- 1Department of Animal Science, University of California, Davis, Davis, CA, United States
- 2Department of Population Health and Reproduction, School of Veterinary Medicine, University of California, Davis, Davis, CA, United States
Our previous research reported that supplementation of Bacillus subtilis DSM 25841 promoted growth and disease resistance of weaned pigs under enterotoxigenic Escherichia coli (ETEC) challenge and its efficacy is comparable to carbadox. This follow-up study aimed to characterize the effects of ETEC infection, supplementing B. subtilis DSM 25841 or carbadox on intestinal microbiota of pigs. Forty-eight weaned pigs (6.17 ± 0.36 kg BW) were randomly allotted to one of four treatments: negative control (NC), positive control (PC), antibiotics (AGP, 50 mg/kg of carbadox), and direct fed microbials (DFM, 2.56 × 109 CFU/kg of B. subtilis). The experiment lasted 28 days with 7 days before and 21 days after first E. coli inoculation (day 0). Pigs in the PC, AGP, and DFM groups were orally inoculated with F18 ETEC for 3 consecutive days with 1010 CFU per dose per day. Fecal samples were collected on day −7, and day 7 and day 21 post inoculation, digesta samples were collected from jejunum, ileum, and distal colon on day 21 post inoculation to perform 16S rRNA sequencing. Sampling days and locations influenced (p < 0.05) Chao1 index and beta-diversity. Age increased (p < 0.05) the relative abundance of Firmicutes but decreased (p < 0.05) the relative abundance of Bacteroidetes in feces. ETEC infection increased (p < 0.05) the relative abundance of Proteobacteria in feces on day 7 post inoculation. AGP reduced (p < 0.05) relative abundance of Firmicutes and Lactobacillaceae in feces compared with PC and DFM. AGP reduced (p < 0.05) relative abundance of Bifidobacteriaceae in jejunum and ileum, while DFM reduced (p < 0.05) relative abundance of Actinomycetaceae in jejunum and Lachnospiraceae in ileum, compared with PC. Pigs fed with DFM had greater (p < 0.05) relative abundance of Ruminococcaceae, Veillonellaceae, Bifidobacteriaceae in jejunum, Lactobacillaceae in ileum and colon, and Bifidobacteriaceae in colon than pigs in AGP. Current results indicate that carbadox or B. subtilis had stronger influences on microbial diversity and composition in ileum than other intestinal segments and feces. Supplementation of B. subtilis could increase or maintain the relative abundance of beneficial bacteria in ileum compared with carbadox.
Introduction
Transitioning from farrowing to nursery stage is known to aggravate the stress of pigs raised for pork production. Newly weaned pigs can experience extreme discomfort when separated from their sows, as they are accompanied by a sudden change in diet, environment, and social life conditions (Campbell et al., 2013). Prolonged exposure to stress has shown to adversely impact the health and performance of pigs, resulting in huge economical losses. Stress can also induce microbial imbalance in the gut and increase vulnerability to pathogens (Madison and Kiecolt-Glaser, 2019). Enterotoxigenic Escherichia coli (ETEC) is an intestinal pathogenic strain that is commonly known to induce secretory diarrhea and intestinal inflammation in pigs under post-weaning stress (Lallès et al., 2004; Fairbrother et al., 2006). In-feed antibiotics, commonly known as antibiotics growth promoters (AGP), were used to apply into nursery diet to alleviate post-weaning diarrhea and to promote growth in weaned pigs (USDA, 2007). On globally estimation, pigs on average consumed approximately 172 mg of antimicrobials per kilogram body weight globally, which was greater than the amounts that cattle and chicken consumed (Boeckel et al., 2015). The global antimicrobial consumption is estimated to increase by 67% between 2010 and 2030 (Boeckel et al., 2015). The extensive use of AGP, however, may increase the chance of dispersing antimicrobial residues and the development of antimicrobial resistance in bacteria (Menkem et al., 2019; Ma et al., 2021). In addition, AGP can modify the gut microbiota and essentially kill beneficial bacteria, thus, may increase the susceptibility to other infections in weaned pigs (Looft et al., 2014). The heightening concerns toward antibiotics and AGP uses have led to the prohibited use of AGP (FDA-2011-D-0889) in livestock for growth-promoting purposes (FDA, 2013). Thus, alternatives to in-feed antibiotics are to be sought immediately.
The importance of gut microbiome in human and animal health, particularly in gut health has been largely reviewed (Kamada et al., 2013; Fouhse et al., 2016; Ke et al., 2019). During weaning, pigs were reported to have temporary loss of microbial diversity in their intestinal tracts, including a decrease in Lactobacillus spp. and an increase in a family of pathogenic bacteria such as Enterobacteriaceae (Cao et al., 2016; Gresse et al., 2017; Tian et al., 2017). The disturbance of gut microbiome, in combination with immature intestinal immunity, can lead to the expansion of enteric pathogens, such as ETEC infection. Direct-fed microbials (DFM) have been applied to nursery diets to enhance intestinal health, disease resistance, and performance (Liao and Nyachoti, 2017). DFM are categorized into three main groups, including lactic acid-producing bacteria, yeast, and Bacillus spp. Compared with other categories, Bacillus-based DFMs are spore-forming and thermostable, thus, easy to handle for feed storage and processing. Dietary supplementation of Bacillus subtilis was reported to enhance growth performance and diarrhea in nursery pigs by modulating the intestinal immunity and microbiota (Hu et al., 2014; Luise et al., 2019). In our previous studies, we observed that supplementing B. subtilis DSM 25841 reduced diarrhea and enhanced growth performance by enhancing intestinal barrier function of weaned pigs experimentally infected with ETEC F18 (Kim et al., 2019; He et al., 2020). However, the impacts of this Bacillus strain on gut microbiota of weaned pigs under post-weaning diarrhea has yet to be addressed. Thus, the major objectives of the present study included: (1) to determine the impacts of ETEC infection on fecal and intestinal microbiome of weaned pigs; and (2) to investigate the effects of supplementing B. subtilis DSM 25841 on gut microbiome of ETEC infected pigs, in comparison to antibiotics, carbadox.
Materials and methods
Animals and study design
Animal procedures were reviewed and approved by the Institutional Animal Care and Use Committee (IACUC #19322) at the University of California, Davis (UC Davis). A total of 48 weaned pigs (21 day (d) old; 6.17 ± 0.36 kg) with equal number of barrows and gilts were obtained from the Swine Teaching and Research Center and the experiment was conducted at Cole facility at UC Davis. All pigs and their sows did not receive E. coli vaccines, antibiotic injections, or antibiotics in feed prior to the experiment. After weaning, pigs were individually housed (pen size: 0.61 m × 1.22 m) and assigned into one of 4 treatment groups using a randomized complete block design with sex normalized by body weight and litter as blocks and pig as experimental unit. Four treatments were: (1) negative control (NC): control diet and without E. coli challenge, (2) positive control (PC): control diet and with ETEC challenge, (3) AGP: inclusion of 50 mg/kg carbadox and with ETEC challenge, (4) DFM: inclusion of 500 mg/kg B. subtilis DSM 25841 (2.56 × 109 CFU/kg) and with ETEC challenge. There were 12 replicate pigs per treatment. The experiment lasted 28 days with first 2 weeks as phase 1 and last 2 weeks as phase 2. Therefore, 6 diets were prepared and all diets met the current estimates for nutrient requirements for nursery pigs (NRC, 2012; Table 1).
The experiment included a 7-day habituation period and 21 days after the first ETEC F18 inoculation (day 0). Pigs in the ETEC challenge groups received 3 oral doses of ETEC F18 at 1010 CFU per dose per day, while pigs in NC group were orally inoculated with 3 ml phosphate-buffered saline per day. The ETEC F18 were cultured in Dr. Xunde Li’s lab at Western institute for Food Safety & Security at UC Davis. The bacterial strain was originally isolated from a field disease outbreak by the University of Illinois Veterinary Diagnostic Lab (isolate number: U.IL-VDL # 05–27,242) and the strain expresses heat-labile toxin, heat-stable toxin b, and Shiga-like toxins. Our previous published research confirmed the current ETEC challenge dosage induced mild diarrhea in weaned pigs (Liu et al., 2013; Kim et al., 2019). The detailed animal study procedures and data for growth performance and diarrhea were reported in He et al. (2020).
Sample collection
Prior to weaning, tail samples were collected from all piglets to assess their susceptibility to ETEC F18 using the genotyping analysis described in Kreuzer et al. (2013). All pigs used in the present study were susceptible to ETEC F18. Fresh fecal samples were collected from 7 pigs per treatment at the beginning of the experiment (day − 7), and from all pigs on day 0 before ETEC inoculation, and day 7 and day 21 post-inoculation (PI). Samples were immediately stored at −80°C until further analysis. At the termination of the experiment (day 21 PI), all pigs were euthanized. For euthanasia, pigs were anesthetized by intramuscularly injecting 1 ml mixture of telazol (100 mg) ketamine (50 mg), and xylazine (50 mg) prior to an intracardiac injection of 78 mg sodium pentobarbital (Vortech Pharmaceuticals, Ltd., Dearborn, MI) per 1 kg of body weight. Digesta was collected from the middle of jejunum (approximately equal length from pylorus to ileocecal junction), ileum (close to the ileocecal junction), and distal colon (prior to the rectum) and was immediately frozen into liquid nitrogen and stored at −80°C until further analysis. A total of 6 pigs were removed from the whole data set due to health issues after E. coli infection or as outliers, including 3 pigs from the PC group and 3 pigs from the AGP group (He et al., 2020).
Library preparation
Bacterial DNA was extracted from 154 fecal samples and 126 intestinal digesta using the Quick-DNA Fecal/Soil Microbe Kit (Zymo Research, Irvine, CA, United States) according to the manufacturer’s instructions. DNA samples was amplified by PCR at the V4 region of the 16S rRNA gene using primers 515F (5′-XXXXXXXXGTGTGCCAGCMGCCGCGGTAA-3′), which included an 8-nt poly-X sequence indicating a barcode unique to each sample followed by an 2-nt Illumina adapter (bold), and 806R (5′-GGACTACHVGGGTWTCTAAT-3′) (Caporaso et al., 2012). Samples were prepared for PCR amplification in duplicates, and each PCR reaction was comprised of 2 μl template DNA, 9.5 μl nuclease free water, 12.5 μl GoTaq 2× Master Mix (Promega, Madison, WI, United States), 0.5 μl V4 reverse primer (10 μM), and 0.5 μl barcoded forward primer (10 μM). Amplification was performed in a thermocycler with the following setting: 94°C for 3 min for initializing denaturation; followed by 35 cycles of 94°C for 45 s, 50°C for 1 min, and 72°C for 1.5 min; and 72°C for 10 min for final elongation. Agarose gel electrophoresis was used to verify the amplicon size for each sample and band brightness was observed to subjectively quantify the amount of sample to be added when pooling DNA amplication products. Pooled sample was then purified using the QIAquick PCR Purification Kit (Qiagen, Hilden, Germany) and submitted to the UC Davis Genome Center DNA Technologies Core for 250 bp paired-end sequencing on the Illumina MiSeq platform (Illumina, Inc., San Diego, CA, United States).
