catalytic reduction of methylene blue. Sci Rep. 12:12495. doi: 10.1038/s41598-022-16029-1
Ballard, J. W. O., and Towarnicki, S. G. (2020). Mitochondria, the gut microbiome and ROS. Cell. Signall. 75:109737. doi: 10.1016/j.cellsig.2020.109737
Beretta, G., Daghio, M., Espinoza Tofalos, A., Franzetti, A., Mastorgio, A. F., Saponaro, S., et al. (2020). Microbial assisted hexavalent chromium removal in bioelectrochemical systems. Water. 12:466. doi: 10.3390/w12020466
Bryant, S. E., and Schultz, T. W. (1994). Toxicological assessment of biotransformation products of pentachlorophenol: Tetrahymena population growth impairment. Arch. Environ. Contam. Toxicol. 26, 299–303. doi: 10.1007/BF00203555
Camilli, A., and Bassler, B. L. (2006). Bacterial small-molecule signaling pathways. Science 311, 1113–1116. doi: 10.1126/science.1121357
Chang, F., Tian, C. K., Liu, S. T., and Ni, J. R. (2016). Discrepant hexavalent chromium tolerance and detoxification by two strains of Trichoderma asperellum with high homology. Chem. Eng. J. 298, 75–81. doi: 10.1016/j.cej.2016.04.023
Chaudhary, S., Yadav, S., Singh, R., Sadhotra, C., and Patil, S. A. (2022). Extremophilic electroactive microorganisms: Promising biocatalysts for bioprocessing applications. Bioresour. Technol. 347:126663. doi: 10.1016/j.biortech.2021.126663
Chen, F., Liang, B., Li, Z. L., Yang, J. Q., Huang, C., Lyu, M., et al. (2019). Bioelectrochemical assisted dechlorination of tetrachloroethylene and 1,2-dichloroethane by acclimation of anaerobic sludge. Chemosphere 227, 514–521. doi: 10.1016/j.chemosphere.2019.04.066
Chen, J., and Strous, M. (2013). Denitrification and aerobic respiration, hybrid electron transport chains and co-evolution. Biochim. Biophys. Acta. Bioenerg. 1827, 136–144. doi: 10.1016/j.bbabio.2012.10.002
Chen, X., Zhao, Y., Zhang, C., Zhang, D., Yao, C., Meng, Q., et al. (2020b). Speciation, toxicity mechanism and remediation ways of heavy metals during composting: A novel theoretical microbial remediation method is proposed. J. Environ. Manage. 272:111109. doi: 10.1016/j.jenvman.2020.111109
Chen, M., Tong, H., Qiao, J., Lv, Y., Jiang, Q., Gao, Y., et al. (2020a). Microbial community response to the toxic effect of pentachlorophenol in paddy soil amended with an electron donor and shuttle. Ecotoxicol. Environ. Saf. 205:111328. doi: 10.1016/j.ecoenv.2020.111328
Chen, W., Duan, L., and Zhu, D. Q. (2007). Adsorption of polar and nonpolar organic chemicals to carbon nanotubes. Environ. Sci. Technol. 41, 8295–8300. doi: 10.1021/es071230h
Cheng, Z. H., Xiong, J. R., Min, D., Cheng, L., Liu, D. F., Li, W. W., et al. (2020). Promoting bidirectional extracellular electron transfer of Shewanella oneidensis MR-1 for hexavalent chromium reduction via elevating intracellular cAMP level. Biotechnol. Bioeng. 117, 1294–1303. doi: 10.1002/bit.27305
Chiranjeevi, P., and Patil, S. A. (2020). Strategies for improving the electroactivity and specific metabolic functionality of microorganisms for various microbial electrochemical technologies. Biotechnol. Adv. 39:107468. doi: 10.1016/j.biotechadv.2019.107468
Cologgi, D. L., Lampa-Pastirk, S., Speers, A. M., Kelly, S. D., and Reguera, G. (2011). Extracellular reduction of uranium via Geobacter conductive pili as a protective cellular mechanism. Proc. Natl. Acad. Sci. U.S.A. 108, 15248–15252. doi: 10.1073/pnas.1108616108
Cui, M.-H., Liu, W.-Z., and Cui, D. (2021). Recent advancements in azo dye decolorization in bio-electrochemical systems (BESs): Insights into decolorization mechanism and practical application. Water Res. 203:117512. doi: 10.1016/j.watres.2021.117512
