- 1College of Animal Science and Technology, Hebei Agricultural University, Baoding, Hebei, China
- 2College of Veterinary Medicine/Traditional Chinese Veterinary Medicine, Hebei Agriculture University, Baoding, China
- 3College of Agriculture and Forestry, Linyi University, Linyi, Shandong, China
- 4Department of Anatomy, Faculty of Veterinary Science, University of Agriculture, Faisalabad, Pakistan
- 5Key Laboratory of Healthy Breeding in Dairy Cattle (Co-Construction by Ministry and Province), Ministry of Agriculture and Rural Affairs, Baoding, Hebei, China
Lycium barbarums are traditionally used as a homology of medicinal plants in China with a potent role in metabolism and immunomodulation. The current study was performed to explore the attenuation effect and microbiota regulation of Lycium barbarum polysaccharide (BLBP) on lipopolysaccharide (LPS)-induced intestine damage in mice. A total of 70 mice were randomly divided into five groups; negative control (GA), LPS (GB), both treated with an equal volume of normal saline, and BLBP treatment groups GC (100 mg/kg), GD (200 mg/kg), and GE (400 mg/kg) via gavage for 19 days. On Day 19, mice in groups GB, GC, GD, and GE were treated with 10 mg/kg LPS for 24 h and euthanized to collect intestine samples for pathological examination and microbiota sequencing. The results showed a non-significant difference in body weight gain among the five mouse groups; however, mice in the GC and GE groups showed decreased weight gain. An H&E examination revealed that the integrity of intestinal villi was destroyed by LPS, while BLBP supplement alleviated intestinal damage with an increase in villus height and a decrease in crypt depth. A total of over 59,000, 40,000, 50,000, 45,000, and 55,000 raw sequences were found in groups GA, GB, GC, GD, and GE, respectively. LPS challenge decreased alpha diversity indexes significantly (p < 0.05), while a non-significant difference was found between different BLBP treatment groups and the GA group. A total of 8 phyla and 13 genera were found among five mouse groups, and BLBP partly restored the bacterial abundance in mice. LPS changed 282 metabolic pathways in KEGG L2, 77 metabolic pathways in KEGG L3, and 205 metabolic pathways in MetaCyc, respectively. The BLBP-supplemented groups, especially GE, showed reverse effects on those metabolic pathways. The current study revealed that BLBP can effectively decrease intestinal damage through the regulation of intestinal microbiota, which may provide new insights for the prevention of intestinal disease using food and medicine homologous of Lycium ruthenicum.
Introduction
The intestine is an important organ for digestion, absorption, and immunity (Ahern and Maloy, 2020; Li et al., 2023). Any damage to the intestine commonly results in intestinal inflammation. Intestinal microbiota are composed of trillions of microorganisms, including various types of bacteria, eukaryotes, archaea, and viruses (Ahern and Maloy, 2020), which contribute greatly to the host physiology by influencing metabolism and immune modulation and affect mental and neurological functions (Honda and Littman, 2016). In the past, many diseases have been reported with microbiota dysbiosis such as inflammatory bowel diseases (Nishino et al., 2018), allergy (Bunyavanich et al., 2016), and diarrhea (Li et al., 2022).
For several millennia, goji berries or Lycium barbarums (red and black goji) have been used traditionally as a homology of medicinal and food plants in China (Stanoeva et al., 2021), which can prevent diseases like diabetes, hyperlipidemia, and hepatitis (Islam et al., 2017). Due to their health benefits and anti-aging properties, goji berries are growing frequently in western countries (Jin et al., 2013). Polysaccharides are commonly known as important functional components of goji, which present biological activities related to metabolism, antioxidant activity, and immunomodulation (Jin et al., 2013). Currently, approximately 90% of commercial goji berries are red Lycium barbarum (RLB), which is less expensive as compared to black Lycium barbarum (BLB), especially those from the Qinghai Tibetan plateau (Liu et al., 2020). There is an increasing trend in the cultivation of BLB across the world as it has high in polyphenols and is rich in antioxidants and polysaccharide contents than RLB (Ni et al., 2013; Sun et al., 2017). A previous study found that the extracts from BLB had significantly higher antioxidant and anti-inflammatory activities than RLB in lipopolysaccharides-stimulated BV2 microglial cells (Magalhães et al., 2022). Gram-negative bacteria membrane-extracted LPS is commonly known for causing oxidative damage and inflammatory reaction into host (Chen X. et al., 2022). However, information about the effect of black Lycium barbarum polysaccharide (BLBP) on LPS-induced intestine damage in mice is limited. Hence, the current study was performed to explore the attenuation effect and microbiota regulation of BLBP on LPS-induced mice.
Materials and methods
Black Lycium barbarum polysaccharide (blbp) extraction and content determination
Approximately 500 g of BLBP was purchased from Tongren Tang (Nanjing, China), and polysaccharide extraction was performed as described in previous studies (Zhao et al., 2016; Yang et al., 2018). The vacuum-dried BLBP was stored at –20°C for future use. The concentration of polysaccharides was detected by piloting the phenol-sulfuric method as described in a previous study (Liu et al., 2021).
Animal experiment design
A total of 70, 4-weeks-old Kunming mice with an equal number of male and female animals (average wight of 22 ± 2 g) were purchased from Skyford Laboratory Animal Technology Co., Ltd., China. All the mice were given 3 days to accommodate with the surroundings, and the mice were randomly divided into five groups, namely negative control (GA), lipopolysaccharide (GB), and treatment groups (GC, GD, GE). Mice in groups GC (100 mg/kg), GD (200 mg/kg), and GE (400 mg/kg) were treated by BLBP via gavage for 19 days, while mice in groups GA and GB were treated with an equal volume of normal saline. On Day 19, mice in groups GB, GC, GD, and GE were treated with 10 mg/kg LPS (Sigma-Aldrich®, Germany), and after 24 h, all mice were euthanized to collect the intestine (the duodenum, the jejunum, the ileum, the cecum, and the rectum) samples. The body weights were documented daily. All of the experimental animals used in the current study were given standard feeding in the laboratory animal center of Hebei Agricultural University.
Hematoxylin and eosin (H&E) staining
Intestinal samples from the mice of each group were collected primarily and preserved in paraformaldehyde (4.0%) for over 48 h followed by H&E staining from Pinuofei Biological Technology Co., Ltd (Wuhan, China). Olympus CX23 microscope (Olympus Co., Japan) was used for histological slide analysis. The villus height and crypt depth were recorded according to the previous study as depicted by Xu et al. (2021).
DNA extraction and sequencing
Microbial DNA from the recta of each mouse group (n = 6) were retreived through fast DNA Stool Mini Kit (Qiagen, German) guided by the instructions. DNA products’ quantity and quality were detected via NanoDrop 2000 UV–vis spectrophotometer (Thermo Scientific, USA), and were examined through agarose gel electrophoresis (0.8%). The V3–V4 regions of bacteria 16S rRNA gene were amplified using primer pairs of 338F (5′-ACTCCTACGGGAGGCAGCAG-3′) and 806R (5′-GGACTA CHVGGG TWTCTA AT-3′) as reported in the previous study (Wang et al., 2019). Then, all the reaction products were purified and quantified using commercial AxyPrep DNA Gel Extraction Kit (Axygen Biosciences, USA) and QuantiFluor™-ST (Promega, USA), respectively, according to the instructions, followed by sequencing via the Illumina MiSeq platform (Bioyi Biotechnology Co., Ltd., China).
Gut microbiota analysis
First, DADA2 and QIIME21 were utilized to get cleaned results, amplicon sequence variant (ASV) (Callahan et al., 2016), and the taxonomy table (Bokulich et al., 2018). Alpha diversity analysis among different mouse groups were performed by calculating metrics such as Chao1, Observed species, Shannon, Faith’s PD, Pielou’s evenness, and Good’s coverage, as described in the previous study (Chen X. et al., 2022). Beta diversity analysis was carried out through the analysis of principal coordinate, monomeric multidimensional scaling (Vazquez-Baeza et al., 2013), unweighted pair-group method with arithmetic, and partial least squares discriminant. Different abundance among mice groups were explored by piloting methods of ANCOM, ANOVA, Kruskal Wallis, LEFSe, and DEseq2 (Segata et al., 2011; Love et al., 2014; Mandal et al., 2015). Finally, the potential functional profiles of KEGG Ortholog of mice gut microbiota were predicted through PICRUSt annotating MetaCyc and ENZYME database (Langille et al., 2013).
Statistical analysis
All the currently obtained data were evaluated through ANOVA and Student’s t-test by employing IBM SPSS (20.0). Data were presented as means ± SD and were considered statistically significant when the p-value was < 0.05.
Results
Polysaccharide concentration, mice weights, and intestinal H&E examination
The concentration of present BLBP was 24.97% by the phenol-sulfuric method (Figure 1). There was no significant difference in the average daily weight of mice in different groups, the weight losses were slightly lower in groups GC and GE caused by the LPS challenge (Figure 2). An H&E examination revealed that the integrity of intestinal villi was destroyed by LPS, while the BLBP supplement alleviated the intestine damage. In the jejunum, the villus height in mice with the LPS challenge was significantly shorter (p < 0.0001), while the BLBP supplementation improved the villus height in mice in groups GC (p < 0.0001), GD (p < 0.0001), and GE (p < 0.0001). The crypt depth of the intestine of mice in group GB was significantly higher than that of mice in group GA (p < 0.0001) but decreased in the BLBP-supplemented groups (p < 0.0001). LPS significantly decreased the villus height and crypt depth of the intestine of mice in group GB (p < 0.01), while the BLBP supplementation significantly increased the villus height and the crypt depth of the intestine of mice in groups GC (p < 0.0001), GD (p < 0.0001), and GE (p < 0.0001). Similar results were found in the ileum of mice, and LPS challenge evidently decreased the villus height (p < 0.0001) and villus height/crypt depth (p < 0.0001) but increased crypt depth (p < 0.05) of the intestine of mice in group GB. Mice in groups GC and GE showed higher villus height (p < 0.0001) and villus height/crypt depth (p < 0.05) but lower crypt depth (p < 0.05; Figure 3).
