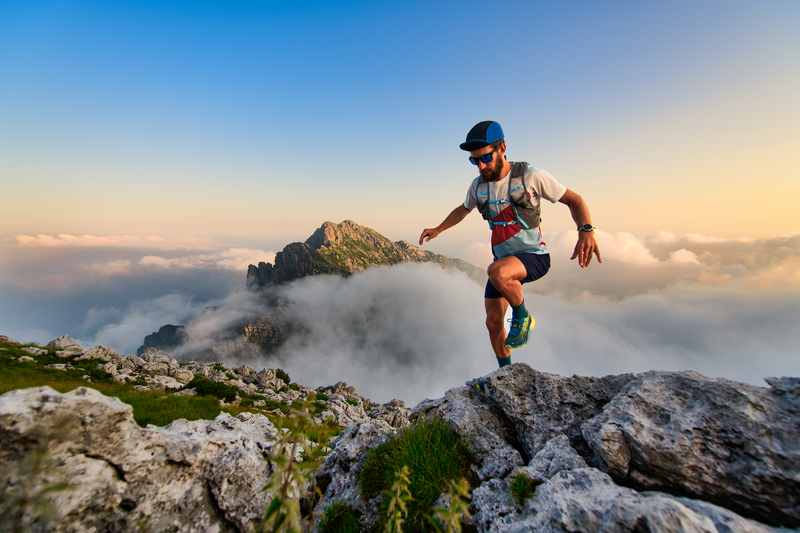
95% of researchers rate our articles as excellent or good
Learn more about the work of our research integrity team to safeguard the quality of each article we publish.
Find out more
ORIGINAL RESEARCH article
Front. Microbiol. , 31 October 2022
Sec. Infectious Agents and Disease
Volume 13 - 2022 | https://doi.org/10.3389/fmicb.2022.1046640
This article is part of the Research Topic Pathogenomics of the Genus Brucella and Beyond, Volume II View all 14 articles
Brucella spp. are the etiological agent of animal and human brucellosis. We have reported previously that cyclophilins of Brucella (CypA and CypB) are upregulated within the intraphagosomal replicative niche and required for stress adaptation and host intracellular survival and virulence. Here, we characterize B. abortus cyclophilins, CypA, and CypB from a biochemical standpoint by studying their PPIase activity, chaperone activity, and oligomer formation. Even though CypA and CypB are very similar in sequence and share identical chaperone and PPIase activities, we were able to identify outstanding differential features between them. A series of differential peptide loops were predicted when comparing CypA and CypB, differences that might explain why specific antibodies (anti-CypA or anti-CypB) were able to discriminate between both cyclophilins without cross-reactivity. In addition, we identified the presence of critical amino acids in CypB, such as the Trp134 which is responsible for the cyclosporin A inhibition, and the Cys128 that leads to CypB homodimer formation by establishing a disulfide bond. Here, we demonstrated that CypB dimer formation was fully required for stress adaptation, survival within HeLa cells, and mouse infection in B. abortus. The presence of Trp134 and the Cys128 in CypB, which are not present in CypA, suggested that two different kinds of cyclophilins have evolved in Brucella, one with eukaryotic features (CypB), another (CypA) with similar features to Gram-negative cyclophilins.
Cyclophilins are enzymes that belong to the superfamily of peptidyl-prolyl cis/trans isomerases (PPIases: EC 5.2.1.8). These enzymes act as biological catalysts speeding up the rate-limiting cis/trans or trans/cis conformational changes at Xaa-Pro bonds during protein folding in both eukaryotes and prokaryotes. The spontaneous isomerization of the peptidyl proline bonds is a slow reaction and consequently requires the assistance of PPIases that accelerates this step during protein folding. In addition to the cyclophilins, the superfamily of PPIases, also includes the FK506-binding proteins (FKBPs) and the parvulins, a classification that is based on their structure and specific inhibitor compound. Thus, while cyclophilins are inhibited by the immunosuppressive cyclosporin A (CsA), the FKBPs and parvulins are inhibited by the compounds FK506, and rapamycin, respectively (Galat, 2003; Fanghanel and Fischer, 2004).
Cyclophilins are either small single-domain proteins or large multi-domain ones (Pemberton, 2006; Krucken et al., 2009). In the case of the multi-domain cyclophilins, it has been described that additionally to the cyclophilin domain there are also protein domains that act as chaperones or promote the oligomerization state of the protein. Interestingly, even though single-domain cyclophilins are devoid of a canonical chaperone protein domain they can still present certain chaperone activity, which in some cases it has been shown to be independent of the PPIase catalytic activity (Dimou et al., 2011; Zhang et al., 2013; Pandey et al., 2016). Moreover, it has been described that some of the single-domain cyclophilins are still able to oligomerize (Zhang et al., 2011; Jakob et al., 2016).
Of relevance, the over-expression of many cyclophilin-encoding genes is triggered in response to a variety of stressors, suggesting a possible function of cyclophilins (Cyps) in stress adaptation. In agreement with this, microbial Cyps have been described to improve microbial survival under stress conditions and to be upregulated upon host-cell internalization, suggesting a possible function of these proteins in microbial-host interaction (Dimou et al., 2017). Interestingly, the critical role of PPIases in stress tolerance and pathogenesis of bacteria has been demonstrated in Yersinia pseudotuberculosis (Obi et al., 2011), Streptococcus pneumoniae (Hermans et al., 2006), Enterococcus faecalis (Reffuveille et al., 2012), Streptococcus gordoni (Cho et al., 2013), Mycobacterium tuberculosis (Pandey et al., 2017), Staphylococcus aureus (Wiemels et al., 2017; Keogh et al., 2018), Legionella pneumophila (Rasch et al., 2019), Burkholderia pseudomallei (Bzdyl et al., 2019), and Salmonella Typhimurium (Kumawat et al., 2020).
Brucellosis is a worldwide zoonotic disease caused by the intracellular bacterial pathogen, Brucella spp. Brucella spp. are Gram-negative bacteria that belongs to the α-2 group of Proteobacteria, a bacterial group characterized for living in close association with eukaryotic hosts such as plants or mammals (Corbel, 1997). Brucella infection causes abortion and sterility in animals, and undulating fever and debilitating disorders in humans, resulting in a serious public health problem and economic losses (de Figueiredo et al., 2015). Brucella virulence relies on its ability to adapt to an intracellular lifestyle within the host cells. To gain insight into the molecular mechanisms involved in intracellular adaptation and virulence of Brucella, we performed a comparative proteome analysis of Brucella grown in culture media or recovered from Brucella infected macrophages using two complementary technologies: 2D gel (Roset et al., 2013) and iTraq isobaric tag (Roset et al., 2017). We demonstrated through 2D gel analysis, that, upon intracellular localization, B. abortus over-expresses two PPIases (BAB1_1117 and BAB1_1118), belonging to the cyclophilin family (COG0652), referred to as CypB and CypA, respectively. Analysis of their function by mutagenesis and subsequent characterization showed that they are involved in stress adaptation, intracellular survival, and Brucella virulence (Roset et al., 2013). In this report, we characterized CypA and CypB from a biochemical and functional standpoint exploring the role of the cyclophilins in Brucella-host cell interaction.
Bacterial strains and plasmids used are shown in Table 1. Escherichia coli strains were grown in Luria-Bertani (LB) media at 37°C on a rotatory shaker (250 rpm) or in LB agar for 16–24 h. Brucella abortus strains were grown in tryptic soy agar (TSA) or tryptic soy broth (TSB) media at 37°C on a rotatory shaker (250 rpm) for 16–24 h. When necessary, media were supplemented with the following antibiotics: kanamycin (km), 50 μg/ml, ampicillin 100 μg/ml, or nalidixic acid 5 μg/ml. Experiments involving live Brucella were performed in a Biosafety level 3 (BSL3) facility at the University of San Martín, Buenos Aires, Argentina.
cypB and cypBR59A/F64A genes were amplified from Brucella genomic DNA or pcypBR55A/F60A plasmid, respectively, by PCR using the oligonucleotides (pFWCypBBamHI CGGGATCCGACCCAGAAAATACGCTCG and pRVCypBXhoI CCCTCGAGTCAGTCGGCGGCGATACG). Amplicons were digested with BamHI and XhoI restriction enzymes and cloned in pET-28a(+) vector from Novagen.