Davies, M. J., Gilbert, B. C., and Haywood, R. M. (1991). Radical-induced damage to proteins: E.S.R. spin-trapping studies. Free Radical. Res. Commun. 15, 111–127. doi: 10.3109/10715769109049131
Dolan, M. E., and McCarty, P. L. (1995). Methanotrophic chloroethene transformation capacities and 1,1-dichloroethene transformation product toxicity. Environ. Sci. Technol. 29, 2741–2747. doi: 10.1021/es00011a007
Dudev, T., and Lim, C. (2014). Competition among metal ions for protein binding sites: Determinants of metal ion selectivity in proteins. Chem. Rev. 114, 538–556. doi: 10.1021/cr4004665
El-Naggar, M. Y., Wanger, G., Leung, K. M., Yuzvinsky, T. D., Southam, G., Yang, J., et al. (2010). Electrical transport along bacterial nanowires from Shewanella oneidensis MR-1. Proc. Natl. Acad. Sci. U.S.A. 107, 18127–18131. doi: 10.1073/pnas.1004880107
Feng, H. J., Liang, Y. X., Guo, K., Long, Y. Y., Cong, Y. Q., and Shen, D. S. (2015). Addition of nitrite enhances the electrochemical defluorination of 2-fluoroaniline. J. Hazard. Mater. 300, 607–614. doi: 10.1016/j.jhazmat.2015.06.071
Fuchs, G., Boll, M., and Heider, J. (2011). Microbial degradation of aromatic compounds - from one strategy to four. Nat. Rev. Microbiol. 9, 803–816. doi: 10.1038/nrmicro2652
Gao, N., Huang, Z., Liu, H., Hou, J., and Liu, X. (2019). Advances on the toxicity of uranium to different organisms. Chemosphere 237:124548. doi: 10.1016/j.chemosphere.2019.124548
Gorby, Y. A., Yanina, S., McLean, J. S., Rosso, K. M., Moyles, D., Dohnalkova, A., et al. (2006). Electrically conductive bacterial nanowires produced by Shewanella oneidensis strain MR-1 and other microorganisms. Proc. Natl. Acad. Sci. U.S.A. 103, 11358–11363. doi: 10.1073/pnas.0604517103
Guo, K., Soeriyadi, A. H., Feng, H., Prevoteau, A., Patil, S. A., Gooding, J. J., et al. (2015). Heat-treated stainless steel felt as scalable anode material for bioelectrochemical systems. Bioresour. Technol. 195, 46–50. doi: 10.1016/j.biortech.2015.06.060
Guo, S. Y., Xiao, C. Q., Zhou, N., and Chi, R. (2021). Speciation, toxicity, microbial remediation and phytoremediation of soil chromium contamination. Environ. Chem. Lett. 19, 1413–1431. doi: 10.1007/s10311-020-01114-6
He, C. W., Gu, L. P., Xu, Z. X., He, H., Fu, G., Han, F. X., et al. (2020). Cleaning chromium pollution in aquatic environments by bioremediation, photocatalytic remediation, electrochemical remediation and coupled remediation systems. Environ. Chem. Lett. 18, 561–576. doi: 10.1007/s10311-019-00960-3
Huang, B., Gao, S., Xu, Z., He, H., and Pan, X. (2018). The functional mechanisms and application of electron shuttles in extracellular electron transfer. Curr. Microbiol. 75, 99–106. doi: 10.1007/s00284-017-1386-8
Huang, L. J., Zhang, X. Q., Shen, D. S., Li, N., Ge, Z. P., Zhou, Y. Y., et al. (2017). Effect of heat-treatment atmosphere on the current generation of TiO2 nanotube array electrodes in microbial fuel cells. Electrochim. Acta. 257, 203–209. doi: 10.1016/j.electacta.2017.10.068
Ismail, N. A., Kasmuri, N., and Hamzah, N. (2022). Microbial bioremediation techniques for polycyclic aromatic hydrocarbon (PAHs)—a review. Water Air Soil Pollut. 233:124. doi: 10.1007/s11270-022-05598-6
Israel, Y., Hurwitz, E., Niemelä, O., and Arnon, R. (1986). Monoclonal and polyclonal antibodies against acetaldehyde-containing epitopes in acetaldehyde-protein adducts. Proc. Natl. Acad. Sci. U.S.A. 83, 7923–7927. doi: 10.1073/pnas.83.20.7923
Jensen, H. M., Albers, A. E., Malley, K. R., Londer, Y. Y., Cohen, B. E., Helms, B. A., et al. (2010). Engineering of a synthetic electron conduit in living cells. Proc. Natl. Acad. Sci. U.S.A. 107, 19213–19218. doi: 10.1073/pnas.1009645107