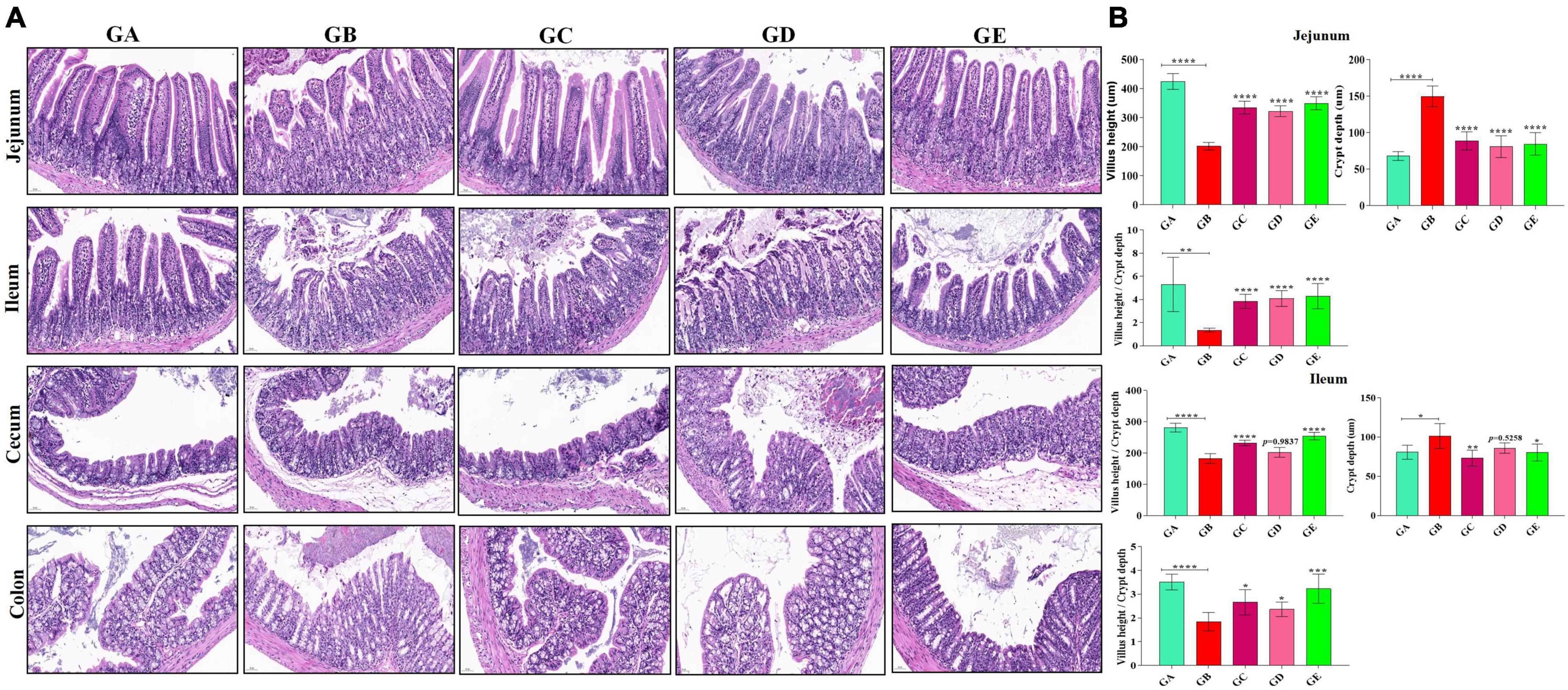
Figure 3. Effects of BLBP on the intestine of LPS-induced mice. (A) H&E staining analysis, (B) villus height, crypt depth, and villus height/crypt depth ratio. Scale bar 50 μm. Significance is presented as *p < 0.05, **p < 0.01, ***p < 0.001, and ****p < 0.0001; data are presented as the mean ± SEM (n = 6).
BLBP partly restored gut microbiota in mice induced by LPS
A total of over 59,000, 40,000, 50,000, 45,000, and 55,000 raw sequences were found in groups GA, GB, GC, GD, and GE, respectively (Table 1). More than 32,000 filtered sequences and 290,000 non-chimeric sequences were detected in all mice samples, respectively. Alpha diversity analysis was performed by examining the diversity indexes and revealed that chao1 (p < 0.05), faith_pd (p < 0.05), observed_otus (p < 0.05), and Simpson (p < 0.05) indices in group GB were lower as compared to group GA, while a non-significant difference was observed between different BLBP supplemented groups and the negative control group (GA; Figure 4). A total of 224 shared ASVs were found among all mouse groups (Figure 5A), and then, ASVs in all mouse groups were utilized for taxa analysis. At the phylum level, the dominant phyla in mice groups were Firmicutes (61.69%) and Bacteroidetes (35.30%) in GA, Proteobacteria (63.93%) and Firmicutes (21.40%) in GB, Firmicutes (52.46%) and Proteobacteria (28.58%) in GC, Proteobacteria (52.47%) and Firmicutes (27.03%) in GD, Firmicutes (67.04%) and Bacteroidetes (26.61%) in GE (Figure 5B). At the class level, Bacilli (56.06%) and Bacteroidia (35.30%) in GA, Gammaproteobacteria (58.58%) and Bacilli (18.05%) in GB, Bacilli (46.98%), Bacteroidia (14.44%), and Epsilonproteobacteria (13.00%) in GC, Gammaproteobacteria (26.47%), Bacilli (23.77%), and Epsilonproteobacteria (22.50%) in GD, and Bacilli (61.24%) and Bacteroidia (26.61%) in GE were mainly found (Figure 5C). At the order level, the primary orders in group GA were Lactobacillales (53.56%) and Bacteroidales (35.30%), in group GB were Enterobacteriales (58.58%), Lactobacillales (17.49%), and Bacteroidales (10.67%), in group GC were Lactobacillales (41.72%), Bacteroidales (14.44%), and Enterobacteriales (12.06%), in group GD were Enterobacteriales (26.45%), Lactobacillales (23.17%), and Campylobacterales (22.50%), and in group GE were Lactobacillales (53.80%) and Bacteroidales (26.61%; Figure 5D). At the family level, the highest abundance of Lactobacillaceae was found in groups GA (53.45%), GC (41.32%), and GE (53.72%), while the staple family in groups GB (58.58%) and GD (26.45%) was Enterobacteriaceae (Figure 5E). At the genus level, Lactobacillus and unclassified were the highest genera in groups GA, GC, GD, and GE, while a higher abundance of Escherichia (20.37%) was found in group GB (Figure 5F). Phylogenetic analysis of the top 50 abundant specific genera by ggtree in R showed that a high abundance of Helicobacter, Parabacteroides, Mucispirillum, and Enterococcus was uncovered in groups GB and GD, while a relatively higher abundance of Odoribacter and Clostridium was revealed in the mouse of groups GC, GD, and GE (Figure 6).

Figure 4. Alpha diversity analysis of mice gut microbiota in different groups. (A) chao1, (B) faith_pd, (C) observed_otus, (D) shannon, (E) simpson. Significance is presented as *p < 0.05; data are presented as the mean ± SEM (n = 6).
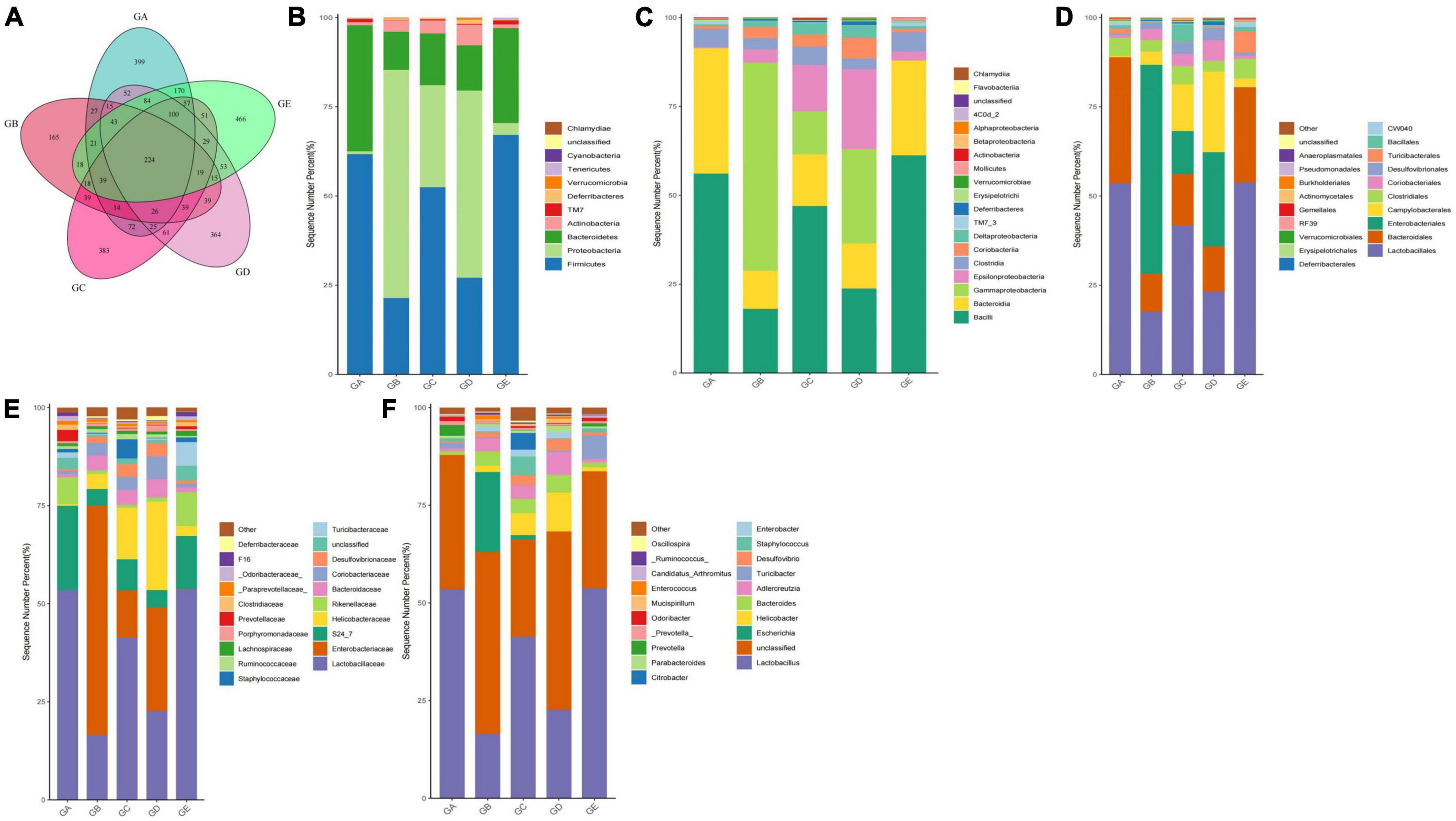
Figure 5. BLBP partly restored the gut microbiota in mice induced by LPS in different taxa. (A) Venn diagram, (B) Phylum, (C) Class, (D) Order, (E) Family, (F) Genus.