Synthetic genes, synthesized by Gene Universal Inc. (United States), cypA, cypBC128M, cypBW134F, cypB(L2cypA), cypB(L3cypA), cypB(L2-L3cypA), and cypA(L1cypB) were cloned in pET-28a (+). Genes cypA3flag, 3flagcypB, 3flagcypBR59A/F64A, and 3flagcypBC128M were cloned in pBlueScript II SK(+) and then digested with BamHI and SacII and subcloned in pDCyaA plasmid.
Electroporation of the E. coli strain was performed with the Pulser-BioRad electroporator according to the manufacturer’s protocol.
Brucella abortus ΔcypAB mutant was genetically complemented by introducing p3flagcypA, p3flagcypB, p3flagcypBR59A/F64A, and p3flagcypBC128M plasmids by biparental mating using E. coli S17.1 as donor strain (Ditta et al., 1980). Brucella complemented strains were selected in ampicillin and nalidixic acid TSA plates, and the presence of different cyclophilins were confirmed by PCR and Western blot analysis (anti-CypA and anti-CypB antibodies).
Brucella abortus 2,308 was genetically transformed by introducing p3flagcypB or p3flagcypBR59A/F64A plasmids by biparental mating using E. coli S17.1 as donor strain (Ditta et al., 1980). Brucella transformed strains were selected in ampicillin and nalidixic acid TSA plates, and the presence of different cyclophilins were confirmed by PCR and Western blot analysis (anti-3FLAG antibody).
His-tagged recombinant proteins (CypA, CypB, CypBR59A/F64A, CypBC128M, CypBW134F CypA(L1CypB), CypB(L2CypA), CypB(L3CypA), CypB(L2-L3CypA), and GFP) were expressed in E. coli and purified using nickel affinity chromatography. Briefly, E. coli strains were grown at 37°C at 200 rpm and the expression was induced with 0.1 mM IPTG at A600 = 0.5. Two hours post-induction cells were harvested at 7000 X g and lysed by sonication. Recombinant proteins were purified from soluble fractions with the HisTrap™ HP column (GE Healthcare). Elution was performed with an imidazole gradient (20 to 500 mM). Fractions with recombinant proteins were dialyzed and quantified with NanoDrop One Microvolume UV–Vis Spectrophotometer (Thermo Fischer). Expression was confirmed by Western blotting. The predicted molecular weight of the recombinant proteins are: 21.5 kDa (CypA), 21.1 kDa (CypB), 21.0 kDa (CypBR59A/F64A), 20.2 kDa (CypBC128M), 20.1 kDa (CypBW134F), 21.0 kDa (CypA(L1CypB)), 20.9 kDa (CypB(L2CypA)), 20.5 kDa (CypB(L3CypA)), 21.3 kDa (CypB(L2-L3CypA)), and 46.6 kDa (GFP).
Protein samples were suspended in cracking buffer (2% SDS, 10% Glycerol, 60 mM Tris-Cl pH 6.8, 0.01% Bromophenol Blue, and 100 mM DTT) and incubated for 5 min at 100°C. Protein electrophoresis was performed at 120 V on a 12% SDS-PAGE gel. Gels were stained in Coomassie-Blue solution (20% methanol, 10% acetic acid).
For Western Blot analysis, proteins were transferred to a nitrocellulose membrane (Immobilon – Merck Millipore Ltd) for 55 min at 15 V using a semi-dry electroblotting transfer unit (Bio-Rad, Hercules, CA, USA). Membranes were incubated for 1 h with blocking buffer (1% dry skim milk, 0.1% Tween in PBS) and then incubated for 1 h with primary antibody diluted in blocking buffer (1/500). After washing with PBS-0.1%Tween, membranes were incubated for 1 h with secondary antibody labeled with IRDye fluorophores (LI-COR, Lincoln, NE, United States) diluted in blocking buffer (1/20,000). Finally, the membranes were scanned using the Odyssey Imaging System (LI-COR).
Proteins were quantified with the UV–Vis NanoDrop One spectrometer (Thermo Scientific).
BALB/c mice were immunized intraperitoneally with a volume of 200 μl containing 10 μg of the different purified recombinant proteins (CypA or CypB) using aluminum hydroxide as an adjuvant. Boosters with 5 μg of protein were further performed at 2 and 4 weeks. One week after the last immunization, the mice were bled, and the serum was stored at −20°C for later use.
Determination of the PPIase activity of recombinant cyclophilins was performed as described (Mares et al., 2011). Briefly, a 5 μM acid denatured Green Fluorescent Protein (GFP) solution was prepared by diluting 10 μM GFP solution in denaturation buffer (150 mM NaCl, 50 mM Tris–HCl, pH 7.5) with an equal volume of 125 mM HCl solution. The mixture was incubated for 1 min at room temperature verifying the denaturation with fluorescence measurements. Then, the 2.5 μM denatured GFP solution was diluted 1:100 in refolding buffer (25 mM MgCl2, 100 mM KCl, 50 mM Tris–HCl, pH 7.5) in the absence or presence of cyclophilins in different concentrations. The reaction was carried out in a final volume of 200 μl at room temperature measuring the fluorescence for 20 min on the FilterMax F5 spectrometer at 485 nm excitation and 538 nm emission wavelengths.
PPIase inhibition assay was performed as it was described for activity measurement but with the addition of different concentrations of CsA (0, 5, 10, 15, and 20 μM) to the reaction mix in the presence of 2 μM of cyclophilin.
To estimate change percentage in GFP refolding assay, fluorescence value corresponding to 15-min reaction was compared. Signal corresponding to the basal control (spontaneous refolding of GFP) was considered as 0% of PPIase activity. PPIase activity of CypB (that was the highest activity in our assay) was selected as 100%.
The optimal denaturation temperature for the restriction enzyme NdeI was determined. For this, 1 U of enzyme in NEB 3.1 buffer was heated at different temperatures in the range of interest for 20 min. Then, 150 ng of pET28a (+) plasmid were added and incubated for 1 h at 37°C to digest. Restriction digestion of pET28a (+) plasmid was analyzed by 1% agarose gel electrophoresis.
To analyze the chaperone activity of the recombinant proteins, the method already described was adapted (Pandey et al., 2016). Briefly, 1, 2.5, and 5 μg of CypA, CypB, CypBR59A/F64A, CypBC128M, or 5 μg of BSA (control) were mixed with 1 U of enzyme NdeI in NEB 3.1 buffer and heated at 53.8°C for 20 min (optimal denaturation temperature). The residual NdeI activity was measured by digesting 150 ng of pET28a (+) plasmid for 1 h at 37°C. The result of the digestion was analyzed by electrophoresis on 1% agarose gel, stained with ethidium bromide, and subsequent UV visualization.
HeLa cells were maintained at 37°C in a 5% CO2 atmosphere in Dulbecco modified Eagle medium (DMEM) supplemented with 5% fetal bovine serum and streptomycin (50 μg/ml)-penicillin (50 U/ml). 5 × 104 cells per well were seeded on 24-well plates and kept for 24 h in antibiotic-free DMEM. Infection with B. abortus was carried out with a multiplicity of infection (MOI) of 1,000: 1.
First, cells were incubated for 60 min with the bacteria. Then, to eliminate non-internalized bacteria, wells were washed five times with phosphate-buffered saline (PBS) and incubated with a fresh medium supplemented with 50 μg/ml gentamicin and 100 μg/ml streptomycin. Finally, infected cells at 4 h post-infection were washed with PBS five times and lysed with 500 μl 0.1% Triton X-100. Intracellular CFU was determined by plating serial dilutions in TSA with the corresponding antibiotics.
Brucella abortus cultures were adjusted to a standardized optical density at λ = 600 nm (OD600) and suspended in 1 ml of PBS. Immediately, cultures were serially diluted in PBS and plated in TSA plates containing 1,000 μg/ml deoxycholate. Plates were incubated for 3 days at 37°C.
Before inoculation, 0.1 ml of 10% sodium bicarbonate was administrated to groups of five female BALB/c mice. Oral infection was performed with a volume of 200 μl containing 109 CFU of Brucella. Mice were euthanized at 6 weeks after infection and bacteria were recovered from the spleens. Spleens were homogenized in 2 ml of PBS, and serial dilutions were plated on TSA.