Jiang, J., and Kappler, A. (2008). Kinetics of microbial and chemical reduction of humic substances: Implications for electron shuttling. Environ. Sci. Technol. 42, 3563–3569. doi: 10.1021/es7023803
Jiang, Y., Yang, F., Dai, M., Ali, I., Shen, X., Hou, X., et al. (2022). Application of microbial immobilization technology for remediation of Cr(VI) contamination: A review. Chemosphere 286:131721. doi: 10.1016/j.chemosphere.2021.131721
Kang, Y., Li, M., Guo, Z. Z., Zhang, Y., Li, W. C., Wu, H. M., et al. (2022). Effect of electron shuttles on typical perfluoroalkyl substance removal via iron oxide reduction in wetland sediment. J. Cleaner Prod. 365:132821. doi: 10.1016/j.jclepro.2022.132821
Leang, C., Malvankar, N. S., Franks, A. E., Nevin, K. P., and Lovley, D. R. (2013). Engineering Geobacter sulfurreducens to produce a highly cohesive conductive matrix with enhanced capacity for current production. Energy Environ. Sci. 6, 1901–1908. doi: 10.1039/c3ee40441b
Li, Q., Feng, X. L., Lu, X. R., Li, T. T., Han, X., Xiao, X., et al. (2018b). Combined intra- and extracellular reduction involved in the anaerobic biodecolorization of cationic azo dye by Shewanella oneidensis MR-1. Chemosphere 211, 701–708. doi: 10.1016/j.chemosphere.2018.08.006
Li, F., Li, Y. X., Cao, Y. X., Wang, L., Liu, C. G., Shi, L., et al. (2018a). Modular engineering to increase intracellular NAD (H/(+)) promotes rate of extracellular electron transfer of Shewanella oneidensis. Nat. Commun. 9:3637. doi: 10.1038/s41467-018-05995-8
Li, S. W., Sheng, G. P., Cheng, Y. Y., and Yu, H. Q. (2016). Redox properties of extracellular polymeric substances (EPS) from electroactive bacteria. Sci. Rep. 6:39098. doi: 10.1038/srep39098
Li, W. W., and Yu, H. Q. (2015). Electro-assisted groundwater bioremediation: Fundamentals, challenges and future perspectives. Bioresour. Technol. 196, 677–684. doi: 10.1016/j.biortech.2015.07.074
Li, X. X., Lan, X., Liu, W., Cui, X. W., and Cui, Z. J. (2020). Toxicity, migration and transformation characteristics of lead in soil-plant system: Effect of lead species. J. Hazard. Mater. 395:122676. doi: 10.1016/j.jhazmat.2020.122676
Li, Y., Chen, Z., Shi, Y., Luo, Q., Wang, Y., Wang, H., et al. (2020). Function of c-type cytochromes of Shewanella xiamenensis in enhanced anaerobic bioreduction of Cr(VI) by graphene oxide and graphene oxide/polyvinyl alcohol films. J. Hazard. Mater. 387:122018. doi: 10.1016/j.jhazmat.2020.122018
Liu, F. H., Rotaru, A. E., Shrestha, P. M., Malvankar, N. S., Nevin, K. P., and Lovley, D. R. (2015). Magnetite compensates for the lack of a pilin-associated c-type cytochrome in extracellular electron exchange. Environ. Microbiol. 17, 648–655. doi: 10.1111/1462-2920.12485
Liu, S. M., Wu, C. H., and Huang, H. J. (1998). Toxicity and anaerobic biodegradability of pyridine and its derivatives under sulfidogenic conditions. Chemosphere 36, 2345–2357. doi: 10.1016/S0045-6535(97)10203-X
Liu, T., Li, X., Li, F., Han, R., Wu, Y., Yuan, X., et al. (2016). In situ spectral kinetics of Cr(VI) reduction by c-type cytochromes in a suspension of living Shewanella putrefaciens 200. Sci. Rep. 6:29592. doi: 10.1038/srep29592
Liu, T., Luo, X., Wu, Y., Reinfelder, J. R., Yuan, X., Li, X., et al. (2020). Extracellular electron shuttling mediated by soluble c-type cytochromes produced by shewanella oneidensis MR-1. Environ. Sci. Technol. 54, 10577–10587. doi: 10.1021/acs.est.9b06868
Liu, X., Shi, L., and Gu, J. D. (2018). Microbial electrocatalysis: Redox mediators responsible for extracellular electron transfer. Biotechnol. Adv. 36, 1815–1827. doi: 10.1016/j.biotechadv.2018.07.001