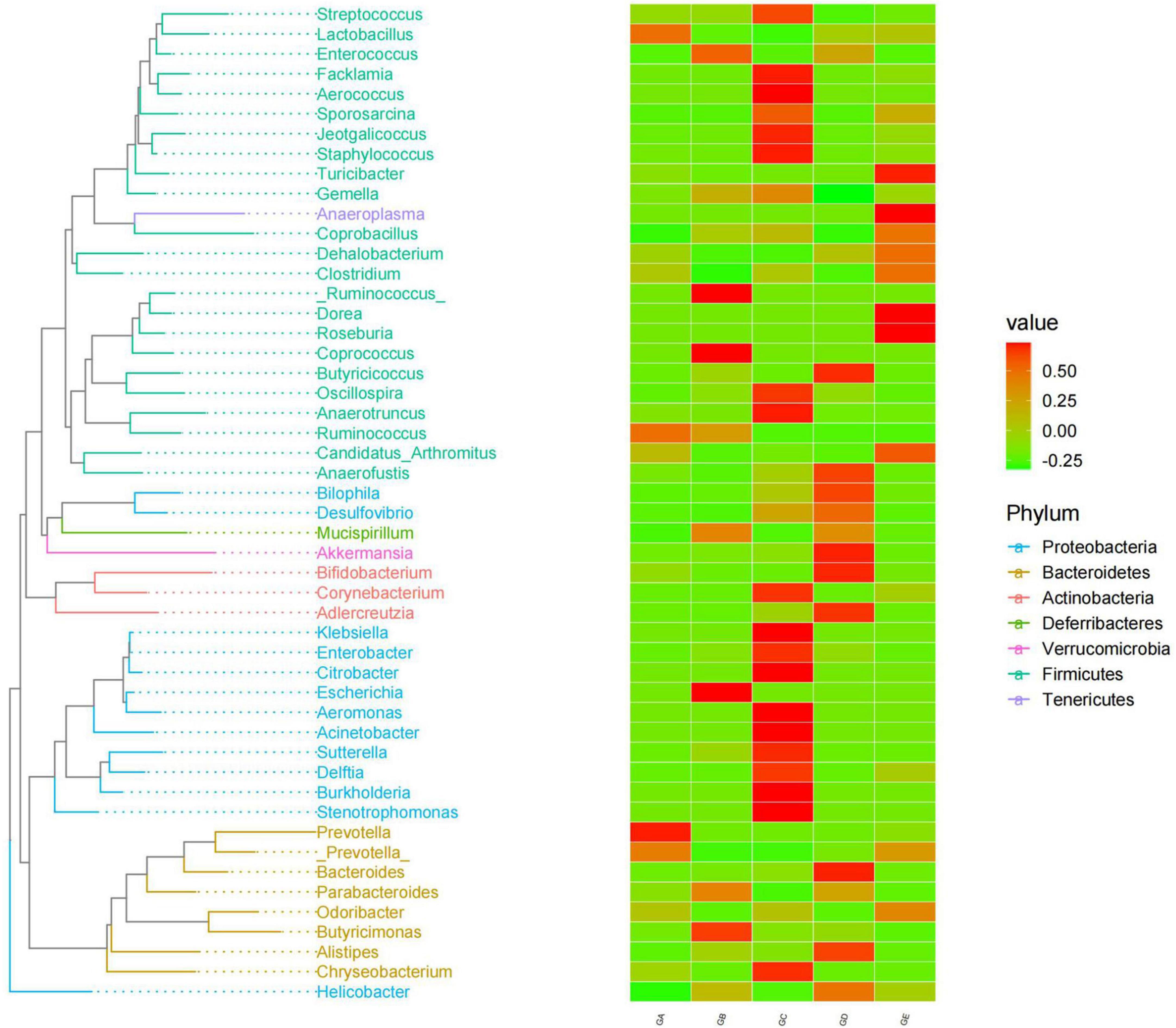
Figure 6. BLBP partly restored the gut microbiota in mice induced by LPS through phylogenetic analysis of the top 50 abundance-specific genera.
BLBP regulated remarkable species in gut microbiota in mice induced by LPS
Gut microbiota beta diversity analysis of mice in different groups through methods of heat map of diversity index (Figure 7A), PCoA (Figure 7B), NMDS (Figure 7C), PLS-DA (Figure 7D), and PCA demonstrated that species diversity difference in group GB compared with groups GA and GE, respectively (Figure 7E). To further reveal the remarkable species in mice gut microbiota, we performed a species comparison analysis. LEFSe analysis found that higher abundance of g_Prevotella, f_Prevotellaceae, g_Odoribacter, f_Odoribacteraceae, g_Gemella, f_Gemellaceae, o_Gemellales, g_Lactobacillus, f_Lactobacillaceae, g_Weissella, f_Leuconostocaceae, and o_Lactobacillales in mice in group GA, g_Enterococcus, f_Enterococcaceae, g_Escherichia, f_Enterobacteriaceae, o_Enterobacteriales, c_Gammaproteobacteria, and p_Proteobacteria in mice in group GB, g_Corynebacterium, f_Corynebacteriaceae, o_Actinomycetales, g_Jeotgalicoccus, g_Sphingomonas, f_Sphingomonadaceae, and o__Sphingomonadales in mice in group GC, g_Adlercreutzia, f_Coriobacteriaceae, o_Coriobacteriales, c_Coriobacteriia, p_Actinobacteria, g_Mucispirillum, f_Deferribacteraceae, o_Deferribacterales, c_Deferribacteres, p_Deferribacteres, g_Helicobacter, f_Helicobacteraceae, o_Campylobacterales, c_Epsilonproteobacteria, and g_Enterobacter in mice in group GD, and g_Turicibacter, f_Turicibacteraceae, o_Turicibacterales, c_Bacilli, g_Anaerostipes, p_Firmicutes, g_Anaeroplasma, f_Anaeroplasmataceae, o_Anaeroplasmatales, c_Mollicutes, and p_Tenericutes in mice in group GE (Figure 8A). Similar biomarkers were also detected by the LDA diagram (Figure 8B). The higher abundance of f_Lactobacillaceae, g_Lactobacillus, o_Lactobacillales, g_Weissella, f_Leuconostocaceae, g_Prevotella, f_Prevotellaceae, g_Odoribacter, f_Odoribacteraceae, o_Gemellales, f_Gemellaceae, and g_Gemella in mice in group GA, p_Proteobacteria, f_Enterobacteriaceae, o_Enterobacteriales, c_Gammaproteobacteria, g_Escherichia, g_Enterococcus, and f_Enterococcaceae in mice in group GB, o_Sphingomonadales, f_Sphingomonadaceae, g_Sphingomonas, g_Jeotgalicoccus, f_Corynebacteriaceae, g_Corynebacterium, and o__Actinomycetales in mice in group GC, c_Epsilonproteobacteria, f_Helicobacteraceae, o_Campylobacterales, g_Helicobacter, o_Coriobacteriales, g_Adlercreutzia, f_Coriobacteriaceae, c_Coriobacteriia, p_Actinobacteria, g_Enterobacter, o_Deferribacterales, g_Mucispirillum, p_Deferribacteres, f_Deferribacteraceae, c_Deferribacteres, g_Anaerofustis, and f_Eubacteriaceae in mice in group GD, and c_Bacilli, p_Firmicutes, g_Turicibacter, f_Turicibacteraceae, o_Turicibacterales, g_Anaerostipes, g_Anaeroplasma, f_Anaeroplasmataceae, c_Mollicutes, p_Tenericutes, and o_Anaeroplasmatales in mice in group GE.