Microbiota analysis
Raw fastq files were first demultiplexed and barcode sequences were removed using the software saber1 and demultiplexed sequences were then imported into Quantitative Insights into Microbial Ecology 2 (QIIME2; version 2019.4; Bolyen et al., 2019, 2). Using the DADA2 plugin, primers and lower quality reads were removed and the paired end reads were denoised and merged (Callahan et al., 2016, p. 2). Chimeras were removed after merging and amplicon sequence variants (ASVs) were constructed as well. Representative sequences for each ASV were aligned using MAFFT and masked alignments were used to generate phylogenetic trees using FastTree2 (Price et al., 2010; Katoh and Standley, 2013). Python library scikit-learn was used to assign taxonomy based on representative sequences against Silva (version 138), which was pre-trained in QIIME2 and clipped to only the V4 hypervariable region and clustered at 99% sequence identity (Pedregosa et al., 2011; Quast et al., 2012; Bokulich et al., 2018).
Shannon and Chao1 indices were measured for alpha diversity by using the estimate_richness function in phyloseq (McMurdie and Holmes, 2013). To compare community composition among treatments and day or intestinal site, Bray-Curtis matrix was measured to calculate beta diversity. Relative abundance of each taxon in each sample was calculated by dividing the taxa count by total number of filtered reads within each sample.
Statistical analyzes
Files were exported from QIIME2 and imported into the R 4.1.0 for data visualization and statistical analysis (Team, 2021). All microbiota analyzes was performed using the phyloseq package and data were visualized using the ggplot2 package (Wickham, 2011). Normality and homoscedasticity were tested using the Shapiro Wilks test and Bartlett test, respectively. Linear mixed-effect model was fitted using the lme4 package with treatment and site or day and interaction as fixed effects while pig as random effect (Bates et al., 2014, p. 4). Significance of each term in the model was determined using the F-test as type 3 analysis of variance using the Anova function in the car package, followed by group comparison using the cld function in the emmeans package (Fox and Weisberg, 2018; Lenth, 2021). When normality or homoscedasticity was not observed, non-parametric test was performed using the Kruskal-Wallis sum-rank test using the agricolae package (de Mendiburu and de Mendiburu, 2019). Bray–Curtis dissimilarity was first tested for homoskedasticity using the betadisper function and the statistical significance was tested using PERMANOVA and the vegan package (Oksanen et al., 2013). Statistical significance was assessed at α = 0.05 and statistical tendency at α = 0.10, and p-values were adjusted for multiple comparisons using false discovery rate (FDR).
Results
Shifts in fecal microbiota with age
After quality filtering in QIIME2, a total of 3,908,518 filtered sequences were obtained from 273 samples. The median reads per sample was 14,338 and the total number of taxa discovered was 3,430. No significant difference in Shannon index was observed among treatments throughout the experiment (Figure 1A). Sampling days influenced (p < 0.05) Chao1 index and an increase (p < 0.05) in Chao1 index was observed in all treatments on day 0 than day − 7 (Figure 1B). Feces collected from pigs in the PC and DFM groups had decreased (p < 0.05) Chao1 index on day 7 PI, compared with feces collected on day 0. The principal coordinate analysis (PCoA) plot visualized dissimilarities between samples employing Bray-Curtis distance matrix. The samples grouped according to sampling days, as indicated by the statistical difference using adonis2 (R2 = 0.20; p < 0.05) (Figure 2A). No distinctive clusters were observed among treatments on day − 7. However, a separated cluster among treatments was observed on day 0 (R2 = 0.15; p < 0.05), day 7 PI (R2 = 0.10; p < 0.05) and day 21 PI (R2 = 0.14; p < 0.05; Figure 2B).
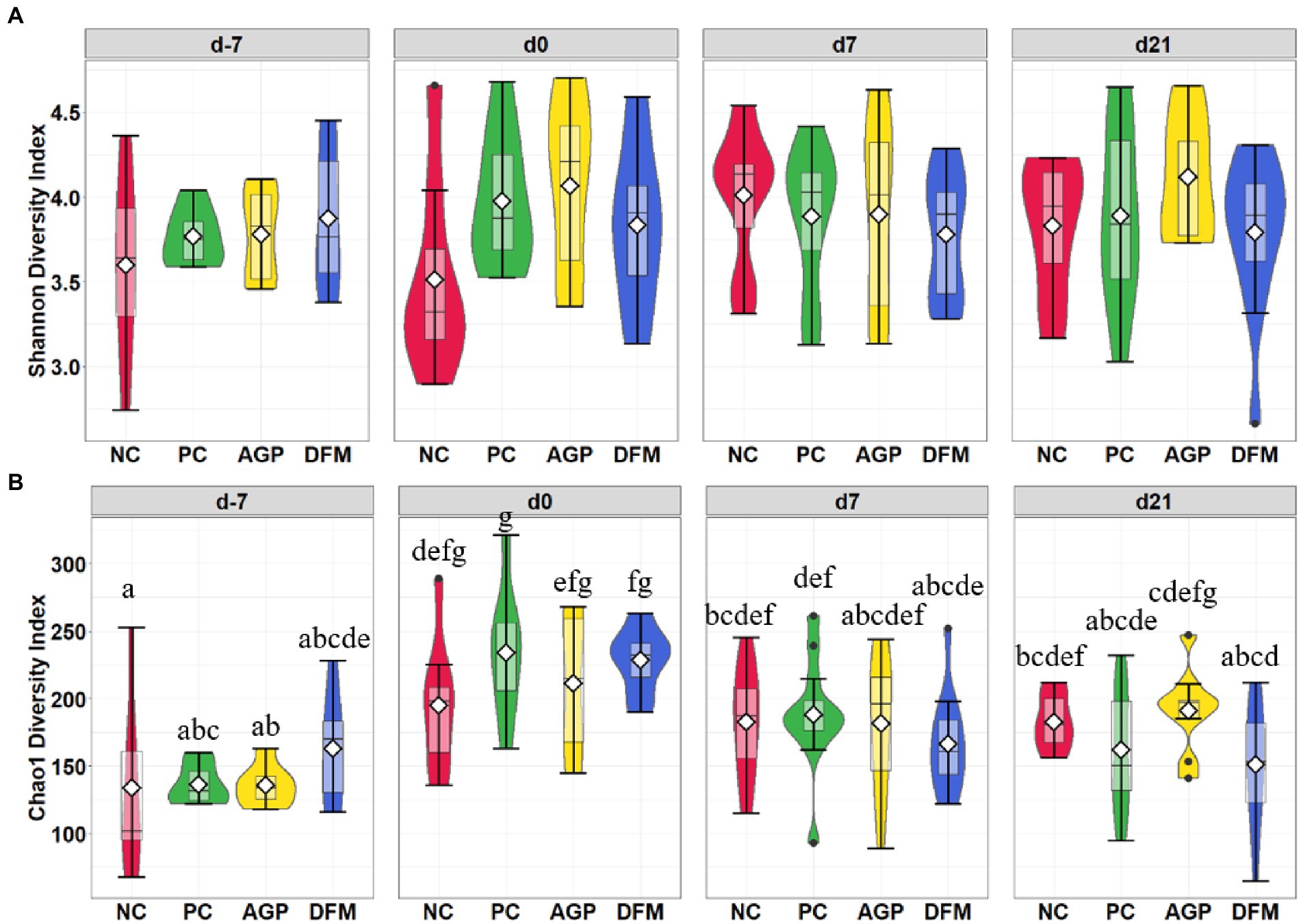
Figure 1. Alpha diversity as indicated by Shannon (A) and Chao1 (B) indices in feces collected from enterotoxigenic Escherichia coli challenged pigs fed diets supplemented with antibiotics (AGP) or Bacillus subtilis (DFM) at the beginning of the experiment, on day 0 before inoculation (day − 7), on day 7 and 21 post-inoculation. No difference was observed in Shannon (A). NC = negative control, PC = positive control, AGP = antibiotics, DFM = B. subtilis. Violin plots are colored by diet. Data are expressed as mean (diamond) ± SEM. a-gMeans without a common superscript are different (p < 0.05). Each mean represents 7 observations on day −7 and each mean represents 9–12 observations on day 0 and day 7 and 21 post-inoculation.
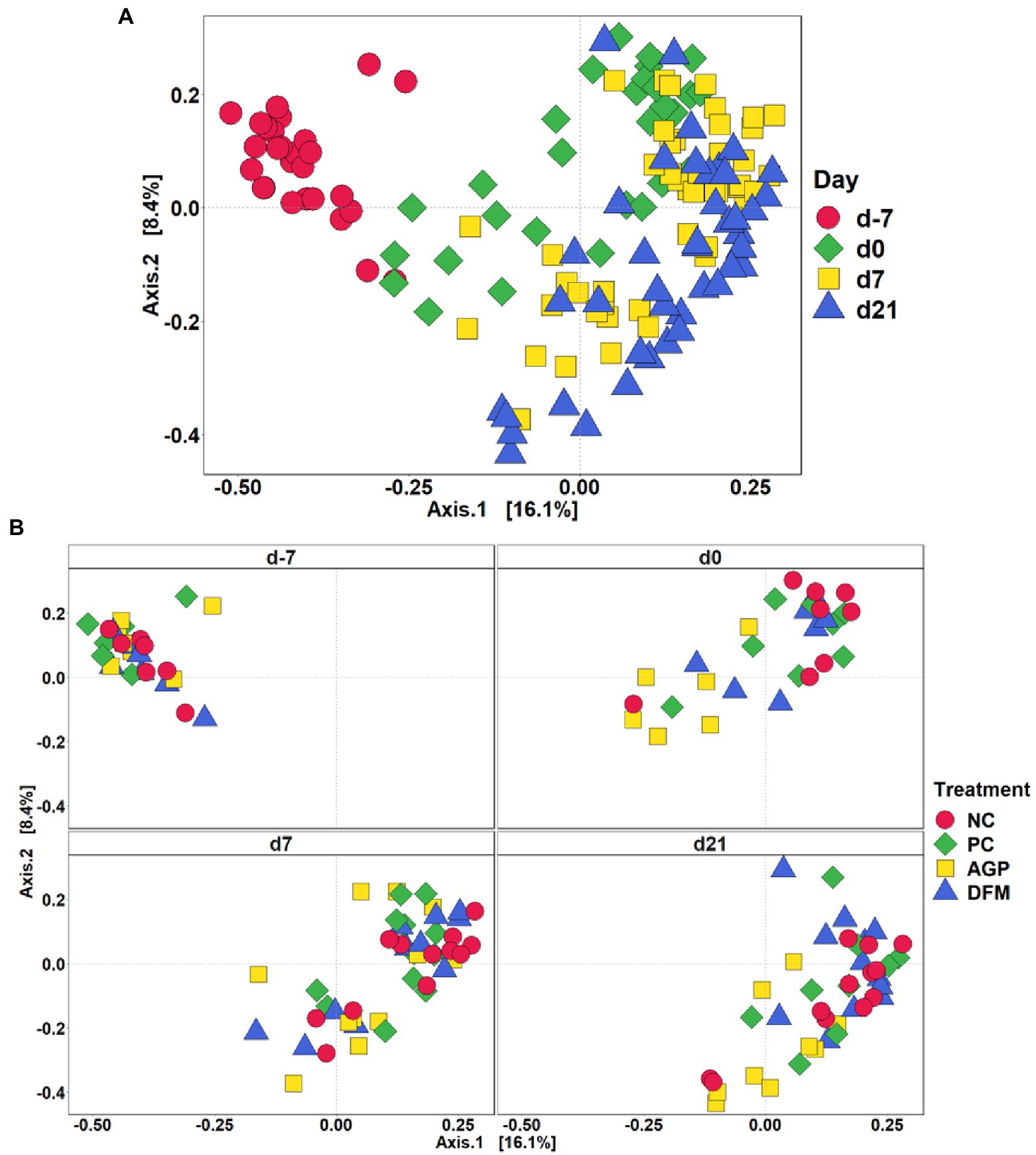
Figure 2. Beta diversity of fecal microbiota in enterotoxigenic E. coli F18 challenged pigs at the beginning of the experiment, on day 0 before inoculation, on day 7 and 21 post-inoculation by day (A) and treatment (B). Data were analyzed by principal coordinate analysis (PCoA) based on the Bray–Curtis dissimilarity. NC = negative control, PC = positive control, AGP = antibiotics, DFM = B. subtilis. Each mean represents 7 observations on day −7 and each mean represents 9–12 observations on day 0 and day 7 and 21 post-inoculation.