Enzyme-linked immunosorbent assay was performed as published (Uriza et al., 2020). Briefly, wells were coated with 125 ng of recombinant proteins in 50 μl of buffer (0.5 M carbonate–bicarbonate pH 9.6). Wells were washed four times (0.1% Tween 20 in PBS buffer). Then, 50 μl of primary antibodies α-CypA or α-CypB per well (1:500) were incubated for 1 h. After that, wells were washed again four times and incubated for 1 h with HRP-anti-mouse IgG (1, 1,000). Finally, wells were washed and incubated for 10 min with 50 μl of substrate solution containing 3, 3′,5,5’ Tetramethylbenzidine (Sigma) and 50 μl of stopping solution for 10 min. Absorbance was measured in the FilterMax F5 Microplate Reader at 450 nm.
Protein structure modeling and comparison between CypA and CypB protein structures were conducted by SWISS-MODEL1 and UCSF Chimera 1.14 software, respectively (Meng et al., 2006).
Sequences alignment comparison of CypA and CypB was done with UCSF Chimera 1.14 (Meng et al., 2006).
GraphPad Prism 5 software was used to perform graphs and statistical analyses. One-way analysis of variance (ANOVA) with Bonferroni post-hoc test and Two-way ANOVA-Tukey’s multiple comparison test (within each row, compare columns) were used to analyze statistical significance.
A three-dimensional structural model of the cyclophilins CypA and CypB from B. abortus was built, showing that both Cyps have in common several secondary structural features. As shown in Figure 1A, the cyclophilin domain of both CypA and CypB share eight stranded antiparallel β-barrel with two α-helix covering the top and the bottom of the barrel, which is consistent with other structures from the cyclophilin family, such as the human PpiA and the E. coli EcCypB (Ke et al., 1991; Ke, 1992; Edwards et al., 1997). However, the 3D structure modeling of both cyclophilins was also able to reveal some differences in their structure over three peptide loops (Figures 1B,C). As shown in Figures 1B,C and Figure 2, catalytic active sites of both Cyps are completely conserved suggesting that both cyclophilins can potentially recognize similar protein substrates. Sequence alignment and 3D models also highlighted the presence of a critical tryptophan at position 134 of the CypB sequence (Trp134) which is absent in Brucella CypA and replaced by a phenylalanine in the equivalent position (Phe121). As shown in Figure 2, this critical tryptophan is also present within the active site of the human hCyp18 and absent in the E. coli EcCypB, where it is also replaced by phenylalanine. Of particular interest, it has been reported that this conserved tryptophan is critical for the interaction with the immunosuppressor compound cyclosporin A (CsA) in all the described eukaryotic Cyps (Bossard et al., 1991; Liu et al., 1991). Differently, in cyclophilins derived from gram negative-bacteria, this critical tryptophan is absent, a modification that correlates with the CsA-insensitivity observed for these Cyps (Liu and Walsh, 1990; Liu et al., 1991). Another feature, which is characteristic of eukaryotic cyclophilins (eCyps), is the presence of cysteines along their amino acid sequence (Liu et al., 1990) that in some cases have been reported to modulate the PPIase activity (Motohashi et al., 2003; Gourlay et al., 2007). Interestingly, Brucella CypB has a single cysteine in its sequence which is not conserved in Brucella CypA (Figure 1C).
Figure 1. Comparison of the three-dimensional structural model of Brucella abortus cyclophilins. (A) Comparison of the three-dimensional structural model for B. abortus cyclophilins CypA and CypB. Swiss-model and Chimera 1.14 programs were used for the model and to compare three-dimensional structural, respectively. The Swiss-model program selected the structure of AquaCyp293 (PDB ID: 5ex2.1A) as a template for CypA and CypB. The predicted general protein structure consists of eight antiparallel beta sheets (green) and two alpha-helices (red). (B) Differential loops detected between CypA and CypB were indicated in red and green, respectively. Dot clouds highlighted the PPIase active site areas (C) Sequence alignment of B. abortus cyclophilins. Amino acid residues involved in cyclosporin (CsA) binding are indicated by black arrows, and those involved in peptidyl-prolyl cis/trans isomerase (PPIase) activity are highlighted in gray. Cysteine is indicated by a white arrow and highlighted in black and conserved tryptophan is highlighted in light blue. Secondary structures are indicated in green arrows (beta sheets) and red boxes (alpha-helices). Differential peptide loops are marked with black boxes. The alignment was performed with the Chimera 1.4 program.
Figure 2. Active site structure of Brucella abortus cyclophilins CypB (A) and CypA (B) [in comparison with hCyp18 (2cpl.pdb) (C) and EcCypB (1Lop.pdb) (D)]. Residues that contribute to the active site of the cyclophilin family are labeled and shown in stick representation. The tryptophan involved in CsA inhibition is highlighted in red for CypB (Trp134) and hCyp18 (Trp121). Phe121 is highlighted in green for CypA and EcCypB.
Considering that differences between Brucella CypA and CypB might reflect diverse physiological functions we decided to characterize both Cyps from a biochemical and functional standpoint. With that in mind, genes encoding CypA or CypB were recombinantly expressed in E. coli BL21 (DE3) and further purified using Ni-NTA chromatography as described in Materials and Methods (Figure 3A). These recombinant proteins were used for the characterization of biochemical activities.
Figure 3. PPIase activity determination of recombinant CypA, CypB, and CypBR59A/F64A proteins. (A) SDS-PAGE of purified recombinant cyclophilins stained with Coomassie blue. (B) GFP PPIase activity assay with 2 μM of CypA, CypB, and with the point mutant in the active site CypBR59A/F64A. The figure shows the mean and standard deviation of the triplicate experiment and is representative of three independent experiments. Two-way ANOVA and Tukey’s multiple comparison test (within each row, compare columns) were used to analyze the statistical significance of the results, ****p < 0.0001.
The B. abortus cyclophilins CypA and CypB present a typical isomerase domain, containing residues involved in PPIase activity (Figures 1C, 2). To confirm if Brucella CypA and CypB are functional PPIases, their enzymatic determination was performed with purified recombinant cyclophilins, as described by Mares et al. (2011). To test cyclophilin enzymatic activity, refolding of acid-denatured GFP was adapted for PPIase determination since proline isomerization is the limiting step in the refolding process of GFP (Andrews et al., 2007). As shown in Figure 3B, the addition of CypA or CypB resulted in a highly significant improvement (p < 0.0001) in the refolding of the acid-denatured GFP confirming their true enzymatic activity. Interestingly, results from the GFP refolding assay showed that CypB presented a higher rate of reaction compared with CypA that presented an activity reduction of 25% (Figure 3B). To confirm PPIase activity, a double point mutation was performed in CypB to replace two amino acids reported to be critical for PPIase activity (R59A and F64A; Zydowsky et al., 1992). The resulting recombinant protein CypBR59A/F64A was expressed and purified (Figure 3A) and used in the GFP refolding assay. As shown in Figure 3B, CypBR59A/F64A displayed a 45% diminished PPIase activity compared with CypB.
As shown in Figures 1C, 2, a comparative analysis of the amino acid sequence between CypA and CypB revealed the presence of some features in CypB which are characteristic of eukaryotic cyclophilins like the presence of a critical tryptophan (Trp134), an amino acid predicted to be involved in the CsA interaction (Bossard et al., 1991; Liu et al., 1991). Thus, we explored the inhibition effect of CsA on the enzymatic activity of CypA and CypB of B. abortus. After the preincubation with increasing concentrations of CsA, the PPIase activity was highly significantly inhibited (p < 0.0001) in a dose-dependent manner in the case of CypB (more than 50% inhibition with 15 μM; Figure 4A), but not in CypA (Figure 4B). CsA inhibition of CypB was abolished in the case of CypBW134F mutant, where tryptophan, was replaced for phenylalanine (Figures 4C,D). Altogether, these results are in accordance with the in-silico prediction that CypB behaves in terms of CsA inhibition like a eukaryotic cyclophilin.