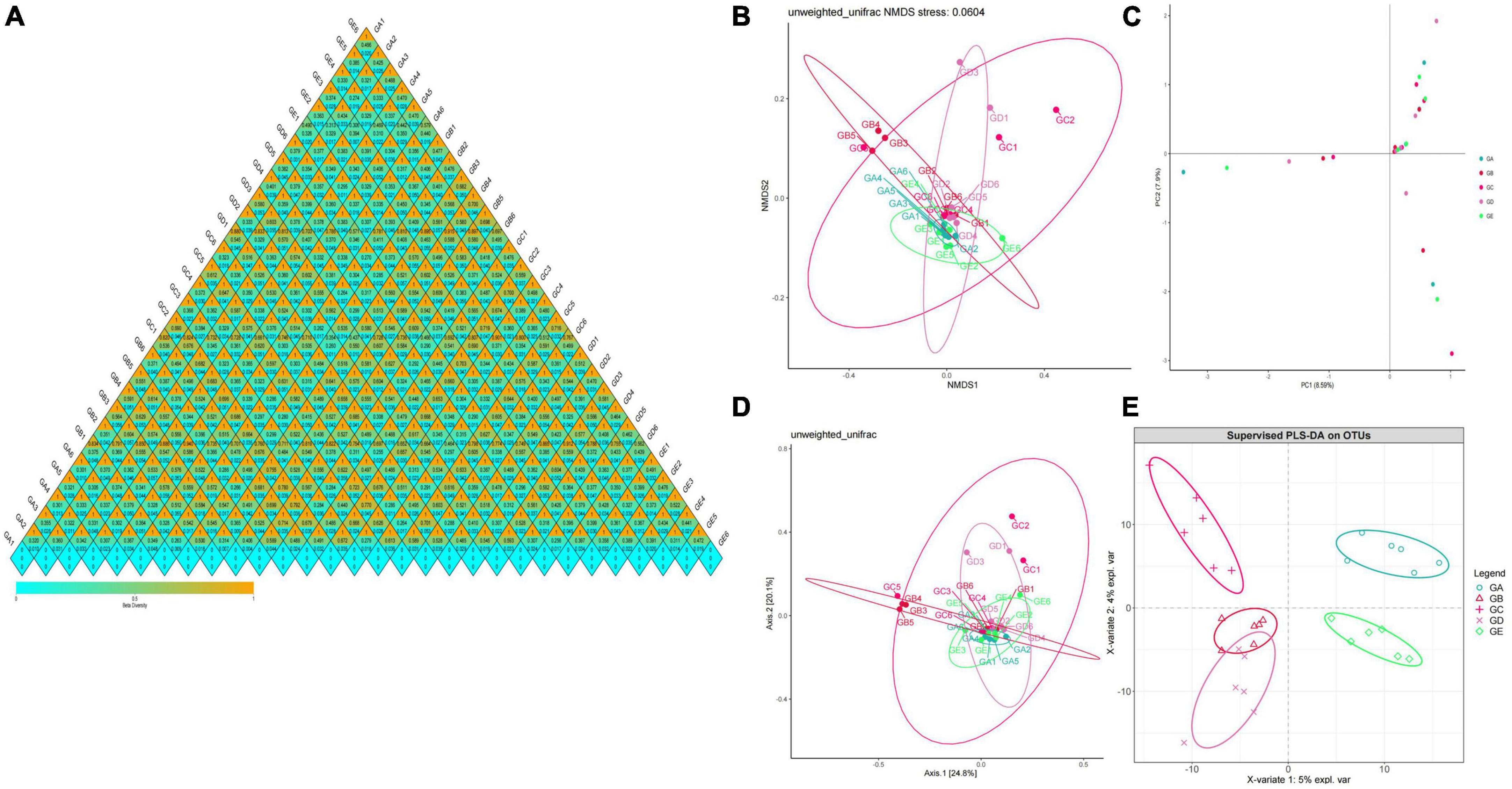
Figure 7. Beta diversity analysis of mice gut microbiota in different groups. (A) A heatmap of diversity index, (B) PCoA, (C) NMDS, (D) PLS-DA, (E) PCA.
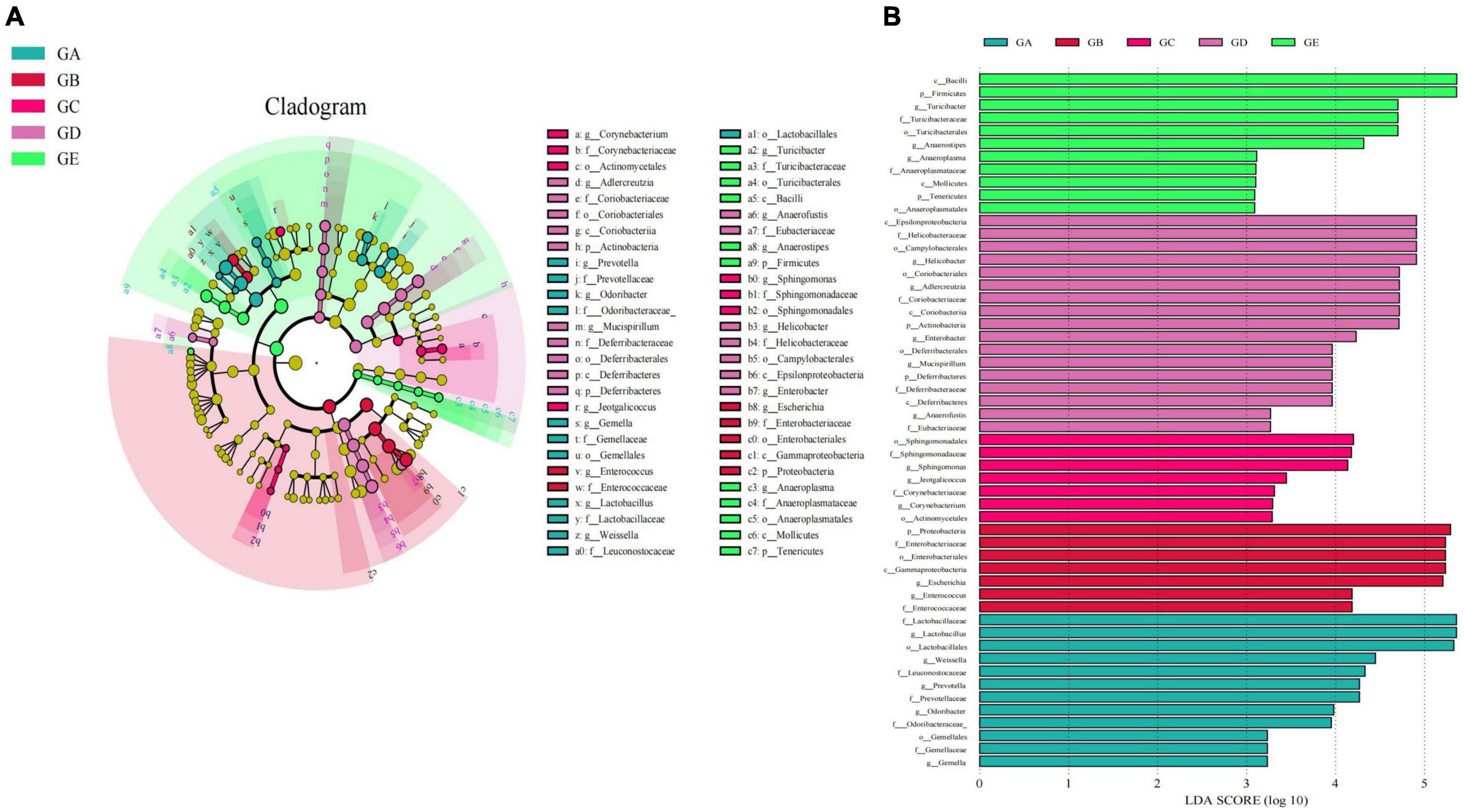
Figure 8. LEFSe analysis of significant difference species at genus level in mice gut microbiota. (A) Cladogram diagram, (B) LDA diagram.
To describe the effect of BLBP on regulating remarkable species in gut microbiota in mice, ANOVA analysis was performed. At the phylum level, the abundance of Actinobacteria in mice in group GA was significantly lower than that in mice in groups GC (p < 0.05) and GD (p < 0.05). The abundance of Bacteroidetes in mice in group GA was significantly higher than that in mice in groups GB (p < 0.01), GC (p < 0.01), and GD (p < 0.01). The abundance of Deferribacteres in mice in group GD was noticeably higher than in mice in group GA (p < 0.05). The abundance of Firmicutes in mice in groups GB (p < 0.01) and GD (p < 0.01) were significantly lower than that in mice in group GA, while the abundance of Firmicutes in mice in groups GC (p < 0.05) and GE (p < 0.0001) were significantly higher than that in mice in group GB. The abundance of Proteobacteria in mice in group GB was significantly higher than that in mice in groups GA (p < 0.0001), GC (p < 0.01), and GE (p < 0.0001). The abundance of TM7 in mice in group GA was significantly higher than that in mice in groups GB (p < 0.05) and GD (p < 0.05). The abundance of Tenericutes was found to be significantly lower in mice in groups GB (p < 0.01), GC (p < 0.05), and GD (p < 0.05) but higher in mice in group GE (Figure 9). At the genus level, Corynebacterium (p < 0.01), Odoribacter (p < 0.01), and Lactobacillus (p < 0.001) were significantly higher in mice in groups GA and GB, while Butyricimonas (p < 0.01) was higher only in mice in group GB (Figure 10).