Relative abundances of various phyla and families are presented in Table 2. Firmicutes and Bacteroidetes were the two most abundant phyla in feces throughout the experiment, accounting for more than 75% in relative abundance per treatment group on each sampling day. No difference was observed in the relative abundance of top 6 phyla in feces among all treatments on day − 7. The relative abundance of Firmicutes was increased (p < 0.05), while the relative abundance of Bacteroidetes was decreased (p < 0.05) in NC as the pig age was increased. No difference was observed in the relative abundance of Spirochaetes, Actinobacteria, and Euryarchaeota among treatments and sampling days. On day 0 and day 7 PI, pigs in NC and DFM had greater (p < 0.05) relative abundance of Firmicutes in feces than pigs in PC and AGP. At the concluding day of the experiment (day 21 PI), pigs in the AGP group had lower (77.22% vs. 82.36%, p < 0.05) relative abundance of Firmicutes in feces than pigs in NC. No difference was observed in the relative abundance of Bacteroidetes between NC and PC on any sampling date. The relative abundance of Bacteroidetes was lower (10.03% vs. 18.31%, p < 0.05) in feces of pigs fed with DFM than pigs supplemented with AGP on day 7 PI. Pigs in PC had greater (p < 0.05) relative abundance of Proteobacteria in feces than pigs in NC on day 7 PI. No difference was observed in the relative abundance of Proteobacteria between AGP and DFM throughout the experiment.
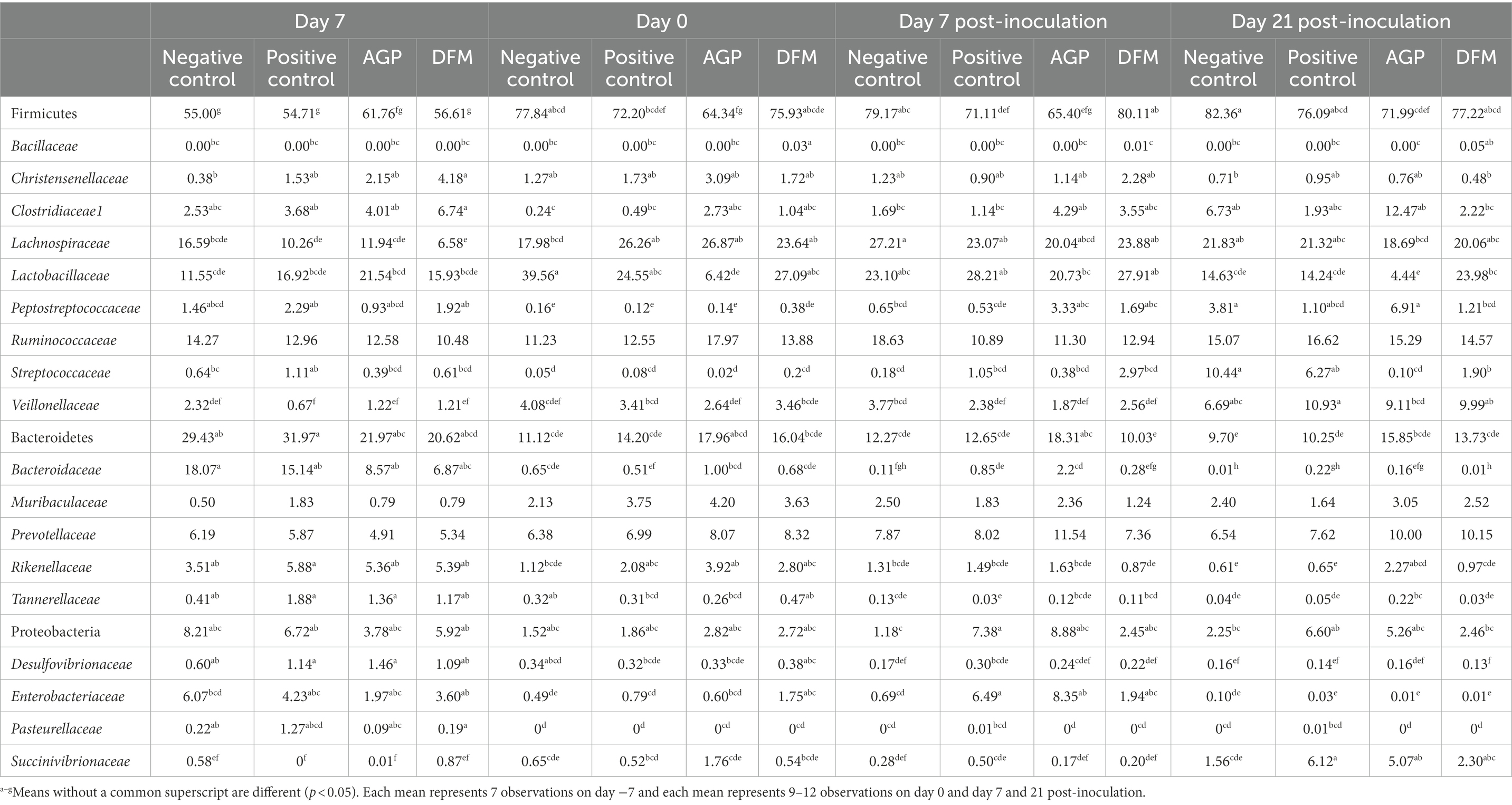
Table 2. Relative abundance (%) of Firmicutes, Bacteroidetes, and Proteobacteria and their top families in feces of enterotoxigenic Escherichia coli challenged pigs fed diets supplemented with antibiotics (AGP) or Bacillus subtilis (DFM).
Within Firmicutes phylum, the relative abundance of Bacillaceae was the greatest (p < 0.05) in DFM among all treatment on day 0, 7, and 21 PI. The relative abundance of Lactobacillaceae in NC was increased (p < 0.05) in feces on day 0 but decreased (p < 0.05) on day 21 PI, compared with feces collected on day − 7 (Table 2). In addition, pigs supplemented with AGP had reduced (p < 0.05) relative abundance of Lactobacillaceae in feces on day 0 and day 21 PI. The relative abundance of Lactobacillaceae was lower (p < 0.05) in feces of pigs fed with AGP than that in DFM (4.44% vs. 23.98%) on day 21 PI. Pigs in PC, AGP, and DFM had increased (p < 0.05) relative abundance of Lachnospiraceae from day − 7 to 0. Bacteroidaceae was the most abundant family in Bacteroidetes phylum on day − 7, while Prevotellaceae was the most abundant one on day 0, 7, and day 21 PI (Table 2). Pigs in AGP had greater (p < 0.05) relative abundance of Bacteroidaceae in feces than pigs in NC and DFM on day 7 and day 21 PI. Within Proteobacteria phylum, the relative abundance of Enterobacteriaceae was decreased (p < 0.05) but the relative abundance of Succinivibrionaceae was increased (p < 0.05), as pig age was increased (Table 2). Pigs in PC and AGP had greater (p < 0.05) relative abundance of Enterobacteriaceae than NC on day 7 PI. However, no difference was observed in the relative abundance of Enterobacteriaceae among other treatments on day 7 PI and other sampling dates. The relative abundance of Succinivibrionaceae was higher (p < 0.05) in PC and AGP than pigs in NC on day 21 PI.
The top 11 abundant genera in feces are presented in Figure 3. From day − 7 to day 21 PI, all treatments had increased (p < 0.05) relative abundance of Blautia and reduced (p < 0.05) relative abundance of Bacteroides and Escherichia-Shigella. All treatments, except AGP, had reduced (p < 0.05) relative abundance of Megasphaera from day −7 to day 21 PI. The most abundant genus was Lactobacillus. In NC pigs, the relative abundance of Lactobacillus was increased (p < 0.05) from day −7 to 0, and then decreased (p < 0.05) by day 21 PI. Pigs in AGP had the lowest abundance (p < 0.05) of Lactobacillus on day 0 and pigs in DFM had the greatest abundance (p < 0.05) of Lactobacillus on day 21 PI among treatments. In addition, the relative abundance of Lactobacillus was greater in DFM (27.91% vs. 20.73%, p < 0.05) than in AGP on day 7 PI. The relative abundance of Blautia was greater (p < 0.05) in feces collected on day 0, 7 PI, and day 21 PI than in feces collected on day −7. The relative abundance of Bacteroides was greater (p < 0.05) in AGP than in PC on day 0 and was greater (p < 0.05) in AGP than in DFM on day 7 and day 21 PI.