Figure 4. Inhibition of enzyme activity by cyclosporin A. CypB (A), CypA (B), or CypBW134F (D) were preincubated with increasing concentrations of CsA and the remaining PPIase activity was analyzed with the GFP PPIase activity assay. (C) GFP PPIase activity assay with 2 μM of CypBW134F. In inset Coomassie blue of purified CypBW134F. The figures show the mean and standard deviation of triplicate experiments and are representative of three independent experiments. Two-way ANOVA and Tukey’s multiple comparison test (within each row, compare columns) were used to analyze the statistical significance of the results, ****p < 0.0001.
It has been described that some cyclophilins, in addition to their PPIase activity, also have chaperone activity (Dimou et al., 2011; Zhang et al., 2013; Pandey et al., 2016). Therefore, we investigated whether CypA and CypB recombinant proteins of B. abortus were able to prevent the thermal denaturation of the restriction enzyme NdeI, an assay used to determine chaperone activity. The ability of NdeI to digest the pET-28a(+) plasmid in 1 h at 37°C after thermal denaturation (53.8°C for 20 min), was evaluated in the presence or absence of cyclophilins. As shown in Figure 5, pET-28a(+) plasmid (lane 1) when was incubated with native NdeI was fully digested (lane 2). In absence of cyclophilins or in presence of BSA as a control, NdeI enzyme activity was completely inactivated by the heat treatment and therefore was not able to cut and linearize the pET-28a(+) plasmid (Figure 5, lane 3–4). Interestingly, the addition of increasing concentrations of CypA or CypB during the heat treatment protected NdeI from thermal inactivation (Figure 5, lanes 5–10). These results demonstrated that both cyclophilins have chaperone-like activity. To determine if this chaperone-like activity was dependent on PPIase activity, the double point mutant CypBR59A/F64A was also analyzed. As shown in Figure 5, lanes 11–13, CypBR59A/F64A protects against thermal denaturation to the same extent as CypB, indicating that chaperone-like activity is independent of the PPIase activity.
Figure 5. CypA and CypB of B. abortus prevent thermal inactivation of NdeI activity. Enzymatic activity of thermal-denatured Nde1 (53.8°C for 20 min) in the absence or presence of CypA, CypB, CypBR59A/F64A, or BSA as a control protein. Lane 1, uncut pET28 plasmid; lane 2, pET28 plasmid incubated with native NdeI; Lane 3, pET28 plasmid incubated with heat-inactivated NdeI; Lane 4, pET28 plasmid incubated with heat-inactivated NdeI in the presence of BSA (5 μg); Lane 5, 6, and 7, pET28 plasmid incubated with heat-inactivated NdeI in the presence of increasing concentrations of CypA protein (1, 2.5, and 5 μg); Lane 8, 9, and 10, pET28 plasmid incubated with heat-inactivated NdeI in the presence of increasing concentrations of CypB protein (1, 2.5, and 5 μg); Lane 11, 12, and 13, pET28 plasmid incubated with heat-inactivated NdeI in the presence of increasing concentrations of CypBR59A/F64A protein (1, 2.5, and 5 μg). The gel is representative of three independent experiments.
To generate molecular tools for this research, recombinant proteins CypA and CypB were used as antigens to produce antibodies in mice. As shown in Figure 6A, when evaluating mouse sera, we found that antibodies raised against CypA were not able to recognize the recombinant CypB and vice versa. As shown in Figure 6B, the same was also observed in whole-cell lysates of B. abortus 2,308, B. abortus ∆cypAB (pcypA), and B. abortus ∆cypAB(pcypB). As expected, no signal was detected for the whole-cell lysate of B. abortus ∆cypAB mutant either with α-CypA or α-CypB antibodies. These results are remarkable because, although CypA and CypB share a 63% of identity in the amino acid sequence, the immune system still was able to reveal structural differences existing between both cyclophilins. As shown in Figure 6C,D, these antigenic differences can be potentially mapped on three differential amino acid loops. Interestingly, analysis of CypA and CypB sequences by the BepiPred-2.0 algorithm predicted a series of differential linear epitopes that can be located within these differential loops (Figures 6C,D). To pinpoint which peptide loop is contributing to the antibody differential recognition, an experimental approach of swapping loops between CypA and CypB was performed (Figure 6E). Based on the 3D structure prediction, it was observed that formation of loop-1 was determined by the interloop (i-Loop) region (Figures 6D,E). Consequently, we swapped loop1 + i-Loop either of CypA or CypB. To analyze linear and conformational epitopes of CypA, CypB, and their derived chimeras, Western blot analysis, and ELISA tests were carried out (Figure 6E). Thus, the replacement of loop-1 of CypA by the loop-1 from CypB (see CypA(L1CypB) in Figure 6E) determined the lack of recognition of α-CypA antibody and the gain of recognition of α-CypB antibody indicating that loop-1 contained a major determinant of the differential immunogenicity of B. abortus Cyps. In addition, loop-2 of CypA was also important for the recognition of α-CypA antibody since CypB(L2CypA) and CypB(L2-L3CypA) were recognized by α-CypA antibodies. Analysis of CypB(L3CypA) immunogenicity suggested that loop-3 of both Cyps is devoid of immunodominant epitopes. Altogether these immunological results confirmed structural differences predicted by the in-silico analysis between CypA and CypB.
Figure 6. Differential immunodominant loops between CypA and CypB. Western blot analysis of (A) CypA and CypB recombinant proteins and (B) different Brucella whole-cell lysates probed with mouse antibodies produced against CypA or CypB. (C) Overlap of the three-dimensional structural model for B. abortus cyclophilins CypA and CypB. Swiss-model and Chimera 1.14 programs were used for modeling and the comparison of the predicted three-dimensional structure, respectively. The BepiPred-2.0 algorithm was used to predict linear epitopes. Immunodominant epitopes of CypA and CypB are marked in red and green, respectively. (D) Sequence alignment of B. abortus cyclophilins. Loops and i-Loop are indicated in a black open box. Linear epitopes are indicated in green open boxes for CypA or red open boxes for CypB. (E) Western blot analysis and indirect ELISA of different recombinant chimeras of CypA and CypB were revealed with anti-CypA or anti-CypB antibodies. The results are representative of three independent experiments.
Since it was reported that PPIases can form homo-oligomers (Budiman et al., 2009; Zhang et al., 2011; Jakob et al., 2016; Polley et al., 2016) we studied the possibility that CypA and CypB were able to oligomerize. As shown in Figure 7A, when we subjected CypA or CypB to SDS-PAGE and Western blot analysis, a single protein band corresponding to the recombinant CypA or CypB was detected as expected for the monomeric form of these cyclophilins. However, when samples were treated in a non-reducing condition (without DTT in the loading sample) in addition to the monomeric band of CypB or CypBR59A/F64A, a larger band compatible with the formation of a homodimer of CypB or CypBR59AF64A was observed (Figure 7A). Interestingly, CypA either in the presence or absence of DTT was observed like a single band, as expected for a monomeric CypA (Figure 7A). These results indicate that CypB but not CypA was able to interact to form homodimers in solution, a process that was independent of the PPIase activity.
Figure 7. CypB is a homodimeric protein. Coomassie blue staining and Western blot analysis of protein samples incubated in the presence or absence of DTT. (A) CypB, CypBR59A/F64A, CypA, (B) CypB and CypBC128M. (C) GFP PPIase activity assay with 2 μM of CypB, CypBC128M, or without CypB. (D) Enzymatic activity of thermal-denatured Nde1 (53.8°C for 20 min) in the presence of CypB, CypBC128M, or control protein BSA. Lane 1, uncut pET28 plasmid; Lane 2, pET28 plasmid incubated with native NdeI; Lane 3, pET28 plasmid incubated with heat-inactivated NdeI; Lane 4, pET28 plasmid incubated with heat-inactivated NdeI in the presence of BSA (5 μg), Lane 5, 6, and 7, pET28 plasmid incubated with heat-inactivated NdeI in the presence of increasing concentrations of CypB protein (1, 2.5, and 5 μg); Lane 8, 9, and 10, pET28 plasmid incubated with heat-inactivated NdeI in the presence of increasing concentrations of CypBC128M protein (1, 2.5, and 5 μg). The results are representative of three independent experiments.