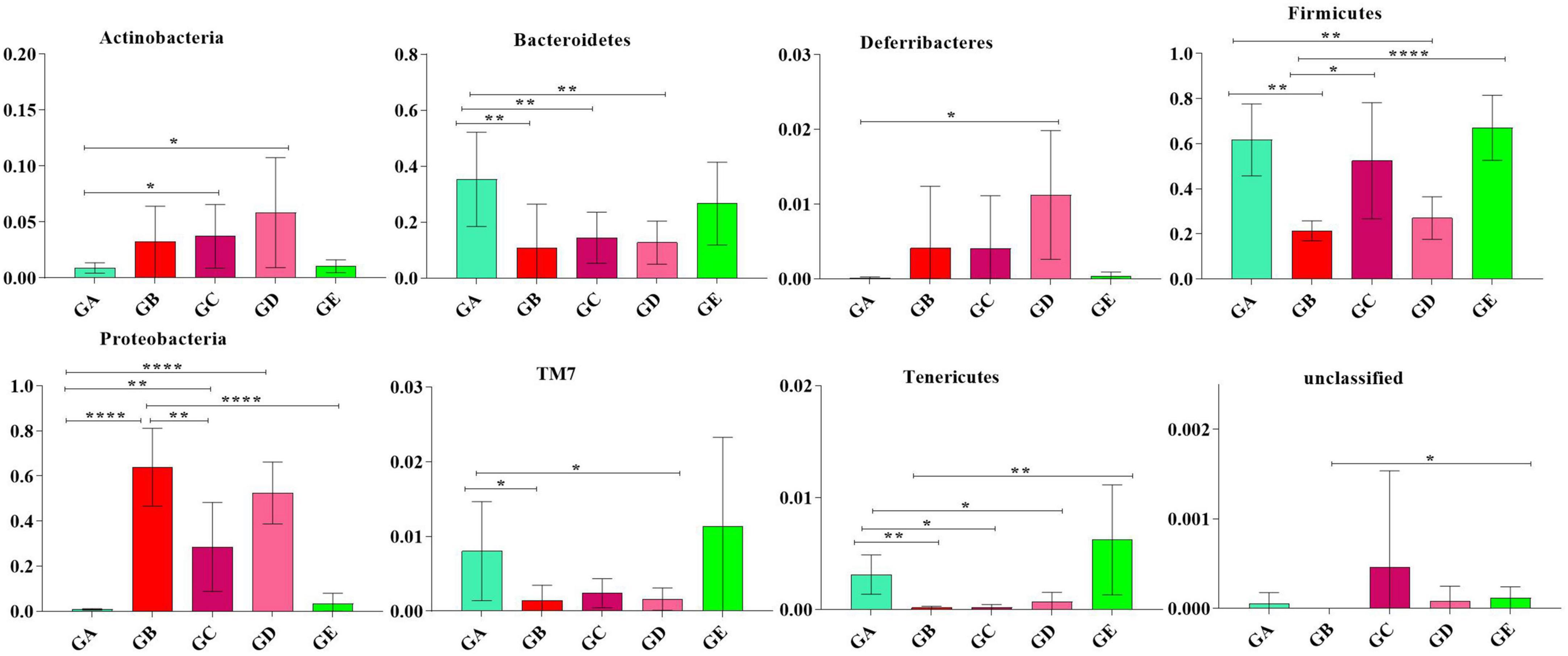
Figure 9. ANOVA analysis of remarkable species in gut microbiota at the phylum level in mice. Significance is presented as *p < 0.05, **p < 0.01, and ****p < 0.0001; data are presented as the mean ± SEM (n = 6).
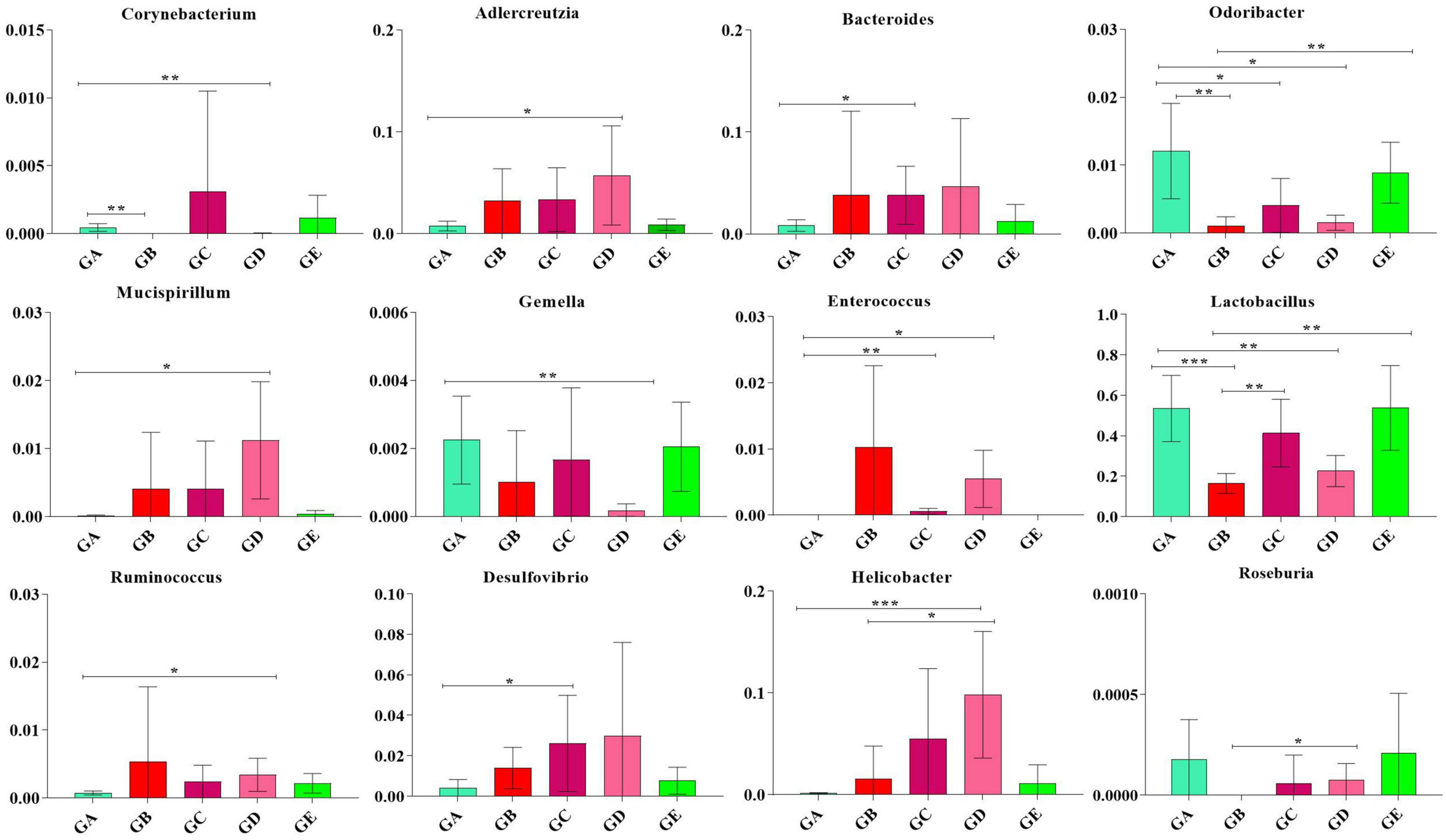
Figure 10. ANOVA analysis of remarkable species in gut microbiota at the genus level in mice. Significance is presented as *p < 0.05, **p < 0.01, and ***p < 0.001; data are presented as the mean ± SEM (n = 6).
BLBP affected gut microbiota function in mice induced by LPS
Species interaction network diagram analysis found that the bacterial interaction was positively related to Lactobacillus, Facklamia, Anaerofustis, Dehalobacterium, and Anaerotruncus but negatively related to Mucispirillum, Gemella, Jeotgalicoccus, Streptococcus, and Enterobacter (Figure 11). KEGG L1 analysis revealed that the LPS challenge significantly affected the microbiota function of the cellular processes (p < 0.0001), the environmental information processes (p < 0.0001), the genetic information processes (p < 0.0001), the human diseases (p < 0.01), and the organismal systems (p < 0.01). The BLBP supplementation in mice in groups of GC and GE reversed the effect, especially in GE mice (Figure 12A). KEGG L2 analysis showed that the LPS significantly changed cell growth and death (p < 0.0001), cell motility (p < 0.0001), cellular community-prokaryotes (p < 0.0001), membrane transport (p < 0.001), signal transduction (p < 0.0001), folding, sorting, and degradation (p < 0.01), replication and repair (p < 0.0001), transcription (p < 0.0001), translation (p < 0.0001), drug resistance: antimicrobial (p < 0.01), drug resistance: antineoplastic (p < 0.0001), infectious disease: bacterial (p < 0.0001), amino acid metabolism (p < 0.05), biosynthesis of other secondary metabolites (p < 0.0001), carbohydrate metabolism (p < 0.01), energy metabolism (p < 0.001), glycan biosynthesis and metabolism (p < 0.05), lipid metabolism (p < 0.0001), and xenobiotic biodegradation and metabolism (p < 0.001), and the BLBP supplementation in groups GC and GE partly reversed the effect, especially in the GE group (Figure 12B). KEGG L3 analysis revealed that the significant changes of bacterial chemotaxis (p < 0.01), biofilm formation-Escherichia coli (p < 0.0001), bacterial secretion system (p < 0.0001), protein processing in the endoplasmic reticulum (p < 0.0001), DNA replication (p < 0.0001), bacterial invasion of epithelial cells (p < 0.01), and Staphylococcus aureus infection (p < 0.01) in mice induced by the LPS, while the BLBP supplementation partly reversed the effect, especially in GE mice (Figure 12C). MetaCyc analysis described that mice treated with the LPS showed visible differences in the signaling pathways of 1CMET2-PWY (p < 0.0001), ALL-CHORISMATE-PWY (p < 0.0001), ARG + POLYAMINE-SYN (p < 0.0001), and so forth, while the BLBP supplementation in mice in the GC and GE groups partly reversed the effect, especially in mice in the GE group (Figure 12D).