Liu, X., Zhan, J., Jing, X. Y., Zhou, S. G., and Lovley, D. R. (2019). A pilin chaperone required for the expression of electrically conductive Geobacter sulfurreducens pili. Environ. Microbiol. 21, 2511–2522. doi: 10.1111/1462-2920.14638
Loeppky, R. N., and Shi, J. Z. (2008). N-nitrosotolazoline: Decomposition studies of a typical N-nitrosoimidazoline. Chem. Res. Toxicol. 21, 308–318. doi: 10.1021/tx700318k
Logan, B. E., Rossi, R., Ragab, A. A., and Saikaly, P. E. (2019). Electroactive microorganisms in bioelectrochemical systems. Nat. Rev. Microbiol. 17, 307–319. doi: 10.1038/s41579-019-0173-x
Lovley, D. R. (2011). Live wires: Direct extracellular electron exchange for bioenergy and the bioremediation of energy-related contamination. Energy Environ. Sci. 4, 4896–4906. doi: 10.1039/c1ee02229f
Luan, F., Liu, Y., Griffin, A. M., Gorski, C. A., and Burgos, W. D. (2015). Iron(III)-bearing clay minerals enhance bioreduction of nitrobenzene by Shewanella putrefaciens CN32. Environ. Sci. Technol. 49, 1418–1426. doi: 10.1021/es504149y
Luo, J. M., Wang, T. T., Li, X., Yang, Y. N., Zhou, M. H., Li, M., et al. (2018). Enhancement of bioelectricity generation via heterologous expression of IrrE in Pseudomonas aeruginosa-inoculated MFCs. Biosens. Bioelectron. 117, 23–31. doi: 10.1016/j.bios.2018.05.052
Ma, X. K., Ding, N., Peterson, E. C., and Daugulis, A. J. (2016). Heavy metals species affect fungal-bacterial synergism during the bioremediation of fluoranthene. Appl. Environ. Microbiol. 100, 7741–7750. doi: 10.1007/s00253-016-7595-4
Marsili, E., Baron, D. B., Shikhare, I. D., Coursolle, D., Gralnick, J. A., and Bond, D. R. (2008). Shewanella secretes flavins that mediate extracellular electron transfer. Proc. Natl. Acad. Sci. U.S.A. 105, 3968–3973. doi: 10.1073/pnas.0710525105
Mauch, T. J., Donohue, T. M. J., Zetterman, R. K., Sorrell, M. F., and Tuma, D. J. (1986). Covalent binding of acetaldehyde selectively inhibits the catalytic activity of lysine-dependent enzymes. Hepatology 6, 263–269. doi: 10.1002/hep.1840060218
Maurya, A., Kumar, R., Yadav, P., Singh, A., Yadav, A., Chowdhary, P., et al. (2022). Biofilm formation and extracellular polymeric substance (EPS) production by Bacillus haynesii and influence of hexavalent chromium. Bioresour. Technol. 352:127109. doi: 10.1016/j.biortech.2022.127109
McLean, J. S., Pinchuk, G. E., Geydebrekht, O. V., Bilskis, C. L., Zakrajsek, B. A., Hill, E. A., et al. (2008). Oxygen-dependent autoaggregation in Shewanella oneidensis MR-1. Environ. Microbiol. 10, 1861–1876. doi: 10.1111/j.1462-2920.2008.01608.x
Mehta, T., Coppi, M. V., Childers, S. E., and Lovley, D. R. (2005). Outer membrane c-type cytochromes required for Fe(III) and Mn(IV) oxide reduction in Geobacter sulfurreducens. Appl. Environ. Microbiol. 71, 8634–8641. doi: 10.1128/AEM.71.12.8634-8641.2005
Morcillo, P., Esteban, M. Á, and Cuesta, A. (2016). Heavy metals produce toxicity, oxidative stress and apoptosis in the marine teleost fish SAF-1 cell line. Chemosphere 144, 225–233. doi: 10.1016/j.chemosphere.2015.08.020
Nevin, K. P., Kim, B.-C., Glaven, R. H., Johnson, J. P., Woodard, T. L., Methe, B. A., et al. (2009). Anode biofilm transcriptomics reveals outer surface components essential for high density current production in Geobacter sulfurreducens fuel cells. PLoS One 4:e5628. doi: 10.1371/journal.pone.0005628
Nzila, A. (2018). Biodegradation of high-molecular-weight polycyclic aromatic hydrocarbons under anaerobic conditions: Overview of studies, proposed pathways and future perspectives. Environ. Pollut. 239, 788–802. doi: 10.1016/j.envpol.2018.04.074
Pan-Hou, H. (2010). Application of mercury-resistant genes in bioremediation of mercurials in environments. Yakugaku Zasshi. 130, 1143–1156. doi: 10.1248/yakushi.130.1143
