- 1Department of Microbiology and Immunology, Cornell University, Ithaca, NY, United States
- 2Department of Health Promotion and Physical Education, Ithaca College, Ithaca, NY, United States
- 3Department of Biology, Macalester College, Saint Paul, MN, United States
- 4Cornell Cooperative Extension of Suffolk County, Southold, NY, United States
- 5Northeast Center for Vibrio Disease and Ecology, University of New Hampshire, Durham, NH, United States
- 6Department of Molecular, Cellular, and Biomedical Sciences, University of New Hampshire, Durham, NH, United States
- 7Department of Natural Resources and the Environment, University of New Hampshire, Durham, NH, United States
- 8Department of Food Science, Cornell University, Ithaca, NY, United States
- 9Florida Department of Health, Tallahassee, FL, United States
Introduction: Gastrointestinal illnesses associated with the consumption of shellfish contaminated with Vibrio parahaemolyticus have a negative impact on the shellfish industry due to recalls and loss of consumer confidence in products. This bacterial pathogen is very diverse and specific sequence types (STs), ST631 and ST36, have emerged as prevalent causes of Vibrio foodborne disease outbreaks in the US, though other STs have been implicated in sporadic cases. We investigated whether bacteriophages could be used as a proxy to monitor for the presence of distinct V. parahaemolyticus STs in coastal waters.
Methods: For this purpose, bacteriophages infecting V. parahaemolyticus were isolated from water samples collected on the Northeast Atlantic coast. The isolated phages were tested against a collection of 29 V. parahaemolyticus isolates representing 18 STs, including six clonal complexes (CC). Four distinct phages were identified based on their ability to infect different sets of V. parahaemolyticus isolates.
Results and Discussion: Overall, the 29 bacterial isolates segregated into one of eight patterns of susceptibility, ranging from resistance to all four phages to susceptibility to any number of phages. STs represented by more than one bacterial isolate segregated within the same pattern of susceptibility except for one V. parahaemolyticus ST. Other patterns of susceptibility included exclusively clinical isolates represented by distinct STs. Overall, this study suggests that phages populating coastal waters could be exploited to monitor for the presence of V. parahaemolyticus STs known to cause foodborne outbreaks.
Introduction
Gastrointestinal illnesses associated with the consumption of shellfish contaminated with pathogenic Vibrio parahaemolyticus have increased in prevalence in recent decades (Haendiges et al., 2014; Newton et al., 2014; Xu et al., 2015; Baker-Austin et al., 2016; Abanto et al., 2020). Between 2009 and 2020, the CDC National Outbreak Reporting System (NORS) documented 151 outbreaks related to Vibrio contaminated food in the United States.1 In 2014 alone, it was estimated that V. parahaemolyticus accounted for over 92,000 individual infections in the United States (Collier et al., 2021). The association of foodborne infections with contaminated shellfish has a significant negative impact not only on public health, but also on the industry due to recalls and, importantly, a loss of consumer confidence in the product. Therefore, there is a need to develop enhanced measures to monitor coastal waters surrounding aquaculture farms for the presence of V. parahaemolyticus associated with food infection.
Vibrio parahaemolyticus is ubiquitous in coastal waters and bacterial growth increases with an increase in water temperature during the summer months (Ellis et al., 2012; Rodgers et al., 2014). Shellfish, such as oysters and clams, filter large amounts of water to acquire nutrients; consequently, shellfish are more susceptible to contamination with V. parahaemolyticus as water temperatures increase during the summer months (Ellis et al., 2012; Rodgers et al., 2014). The National Shellfish Sanitation Program tightly regulates the shellfish industry, and has developed measures to decrease the incidence of contamination from harvest to table.2 Diagnostic tests for detection of V. parahaemolyticus in seafood exist, e.g., detection of toxin genes by PCR and bacterial isolation. However, results from these tests do not distinguish strains that are associated with gastrointestinal infections in humans from those that do not. Vibrio parahaemolyticus is a genetically diverse bacterial species represented by multiple sequence types (STs), some of which are grouped within clonal complexes (CC; Mahoney et al., 2010; Schuster et al., 2011; Ellis et al., 2012; Whistler et al., 2015; Xu et al., 2015, 2017a,b). The large majority of V. parahaemolyticus strains are avirulent and no known absolute common genetic features exist among virulent strains (Ronholm et al., 2015). However, specific V. parahaemolyticus STs and CCs have been associated with foodborne illnesses. For example, ST3, ST36, ST631 have been the main STs associated with outbreaks. About 25 years ago, infectious V. parahaemolyticus strains belonging to CC3, which includes ST3, emerged in India and rapidly disseminated worldwide (Nair et al., 2007). ST3 is still abundant in Asia (Tan et al., 2021), but strains belonging to ST36 and ST631 have been the prevalent sources of foodborne infections in the United States in recent decades (Haendiges et al., 2014; Xu et al., 2015, 2017a,b). ST36 was initially isolated from the Pacific Northwest, and subsequently spread to the Atlantic Northeast. Vibrio parahaemolyticus ST36 caused an outbreak in Spain in 2012 and was identified as a source of infection in Peru between 2012 and 2016 (Abanto et al., 2020). In addition, some STs have been associated with sporadic clinical cases (Miller et al., 2021).