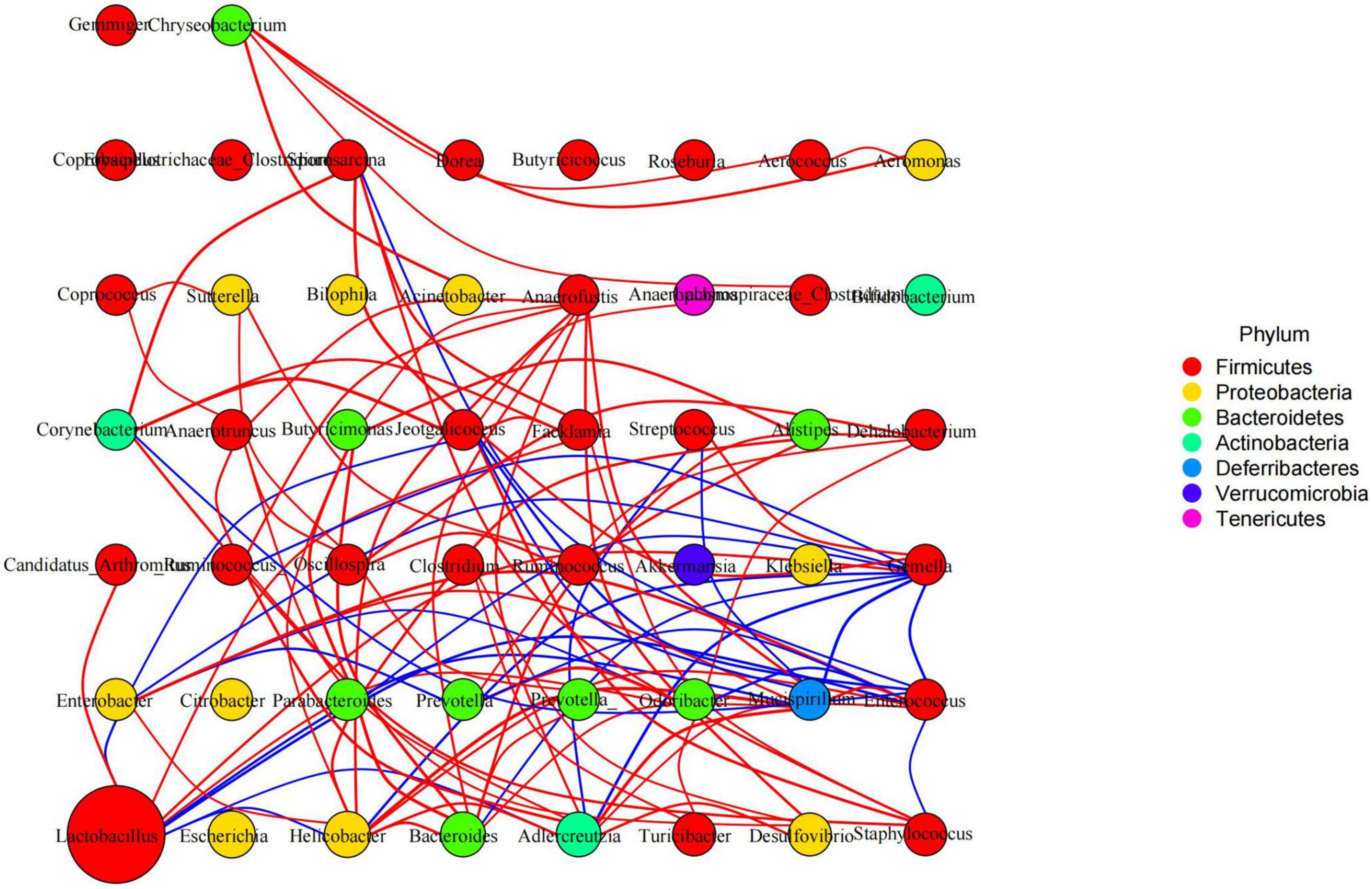
Figure 11. Species interaction network diagram of mice gut microbiota at the genus level. Red represents a positive correlation, blue represents a negative correlation.
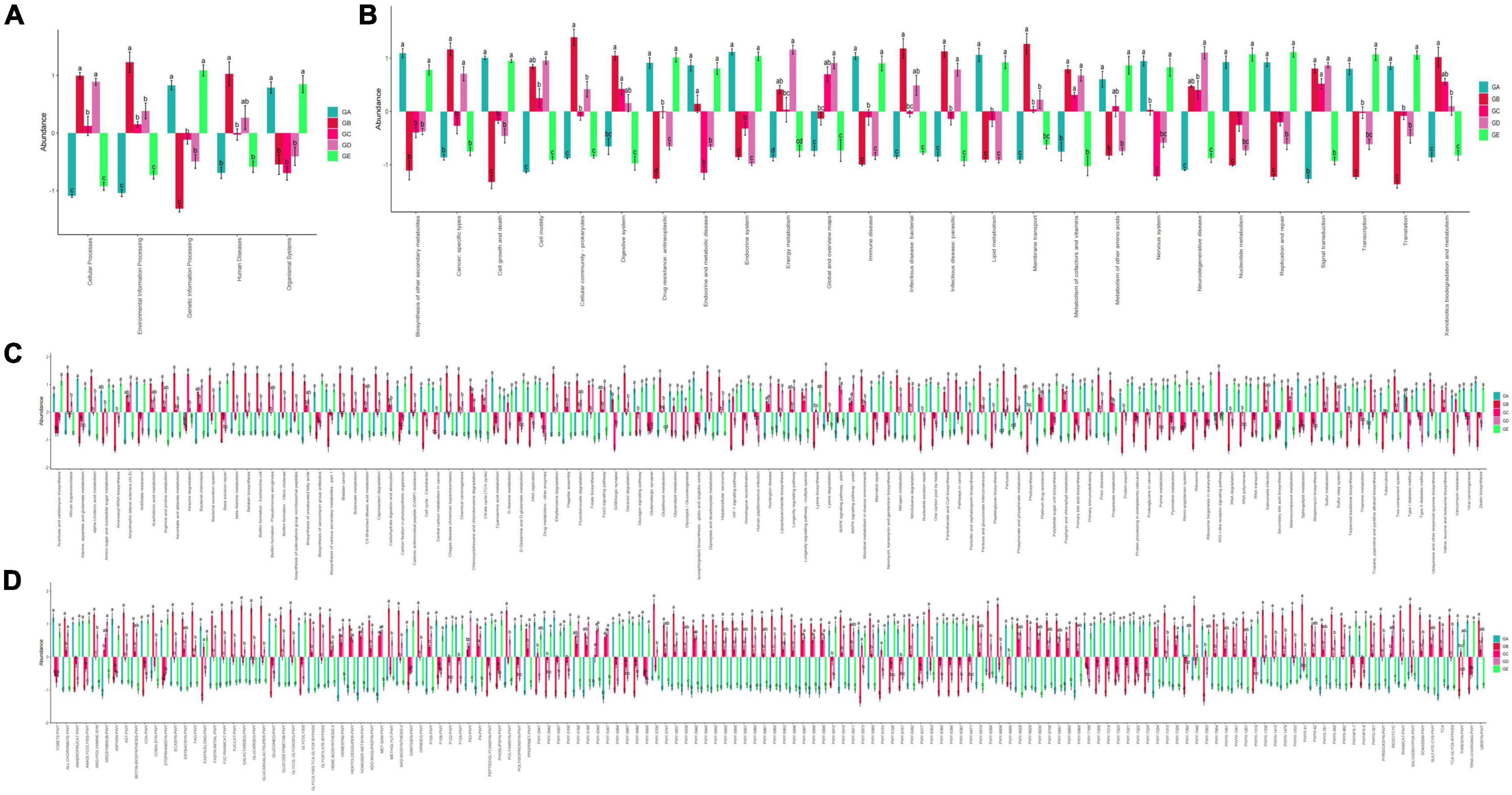
Figure 12. Function predicting analysis of mice gut microbiota. (A) KEGG L1, (B) KEGG L2, (C) KEGG L3, (D) MetaCyc.
Discussion
Many studies found that “microbiota repair” could alleviate diseases through complete fecal communities, probiotics, synbiotics, and herbal extracts (Blanton et al., 2016; Ahern and Maloy, 2020; Gomaa, 2020; Lv et al., 2022). Lycium barbarum is a popular fruit and functional medicinal plant (Zhao et al., 2020), which has anti-oxidative, anti-inflammatory, and anti-aging functions. Besides, it regulates gut microbiota (Mocan et al., 2014) and antagonizes the LPS-induced inflammation by possibly altering the glycolysis of macrophages (Ding et al., 2021). Intestinal inflammation and oxidative damage are widely detected in different diseases, whereas the functional characteristic of Lycium barbarum makes it a potential therapy for intestinal damage.
In the current study, we explored the effect of BLBP on the LPS-induced damage and intestinal flora disturbance in mice by piloting 16s rRNA sequencing, which is an important and widely utilized method in characterizing microbiota (Wong et al., 2022). The results showed that the BLBP supplement could regulate the weight losses caused by the LPS, especially in mice in groups GC and GE (Figure 2). Intestinal integrity damage and broken villus with significantly shorter villus heights and higher crypt depths were seen in LPS-exposed mice similar to what was reported by previous studies (Duan et al., 2018; Wang et al., 2021; Chen X. et al., 2022). It was found that the treatment with BLBP could mediate intestinal damage in mice, especially in mice from groups GC and GD (Figure 3). To uncover the potential relationship with the intestinal microbiota, we performed 16s rRNA sequencing and achieved a total of 1,689,473 raw and 1,399,690 filtered sequences in this study. LPS-induced changes also decreased the alpha diversity indexes (p < 0.05), while no significant differences were found among the different BLBP treatment groups (Figure 4), which demonstrated that the BLBP could reverse the decreasing trend of species diversity caused by LPS. Further analysis revealed that the BLBP supplement could partly restore the primary and remarkable species in gut microbiota in mice induced by LPS through analysis of ASVs taxa (Figure 5), phylogenetic tree (Figure 6), and LEFSe (Figure 8). Those results revealed that the BLBP could relieve intestinal damage through “microbiota repair.” Alteration of intestinal flora will affect its function; hence, we preformed the functional predicting analysis of mice and found that the LPS changed 282 metabolic pathways in KEGG L2, 77 metabolic pathways in KEGG L3, and 205 metabolic pathways in MetaCyc, respectively; the reverse effects on those metabolic pathways were watched in mice with the treatment of BLBP, especially in mice in group GE (Figure 12).