Park, S., Anderson, C., Loeber, R., Seetharaman, M., Jones, R., and Tretyakova, N. (2005). Interstrand and intrastrand DNA-DNA cross-linking by 1,2,3,4-diepoxybutane: Role of stereochemistry. J. Am. Chem. Soc. 127, 14355–14365. doi: 10.1021/ja051979x
Pirbadian, S., Barchinger, S. E., Leung, K. M., Byun, H. S., Jangir, Y., Bouhenni, R. A., et al. (2014). Shewanella oneidensis MR-1 nanowires are outer membrane and periplasmic extensions of the extracellular electron transport components. Proc. Natl. Acad. Sci. U.S.A. 111, 12883–12888. doi: 10.1073/pnas.1410551111
Qian, D. S., Liu, H. M., Hu, F., Song, S., and Chen, Y. C. (2022). Extracellular electron transfer-dependent Cr(VI)/sulfate reduction mediated by iron sulfide nanoparticles. J. Biosci. Bioeng. 134, 153–161. doi: 10.1016/j.jbiosc.2022.05.005
Radzig, M. A., Nadtochenko, V. A., Koksharova, O. A., Kiwi, J., Lipasova, V. A., and Khmel, I. A. (2013). Antibacterial effects of silver nanoparticles on gram-negative bacteria: Influence on the growth and biofilms formation, mechanisms of action. Colloids Surf. B. 102, 300–306. doi: 10.1016/j.colsurfb.2012.07.039
Rathore, S., Varshney, A., Mohan, S., and Dahiya, P. (2022). An innovative approach of bioremediation in enzymatic degradation of xenobiotics. Biotechnol. Genet. Eng. Rev. 38, 1–32. doi: 10.1080/02648725.2022.2027628
Reguera, G. (2018). Microbial nanowires and electroactive biofilms. FEMS Microbiol. Ecol. 94:86. doi: 10.1093/femsec/fiy086
Rosenbaum, M. A., and Henrich, A. W. (2014). Engineering microbial electrocatalysis for chemical and fuel production. Curr. Opin. Biotechnol. 29, 93–98. doi: 10.1016/j.copbio.2014.03.003
Roy, D. R., Parthasarathi, R., Maiti, B., Subramanian, V., and Chattaraj, P. K. (2005). Electrophilicity as a possible descriptor for toxicity prediction. Bioorg. Med. Chem. 13, 3405–3412. doi: 10.1016/j.bmc.2005.03.011
Seck, E. I., Dona-Rodriguez, J. M., Fernandez-Rodriguez, C., Gonzalez-Diaz, O. M., Arana, J., and Perez-Pena, J. (2012). Photocatalytic removal of 2,4-dichlorophenoxyacetic acid by using sol-gel synthesized nanocrystalline and commercial TiO2: Operational parameters optimization and toxicity studies. Appl. Catal. B. 125, 28–34. doi: 10.1016/j.apcatb.2012.05.028
Sedenho, G. C., Modenez, I., Mendes, G. R., and Crespilho, F. N. (2021). The role of extracellular polymeric substance matrix on Saccharomyces cerevisiae bioelectricity. Electrochim. Acta. 393:139080. doi: 10.1016/j.electacta.2021.139080
Seo, J. S., Keum, Y. S., and Li, Q. X. (2009). Bacterial degradation of aromatic compounds. Int. J. Environ. Res. Public Health. 6, 278–309. doi: 10.3390/ijerph6010278
Sharma, P., and Melkania, U. (2018). Impact of heavy metals on hydrogen production from organic fraction of municipal solid waste using co-culture of Enterobacter aerogenes and E. Coli. Waste Manage. 75, 289–296. doi: 10.1016/j.wasman.2018.02.005
Shelobolina, E. S., Coppi, M. V., Korenevsky, A. A., DiDonato, L. N., Sullivan, S. A., Konishi, H., et al. (2007). Importance of c-type cytochromes for U(VI) reduction by Geobacter sulfurreducens. BMC Microbiol. 7:16. doi: 10.1186/1471-2180-7-16
Shi, J., Zhang, B., Cheng, Y., and Peng, K. (2020). Microbial vanadate reduction coupled to co-metabolic phenanthrene biodegradation in groundwater. Water Res. 186:116354. doi: 10.1016/j.watres.2020.116354
Shi, L., Dong, H. L., Reguera, G., Beyenal, H., Lu, A. H., Liu, J., et al. (2016). Extracellular electron transfer mechanisms between microorganisms and minerals. Nat. Rev. Microbiol. 14, 651–662. doi: 10.1038/nrmicro.2016.93