As shown above, a comparison between both cyclophilin sequences showed that CypB has a unique cysteine residue which is absent in the CypA sequence (Figure 1C). To study the potential role of this conserved cysteine residue in dimer formation, a point mutant CypBC128M was constructed, where the Cys128 was replaced by methionine (Figure 7B). The resulting recombinant protein CypBC128M was expressed and purified and its ability to form homodimers was evaluated. As shown in Figure 7B, CypBC128M was not able to form homodimers in non-reducing conditions indicating that cysteine was responsible for CypB dimerization. In addition, it was interesting to investigate if Cys128 was also important for PPIase or chaperone activities of CypB. As shown in Figure 7C, the PPIase activity of CypBC128M was not different from CypB. In addition, results shown in Figure 7D indicated that CypBC128M was also able to protect NdeI from thermal denaturation as efficiently as CypB. Altogether these results showed that the loss of the ability to form homodimers does not affect the in vitro activities of CypB analyzed in this study.
Although the loss of CypB ability to form homodimers showed no effect on the in vitro PPIase or chaperone activities, we explored if CypB oligomerization can affect in vivo functions of CypB in B. abortus related to stress and intracellular host-cell adaptation. For that, the plasmid pfcypBC128M was introduced into B. abortus ∆cypAB mutant strain and the stress adaptation, intracellular survival, and virulence in mice were examined (Figure 8). The expression of CypBC128M protein in B. abortus was confirmed by Western blot analysis (Figure 8A). As described previously in Roset et al. (2013) ∆cypAB deletion generates a phenotype in B. abortus characterized by an increased sensitivity to a set of different stressors such as deoxycholate acid (DOC). As shown in Figure 8B, the plasmid pfcypBC128M was unable to complement the DOC sensitivity of B. abortus ΔcypAB mutant. These results indicated that CypB homodimer formation is necessary for Brucella stress adaptation.
Figure 8. Dimeric form of CypB is required for survival and virulence of B. abortus. (A) Western blot analysis of whole-cell lysates of B. abortus strains expressing wild-type CypB, the monomeric form CypBC128M, and the active site mutant CypBR59A/F64A detected by a mouse antibody against CypB. (B) Detergent sensitivity assay using serial dilutions of different B. abortus strains plated in triplicate onto TSB agar plates containing deoxycholate (DOC) (1,000 μg/ml). Plates were incubated for 72 h, and the number of CFU was scored. (C) Intracellular survival of the B. abortus strains in HeLa cells. Numbers of CFU of intracellular bacteria were determined after lysis of infected cells at 4 h post-infection. Each determination was performed in triplicate, and values are shown as the mean with its respective standard deviation and are representative of three independent experiments. (D) BALB/c mice were infected orally (1 × 109 CFU) with different B. abortus strains. At 6 weeks post-infection, the numbers of CFU recovered from spleens were determined by serial dilutions and plating onto TSA. Five animals were used for each determination. One-way ANOVA and Bonferroni’s Multiple Comparison Test were used to analyze the statistical significance of the results. *, p < 0.05, **, p < 0.01, *** p < 0.001.
To examine if the homodimeric formation of CypB is required for B. abortus intracellular survival, Hela cells were infected with B. abortus 2,308 wild-type strain, B. abortus ΔcypAB mutant, B. abortus ΔcypAB(pfcypB), B. abortus ΔcypAB(pfcypBR59A/F64A) and, B. abortus ΔcypAB(pfcypBC128M) (Figure 8C). Results showed that at 4 h post-infection B. abortus ΔcypAB(pfcypBC128M) showed a 10-fold reduction in intracellular survival, like what was observed in B. abortus ΔcypAB mutant suggesting that the dimeric form of CypB was also necessary for intracellular survival in Hela cells. Since B. abortus ΔcypAB(pfcypBC128M) was affected in intracellular adaptation it was interesting to investigate if this mutant was also affected in the mouse infection model. As shown in Figure 8D, orally infected mice had a reduced number (more than hundred-fold decrease) of B. abortus ΔcypAB(pfcypBC128M) in spleen at 6 weeks post-infection compared with those infected with B. abortus ΔcypAB(pfcypB) and like B. abortus ΔcypAB mutant. These results also showed that the dimeric form of Brucella CypB was fully required to establish a persistent infection in mice. All these results showed that homodimer formation was necessary for the in vivo functions of CypB.
As we described previously (Roset et al., 2013), the plasmid pcypBR59A/F64A partially rescued the B. abortus ΔcypAB mutant for DOC sensitivity (Figure 8B). Intermediate results observed in complementation assays with pcypBR59A/F64A can be explained by the existence of certain residual PPIase activity in the cyclophilin mutant CypBR59A/F64A (Figure 3B). This CypBR59A/F64A residual activity was sufficient to complement intracellular survival of the B. abortus ΔcypAB mutant to the wild-type level (Figure 8C; Roset et al., 2013). To understand if the residual cyclophilin activity present in the mutant protein CypBR59A/F64A was also sufficient to complement the B. abortus ΔcypAB mutant in the mouse model, an infection experiment was performed. As shown in Figure 8D, after 6 weeks post-infection B. abortus ΔcypAB(pfcypBR59A/F64A) was 66-fold less efficient in spleen colonization in BALB/c mice than B. abortus ΔcypAB(pfcypB) and similar to B. abortus ΔcypAB mutant. It is possible to speculate that the discrepancies observed can be explained by the different requirements of CypB activity dependent on the chosen experimental model. Thus, mouse oral infection is predicted to be the most demanding assay since B. abortus ΔcypAB mutant had to face a variety of sequential stressors when progressing in the intestinal tract (pH, bile salts, proteases, etc). Consequently, the mouse experiment highlighted the critical importance of CypB PPIase activity in Brucella intracellular survival and virulence.
To explore if the loss-of-function mutation of CypB (cypBR59A/F64A) can exert a dominant-negative effect on B. abortus 2,308 wild-type strain, the plasmid pfcypBR59AF64A was introduced into this strain by biparental mating and ectopic expression of CypBR59A/F64A was confirmed by Western blot analysis (Figure 9A). As shown in Figure 9, the expression of CypBR59A/F64A impaired the ability of Brucella to survive in DOC sensitivity assay (Figure 9B) and reduced 70-fold the ability to survive within HeLa cells (Figure 9C). Considering that CypBR59A/F64A still can interact with CypB wild type to form dimers, these results might suggest that CypB requires dimer formation for full activity, having both monomers a fully intact active site.
Figure 9. Dominant-negative mutation. (A) Western blot analysis of whole-cell lysates of B. abortus 2,308 strain expressing wild-type CypB, or CypBR59A/F64A detected by an anti-flag antibody. The strains were assayed for detergent sensitivity (DOC) (B), and survival in HeLa cells (C) as described in “Materials and methods.” One-way ANOVA and Bonferroni’s Multiple Comparison Test were used to analyze the statistical significance of the results. The results shown are representative of three independent experiments, **p < 0.01, ***p < 0.001.
Cyclophilins are a family of highly conserved enzymes that catalyze the process of cis-trans isomerization of the Xaa-proline bonds, which is the rate-limiting step in protein folding. This activity is critical for many biological processes including bacterial virulence (Dimou et al., 2017). We have previously reported that B. abortus has two cyclophilins, named cyclophilin A (CypA) and cyclophilin B (CypB) that are upregulated within the intraphagosomal replicative niche during B. abortus infection (Roset et al., 2013). In addition, we have also demonstrated that both cyclophilins play an important role in stress adaptation, intracellular survival, and virulence. Interestingly, defective phenotypes for stress and intracellular survival can be complemented either with CypA or CypB alone, suggesting that both Cyps show certain redundancy in their functions (Roset et al., 2013). In this report, we have also identified a group of differential features by comparing CypA with CypB. For instance, although both B. abortus cyclophilins share a conserved secondary structure, we have identified structural differences over three immunodominant loops identified by an in-silico approach and by different immunoassays. Cyclophilins are enzymes that can interact with different protein targets to help them in their folding process allowing them to acquire their functional protein structure. It would be interesting to hypothesize if differences observed in structure and antigenicity of CypA and CypB might also be reflecting differences in their preferred protein targets that can reveal novel functions for these cyclophilins.