In total, 8 phyla and 13 genera were found among the current five mouse groups through ANOVA analysis (Figures 9, 10). Cani and Delzenne (2010) reported that gut microbiota is critical for numerous aspects of host physiology and contributes effectively to regulating metabolism, development, and immunity besides their potent role in regulating inflammation and pain. In comparison to bacterial species (genus) content, Adlercreutzia was identified with the LPS-induced mice in this study. The high concentration of BLBP-treated mice in group GD showed a similar abundance of Adlercreutzia. Jin and Zhang (2020) reported lower proportions of Adlercreutzia in high-fat diet mice, proposing its role in intestinal susceptibility toward carcinogens. However, Dekker Nitert et al. (2020) reported the presence of Adlercreutzi in the intestine along with back pain issues, showing a robust relationship with the pathogenesis of back pain. It is assumed that the mere presence or absence of Adlercreutzia rather than its abundance (% age) drives the impact associated with the presence of this particular genus. A slight increase in Bacteroides, Mucispirillum, opportunistic pathogenic Enterococcus, Ruminococcus, and pro-inflammatory bacteria of Desulfovibrio and Helicobacter wwas found in LPS-induced mice, which was in accordance with the results found in dogs with mammary tumor (Zheng et al., 2022), mice with colitis (Lee et al., 2022), patients with atrial fibrillation (Huang et al., 2022), people with obseity (Xu et al., 2022), and in high-fat diet-induced obesity mice. Mice in group GE demonstrated a decrease in those genera to the same level as control in mice in group GA, which may explain that BLBP mediates intestinal damage via a decrease of those relatively negative bacteria in mice. The abundance of Gemella and beneficial bacteria of Roseburia in presently used mice were slightly lower in LPS-induced mice, which was in line with infants with food allergy and atopic dermatitis (Łoś-Rycharska et al., 2021) and ulcerative colitis rats (Ran et al., 2022), respectively, and similar to the effect of Chinese dwarf cherry fermentation juice on ulcerative colitis rats, mice treated by BLBP show higher abundance of Gemella and Roseburia. As mentioned earlier, a lower abundance of Corynebacterium was examined in Eimeria-infected birds and a supplement of probiotic bacillus subtilis could increase the abundance of Corynebacterium (Memon et al., 2022), which was in line with the current study and found to increase Corynebacterium in BLBP-treated mice with the LPS challenge. Previous study found increased Odoribacter in fecal microbiota transplantation-treated colitis mice (Zhang et al., 2020), which was in line with LPS-induced mice, and higher concentration treatment of BLBP depicted a higher abundance of Odoribacter in mice in group GE (p < 0.01). Lactobacillus is a commonly recognized genus of probiotics, which can maintain the intestinal barrier and provide protection against inflammation (Bai et al., 2022), and a lower abundance of Lactobacillus had been found in antibiotic-associated diarrhea in mice (Chen C. et al., 2022) and ulcerative colitis mice (Hu et al., 2022). In the current study, a higher abundance of Lactobacillus was detected in mice in all BLBP-supplemented groups, especially in group GC and GE, which was in accordance with Lycium barbarum arabinogalactan treated mice with colitis (Cao et al., 2022). The current study may indicate that the LPS challenge caused intestinal damage by decreasing the abundance of Corynebacterium, Odoribacter, and Lactobacillus while BLBP could alleviate it by increasing the abundance of bacteria from those genera.
Conclusion
In conclusion, the current study shows that BLBP can effectively attenuate intestine damage through the regulation of intestinal microbiota, and Corynebacterium, Odoribacter, and Lactobacillus are important links. These findings may provide new insights in the prevention of intestinal disease using food and medicine homologous of Lycium ruthenicum. This is an exploratory study of gut microbiota influenced by BLBP intake; however, further investigations are needed to confirm our findings and to clarify the potential mechanism by which BLBP attenuates intestinal damage other than microbiota regulation to establish its role as a therapeutic agent.
Data availability statement
The datasets presented in this study can be found in online repositories. The names of the repository/repositories and accession number(s) can be found below: https://www.ncbi.nlm.nih.gov/, PRJNA889725.
Ethics statement
All the experiment operations were under the instructions and approval of Laboratory Animals Research Centre and the Ethics Committee of Hebei Agriculture University.
Author contributions
AY and YS: research idea and methodology. AY, HD, and JL: reagents, materials, and analysis tools. AY, CB, ZH, and ZW: writing – original draft and preparation. YS, SN, and SL: writing – review and editing. YS and SL: visualization and supervision. All authors are known and approved the final article.
Funding
This current research was supported by the Joint Fund of Hebei Province (C2022204247), the Precision Animal Husbandry Discipline Group Construction Project of Hebei Agricultural University (1090064), and the Natural Science Foundation of Shandong Province: ZR2020QC184.
Conflict of interest
The authors declare that the research was conducted in the absence of any commercial or financial relationships that could be construed as a potential conflict of interest.
Publisher’s note
All claims expressed in this article are solely those of the authors and do not necessarily represent those of their affiliated organizations, or those of the publisher, the editors and the reviewers. Any product that may be evaluated in this article, or claim that may be made by its manufacturer, is not guaranteed or endorsed by the publisher.
Footnotes
References
Ahern, P. P., and Maloy, K. J. (2020). Understanding immune–microbiota interactions in the intestine. Immunology 159, 4–14. doi: 10.1111/imm.13150
Bai, Y., Ma, K., Li, J., Ren, Z., Zhang, J., and Shan, A. (2022). Lactobacillus rhamnosus GG ameliorates DON-induced intestinal damage depending on the enrichment of beneficial bacteria in weaned piglets. J. Anim. Sci. Biotechnol. 13:90. doi: 10.1186/s40104-022-00737-9
Blanton, L. V., Charbonneau, M. R., Salih, T., Barratt, M. J., Venkatesh, S., Ilkaveya, O., et al. (2016). Gut bacteria that prevent growth impairments transmitted by microbiota from malnourished children. Science 351:10. doi: 10.1126/science.aad3311
Bokulich, N. A., Kaehler, B. D., Rideout, J. R., Dillon, M., Bolyen, E., Knight, R., et al. (2018). Optimizing taxonomic classification of marker-gene amplicon sequences with QIIME 2’s q2-feature-classifier plugin. Microbiome 6:90. doi: 10.1186/s40168-018-0470-z
Bunyavanich, S., Shen, N., Grishin, A., Wood, R., Burks, W., Dawson, P., et al. (2016). Early-life gut microbiome composition and milk allergy resolution. J. Allergy Clin. Immun. 138, 1122–1130. doi: 10.1016/j.jaci.2016.03.041
Callahan, B. J., McMurdie, P. J., Rosen, M. J., Han, A. W., Johnson, A. J. A., and Holmes, S. P. (2016). DADA2: High-resolution sample inference from Illumina amplicon data. Nat. Methods 13, 581–583. doi: 10.1038/nmeth.3869
Cani, P. D., and Delzenne, N. M. (2010). Involvement of the gut microbiota in the development of low grade inflammation associated with obesity: Focus on this neglected partner. Acta Gastroenterol. Belg. 73, 267–269.
Cao, C., Wang, L., Ai, C., Gong, G., Wang, Z., Huang, L., et al. (2022). Impact of Lycium barbarum arabinogalactan on the fecal metabolome in a DSS-induced chronic colitis mouse model. Food Funct. 13, 8703–8716. doi: 10.1039/D2FO01283A
Chen, C., Guan, X., Liu, X., Zhuang, W., Xiao, Y., Zheng, Y., et al. (2022). Polysaccharides from bamboo shoot (Leleba oldhami Nakal) byproducts alleviate antibiotic-associated diarrhea in mice through their interactions with gut microbiota. Foods 11:2647. doi: 10.3390/foods11172647
Chen, X., Kong, Q. H., Zhao, X. X., Zhao, C. X., Hao, P., Irshad, I., et al. (2022). Sodium acetate/sodium butyrate alleviates lipopolysaccharide-induced diarrhea in mice via regulating the gut microbiota, inflammatory cytokines, antioxidant levels, and NLRP3/Caspase-1 signaling. Front. Microbiol. 13:1036042. doi: 10.3389/fmicb.2022.1036042
Dekker Nitert, M., Mousa, A., Barrett, H. L., Naderpoor, N., and De Courten, B. (2020). Altered gut microbiota composition is associated with back pain in overweight and obese individuals. Front. Endocrinol. 11:605. doi: 10.3389/fendo.2020.00605
Ding, H., Wang, J. J., Zhang, X. Y., Yin, L., and Feng, T. (2021). Lycium barbarum polysaccharide antagonizes LPS-induced inflammation by altering the glycolysis and differentiation of macrophages by triggering the degradation of PKM2. Biol. Pharm. Bull. 44, 379–388. doi: 10.1248/bpb.b20-00752
Duan, Y., Wang, Y., Zhang, J., Liu, Q., and Ding, X. (2018). Morphologic, digestive enzymes and immunological responses of intestine from Litopenaeus vannamei after lipopolysaccharide injection. J. Invertebr. Pathol. 153, 186–194. doi: 10.1016/j.jip.2018.03.003
Gomaa, E. Z. (2020). Human gut microbiota/microbiome in health and diseases: A review. Antonie Van Leeuwenhoek 113, 2019–2040. doi: 10.1007/s10482-020-01474-7
Honda, K., and Littman, D. R. (2016). The microbiota in adaptive immune homeostasis and disease. Nature 535, 75–84. doi: 10.1038/nature18848