Shibata, T., and Uchida, K. (2019). Protein adductomics: A comprehensive analysis of protein modifications by electrophiles. Free Radical Biol. Med. 144, 218–222. doi: 10.1016/j.freeradbiomed.2019.02.034
Sies, H., and Jones, D. P. (2020). Reactive oxygen species (ROS) as pleiotropic physiological signalling agents. Nat. Rev. Mol. Cell Biol. 21, 363–383. doi: 10.1038/s41580-020-0230-3
Smith, S. L., Jennett, R. B., Sorrell, M. F., and Tuma, D. J. (1989). Acetaldehyde substoichiometrically inhibits bovine neurotubulin polymerization. J. Clin. Invest. 84, 337–341. doi: 10.1172/JCI114159
Sturm, G., Richter, K., Doetsch, A., Heide, H., Louro, R. O., and Gescher, J. (2015). A dynamic periplasmic electron transfer network enables respiratory flexibility beyond a thermodynamic regulatory regime. ISME J. 9, 1802–1811. doi: 10.1038/ismej.2014.264
Sun, S. Y., Hou, Y. N., Wei, W., Sharif, H. M. A., Huang, C., Ni, B. J., et al. (2022b). Perturbation of clopyralid on bio-denitrification and nitrite accumulation: Long-term performance and biological mechanism. Environ. Sci. Ecotechnol. 9:100144. doi: 10.1016/j.ese.2021.100144
Sun, Q., Li, Y., Shi, L., Hussain, R., Mehmood, K., Tang, Z., et al. (2022a). Heavy metals induced mitochondrial dysfunction in animals: Molecular mechanism of toxicity. Toxicology. 469:153136. doi: 10.1016/j.tox.2022.153136
Tanaka, Y., and Ono, A. (2008). Nitrogen-15 NMR spectroscopy of N-metallated nucleic acids: Insights into N-15 NMR parameters and N-metal bonds. Dalton Trans. 40, 4965–4974. doi: 10.1039/b803510p
Tas, D. O., and Pavlostathis, S. G. (2014). Occurrence, toxicity, and biotransformation of pentachloronitrobenzene and chloroanilines. Crit. Rev. Environ. Sci. Technol. 44, 473–518. doi: 10.1080/10643389.2012.728809
Tasis, D., Tagmatarchis, N., Bianco, A., and Prato, M. (2006). Chemistry of carbon nanotubes. Chem. Rev. 106, 1105–1136. doi: 10.1021/cr050569o
Tekpli, X., Holme, J. A., Sergent, O., and Lagadic-Gossmann, D. (2011). Importance of plasma membrane dynamics in chemical-induced carcinogenesis. Recent Pat. Anti-Cancer Drug Discovery. 6, 347–353. doi: 10.2174/157489211796957784
Thapa, B. S., Kim, T., Pandit, S., Song, Y. E., Afsharian, Y. P., Rahimnejad, M., et al. (2022). Overview of electroactive microorganisms and electron transfer mechanisms in microbial electrochemistry. Bioresour. Technol. 347:126579. doi: 10.1016/j.biortech.2021.126579
Thatoi, H., Das, S., Mishra, J., Rath, B. P., and Das, N. (2014). Bacterial chromate reductase, a potential enzyme for bioremediation of hexavalent chromium: A review. J. Environ. Manage. 146, 383–399. doi: 10.1016/j.jenvman.2014.07.014
Thiebault, C., Carriere, M., Milgram, S., Simon, A., Avoscan, L., and Gouget, B. (2007). Uranium induces apoptosis and is genotoxic to normal rat kidney (NRK-52E) proximal cells. Toxicol. Sci. 98, 479–487. doi: 10.1093/toxsci/kfm130
Tobler, N. B., Hofstetter, T. B., and Schwarzenbach, R. P. (2007). Assessing iron-mediated oxidation of toluene and reduction of nitroaromatic contaminants in anoxic environments using compound-specific isotope analysis. Environ. Sci. Technol. 41, 7773–7780. doi: 10.1021/es071129c
Vahter, M. (2002). Mechanisms of arsenic biotransformation. Toxicology 181-182, 211–217. doi: 10.1016/S0300-483X(02)00285-8
van der Vliet, A., Eiserich, J. P., O’Neill, C. A., Halliwell, B., and Cross, C. E. (1995). Tyrosine modification by reactive nitrogen species: A closer look. Arch. Biochem. Biophys. 319, 341–349. doi: 10.1006/abbi.1995.1303
Vargas, M., Malvankar, N. S., Tremblay, P.-L., Leang, C., Smith, J. A., Patel, P., et al. (2013). Aromatic amino acids required for pili conductivity and long-range extracellular electron transport in Geobacter sulfurreducens. mBio 4:e105–e113. doi: 10.1128/mBio.00210-13