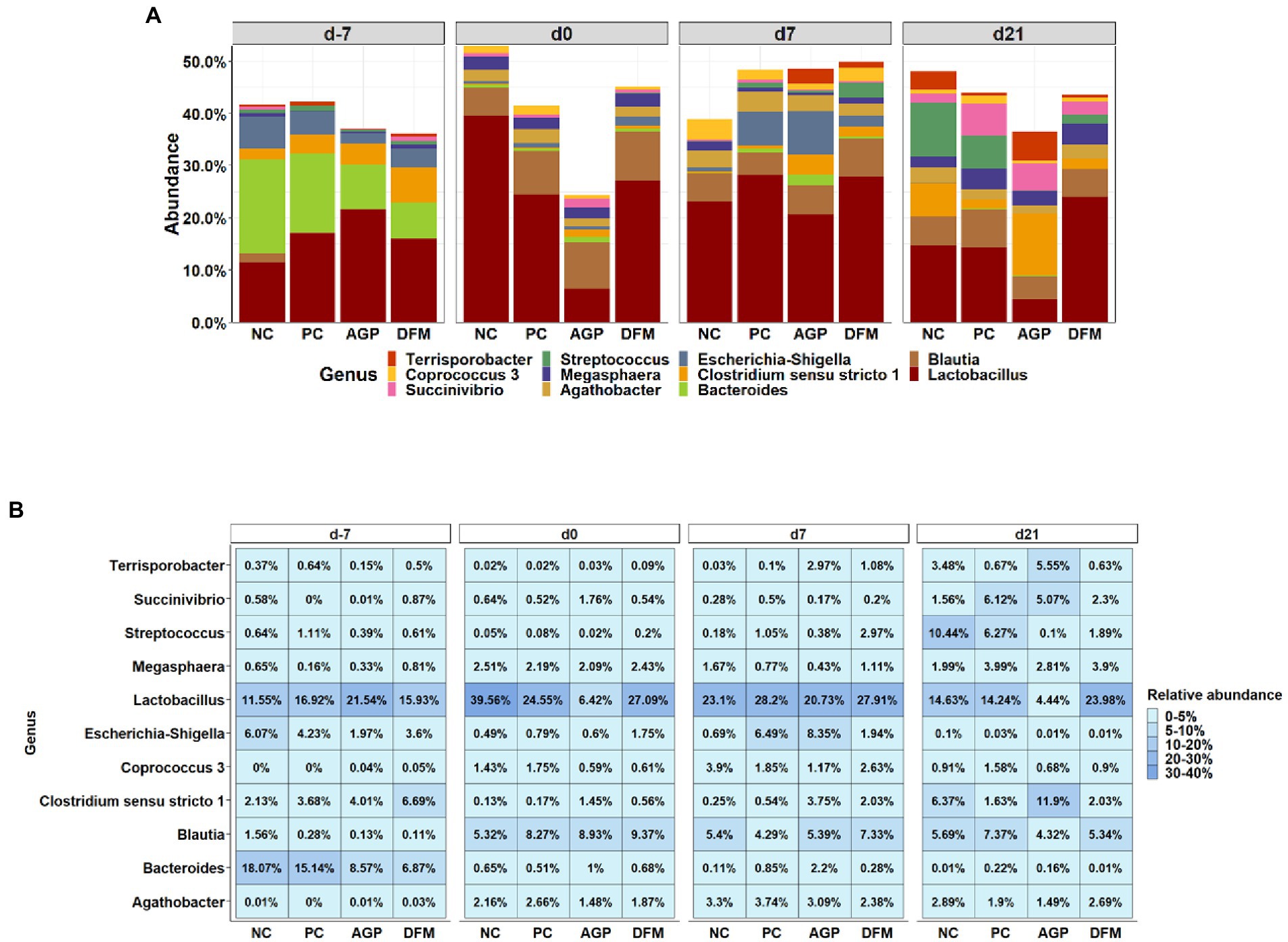
Figure 3. Relative abundance of genus that were most abundant in fecal microbiota of piglets visualized in bar plot (A) and heatmap (B). Each mean represents 7 observations on day −7 and each mean represents 9–12 observations on day 0 and day 7 and 21 post-inoculation.
Shifts in gut microbiota within different intestinal sites
The microbial composition in the digesta of jejunum, ileum, and colon was also investigated at the conclusion of this experiment (day 21 PI). Significant interaction between treatment and intestinal site was observed (p < 0.001) in both Shannon and Chao1 diversities (Figure 4). Shannon and Chao1 indices in colon digesta were greater (p < 0.05) than in jejunal and ileal digesta. No difference was observed in Shannon and Chao1 indices between NC and PC in any of intestinal sites. Pigs supplemented with AGP had greater (p < 0.05) Shannon index than other treatments in the ileum (Figure 4A) and had greater (p < 0.05) Chao1 index in the jejunum than NC (Figure 4B). Supplementation of DFM reduced (p < 0.05) Chao 1 index in ileal digesta when compared with NC.
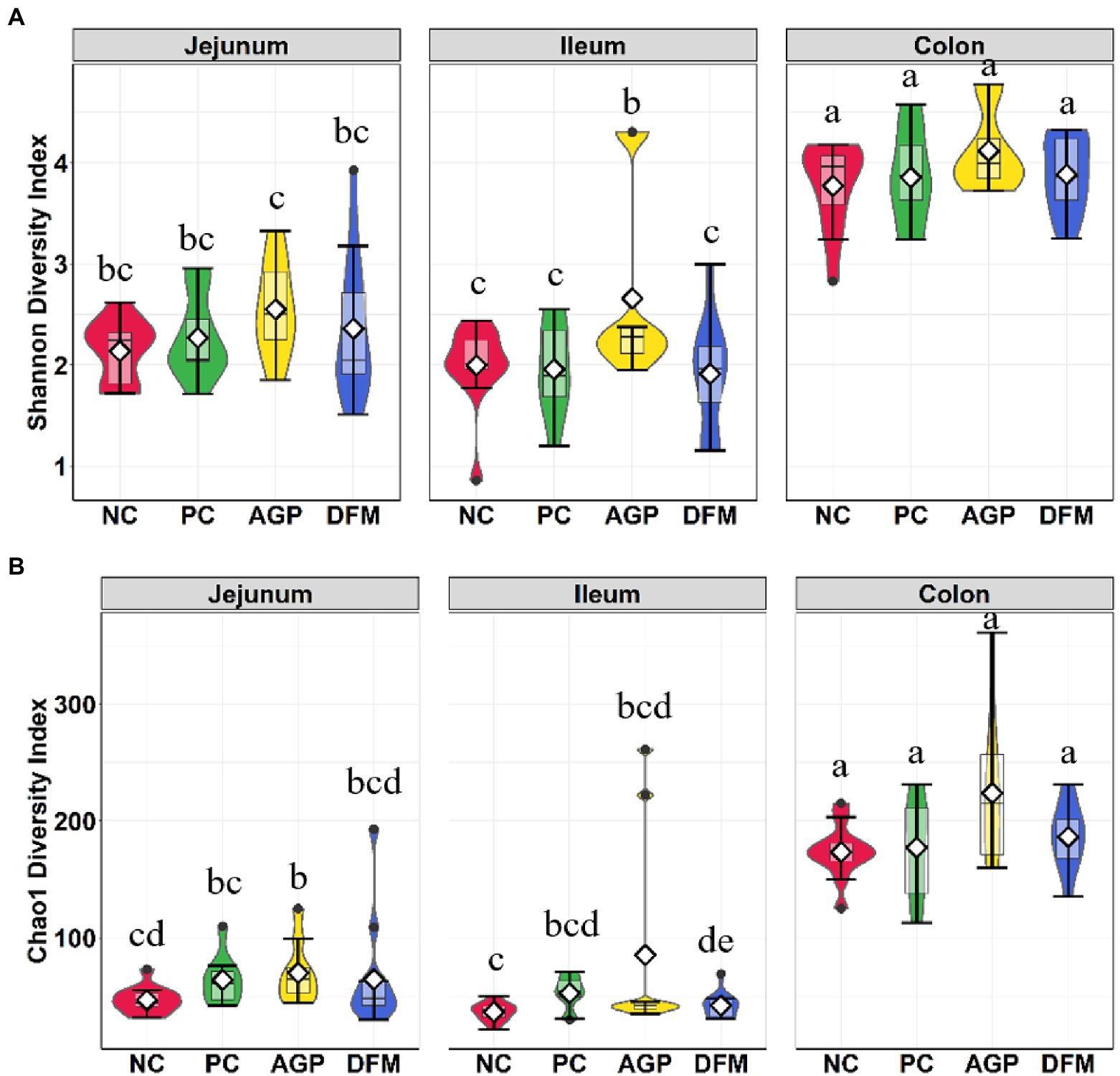
Figure 4. Alpha diversity as indicated by Shannon (A) and Chao1 (B) indices in intestinal digesta collected from weaned pigs challenged with enterotoxigenic E. coli. Pigs were supplemented with antibiotics (AGP) or B. subtilis (DFM). NC = negative control, PC = positive control. Violin plots are colored by diet. Data are expressed as mean (diamond) ± SEM. a-gMeans without a common superscript are different (p < 0.05). Each mean represents 9–12 observations.
The adonis2 test demonstrated significance in treatment (R2 = 0.10, p < 0.05), intestinal site (R2 = 0.18, p < 0.05), and treatment and intestinal site interaction (R2 = 0.05, p < 0.05). In Figure 5A, all colon samples were clustered together and were separated from jejunal and ileal samples, whereas clusters for ileum and jejunum were indistinguishable from each other. No clear separation was observed among treatments in jejunum, while the AGP cluster was distinct from DFM cluster in ileum (Figure 5B). Within colon samples, AGP cluster partially overlapped with DFM cluster, while NC and PC clusters were overlapping with each other.
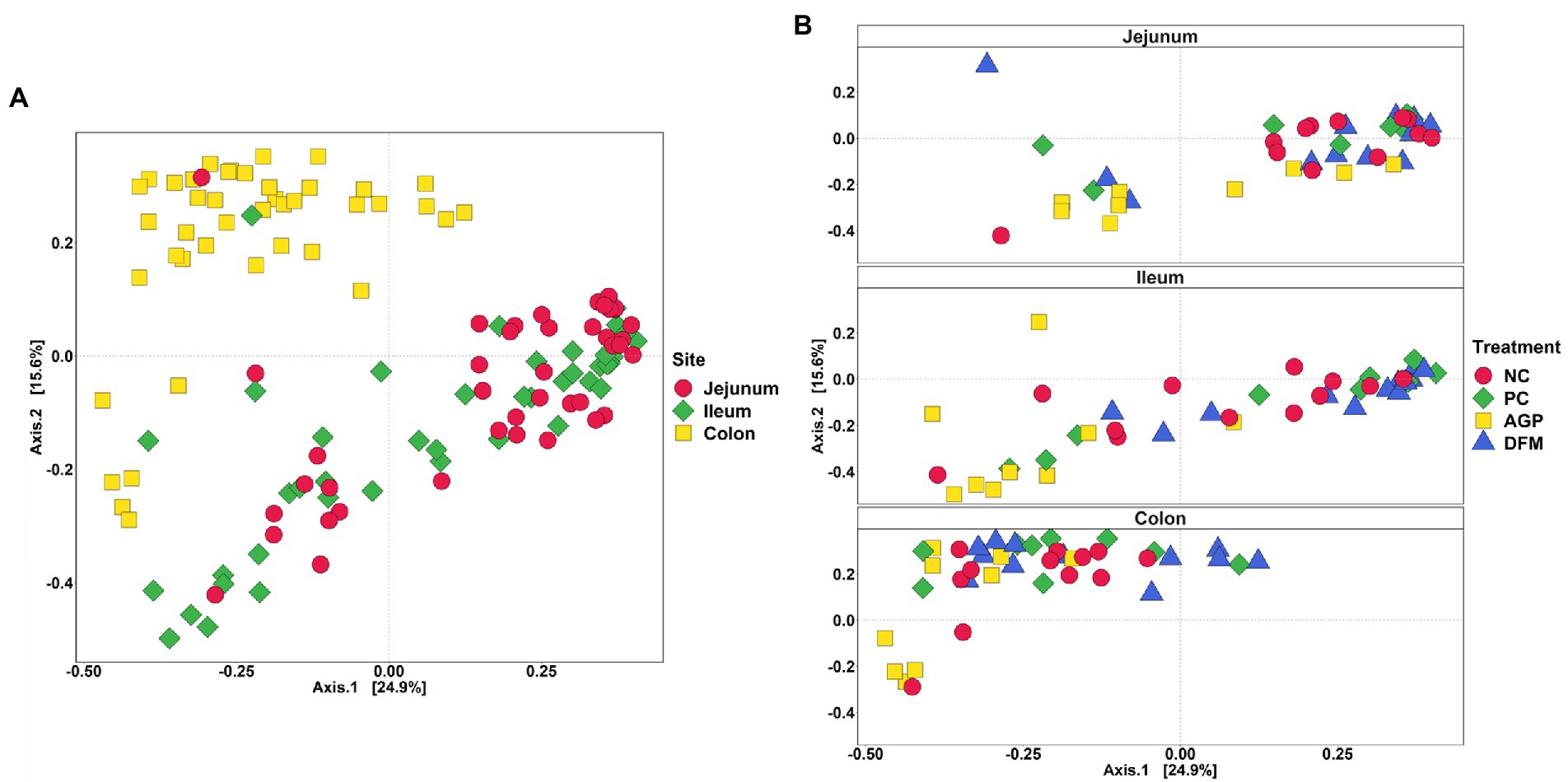
Figure 5. Beta diversity of intestinal digesta microbiota in pigs challenged with enterotoxigenic E. coli by intestinal site (A) and treatment (B). Data were analyzed by principal coordinate analysis (PCoA) based on Bray–Curtis dissimilarity. NC = negative control, PC = positive control, AGP = antibiotics, DFM = B. subtilis. Each mean represents 9–12 observations.