We demonstrated here that unlike CypA, CypB presents a Trp134 which is involved in CsA inhibition, like in all characterized Cyps from eukaryotic origin (Bossard et al., 1991; Liu et al., 1991). On the other hand, CypA shares homology with Cyps of Gram-negative bacteria, presenting a replacement of the Trp134 by a Phe residue, a modification that correlates with CsA insensitivity observed for this kind of Cyps (Liu and Walsh, 1990; Liu et al., 1991). Interestingly, the replacement of Trp134 in CypB (CypBW134F) does not modify the PPIase activity either in the presence or absence of CsA indicating that Trp134 is not required for peptidyl-prolyl cis-trans isomerization. As mentioned above, another characteristic feature that has been described in eukaryotic cyclophilins is the presence of cysteines in their sequences (Liu et al., 1990; Motohashi et al., 2003; Gourlay et al., 2007). Brucella CypB has a single cysteine residue in its sequence (Cys128) which is absent in CypA. As shown here, Brucella CypB has the capacity to self-associate to form homodimers. This interaction was reversed by the addition of the reducing agent DTT indicating that Cys128 is the amino acid responsible for dimer formation. Thus, CypBC128M mutant was unable to form homodimers showing the same behavior as CypA which is only present in a monomeric state.
It has been reported that PPIases can form homo-oligomers (Zhang et al., 2011; Jakob et al., 2016; Polley et al., 2016) although, in the family of cyclophilins, only a few cases have been reported: the human hCypA (Zhang et al., 2011) and the Trichomonas vaginalis cyclophilin 1 (Martin et al., 2018). In addition, in the case of dimeric PPIases, no homodimer formation has been reported dependent on disulfide bonds. Hence, Brucella CypB is the first example of a dimeric PPIase stabilized by disulfide bridges.
Interestingly, it has been also reported that oligomerization of enzymes modulates their activities (Kropp et al., 2022). We showed here that CypB dimer formation was fully required to complement the defective phenotype of Brucella ΔcypAB mutant in stress survival, intracellular adaptation, and virulence in mice but not required for the in vitro PPIase activity. This apparent discrepancy can be explained because the in vitro PPIase substrate, the acid-denatured GFP, is not expected to be a physiological protein target. Similar results to those described here were reported for Legionella protein MIP, a PPIase belonging to the FKB family (Kohler et al., 2003).
As shown here, the defective phenotype of B. abortus ΔcypAB mutant cannot be complemented by CypBC128M indicating that the formation of homodimers is critical for PPIase activity of CypB.
To understand in more detail how Brucella cyclophilins participate in the process of stress adaptation and intracellular survival, a dominant-negative experiment was performed. As shown here, the over-expression of CypBR59A/F64A interfered with bacterial ability to survive to stressors and within the host cell resembling what is observed in the B. abortus ΔcypAB mutant. Interpretations of these results suggested that for the fully enzymatic activity of the dimeric CypB, both monomers must present functional active sites. As we mentioned before and it was shown previously (Roset et al., 2013), CypA and CypB are equivalent when complemented the B. abortus ΔcypAB mutant indicating a functional redundancy. Interestingly, in the dominant-negative experiment, the wild-type activity of CypA was also surprisingly inhibited, although CypA is not expected to form heterodimers with CypBR59A/F64A. An explanation for these results can be that homodimers formed by CypBR59A/F64A or heterodimers formed by CypB-CypBR59A/F64A were able to trap also the protein targets of CypA preventing their folding.
It has been reported that there is a general link between cyclophilins and cellular stress response (Dimou et al., 2017). We have described that Brucella’s Cyps participate in survival to diverse types of stresses which is dependent on the PPIase activity (Roset et al., 2013). Proteins involved in oxidative stress such as OxyR and Hsp33 are regulated by redox activity and activated by the formation of intramolecular disulfide bridges (Zheng et al., 1998; Graumann et al., 2001). Moreover, evidence for redox regulation of PPIase activity of cyclophilins has been reported for hCypA from T lymphocytes (Ghezzi et al., 2006), Chloroplast CypA from Arabidopsis thaliana (Motohashi et al., 2003), and CypA from Schistosoma mansoni (Gourlay et al., 2007), suggesting that redox regulation involving cysteine residues must be a common mechanism of cyclophilin regulation. In all the mentioned cases, activity regulation is mediated by the formation of intramolecular disulfide bridges. It is conceivable that in the case of Brucella, upon exposure to an oxidative stress condition (for instance when the bacterium enters its host cell) the intermolecular formation of disulfide bridges between two monomers of CypB can be triggered to obtain fully functional PPIase activity. Thus, the dimerization of CypB might function as a regulatory switch to respond to oxidative stress in Brucella.
Bacterial pathogens that have co-evolved with their host have acquired mechanisms to modulate the host cell physiology for their own benefit. Thus, pathogenic bacteria can translocate virulence proteins (known as effector proteins) into the host cell, using specialized secretion systems to hijack different processes, such as the acquisition of nutrients, vesicle trafficking, and modulation of the immune system to allow adequate time for bacterial replication. To accomplish these functions, some effector proteins use “eukaryotic-like” protein domains to mimic the structure or the function of host proteins, promoting the manipulation of a particular host cell pathway (Ke et al., 2015). In the light of, i) Brucella Cyps are overexpressed during B. abortus intracellular life, ii) Brucella Cyps are required for stress adaptation, intracellular survival, and virulence in BALB/c mice, iii) Brucella CypA and CypB differ in immunodominant loops that may be reflecting differential functions as well, iv) Brucella CypB has structural and functional eukaryotic characteristics, it is possible to hypothesize that CypB might function as an effector protein. Further studies to understand if CypB can function as an effector bacterial protein are still in progress.
Remarkably, we have shown here that Brucella cyclophilins come in two different “flavors”: eukaryotic and prokaryotic. In addition, we reported here that Brucella cyclophilins CypA and CypB differ in various immunological and biochemical properties, despite their high degree of sequence similarity and conserved functional features. Also, we highlighted the importance of dimer formation and PPIase activity of CypB for a progressive infection in an animal model. These findings shed some light on the potential novel functions of Brucella Cyps, some of them could be due to the putative role of CypB as an effector bacterial protein.
The original contributions presented in the study are included in the article/supplementary material, further inquiries can be directed to the corresponding authors.
The animal study was reviewed and approved by The experimental procedure of this study (permit number CICUAE UNSAM 15/2018) was approved by the Committee on the Ethics of Animal Experiments of the Universidad Nacional de San Martín (UNSAM), under the recommendations for animal experimentation (Helsinki Declaration and its amendments, Amsterdam Protocol of welfare and animal protection and National Institutes of Health, USA NIH, guidelines: Guide for the Care and Use of Laboratory Animals).
MR and GB conceived and design the experiments. EM and MR performed experiments. EM, MR, and GB analyzed the data. MR and GB wrote the paper. All authors contributed to the article and approved the submitted version.
This work was supported by grants from the Agencia Nacional de Promoción Científica y Tecnológica, Buenos Aires, Argentina (PICT-2018-0778, PICT-2016-0412) and CONICET (PUE-0086, PIP-11220200102517CO).
We would like to thank Dr. Rodrigo Sieira for his careful and critical reading of this manuscript. EM is doctoral fellow from CONICET. MR and GB are members of the Research Career of CONICET.
The authors declare that the research was conducted in the absence of any commercial or financial relationships that could be construed as a potential conflict of interest.
All claims expressed in this article are solely those of the authors and do not necessarily represent those of their affiliated organizations, or those of the publisher, the editors and the reviewers. Any product that may be evaluated in this article, or claim that may be made by its manufacturer, is not guaranteed or endorsed by the publisher.