Vondracek, J., and Machala, M. (2021). The role of metabolism in toxicity of polycyclic aromatic hydrocarbons and their non-genotoxic modes of action. Curr. Drug Metab. 22, 584–595. doi: 10.2174/1389200221999201125205725
Wang, H. F., Zhao, H. P., and Zhu, L. Z. (2020). Structures of nitroaromatic compounds induce Shewanella oneidensis MR-1 to adopt different electron transport pathways to reduce the contaminants. J. Hazard. Mater. 384:121495. doi: 10.1016/j.jhazmat.2019.121495
Wang, Y. X., Hou, N., Liu, X. L., and Mu, Y. (2022). Advances in interfacial engineering for enhanced microbial extracellular electron transfer. Bioresour. Technol. 345:126562. doi: 10.1016/j.biortech.2021.126562
Wang, Y., Nie, M., Diwu, Z., Chang, F., Nie, H., Zhang, B., et al. (2021). Toxicity evaluation of the metabolites derived from the degradation of phenanthrene by one of a soil ubiquitous PAHs-degrading strain Rhodococcus qingshengii FF. J. Hazard. Mater. 415:125657. doi: 10.1016/j.jhazmat.2021.125657
Watanabe, K., Manefield, M., Lee, M., and Kouzuma, A. (2009). Electron shuttles in biotechnology. Curr. Opin. Biotechnol. 20, 633–641. doi: 10.1016/j.copbio.2009.09.006
Wehr, H., Rodo, M., Lieber, C. S., and Baraona, E. (1993). Acetaldehyde adducts and autoantibodies against VLDL and LDL in alcoholics. J. Lipid Res. 34, 1237–1244. doi: 10.1016/S0022-2275(20)37711-7
Wells, P. G., and Winn, L. M. (1996). Biochemical toxicology of chemical teratogenesis. Crit. Rev. Biochem. Mol. Biol. 31, 1–40. doi: 10.3109/10409239609110574
Wells, P. G., Kim, P. M., Laposa, R. R., Nicol, C. J., Parmana, T., and Winn, L. M. (1997). Oxidative damage in chemical teratogenesis. Mutat. Res. 396, 65–78. doi: 10.1016/S0027-5107(97)00175-9
Wu, Y. D., Luo, X. B., Qin, B. L., Li, F. B., Haggblom, M. M., and Liu, T. X. (2020). Enhanced current production by exogenous electron mediators via synergy of promoting biofilm formation and the electron shuttling process. Environ. Sci. Technol. 54, 7217–7225. doi: 10.1021/acs.est.0c00141
Xia, X., Wu, S., Zhou, Z., and Wang, G. (2021). Microbial Cd(II) and Cr(VI) resistance mechanisms and application in bioremediation. J. Hazard. Mater. 401:123685. doi: 10.1016/j.jhazmat.2020.123685
Xiao, X., and Yu, H. Q. (2020). Molecular mechanisms of microbial transmembrane electron transfer of electrochemically active bacteria. Curr. Opin. Biotechnol. 59, 104–110. doi: 10.1016/j.cbpa.2020.06.006
Xie, Q. Q., Lu, Y., Tang, L., Zeng, G. M., Yang, Z. H., Fan, C. Z., et al. (2020). The mechanism and application of bidirectional extracellular electron transport in the field of energy and environment. Crit. Rev. Environ. Sci. Technol. 51, 1924–1969. doi: 10.1080/10643389.2020.1773728
Xu, Y. F., Ge, Z. P., Zhang, X. Q., Feng, H. J., Ying, X. B., Huang, B. C., et al. (2019). Validation of effective roles of non-electroactive microbes on recalcitrant contaminant degradation in bioelectrochemical systems. Environ. Pollut. 249, 794–800. doi: 10.1016/j.envpol.2019.03.036
Yan, B., Ai, Y., Sun, Q., Ma, Y., Cao, Y., Wang, J., et al. (2021). Membrane damage during ferroptosis is caused by oxidation of phospholipids catalyzed by the oxidoreductases POR and CYB5R1. Mol. Cell. 81, 355–369. doi: 10.1016/j.molcel.2020.11.024
Yan, F. F., He, Y. R., Wu, C., Cheng, Y. Y., Li, W. W., and Yu, H. Q. (2013). Carbon nanotubes alter the electron flow route and enhance nitrobenzene reduction by Shewanella oneidensis MR-1. Environ. Sci. Technol. Lett. 1, 128–132. doi: 10.1021/ez4000093