The relative abundance of phyla, families, and genera are presented in Table 3. Firmicutes, Bacteroidetes, Actinobacteria, and Proteobacteria were the top four abundant phyla in the intestinal tract of weaned pigs. Firmicutes was the most abundant phylum in jejunum, ileum, and colon in all pigs. Unlike fecal samples, Bacteroidetes was second most abundant phylum in colon, Actinobacteria was the second most abundant phylum in jejunum, and Proteobacteria was the second most abundant phylum in ileum. No difference was observed in the relative abundance of phyla between NC and PC in all intestinal sites. The relative abundance of Bacteroidetes in ileal digesta was greater (p < 0.05) in AGP than DFM. The relative abundance of Actinobacteria in ileal digesta was greater (p < 0.05) in PC than AGP. Pigs in AGP had the greatest (p < 0.05) relative abundance of Proteobacteria in jejunal digesta among all treatments and had greater (p < 0.05) relative abundance of Proteobacteria in ileal digesta than pigs in DFM (4.12% vs. 0.22%).
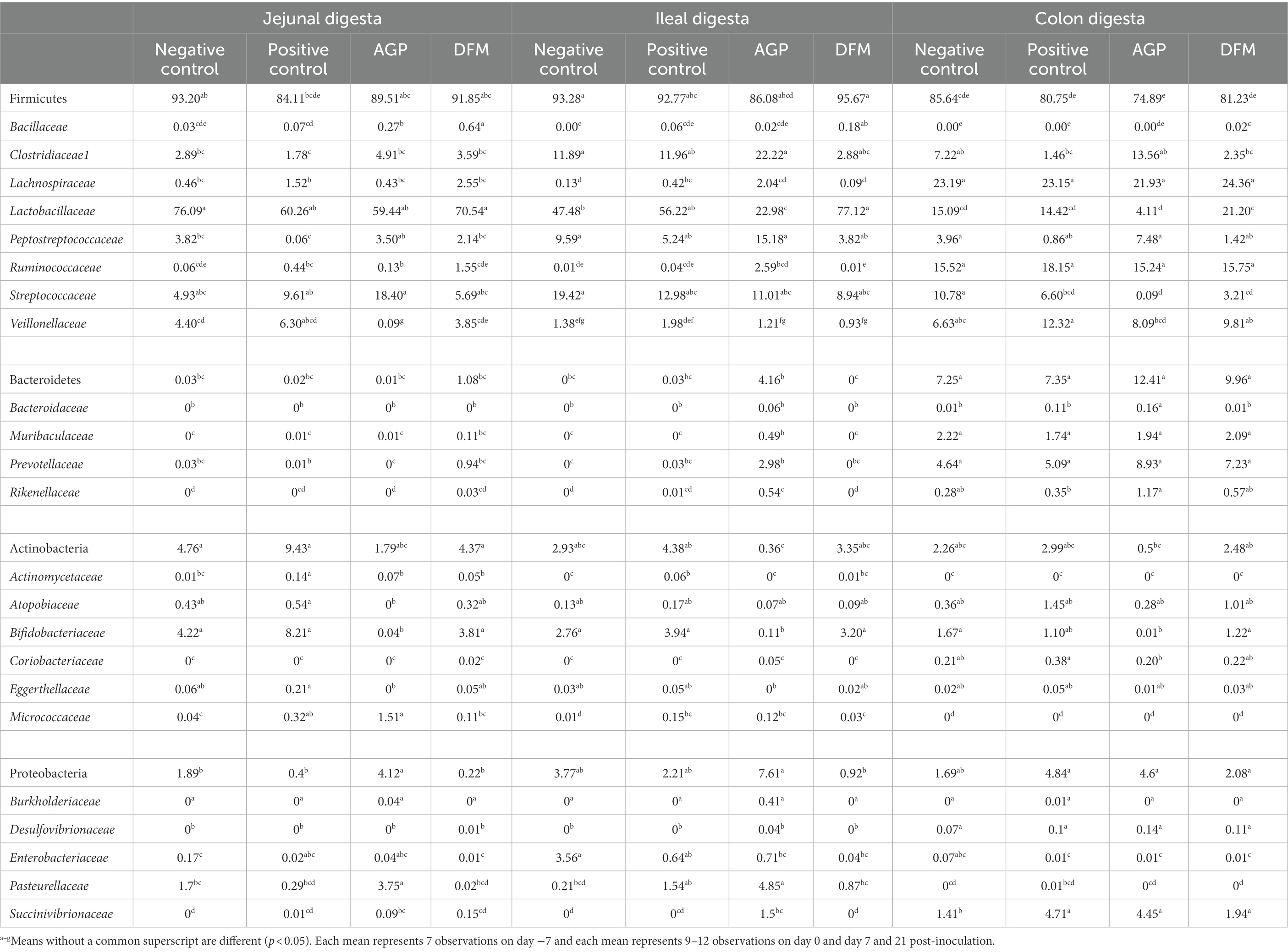
Table 3. Relative abundance (%) of Firmicutes, Bacteroidetes, Actinobacteria, and Proteobacteria and their top families in intestinal digesta of enterotoxigenic E. coli challenged pigs fed diets supplemented with antibiotics (AGP) or B. subtilis (DFM).
Under family level, jejunal digesta contained highest (p < 0.05) relative abundance of Lactobacillaceae, Actinomycetaceae, and Micrococcaceae among three intestinal sites. Ileal digesta had more (p < 0.05) relative abundance of Clostridiaceae1 and Enterobacteriaceae than jejunal and colon digesta. Colon digesta contained more (p < 0.05) Lachnospiraceae, Ruminococcaceae, Veillonellaceae, Coriobacteriaceae, Succinivibrionaceae, and top 4 families under Bacteroidetes than jejunal and ileal digesta. The relative abundance of Lactobacillaceae was greater (p < 0.05) in DFM than NC and AGP in ileal digesta and was greater (p < 0.05) in DFM than AGP in colon digesta. ETEC F18 challenge increased (p < 0.05) the relative abundance of Actinomycetaceae and Micrococcaceae in jejunal and ileal digesta, Lachnospiraceae in ileal digesta and Succinivibrionaceae in colon digesta, but reduced (p < 0.05) Streptococcaceae in colon digesta. Compared with PC, supplementation of AGP enhanced (p < 0.05) the relative abundance of Peptostreptococcaceae and Pasteurellaceae in jejunal digesta, Muribacelaceae in ileal digesta, and Bacteroidaceae and Rikenellaceae in colon digesta. In addition, inclusion of AGP reduced (p < 0.05) the relative abundance of Veillonellaceae, Actinomycetaceae, Atopobiaceae, Bifidobacteriaceae, Eggerthellaceae in jejunal digesta, Lachnospiraceae, Actinomycetaceae, Bifidobacteriaceae in ileal digesta, and Veillonellaceae and Coriobacteriaceae in colon digesta. In comparison to PC, supplementation of DFM reduced (p < 0.05) the relative abundance of Actinomycetaceae in jejunal digesta and Lachnospiraceae in ileal digesta. Pigs fed with DFM had greater (p < 0.05) the relative abundance of Ruminococcaceae, Veillonellaceae, Bifidobacteriaceae in jejunal digesta, Lactobacillaceae in both ileum and colon, and Bifidobacteriaceae in colon when compared with pigs in AGP. However, pigs fed with DFM had reduced (p < 0.05) the relative abundance of Pasteurellaceae in jejunum, Ruminococcaceae, Rikenellaceae, Pasteurellaceae, Succinivibrionaceae in ileum, and Bacteroidaceae in colon, compared with pigs fed with AGP. In all intestinal sites, pigs in DFM had the highest (p < 0.05) relative abundance of Bacillaceae among all treatments.
The relative abundances of top abundant genera are presented in Figure 6. Relative abundance of Lactobacillus was greater (p < 0.05) but the relative abundance of Clostridium sensu stricto 1 was less (p < 0.05) in ileal and colon digesta of pigs fed with DFM than in AGP. ETEC infection reduced the relative abundance of Streptococcus in ileum and colon, however, pigs supplemented with AGP had more (p < 0.05) Streptococcus in jejunum but lower (p < 0.05) Streptococcus in ileum and colon when compared with NC.
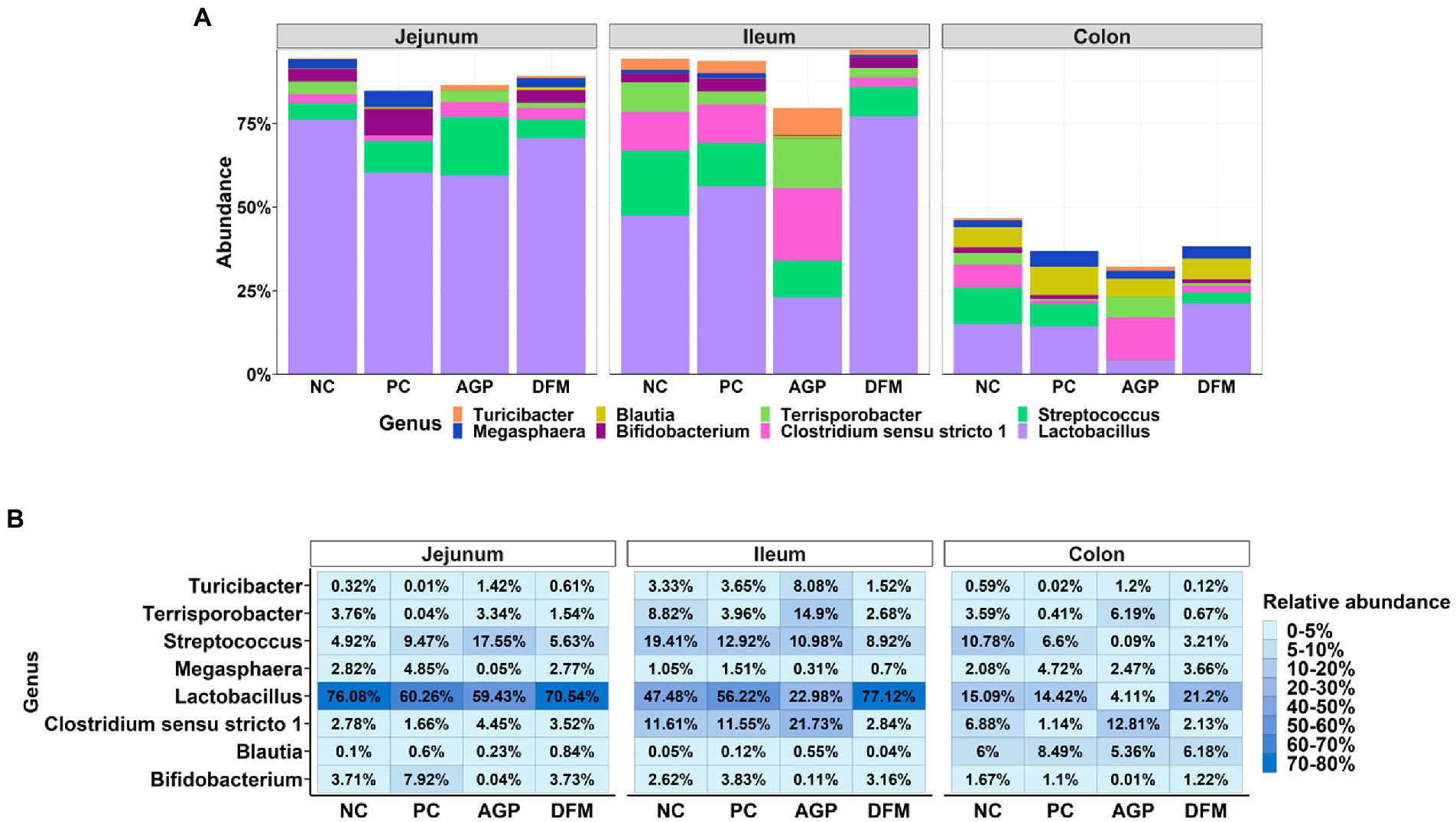
Figure 6. Relative abundance of top 8 genus in intestinal microbiota of piglets visualized in bar plot (A) and heatmap (B). Each mean represents 9–12 observations.