Andrews, B. T., Schoenfish, A. R., Roy, M., Waldo, G., and Jennings, P. A. (2007). The rough energy landscape of superfolder GFP is linked to the chromophore. J. Mol. Biol. 373, 476–490. doi: 10.1016/j.jmb.2007.07.071
Bossard, M. J., Koser, P. L., Brandt, M., Bergsma, D. J., and Levy, M. A. (1991). A single Trp121 to Ala121 mutation in human cyclophilin alters cyclosporin a affinity and peptidyl-prolyl isomerase activity. Biochem. Biophys. Res. Commun. 176, 1142–1148. doi: 10.1016/0006-291X(91)90404-U
Budiman, C., Bando, K., Angkawidjaja, C., Koga, Y., Takano, K., and Kanaya, S. (2009). Engineering of monomeric FK506-binding protein 22 with peptidyl prolyl cis-trans isomerase. Importance of a V-shaped dimeric structure for binding to protein substrate. FEBS J. 276, 4091–4101. doi: 10.1111/j.1742-4658.2009.07116.x
Bzdyl, N. M., Scott, N. E., Norville, I. H., Scott, A. E., Atkins, T., Pang, S., et al. (2019). Peptidyl-prolyl isomerase ppiB is essential for proteome homeostasis and virulence in Burkholderia pseudomallei. Infect. Immun. 87. doi: 10.1128/IAI.00528-19
Cho, K., Arimoto, T., Igarashi, T., and Yamamoto, M. (2013). Involvement of lipoprotein PpiA of Streptococcus gordonii in evasion of phagocytosis by macrophages. Mol. Oral Microbiol. 28, 379–391. doi: 10.1111/omi.12031
Corbel, M. J. (1997). Brucellosis: an overview. Emerg. Infect. Dis. 3, 213–221. doi: 10.3201/eid0302.970219
De Figueiredo, P., Ficht, T. A., Rice-Ficht, A., Rossetti, C. A., and Adams, L. G. (2015). Pathogenesis and immunobiology of brucellosis: review of Brucella-host interactions. Am. J. Pathol. 185, 1505–1517. doi: 10.1016/j.ajpath.2015.03.003
Dimou, M., Venieraki, A., and Katinakis, P. (2017). Microbial cyclophilins: specialized functions in virulence and beyond. World J. Microbiol. Biotechnol. 33:164. doi: 10.1007/s11274-017-2330-6
Dimou, M., Venieraki, A., Liakopoulos, G., Kouri, E. D., Tampakaki, A., and Katinakis, P. (2011). Gene expression and biochemical characterization of Azotobacter vinelandii cyclophilins and protein interaction studies of the cytoplasmic isoform with dnaK and lpxH. J. Mol. Microbiol. Biotechnol. 20, 176–190. doi: 10.1159/000329486
Ditta, G., Stanfield, S., Corbin, D., and Helinski, D. R. (1980). Broad host range DNA cloning system for gram-negative bacteria: construction of a gene bank of rhizobium meliloti. Proc. Natl. Acad. Sci. U. S. A. 77, 7347–7351. doi: 10.1073/pnas.77.12.7347
Edwards, K. J., Ollis, D. L., and Dixon, N. E. (1997). Crystal structure of cytoplasmic Escherichia coli peptidyl-prolyl isomerase: evidence for decreased mobility of loops upon complexation. J. Mol. Biol. 271, 258–265. doi: 10.1006/jmbi.1997.1151
Fanghanel, J., and Fischer, G. (2004). Insights into the catalytic mechanism of peptidyl prolyl cis/trans isomerases. Front. Biosci. 9, 3453–3478. doi: 10.2741/1494
Fina Martin, J., Palomino, M. M., Cutine, A. M., Modenutti, C. P., Fernandez Do Porto, D. A., Allievi, M. C., et al. (2019). Exploring lectin-like activity of the S-layer protein of Lactobacillus acidophilus ATCC 4356. Appl. Microbiol. Biotechnol. 103, 4839–4857. doi: 10.1007/s00253-019-09795-y
Galat, A. (2003). Peptidylprolyl cis/trans isomerases (immunophilins): biological diversity--targets--functions. Curr. Top. Med. Chem. 3, 1315–1347. doi: 10.2174/1568026033451862
Ghezzi, P., Casagrande, S., Massignan, T., Basso, M., Bellacchio, E., Mollica, L., et al. (2006). Redox regulation of cyclophilin A by glutathionylation. Proteomics 6, 817–825. doi: 10.1002/pmic.200500177
Gourlay, L. J., Angelucci, F., Baiocco, P., Boumis, G., Brunori, M., Bellelli, A., et al. (2007). The three-dimensional structure of two redox states of cyclophilin A from Schistosoma mansoni. Evidence for redox regulation of peptidyl-prolyl cis-trans isomerase activity. J. Biol. Chem. 282, 24851–24857. doi: 10.1074/jbc.M702714200
Graumann, J., Lilie, H., Tang, X., Tucker, K. A., Hoffmann, J. H., Vijayalakshmi, J., et al. (2001). Activation of the redox-regulated molecular chaperone Hsp33--a two-step mechanism. Structure 9, 377–387. doi: 10.1016/S0969-2126(01)00599-8
Hermans, P. W., Adrian, P. V., Albert, C., Estevao, S., Hoogenboezem, T., Luijendijk, I. H., et al. (2006). The streptococcal lipoprotein rotamase a (SlrA) is a functional peptidyl-prolyl isomerase involved in pneumococcal colonization. J. Biol. Chem. 281, 968–976. doi: 10.1074/jbc.M510014200
Herrero, M., De Lorenzo, V., and Timmis, K. N. (1990). Transposon vectors containing non-antibiotic resistance selection markers for cloning and stable chromosomal insertion of foreign genes in gram-negative bacteria. J. Bacteriol. 172, 6557–6567. doi: 10.1128/jb.172.11.6557-6567.1990
Jakob, R. P., Schmidpeter, P. A., Koch, J. R., Schmid, F. X., and Maier, T. (2016). Structural and functional characterization of a novel family of Cyclophilins, the AquaCyps. PLoS One 11:e0157070. doi: 10.1371/journal.pone.0157070
Ke, H. (1992). Similarities and differences between human cyclophilin A and other beta-barrel structures. Structural refinement at 1.63 A resolution. J. Mol. Biol. 228, 539–550. doi: 10.1016/0022-2836(92)90841-7
Ke, Y., Wang, Y., Li, W., and Chen, Z. (2015). Type IV secretion system of Brucella spp. and its effectors. Front. Cell. Infect. Microbiol. 5:72. doi: 10.3389/fcimb.2015.00072
Ke, H. M., Zydowsky, L. D., Liu, J., and Walsh, C. T. (1991). Crystal structure of recombinant human T-cell cyclophilin A at 2.5 a resolution. Proc. Natl. Acad. Sci. U. S. A. 88, 9483–9487. doi: 10.1073/pnas.88.21.9483
Keogh, R. A., Zapf, R. L., Wiemels, R. E., Wittekind, M. A., and Carroll, R. K. (2018). The intracellular Cyclophilin PpiB contributes to the virulence of Staphylococcus aureus independently of its peptidyl-prolyl cis/trans isomerase activity. Infect. Immun. 86. doi: 10.1128/IAI.00379-18
Kohler, R., Fanghanel, J., Konig, B., Luneberg, E., Frosch, M., Rahfeld, J. U., et al. (2003). Biochemical and functional analyses of the Mip protein: influence of the N-terminal half and of peptidylprolyl isomerase activity on the virulence of legionella pneumophila. Infect. Immun. 71, 4389–4397. doi: 10.1128/IAI.71.8.4389-4397.2003
Kropp, C., Bruckmann, A., and Babinger, P. (2022). Controlling enzymatic activity by modulating the Oligomerization state via chemical rescue and optical control. Chembiochem 23:e202100490. doi: 10.1002/cbic.202100490
Krucken, J., Greif, G., and Von Samson-Himmelstjerna, G. (2009). In silico analysis of the cyclophilin repertoire of apicomplexan parasites. Parasit. Vectors 2:27. doi: 10.1186/1756-3305-2-27
Kumawat, M., Singh, P. K., Rananaware, S. R., and Ahlawat, S. (2020). Comparative evaluation of structure and characteristic of peptidyl-prolyl cis-trans isomerase proteins and their function in Salmonella Typhimurium stress responses and virulence. Folia Microbiol. (Praha) 65, 161–171. doi: 10.1007/s12223-019-00717-z
Liu, J., Albers, M. W., Chen, C. M., Schreiber, S. L., and Walsh, C. T. (1990). Cloning, expression, and purification of human cyclophilin in Escherichia coli and assessment of the catalytic role of cysteines by site-directed mutagenesis. Proc. Natl. Acad. Sci. U. S. A. 87, 2304–2308. doi: 10.1073/pnas.87.6.2304