Yang, C., Zhang, J. Q., Zhang, B. C., Liu, D. Y., Jia, J. C., Li, F., et al. (2022). Engineering shewanella carassii, a newly isolated exoelectrogen from activated sludge, to enhance methyl orange degradation and bioelectricity harvest. Synth. Syst. Biotechnol. 7, 918–927. doi: 10.1016/j.synbio.2022.04.010
Yang, J., Feng, L., Pi, S., Cui, D., Ma, F., Zhao, H. P., et al. (2020). A critical review of aerobic denitrification: Insights into the intracellular electron transfer. Sci. Total Environ. 731:139080. doi: 10.1016/j.scitotenv.2020.139080
Yong, X. Y., Feng, J., Chen, Y. L., Shi, D. Y., Xu, Y. S., Zhou, J., et al. (2014). Enhancement of bioelectricity generation by cofactor manipulation in microbial fuel cell. Biosens. Bioelectron. 56, 19–25. doi: 10.1016/j.bios.2013.12.058
Zhang, Y., Li, G., Wen, J., Xu, Y., Sun, J., Ning, X. A., et al. (2018b). Electrochemical and microbial community responses of electrochemically active biofilms to copper ions in bioelectrochemical systems. Chemosphere 196, 377–385. doi: 10.1016/j.chemosphere.2018.01.009
Zhang, P., Liu, J., Qu, Y. P., Li, D., He, W. H., and Feng, Y. J. (2018a). Nanomaterials for facilitating microbial extracellular electron transfer: Recent progress and challenges. Bioelectrochemistry 123, 190–200. doi: 10.1016/j.bioelechem.2018.05.005
Zhang, S. J., Shao, T., Bekaroglu, S. S. K., and Karanfil, T. (2009). The impacts of aggregation and surface chemistry of carbon nanotubes on the adsorption of synthetic organic compounds. Environ. Sci. Technol. 43, 5719–5725. doi: 10.1021/es900453e
Zhang, S., Lin, D. H., and Wu, F. C. (2016). The effect of natural organic matter on bioaccumulation and toxicity of chlorobenzenes to green algae. J. Hazard. Mater. 311, 186–193. doi: 10.1016/j.jhazmat.2016.03.017
Zhao, J., Li, F., Cao, Y., Zhang, X., Chen, T., Song, H., et al. (2021). Microbial extracellular electron transfer and strategies for engineering electroactive microorganisms. Biotechnol. Adv. 53:107682.
Zheng, T. W., Li, J., Ji, Y. L., Zhang, W. M., Fang, Y., Xin, F. X., et al. (2020). Progress and prospects of bioelectrochemical systems: Electron transfer and its applications in the microbial metabolism. Front. Bioeng. Biotechnol. 8:10. doi: 10.3389/fbioe.2020.00010
Zhou, X. W., Kang, F. X., Qu, X. L., Fu, H. Y., Liu, J., Alvarez, P. J., et al. (2020). Probing extracellular reduction mechanisms of Bacillus subtilis and Escherichia coli with nitroaromatic compounds. Sci. Total Environ. 724:138291. doi: 10.1016/j.scitotenv.2020.138291
Keywords: electroactive microorganism, toxic pollutants, toxicogenic mechanism, detoxification mechanism, extracellular electron transfer
Citation: Feng H, Xu L, Chen R, Ma X, Qiao H, Zhao N, Ding Y and Wu D (2022) Detoxification mechanisms of electroactive microorganisms under toxicity stress: A review. Front. Microbiol. 13:1084530. doi: 10.3389/fmicb.2022.1084530
Received: 30 October 2022; Accepted: 14 November 2022;
Published: 29 November 2022.
Edited by:
Lean Zhou, Changsha University of Science and Technology, ChinaReviewed by:
Fan Chen, Northwestern Polytechnical University, ChinaYang-Chun Yong, Jiangsu University, China
Jinyou Shen, Nanjing University of Science and Technology, China
Copyright © 2022 Feng, Xu, Chen, Ma, Qiao, Zhao, Ding and Wu. This is an open-access article distributed under the terms of the Creative Commons Attribution License (CC BY). The use, distribution or reproduction in other forums is permitted, provided the original author(s) and the copyright owner(s) are credited and that the original publication in this journal is cited, in accordance with accepted academic practice. No use, distribution or reproduction is permitted which does not comply with these terms.
*Correspondence: Ruya Chen, Y2hlbnJ1eWFAempnc3UuZWR1LmNu