Discussion
Our previous studies reported that dietary supplementation of B. subtilis DSM 25841 promoted growth performance, reduced diarrhea, and enhanced intestinal immunity in weaned piglets under ETEC challenge (Kim et al., 2019; He et al., 2020). However, the potential mechanisms of the beneficial effects of B. subtilis on swine health and the impacts of this B. subtilis strain on intestinal microbiota remain unclear. In the present study, we characterized the impacts of ETEC F18 infection, B. subtilis DSM 25841, and carbadox on the dynamics of microbial composition in feces during the weaning transition period. The microbiota composition in different segments of the intestine were also investigated as ETEC F18 mainly target the small intestine of weaned pigs. Identifying shifts in microbial communities in the intestines could facilitate the development of nutritional interventions to control post-weaning diarrhea when the use of AGP is restricted, and to understand the impacts of currently available DFM on swine health.
Fecal microbiota
Temporal analysis in fecal microbiota was performed using fecal samples collected throughout the experiment. Richness in microbial population was measured in Chao1 and richness and evenness were measured in Shannon index (Shannon, 1948; Chao, 1984, p. 1). Lack of significant treatment effect was observed in the Shannon diversity metric in fecal samples of pigs throughout the experiment. Microbial richness was increased during the habituation period (from day − 7 to day 0) but decreased from day 0 to day 7 PI in feces collected from pigs in the positive control, which indicate that ETEC F18 challenge reduced microbial richness in feces. Beta diversity measures the variability in microbial community composition among samples. Samples collected at the beginning of the experiment (day − 7) separately clustered from samples collected from other time points, suggesting weaning significantly shifted microbial community composition. Distinctive clusters were also observed between samples on day 0 and day 7 PI. However, feces collected from positive control were not fully separated from samples collected from negative control, which suggests the impacts of ETEC F18 on fecal microbial community composition might be limited. Results of alpha and beta diversities also suggest that dietary supplementation of antibiotics or B. subtilis have limited impacts on the microbial diversity in fecal samples.
In consistent with other published research (Frese et al., 2015; Li et al., 2020), Firmicutes and Bacteroidetes were the top two most abundant phyla in fecal samples of weaned pigs. As the age of pigs was increased, the relative abundance of Firmicutes were increased but the relative abundance of Bacteroidetes were decreased (Mach et al., 2015; Chen et al., 2017), indicating that the fecal microbiota has greatly shifted during post-weaning period. The likely reason was the sudden change in diet from sow milk to solid and plant-based ingredients and the changes in environment after weaning. Weaning and dietary change shift the swine core gut microbiome, including Clostridiaceae1, Peptostreptococcaceae, Streptococcaceae, Bacteroidaceae, Enterobacteriaceae, and Lactobacillaceae in feces (Mach et al., 2015; Chen et al., 2017; Luise et al., 2021). The increased Lactobacillaceae is highly correlated with enriched plant-derived mono- and di-saccharides (Frese et al., 2015). At the end of this experiment, most of pigs were recovered from weaning stress and ETEC infection as indicated by the absence of ETEC F18 in feces and normal diarrhea scores (He et al., 2020). The relative abundance of the core gut microbiota listed above was reversed in feces, suggesting that the impacts of weaning stress on gut microbiota are significant but temporary. When pigs adapt to their new diets and environment, diet becomes the main driver of regulating gut microbial composition.
Post-weaning ETEC infection can reduce pig appetite, disrupt intestinal barrier, and induce intestinal inflammation, all of which may contribute to the imbalance of the microbiota (Pollock et al., 2019). ETEC is categorized under the family of Enterobacteriaceae, which is likely the reason for the increased relative abundance of Proteobacteria and Enterobacteriaceae was observed in the current study. Similar results were also reported in mice inoculated with ETEC (Wang et al., 2018). Growing evidence suggests that an increased abundance of Proteobacteria might be associated with dysbiosis (Shin et al., 2015). ETEC infected pigs in the present study were fully recovered on day 21 PI, as reported no diarrhea was observed and no β-hemolytic coliforms were present in feces (He et al., 2020). Thus, the impacts of ETEC infection on fecal microbiome were also gradually reduced on day 21 PI.
The use of carbadox in feed also induced changes in the fecal microbiota. Carbadox is an oxidative DNA-damaging agent that mainly target gram-positive bacteria (Breijyeh et al., 2020). During habituation period, supplementation of carbadox reduced relative abundance of Firmicutes and Lactobacillaceae in feces. Although ETEC infection temporarily increased the relative abundance of Lactobacillaceae in feces of pigs in the antibiotics group, the abundance of Lactobacillaceae was sharply reduced when pigs were recovered from ETEC infection. The relative abundance of Bacteroidetes was also numerically higher in carbadox group than pigs in control. These observations indicate the impacts of in-feed antibiotics on gut microbiome were immediate (Lourenco et al., 2021). The reduced Lactobacillaceae in feces is consistent with previous research (Gao et al., 2018), suggesting that supplementation of carbadox modified the intestinal environment which may be not supportive to maintain the growth of some favorable bacteria in the intestines.
Compared with carbadox, the influences of B. subtilis on fecal microbiome of weaned pigs were limited throughout the experiment. The relative abundance of Bacillaceae was the greatest in feces fed with B. subtilis among all treatments on day 0 before ETEC infection and day 21 PI, which is likely due to B. subtilis is categorized under the family Bacillaceae. Supplementation of B. subtilis significantly enhanced the relative abundance of Firmicutes, but numerically reduced Proteobacteria and Enterobacteriaceae in feces at the peak of ETEC infection. This observation is also consistent with the published fecal culture results, in which pigs fed with B. subtilis had lower percentage of β-hemolytic coliforms in feces on day 7 PI (He et al., 2020). Our previous research revealed that both carbadox and B. subtilis supplementation were effective in enhancing growth performance and reducing diarrhea of weaned pigs challenged with ETEC F18 (He et al., 2020). However, results in fecal microbiome suggest that the impacts of dietary supplementation of B. subtilis and antibiotics on fecal microbiome were different in weaned pigs. The major highlights are the modulation of Firmicutes phylum. Overall, pigs fed with B. subtilis contained relative higher Lactobacillaceae but lower Bacteroidaceae than pigs fed with antibiotics throughout the experiment, although ETEC infection temporarily reduced this difference on day 7 PI. Although carbadox mainly targets gram-positive bacteria, it can also disturb bacterial DNA synthesis and induce the breakdown of chromosome in many gram-negative bacteria, including ETEC (Suter et al., 1978; Cheng et al., 2015). However, B. subtilis as a probiotic strain must colonize into the intestines of pigs in order to perform beneficial effects on gut ecosystem. Thus, it is not supersizing to observe the different impacts of these two supplements on fecal microbiome due to their different modes of action.
Intestinal microbiota
In the current study, the intestinal microbiota changes were also analyzed in intestinal content collected at the end of experiment when pigs were fully recovered from ETEC infection. Similar to the results shown in Crespo-Piazuelo et al. (2018), Shannon and Chao1 index values in distal colon were higher than in jejunum and ileum. Consistently, beta diversity results showed clear separation between colon content vs. jejunal and ileal digesta, indicating the spatial heterogeneity of bacteria colonization across different intestinal sites (Mu et al., 2017). Supplementation of carbadox or B. subtilis had stronger influences on microbial diversity and composition in the ileum than in the jejunum and distal colon.
Ileal and jejunal digesta had greater relative abundance of Firmicutes and lowest relative abundance of Bacteroidetes than colon content, suggesting the luminal environment remarkably modulates digesta microbiome (Gao et al., 2018; Pollock et al., 2019). The relatively acidic environment and high oxygen level prevent the colonization of anaerobes in the small intestine (Donaldson et al., 2016). In addition, the small intestinal digesta contains more simple carbohydrates that could be utilized by the host and the microbiota, while the large intestine lumen comprises more complex carbohydrates for microbial fermentation (Zoetendal et al., 2012). The enriched Lachnospiraceae, Ruminococcaceae, and Prevotellaceae enable the large intestine’s capacity to degrade complex carbohydrates (Duncan et al., 2007; Arumugam et al., 2011; Zhang et al., 2018). The ileum is a major intestinal site where ETEC colonizes, which is probably the major reason that more abundant Enterobacteriaceae were observed in ileal digesta than jejunum and colon (Nagy et al., 1992). However, there were limited changes in the microbial composition in different intestinal segments when comparing positive control vs. negative control pigs on day 21 PI, which was likely due to the recovery of weaned pigs from ETEC F18 infection (He et al., 2020). The relative abundance of Micrococcaceae was proliferated in jejunal and ileal digesta of pigs infected with ETEC. Micrococcaceae are relatively abundant in newborn pigs, but are gradually reduced during pre-weaning period (Pena Cortes et al., 2018). The increased abundance of Micrococcaceae were also observed in diarrheal fecal samples in a human study (De et al., 2020). However, the reason for this change is not clear and needs to be further investigated.
In comparison to the positive control, carbadox supplementation had more influences on the intestinal microbiota than B. subtilis. In addition, carbadox supplementation altered jejunal and ileal microbiota more than colon microbiota in weaned pigs, which is in close agreement with a previous study using a mixed antibiotics supplementation (Mu et al., 2017). This observation also indicates that antibiotics treatment may mainly target the microbiota in the small intestine. At the taxonomic level, pigs fed with carbadox had greater relative abundance of gram-negative bacteria, including Succinivibrionaceae and Pasteurellaceae in jejunum and Muribaculaceae in ileum, but lower relative abundance of Lactobacillaceae and Bifidobacteriaceae in ileum. This observation suggest that gram-negative bacteria may be more tolerant to carbadox due to their complicated outer membrane structure, containing lipopolysaccharides and peptidoglycans that can reduce the chance of carbadox penetrating into the bacterial membranes (Breijyeh et al., 2020). Gram-negative bacteria are more resistant to commonly used antibiotics by developing antibiotics resisting enzymes and undergoing mutations to increase resistance against antibiotics (Miller, 2016; Sumi et al., 2019). Therefore, the accumulation of gram-negative bacteria in the small intestine when carbadox is applied may increase the risk of developing antimicrobial resistance. However, in the production side, supplementation of carbadox was reported to effectively enhance growth performance and reduce intestinal inflammation of weaned pigs (Roof and Mahan, 1982; Stahly et al., 1997; He et al., 2020). The potential reason might include that carbadox can increase the population of short-chain fatty acid producing microbiota (i.e., Ruminococcaceae) in the small intestine, which may benefit to intestinal health and energy utilization in pigs (Bedford and Gong, 2018).