Liu, J., Chen, C. M., and Walsh, C. T. (1991). Human and Escherichia coli cyclophilins: sensitivity to inhibition by the immunosuppressant cyclosporin A correlates with a specific tryptophan residue. Biochemistry 30, 2306–2310. doi: 10.1021/bi00223a003
Liu, J., and Walsh, C. T. (1990). Peptidyl-prolyl cis-trans-isomerase from Escherichia coli: a periplasmic homolog of cyclophilin that is not inhibited by cyclosporin A. Proc. Natl. Acad. Sci. U. S. A. 87, 4028–4032. doi: 10.1073/pnas.87.11.4028
Marchesini, M. I., Herrmann, C. K., Salcedo, S. P., Gorvel, J. P., and Comerci, D. J. (2011). In search of Brucella abortus type IV secretion substrates: screening and identification of four proteins translocated into host cells through VirB system. Cell. Microbiol. 13, 1261–1274. doi: 10.1111/j.1462-5822.2011.01618.x
Mares, R. E., Melendez-Lopez, S. G., and Ramos, M. A. (2011). Acid-denatured green fluorescent protein (GFP) as model substrate to study the chaperone activity of protein disulfide isomerase. Int. J. Mol. Sci. 12, 4625–4636. doi: 10.3390/ijms12074625
Martin, T., Lou, Y. C., Chou, C. C., Wei, S. Y., Sadotra, S., Cho, C. C., et al. (2018). Structural basis of interaction between dimeric cyclophilin 1 and Myb1 transcription factor in trichomonas vaginalis. Sci. Rep. 8:5410. doi: 10.1038/s41598-018-23821-5
Meng, E. C., Pettersen, E. F., Couch, G. S., Huang, C. C., and Ferrin, T. E. (2006). Tools for integrated sequence-structure analysis with UCSF chimera. BMC Bioinformatics 7:339. doi: 10.1186/1471-2105-7-339
Motohashi, K., Koyama, F., Nakanishi, Y., Ueoka-Nakanishi, H., and Hisabori, T. (2003). Chloroplast cyclophilin is a target protein of thioredoxin. Thiol modulation of the peptidyl-prolyl cis-trans isomerase activity. J. Biol. Chem. 278, 31848–31852. doi: 10.1074/jbc.M304258200
Obi, I. R., Nordfelth, R., and Francis, M. S. (2011). Varying dependency of periplasmic peptidylprolyl cis-trans isomerases in promoting Yersinia pseudotuberculosis stress tolerance and pathogenicity. Biochem. J. 439, 321–332. doi: 10.1042/BJ20110767
Pandey, S., Sharma, A., Tripathi, D., Kumar, A., Khubaib, M., Bhuwan, M., et al. (2016). Mycobacterium tuberculosis peptidyl-prolyl isomerases also exhibit chaperone like activity in-vitro and in-vivo. PLoS One 11:e0150288. doi: 10.1371/journal.pone.0150288
Pandey, S., Tripathi, D., Khubaib, M., Kumar, A., Sheikh, J. A., Sumanlatha, G., et al. (2017). Mycobacterium tuberculosis peptidyl-prolyl isomerases are immunogenic, alter cytokine profile and aid in intracellular survival. Front. Cell. Infect. Microbiol. 7:38. doi: 10.3389/fcimb.2017.00038
Pemberton, T. J. (2006). Identification and comparative analysis of sixteen fungal peptidyl-prolyl cis/trans isomerase repertoires. BMC Genomics 7:244. doi: 10.1186/1471-2164-7-244
Polley, S., Chakravarty, D., Chakrabarti, G., and Sau, S. (2016). Determining the roles of a conserved tyrosine residue in a Mip-like peptidyl-prolyl cis-trans isomerase. Int. J. Biol. Macromol. 87, 273–280. doi: 10.1016/j.ijbiomac.2016.02.070
Rasch, J., Unal, C. M., Klages, A., Karsli, U., Heinsohn, N., Brouwer, R., et al. (2019). Peptidyl-prolyl-cis/trans-isomerases Mip and PpiB of legionella pneumophila contribute to surface translocation, growth at suboptimal temperature, and infection. Infect. Immun. 87. doi: 10.1128/IAI.00939-17
Reffuveille, F., Connil, N., Sanguinetti, M., Posteraro, B., Chevalier, S., Auffray, Y., et al. (2012). Involvement of peptidylprolyl cis/trans isomerases in Enterococcus faecalis virulence. Infect. Immun. 80, 1728–1735. doi: 10.1128/IAI.06251-11
Roset, M. S., Alefantis, T. G., Delvecchio, V. G., and Briones, G. (2017). Iron-dependent reconfiguration of the proteome underlies the intracellular lifestyle of Brucella abortus. Sci. Rep. 7:10637. doi: 10.1038/s41598-017-11283-0
Roset, M. S., Garcia Fernandez, L., Delvecchio, V. G., and Briones, G. (2013). Intracellularly induced cyclophilins play an important role in stress adaptation and virulence of Brucella abortus. Infect. Immun. 81, 521–530. doi: 10.1128/IAI.01125-12
Uriza, P. J., Trautman, C., Palomino, M. M., Fina Martin, J., Ruzal, S. M., Roset, M. S., et al. (2020). Development of an antigen delivery platform using Lactobacillus acidophilus decorated with heterologous proteins: a sheep in Wolf's clothing story. Front. Microbiol. 11:509380. doi: 10.3389/fmicb.2020.509380
Wiemels, R. E., Cech, S. M., Meyer, N. M., Burke, C. A., Weiss, A., Parks, A. R., et al. (2017). An intracellular peptidyl-prolyl cis/trans isomerase is required for folding and activity of the Staphylococcus aureus secreted virulence factor nuclease. J. Bacteriol. 199. doi: 10.1128/JB.00453-16
Zhang, X. C., Wang, W. D., Wang, J. S., and Pan, J. C. (2013). PPIase independent chaperone-like function of recombinant human Cyclophilin A during arginine kinase refolding. FEBS Lett. 587, 666–672. doi: 10.1016/j.febslet.2013.01.028
Zhang, X. C., Wang, W. D., Wang, J. S., Pan, J. C., and Zou, G. L. (2011). Evidences of monomer, dimer and trimer of recombinant human cyclophilin A. Protein Pept. Lett. 18, 1188–1193. doi: 10.2174/092986611797642670
Zheng, M., Aslund, F., and Storz, G. (1998). Activation of the OxyR transcription factor by reversible disulfide bond formation. Science 279, 1718–1722. doi: 10.1126/science.279.5357.1718
Zydowsky, L. D., Etzkorn, F. A., Chang, H. Y., Ferguson, S. B., Stolz, L. A., Ho, S. I., et al. (1992). Active site mutants of human cyclophilin A separate peptidyl-prolyl isomerase activity from cyclosporin A binding and calcineurin inhibition. Protein Sci. 1, 1092–1099. doi: 10.1002/pro.5560010903
Keywords: brucellosis, Brucella abortus, Cyclophilins, virulence, PPIase activity, dimeric CypB, Brucella-host interaction, stress adaptation
Citation: Muruaga EJ, Briones G and Roset MS (2022) Biochemical and functional characterization of Brucella abortus cyclophilins: So similar, yet so different. Front. Microbiol. 13:1046640. doi: 10.3389/fmicb.2022.1046640
Received: 17 September 2022; Accepted: 13 October 2022;
Published: 31 October 2022.
Edited by:
Roy Martin Roop II, East Carolina University, United StatesReviewed by:
Clayton Caswell, Virginia Tech, United StatesCopyright © 2022 Muruaga, Briones and Roset. This is an open-access article distributed under the terms of the Creative Commons Attribution License (CC BY). The use, distribution or reproduction in other forums is permitted, provided the original author(s) and the copyright owner(s) are credited and that the original publication in this journal is cited, in accordance with accepted academic practice. No use, distribution or reproduction is permitted which does not comply with these terms.
*Correspondence: Mara S. Roset, bXJvc2V0QGlpYi51bnNhbS5lZHUuYXI=; Gabriel Briones, Z2JyaW9uZXNAaWliLnVuc2FtLmVkdS5hcg==
Disclaimer: All claims expressed in this article are solely those of the authors and do not necessarily represent those of their affiliated organizations, or those of the publisher, the editors and the reviewers. Any product that may be evaluated in this article or claim that may be made by its manufacturer is not guaranteed or endorsed by the publisher.
Research integrity at Frontiers
Learn more about the work of our research integrity team to safeguard the quality of each article we publish.