Hu, X., He, X., Peng, C., He, Y., Wang, C., Tang, W., et al. (2022). Improvement of ulcerative colitis by aspartate via RIPK pathway modulation and gut microbiota composition in mice. Nutrients 14:3707. doi: 10.3390/nu14183707
Huang, K., Wang, Y., Bai, Y., Luo, Q., Lin, X., Yang, Q., et al. (2022). Gut microbiota and metabolites in atrial fibrillation patients and their changes after catheter ablation. Microbiol. Spectr. 10:e0107721. doi: 10.1128/spectrum.01077-21
Islam, T., Yu, X., Badwal, T. S., and Xu, B. (2017). Comparative studies on phenolic profiles, antioxidant capacities and carotenoid contents of red goji berry (Lycium barbarum) and black goji berry (Lycium ruthenicum). Chem. Cent. J. 11:59. doi: 10.1186/s13065-017-0287-z
Jin, H., and Zhang, C. (2020). High fat high calories diet (HFD) increase gut susceptibility to carcinogens by altering the gut microbial community. J. Cancer 11, 4091–4098. doi: 10.7150/jca.43561
Jin, M., Huang, Q., Zhao, K., and Shang, P. (2013). Biological activities and potential health benefit effects of polysaccharides isolated from Lycium barbarum L. Int. J. Biol. Macromol. 54, 16–23. doi: 10.1016/j.ijbiomac.2012.11.023
Langille, M. G. I., Zaneveld, J., Caporaso, J. G., McDonald, D., Knights, D., Reyes, J. A., et al. (2013). Predictive functional profiling of microbial communities using 16S rRNA marker gene sequences. Nat. Biotechnol. 31, 814–821. doi: 10.1038/nbt.2676
Lee, J. G., Lee, J., Lee, A., Jo, S. V., Park, C. H., Han, D. S., et al. (2022). Impact of short-chain fatty acid supplementation on gut inflammation and microbiota composition in a murine colitis model. J. Nutr. Biochem. 101:108926. doi: 10.1016/j.jnutbio.2021.108926
Li, A., Wang, Y., Kulyar, M. F., Iqbal, M., Lai, R., Zhu, H., et al. (2023). Environmental microplastics exposure decreases antioxidant ability, perturbs gut microbial homeostasis and metabolism in chicken. Sci. Total Environ. 856:159089. doi: 10.1016/j.scitotenv.2022.159089
Li, K., Zeng, Z., Liu, J., Pei, L., Wang, Y., Li, A., et al. (2022). Effects of short-chain fatty acid modulation on potentially diarrhea-causing pathogens in yaks through metagenomic sequencing. Front. Cell. Infect. Microbiol. 12:805481. doi: 10.3389/fcimb.2022.805481
Liu, B., Xu, Q., and Yujing, S. (2020). Black goji berry (Lycium ruthenicum) tea has higher phytochemical contents and in vitro antioxidant properties than red goji berry (Lycium barbarum) tea. Food Qual. Safety 4, 193–201. doi: 10.1093/fqsafe/fyaa022
Liu, Y., Ye, Y., Hu, X., and Wang, J. (2021). Structural characterization and anti-inflammatory activity of a polysaccharide from the lignified okra. Carbohyd. Polym. 265:118081. doi: 10.1016/j.carbpol.2021.118081
Łoś-Rycharska, E., Gołȩbiewski, M., Sikora, M., Grzybowski, T., Gorzkiewicz, M., Popielarz, M., et al. (2021). A combined analysis of gut and skin microbiota in infants with food allergy and atopic dermatitis: A pilot study. Nutrients 13:1682. doi: 10.3390/nu13051682
Love, M. I., Huber, W., and Anders, S. (2014). Moderated estimation of fold change and dispersion for RNA-seq data with DESeq2. Genome Biol. 15:550. doi: 10.1186/s13059-014-0550-8
Lv, W., Ma, Y., Huang, J., He, S., Li, S., Lin, J., et al. (2022). Polysaccharides derived from shenling baizhu san improve colitis via modulating tryptophan metabolism in mice. Int. J. Biol. Macromol. 222, 1127–1136. doi: 10.1016/j.ijbiomac.2022.09.246
Magalhães, V., Silva, A. R., Silva, B., Zhang, X., and Dias, A. C. P. (2022). Comparative studies on the anti-neuroinflammatory and antioxidant activities of black and red goji berries. J. Funct. Foods 92:105038. doi: 10.1016/j.jff.2022.105038
Mandal, S., Van Treuren, W., White, R. A., Eggesbø, M., Knight, R., and Peddada, S. D. (2015). Analysis of composition of microbiomes: A novel method for studying microbial composition. Microb. Ecol. Health Dis. 26:27663. doi: 10.3402/mehd.v26.27663
Memon, F. U., Yang, Y., Zhang, G., Leghari, I. H., Lv, F., Wang, Y., et al. (2022). Chicken gut microbiota responses to dietary bacillus subtilis probiotic in the presence and absence of eimeria infection. Microorganisms 10:1548. doi: 10.3390/microorganisms10081548
Mocan, A., Vlase, L., Vodnar, D., Bischin, C., Hanganu, D., Gheldiu, A., et al. (2014). Polyphenolic content, antioxidant and antimicrobial activities of Lycium barbarum L. and Lycium chinense mill. leaves. Molecules 19, 10056–10073. doi: 10.3390/molecules190710056
Ni, W., Gao, T., Wang, H., Du, Y., Li, J., Li, C., et al. (2013). Anti-fatigue activity of polysaccharides from the fruits of four Tibetan plateau indigenous medicinal plants. J. Ethnopharmacol. 150, 529–535. doi: 10.1016/j.jep.2013.08.055
Nishino, K., Nishida, A., Inoue, R., Kawada, Y., Ohno, M., Sakai, S., et al. (2018). Analysis of endoscopic brush samples identified mucosa-associated dysbiosis in inflammatory bowel disease. J. Gastroenterol. 53, 95–106. doi: 10.1007/s00535-017-1384-4
Ran, B., Guo, C., Zhang, Y., Han, C., Cao, T., Huang, H., et al. (2022). Preventive effect of Chinese dwarf cherry [(Bge.) Sok.] fermentation juice on dextran sulfate sodium-induced ulcerative colitis rats through the regulation of IgA and the intestinal immune barrier. Food Funct. 13, 5766–5781. doi: 10.1039/D1FO04218A
Segata, N., Izard, J., Waldron, L., Gevers, D., Miropolsky, L., Garrett, W. S., et al. (2011). Metagenomic biomarker discovery and explanation. Genome Biol. 12:R60. doi: 10.1186/gb-2011-12-6-r60
Stanoeva, J. P., Stefova, M., and Bogdanov, J. (2021). Systematic HPLC/DAD/MSn study on the extraction efficiency of polyphenols from black goji: Citric and ascorbic acid as alternative acid components in the extraction mixture. J. Berry Res. 11, 611–630. doi: 10.3233/JBR-210717
Sun, Y., Rukeya, J., Tao, W., Sun, P., and Ye, X. (2017). Bioactive compounds and antioxidant activity of wolfberry infusion. Sci. Rep. 7:40605. doi: 10.1038/srep40605
Vazquez-Baeza, Y., Pirrung, M., Gonzalez, A., and Knight, R. (2013). EMPeror: A tool for visualizing high-throughput microbial community data. Gigascience 2:16. doi: 10.1186/2047-217X-2-16
Wang, G., Sun, W., Pei, X., Jin, Y., Wang, H., Tao, W., et al. (2021). Galactooligosaccharide pretreatment alleviates damage of the intestinal barrier and inflammatory responses in LPS-challenged mice. Food Funct. 12, 1569–1579. doi: 10.1039/D0FO03020A
Wang, W., Zhai, S., Xia, Y., Wang, H., Ruan, D., Zhou, T., et al. (2019). Ochratoxin A induces liver inflammation: Involvement of intestinal microbiota. Microbiome 7:151. doi: 10.1186/s40168-019-0761-z
Wong, E. O., Brownlie, E. J. E., Ng, K. M., Kathirgamanathan, S., Yu, F. B., Merrill, B. D., et al. (2022). The CIAMIB: A large and metabolically diverse collection of inflammation-associated bacteria from the murine gut. Mbio 13:e0294921. doi: 10.1128/mbio.02949-21
Xu, B., Yan, Y., Yin, B., Zhang, L., Qin, W., Niu, Y., et al. (2021). Dietary glycyl-glutamine supplementation ameliorates intestinal integrity, inflammatory response, and oxidative status in association with the gut microbiota in LPS-challenged piglets. Food Funct. 12, 3539–3551. doi: 10.1039/D0FO03080E
Xu, Z., Jiang, W., Huang, W., Lin, Y., Chan, F. K. L., and Ng, S. C. (2022). Gut microbiota in patients with obesity and metabolic disorders — a systematic review. Genes Nutr. 17:2. doi: 10.1186/s12263-021-00703-6
Yang, L., Lei, L., Zhao, Q., Gao, Z., and Xu, X. (2018). Lycium barbarum polysaccharide improves the development of mouse oocytes vitrified at the germinal vesicle stage. Cryobiology 85, 7–11. doi: 10.1016/j.cryobiol.2018.10.265
Zhang, W., Zou, G., Li, B., Du, X., Sun, Z., Sun, Y., et al. (2020). Fecal microbiota transplantation (FMT) alleviates experimental colitis in mice by gut microbiota regulation. J. Microbiol. Biotechnol. 30, 1132–1141. doi: 10.4014/jmb.2002.02044
Zhao, Q., Li, J., Yan, J., Liu, S., Guo, Y., Chen, D., et al. (2016). Lycium barbarum polysaccharides ameliorates renal injury and inflammatory reaction in alloxan-induced diabetic nephropathy rabbits. Life Sci. 157, 82–90. doi: 10.1016/j.lfs.2016.05.045
Zhao, X., Guo, S., Lu, Y., Hua, Y., Zhang, F., Yan, H., et al. (2020). Lycium barbarum L. leaves ameliorate type 2 diabetes in rats by modulating metabolic profiles and gut microbiota composition. Biomed. Pharmacother. 121:109559. doi: 10.1016/j.biopha.2019.109559
Keywords: Black Lycium barbarum, polysaccharide, LPS, mouse, gut microbiota
Citation: Yan A, Ding H, Liu J, Bi C, Han Z, Wang Z, Nawaz S, Shen Y and Liu S (2023) Black Lycium barbarum polysaccharide attenuates LPS-induced intestine damage via regulation gut microbiota. Front. Microbiol. 13:1080922. doi: 10.3389/fmicb.2022.1080922
Received: 26 October 2022; Accepted: 08 December 2022;
Published: 19 January 2023.
Edited by:
Mujahid Iqbal, Cholistan University of Veterinary and Animal Sciences, PakistanReviewed by:
Ambreen Ashar, North Carolina State University, United StatesLiwei Guo, Yangtze University, China
Copyright © 2023 Yan, Ding, Liu, Bi, Han, Wang, Nawaz, Shen and Liu. This is an open-access article distributed under the terms of the Creative Commons Attribution License (CC BY). The use, distribution or reproduction in other forums is permitted, provided the original author(s) and the copyright owner(s) are credited and that the original publication in this journal is cited, in accordance with accepted academic practice. No use, distribution or reproduction is permitted which does not comply with these terms.
*Correspondence: Yizhao Shen, c2hlbnlpemhhb0AxNjMuY29t; Shudong Liu,
bGl1c2h1ZG9uZzgxOEAxNjMuY29t
†These authors have contributed equally to this work