Pigs supplemented with B. subtilis had increased the relative abundance of gram-positive bacteria than pigs supplemented with carbadox, including Lactobacillaceae, Bacillaceae, and Bifidobacteriaceae in ileal digesta. The increased abundance of Bacillaceae was likely due to the colonization of B. subtilis in the intestinal tract (Pollock et al., 2019), which needs to be further confirmed in the future study. The more enriched Lactobacillaceae and Bifidobacteriaceae suggest that supplementing B. subtilis may help to maintain the desirable bacteria in the small intestine of weaned pigs, which may contribute to the concomitant decrease in enteric bacterial infection. Previous research also reported that B. subtilis supplementation can also decrease luminal pH and oxidation potential of the gut matrix, which may provide a favorable condition for Lactobacillaceae to thrive but inhibit the growth of the pathogenic bacteria (Yang et al., 2015). Therefore, results from the current study indicate that the modulation of gut microbiome by B. subtilis supplementation also contributes to the reduced diarrhea and enhanced intestinal health of ETEC infected pigs (He et al., 2020).
Conclusion
Our previous study reported that supplementation of B. subtilis DSM 25841 improved growth performance and disease resistance of weaned pigs under ETEC challenge (He et al., 2020). The current study characterized the microbial diversity and composition of fecal samples and intestinal contents collected from He et al. (2020). The present results indicate that weaning stress, age of pigs, ETEC infection, and dietary supplements all contributed to the fecal and intestinal microbiome changes in weaned pigs. Supplementation of carbadox and B. subtilis differently modified fecal and intestinal microbiota. Pigs supplemented with B. subtilis had higher abundance of Bacillaceae than pigs supplemented with carbadox in fecal and intestinal content. Supplementation of carbadox increased the relative abundance of gram-negative bacteria including Bacteroidetes and Proteobacteria which may impose risk of increasing antibiotics resistance. Supplementation of B. subtilis was associated with an increase or maintenance of the relative abundance of beneficial bacteria including Lactobacillaceae and Bifidobacteriaceae in the ileum and colon. Future research is needed to quantify B. subtilis colonization in the gastrointestinal tract and to investigate the interactions of B. subtilis with other bacteria, especially ETEC. In addition, the limits of 16S rRNA sequencing has been recognized, thus, the analysis of gut bacterial biomass and the functional genomes from the gut microbiota should be considered in the future research as well.
Data availability statement
The datasets presented in this study can be found in online repositories. The names of the repository/repositories and accession number(s) can be found at: https://www.ncbi.nlm.nih.gov/, PRJNA885119.
Ethics statement
Animal procedures were reviewed and approved by the Institutional Animal Care and Use Committee (IACUC #19322) at the University of California, Davis (UC Davis).
Author contributions
YL and XL designed and supervised the entire experiment. CJ performed all gut microbiome analysis, including sample analysis and data analysis. CJ wrote the manuscript. YL and XL revised and approved the final version. All authors contributed to the article and approved the submitted version.
Acknowledgments
The authors would like to thank the National Pork Board (#18-081) for financial support of this research.
Conflict of interest
The authors declare that the research was conducted in the absence of any commercial or financial relationships that could be construed as a potential conflict of interest.
Publisher’s note
All claims expressed in this article are solely those of the authors and do not necessarily represent those of their affiliated organizations, or those of the publisher, the editors and the reviewers. Any product that may be evaluated in this article, or claim that may be made by its manufacturer, is not guaranteed or endorsed by the publisher.
Footnotes
References
Arumugam, M., Raes, J., Pelletier, E., Le Paslier, D., Yamada, T., Mende, D. R., et al. (2011). Enterotypes of the human gut microbiome. Nature 473, 174–180. doi: 10.1038/nature09944
Bates, D., Mächler, M., Bolker, B., and Walker, S. (2014). Fitting linear mixed-effects models using lme4. arXiv preprint arXiv:1406.5823v1 67:48. doi: 10.48550/arXiv.1406.5823
Bedford, A., and Gong, J. (2018). Implications of butyrate and its derivatives for gut health and animal production. Anim. Nutr. 4, 151–159. doi: 10.1016/j.aninu.2017.08.010
Boeckel, T. P. V., Brower, C., Gilbert, M., Grenfell, B. T., Levin, S. A., Robinson, T. P., et al. (2015). Global trends in antimicrobial use in food animals. PNAS 112, 5649–5654. doi: 10.1073/pnas.1503141112
Bokulich, N. A., Kaehler, B. D., Rideout, J. R., Dillon, M., Bolyen, E., Knight, R., et al. (2018). Optimizing taxonomic classification of marker-gene amplicon sequences with QIIME 2’s q2-feature-classifier plugin. Microbiome 6:90. doi: 10.1186/s40168-018-0470-z
Bolyen, E., Rideout, J. R., Dillon, M. R., Bokulich, N. A., Abnet, C. C., Al-Ghalith, G. A., et al. (2019). Reproducible, interactive, scalable and extensible microbiome data science using QIIME 2. Nat. Biotechnol. 37, 852–857. doi: 10.1038/s41587-019-0209-9
Breijyeh, Z., Jubeh, B., and Karaman, R. (2020). Resistance of gram-negative bacteria to current antibacterial agents and approaches to resolve it. Molecules 25:1340. doi: 10.3390/molecules25061340
Callahan, B. J., McMurdie, P. J., Rosen, M. J., Han, A. W., Johnson, A. J. A., and Holmes, S. P. (2016). DADA2: high resolution sample inference from Illumina amplicon data. Nat. Methods 13, 581–583. doi: 10.1038/nmeth.3869
Campbell, J. M., Crenshaw, J. D., and Polo, J. (2013). The biological stress of early weaned piglets. J. Anim. Sci. Biotechnol. 4:19. doi: 10.1186/2049-1891-4-19
Cao, K. F., Zhang, H. H., Han, H. H., Song, Y., Bai, X. L., and Sun, H. (2016). Effect of dietary protein sources on the small intestine microbiome of weaned piglets based on high-throughput sequencing. Lett. Appl. Microbiol. 62, 392–398. doi: 10.1111/lam.12559
Caporaso, J. G., Lauber, C. L., Walters, W. A., Berg-Lyons, D., Huntley, J., Fierer, N., et al. (2012). Ultra-high-throughput microbial community analysis on the Illumina HiSeq and MiSeq platforms. ISME J. 6, 1621–1624. doi: 10.1038/ismej.2012.8
Chao, A. (1984). Nonparametric estimation of the number of classes in a population. Scand. J. Stat. 11, 265–270.
Chen, L., Xu, Y., Chen, X., Fang, C., Zhao, L., and Chen, F. (2017). The maturing development of gut microbiota in commercial piglets during the weaning transition. Front. Microbiol. 8:1688. doi: 10.3389/fmicb.2017.01688
Cheng, G., Li, B., Wang, C., Zhang, H., Liang, G., Weng, Z., et al. (2015). Systematic and molecular basis of the antibacterial action of quinoxaline 1,4-di-N-oxides against Escherichia coli. PLoS One 10:e0136450. doi: 10.1371/journal.pone.0136450
Crespo-Piazuelo, D., Estellé, J., Revilla, M., Criado-Mesas, L., Ramayo-Caldas, Y., Óvilo, C., et al. (2018). Characterization of bacterial microbiota compositions along the intestinal tract in pigs and their interactions and functions. Sci. Rep. 8:12727. doi: 10.1038/s41598-018-30932-6
De, R., Mukhopadhyay, A. K., and Dutta, S. (2020). Metagenomic analysis of gut microbiome and resistome of diarrheal fecal samples from Kolkata, India, reveals the core and variable microbiota including signatures of microbial dark matter. Gut. Pathog. 12:32. doi: 10.1186/s13099-020-00371-8
Donaldson, G. P., Lee, S. M., and Mazmanian, S. K. (2016). Gut biogeography of the bacterial microbiota. Nat. Rev. Microbiol. 14, 20–32. doi: 10.1038/nrmicro3552
Duncan, S. H., Louis, P., and Flint, H. J. (2007). Cultivable bacterial diversity from the human colon. Lett. Appl. Microbiol. 44, 343–350. doi: 10.1111/j.1472-765X.2007.02129.x
Fairbrother, J., Gyles, C., Straw, B., Zimmerman, J., D’Allaire, S., and Taylor, D. (2006). Diseases of swine.
FDA (2013). Guidance for Industry# 213, New Animal Drugs and New Animal Drug Combination Products Administered in or on Medicated Feed or Drinking Water of Food-producing Animals: Recommendations for Drug Sponsors for Voluntarily Aligning Product use Conditions with GFI# 209. Center for Veterinary Medicine, Rockville, MD.
Fouhse, J. M., Zijlstra, R. T., and Willing, B. P. (2016). The role of gut microbiota in the health and disease of pigs. Anim. Fron. 6, 30–36. doi: 10.2527/af.2016-0031
Fox, J., and Weisberg, S. (2018). An R Companion to Applied Regression. Thousand Oaks: Sage Publications.
Frese, S. A., Parker, K., Calvert, C. C., and Mills, D. A. (2015). Diet shapes the gut microbiome of pigs during nursing and weaning. Microbiome. 3, 28–37. doi: 10.1186/s40168-015-0091-8
Gao, J., Xu, K., Liu, H., Liu, G., Bai, M., Peng, C., et al. (2018). Impact of the gut microbiota on intestinal immunity mediated by tryptophan metabolism. Front. Cell Infect. Microbiol. 8:13. doi: 10.3389/fcimb.2018.00013
Gresse, R., Chaucheyras-Durand, F., Fleury, M. A., Van de Wiele, T., Forano, E., and Blanquet-Diot, S. (2017). Gut microbiota dysbiosis in postweaning piglets: understanding the keys to health. Trends Microbiol. 25, 851–873. doi: 10.1016/j.tim.2017.05.004
He, Y., Kim, K., Kovanda, L., Jinno, C., Song, M., Chase, J., et al. (2020). Bacillus subtilis: a potential growth promoter in weaned pigs in comparison to carbadox. Anim. Sci. J. 98:skaa290. doi: 10.1093/jas/skaa290
Hu, Y., Dun, Y., Li, S., Zhao, S., Peng, N., and Liang, Y. (2014). Effects of Bacillus subtilis KN-42 on growth performance, diarrhea and faecal bacterial flora of weaned piglets. Asian Australas. J. Anim. Sci. 27, 1131–1140. doi: 10.5713/ajas.2013.13737
Kamada, N., Seo, S. U., Chen, G. Y., and Núñez, G. (2013). Role of the gut microbiota in immunity and inflammatory disease. Nat. Rev. Immunol. 13, 321–335. doi: 10.1038/nri3430
Katoh, K., and Standley, D. M. (2013). MAFFT multiple sequence alignment software version 7: improvements in performance and usability. Mol. Bio. Evol. 30, 772–780. doi: 10.1093/molbev/mst010
Ke, S., Fang, S., He, M., Huang, X., Yang, H., Yang, B., et al. (2019). Age-based dynamic changes of phylogenetic composition and interaction networks of health pig gut microbiome feeding in a uniformed condition. BMC. Vet. Res. 15, 1–13.
Kim, K., He, Y., Xiong, X., Ehrlich, A., Li, X., Raybould, H., et al. (2019). Dietary supplementation of Bacillus subtilis influenced intestinal health of weaned pigs experimentally infected with a pathogenic E. coli. J. Anim. Sci. Biotechnol 10, 1–12. doi: 10.1186/s40104-019-0364-3
Kreuzer, S., Reissmann, M., and Brockmann, G. A. (2013). New fast and cost-effective gene test to get the ETEC F18 receptor status in pigs. Vet. Microbiol. 163, 392–394. doi: 10.1016/j.vetmic.2012.12.040
Lallès, J.-P., Boudry, G., Favier, C., Floc’h, N. L., Luron, I., Montagne, L., et al. (2004). Gut function and dysfunction in young pigs: physiology. Anim. Res. 53, 301–316. doi: 10.1051/animres:2004018
Lenth, R. V. (2021). Emmeans: Estimated Marginal Means, Aka Least-squares Means. R Package Version, 1.6. 1.
Li, N., Zuo, B., Huang, S., Zeng, B., Han, D., Li, T., et al. (2020). Spatial heterogeneity of bacterial colonization across different gut segments following inter-species microbiota transplantation. Microbiome. 8, 161–174. doi: 10.1186/s40168-020-00917-7
Liao, S. F., and Nyachoti, M. (2017). Using probiotics to improve swine gut health and nutrient utilization. Anim. Nutr. 3, 331–343. doi: 10.1016/j.aninu.2017.06.007
Liu, Y., Song, M., Che, T. M., Almeida, J. A. S., Lee, J. J., Bravo, D., et al. (2013). Dietary plant extracts alleviate diarrhea and alter immune responses of weaned pigs experimentally infected with a pathogenic Escherichia coli. J. Anim. Sci. 91, 5294–5306. doi: 10.2527/jas.2012-6194
Looft, T., Allen, H. K., Casey, T. A., Alt, D. P., and Stanton, T. B. (2014). Carbadox has both temporary and lasting effects on the swine gut microbiota. Front. Microbiol. 5:276. doi: 10.3389/fmicb.2014.00276
Lourenco, J. M., Hampton, R. S., Johnson, H. M., Callaway, T. R., Rothrock, M. J., and Azain, M. J. (2021). The effects of feeding antibiotic on the intestinal microbiota of weanling pigs. Front. Vet. Sci. 8:131. doi: 10.3389/fvets.2021.601394
Luise, D., Bertocchi, M., Motta, V., Salvarani, C., Bosi, P., Luppi, A., et al. (2019). Bacillus sp. probiotic supplementation diminish the Escherichia coli F4ac infection in susceptible weaned pigs by influencing the intestinal immune response, intestinal microbiota, and blood metabolomics. J. Anim. Sci. Biotechnol. 10, 74–89. doi: 10.1186/s40104-019-0380-3
Luise, D., Sciellour, M. L., Buchet, A., Resmond, R., Clement, C., Rossignol, M.-N., et al. (2021). The fecal microbiota of piglets during weaning transition and its association with piglet growth across various farm environments. PLoS One 16:e0250655. doi: 10.1371/journal.pone.0250655
Ma, F., Xu, S., Tang, Z., Li, Z., and Zhang, L. (2021). Use of antimicrobials in food animals and impact of transmission of antimicrobial resistance on humans. Biosaf. Health 3, 32–38. doi: 10.1016/j.bsheal.2020.09.004
Mach, N., Berri, M., Estellé, J., Levenez, F., Lemonnier, G., Denis, C., et al. (2015). Early-life establishment of the swine gut microbiome and impact on host phenotypes. Environ. Microbiol. Rep. 7, 554–569. doi: 10.1111/1758-2229.12285
Madison, A., and Kiecolt-Glaser, J. K. (2019). Stress, depression, diet, and the gut microbiota: human–bacteria interactions at the core of psychoneuroimmunology and nutrition. Curr. Opin. Behav. Sci. 28, 105–110. doi: 10.1016/j.cobeha.2019.01.011
McMurdie, P. J., and Holmes, S. (2013). Phyloseq: an R package for reproducible interactive analysis and graphics of microbiome census data. PLoS One 8:e61217. doi: 10.1371/journal.pone.0061217
Menkem, Z. E., Ngangom, B. L., Tamunjoh, S. S. A., and Boyom, F. F. (2019). Antibiotic residues in food animals: public health concern. Acta Ecol. Sin. 39, 411–415. doi: 10.1016/j.chnaes.2018.10.004
Miller, S. I. (2016). Antibiotic resistance and regulation of the gram-negative bacterial outer membrane barrier by host innate immune molecules. MBio 7:e01541. doi: 10.1128/mBio.01541-16
Mu, C., Yang, Y., Su, Y., Zoetendal, E. G., and Zhu, W. (2017). Differences in microbiota membership along the gastrointestinal tract of piglets and their differential alterations following an early-life antibiotic intervention. Front. Microbiol. 8:797. doi: 10.3389/fmicb.2017.00797
Nagy, B., Arp, L. H., Moon, H. W., and Casey, T. A. (1992). Colonization of the small intestine of weaned pigs by enterotoxigenic Escherichia coli that lack known colonization factors. Vet. Pathol. 29, 239–246. doi: 10.1177/030098589202900308
Oksanen, J., Blanchet, F. G., Kindt, R., Legendre, P., Minchin, P. R., O’hara, R. B., et al. (2013). Package ‘Vegan’. Community Ecology Package, R Package Version, 2.
Pedregosa, F., Varoquaux, G., Gramfort, A., Michel, V., Thirion, B., Grisel, O., et al. (2011). Scikit-learn: machine learning in python. J. Mach. Learn. Res. 12, 2825–2830.
Pena Cortes, L. C., LeVeque, R. M., Funk, J. A., Marsh, T. L., and Mulks, M. H. (2018). Development of the tonsil microbiome in pigs and effects of stress on the microbiome. Front. Vet. Sci 5:220. doi: 10.3389/fvets.2018.00220
Pollock, J., Hutchings, M. R., Hutchings, K. E. K., Gally, D. L., and Houdijk, J. G. M. (2019). Changes in the ileal, but not fecal, microbiome in response to increased dietary protein level and enterotoxigenic Escherichia coli exposure in pigs. Appl. Environ. Microbiol. 85, e01252–e01219. doi: 10.1128/AEM.01252-19
Price, M. N., Dehal, P. S., and Arkin, A. P. (2010). FastTree 2 – approximately maximum-likelihood trees for large alignments. PLoS One 5:e9490. doi: 10.1371/journal.pone.0009490
Quast, C., Pruesse, E., Yilmaz, P., Gerken, J., Schweer, T., Yarza, P., et al. (2012). The SILVA ribosomal RNA gene database project: improved data processing and web-based tools. Nucleic Acids Res. 41, D590–D596. doi: 10.1093/nar/gks1219
Roof, M. D., and Mahan, D. C. (1982). Effect of carbadox and various dietary copper levels for weanling swine. J. Anim. Sci. 55, 1109–1117. doi: 10.2527/jas1982.5551109x
Shannon, C. E. (1948). A mathematical theory of communication. Bell Syst. Tech. J. 27, 379–423. doi: 10.1002/j.1538-7305.1948.tb01338.x
Shin, N.-R., Whon, T. W., and Bae, J.-W. (2015). Proteobacteria: microbial signature of dysbiosis in gut microbiota. Trends Biotechnol. 33, 496–503. doi: 10.1016/j.tibtech.2015.06.011
Stahly, T. S., Williams, N. H., and Swenson, S. G. (1997). Growth Response to Carbadox in Pigs with a High or Low Genetic Capacity for Lean Tissue Growth. Animal Industry Report, Swine Research Report, 1996. Ames, IA: Iowa State University Digital Press, pp. 1–5.
Sumi, C. D., Heffernan, A. J., Lipman, J., Roberts, J. A., and Sime, F. B. (2019). What antibiotic exposures are required to suppress the emergence of resistance for gram-negative bacteria? A systematic review. Clin. Pharmacokinet. 58, 1407–1443. doi: 10.1007/s40262-019-00791-z
Suter, W., Rosselet, A., and Knüsel, F. (1978). Mode of action of quindoxin and substituted quinoxaline-di-N-oxides on Escherichia coli. Antimicrob. Agents Chemother. 13, 770–783. doi: 10.1128/AAC.13.5.770
Team, R. C. (2021). R: A Language and Environment for Statistical Computing. Vienna, Austria: R Foundation for Statistical Computing.
Tian, L., Bruggeman, G., van den Berg, M., Borewicz, K., Scheurink, A. J. W., Bruininx, E., et al. (2017). Effects of pectin on fermentation characteristics, carbohydrate utilization, and microbial community composition in the gastrointestinal tract of weaning pigs. Mol. Nutr. Food Res. 61. doi: 10.1002/mnfr.201600186
USDA. (2007). Swine 2006, Part II: Reference of Swine Health and Health Management Practices in the United States, 2006 USDA:APHIS:VS, CEAH. Fort Collins, CO., Program No. N479.1207.
Wang, T., Teng, K., Liu, G., Liu, Y., Zhang, J., Zhang, X., et al. (2018). Lactobacillus reuteri HCM2 protects mice against enterotoxigenic Escherichia coli through modulation of gut microbiota. Sci. Rep. 8:17485. doi: 10.1038/s41598-018-35702-y
Yang, J. J., Niu, C. C., and Guo, X. H. (2015). Mixed culture models for predicting intestinal microbial interactions between Escherichia coli and lactobacillus in the presence of probiotic Bacillus subtilis. Benef. Microbes 6, 871–877. doi: 10.3920/BM2015.0033
Zhang, L., Wu, W., Lee, Y.-K., Xie, J., and Zhang, H. (2018). Spatial heterogeneity and co-occurrence of mucosal and luminal microbiome across swine intestinal tract. Front. Microbiol. 9:48. doi: 10.3389/fmicb.2018.00048
Keywords: antibiotics, Bacillus subtilis, Escherichia coli challenge, microbiome, weaned pigs
Citation: Jinno C, Li X and Liu Y (2022) Dietary supplementation of Bacillus subtilis or antibiotics modified intestinal microbiome of weaned pigs under enterotoxigenic Escherichia coli infection. Front. Microbiol. 13:1064328. doi: 10.3389/fmicb.2022.1064328
Edited by:
Chang-Wei Lei, Sichuan University, ChinaReviewed by:
Kun Li, Nanjing Agricultural University, ChinaJinhu Huang, Nanjing Agricultural University, China
Copyright © 2022 Jinno, Li and Liu. This is an open-access article distributed under the terms of the Creative Commons Attribution License (CC BY). The use, distribution or reproduction in other forums is permitted, provided the original author(s) and the copyright owner(s) are credited and that the original publication in this journal is cited, in accordance with accepted academic practice. No use, distribution or reproduction is permitted which does not comply with these terms.
*Correspondence: Yanhong Liu, eWFobGl1QHVjZGF2aXMuZWR1