Bacteriophages that infect Vibrio spp. including V. parahaemolyticus have been previously isolated from both seafood and water samples (Wang et al., 2017; Chen et al., 2019; Richards et al., 2019; Le et al., 2020; Tan et al., 2021). In the present study, we assessed whether bacteriophages that populate coastal waters surrounding oyster farms could be used to assess for the presence of specific V. parahaemolyticus STs. Four de novo isolated bacteriophages were selected based on their ability to infect distinct sets of V. parahaemolyticus isolates. The 29 V. parahaemolyticus isolates, representing 18 STs, segregated into eight phage susceptibility patterns, each composed of up to four distinct STs. The potential applications of monitoring phage populations in coastal waters to assess for the presence of V. parahaemolyticus STs are considered in the discussion.
Materials and methods
Bacterial strains and growth conditions
Twenty-nine isolates of V. parahaemolyticus were obtained from three different labs (Table 1). This array of strains was comprised of clinical, environmental, and seafood isolates, many of which have been previously characterized and include a broad representation of isolation dates and STs. Escherichia coli, Bacillus subtilis, Pseudomonas fluorescens, Pseudomonas aeruginosa, Edwardsiella piscicida, Vibrio cholerae, and Aeromonas hydrophila were included in the study to demonstrate species specificity to bacteriophage infection. Bacteria were grown in LB Lennox (LBL), Tryptic Soy Broth (TSB) or, on Tryptic Soy Agar (TSA) supplemented with 2% NaCl. Isolates were stored at −80°C in 50%glycerol/50%TSB + 2%NaCl.
Sequence typing of Vibrio parahaemolyticus isolates
STs of isolates were either characterized or confirmed by Multi Locus Sequence Typing (MLST) according to the standard method described by Jolley and Maiden (2010) and the database maintained by the University of Oxford and supported by the Wellcome Trust: https://pubmlst.org/organisms/vibrio-parahaemolyticus/ (Mahoney et al., 2010; Ellis et al., 2012; Whistler et al., 2015; Xu et al., 2015, 2017a,b). Chromosomal DNA was purified from each isolate, and seven genes were amplified by PCR using primers listed on the website indicated above. PCR products were purified and submitted to the Cornell genomics facility for sequencing. Sequence results were submitted to the online database to identify each bacterial isolate ST. Results are listed in Supplementary Table S2.
Phage isolation
Phage were isolated from water samples collected along the North and South rims of Long Island, New York, in July 2017 (Supplementary Table S1). Salinity was measured with an American Marine Pinpoint Conductivity Monitor: conversion from μSiemens to ppt was performed as follows according to instructions: [(μSiemens/33) * 17.9]/1,000. All water samples were filtered through an 8 μm filter to eliminate large debris. Some of the water samples were subsequently filtered (0.22 μm) before use (samples indicated in phage name with an F for filtered with 0.22 μm and U for unfiltered). All samples were stored in the dark at 4°C. pH was measured with a Corning pH meter 430 equipped with an automatic temperature compensation (ATC) electrode. Not all water samples collected produced phage.
Vibrio parahaemolyticus infectious phages that were present in the collected coastal water samples were enriched as follows. For each water sample, 1 ml of 10X Tryptic Soy Broth (TSB) was diluted with 9 ml of coastal water and supplemented with 10 mM MgSO4. The broth was inoculated with seven isolates of V. parahaemolyticus: Strain ID# FSL Y1-012, FSL Y1-017, FSL Y1-036, FSL Y1-078, MDOH-04-5M732, F113A, and MA561 listed in Table 1, representing six STs. The seven isolates were selected for inoculation and incubation together. Isolates selected for the study included STs involved in outbreaks, and isolates from both sporadic clinical cases and food sources. Cultures were incubated at 30°C, 200 rpm overnight. Overnight cultures were treated with 500 μl of chloroform at 30°C for 30 min. Supernatants containing phages were cleared by centrifugation and stored at 4°C.
Individual phages were isolated using a plaquing assay. Isolates of V. parahaemolyticus were grown at 30°C 200 rpm to log phase in TSB-NaCl. Each V. parahaemolyticus isolate (200 μl) was mixed with phage supernatant (200 μl) and 10 mM MgSO4, then incubated at 30°C 20 min. Soft agar overlays were prepared by mixing 3.2 ml of melted Tryptic Soy Soft Agar (TSSA has 0.75% agar) + 2% NaCl at 55°C, with 200 μl of bacteria/phage reaction, and 10 mM MgSO4. The melted overlay was briefly vortexed and poured over a plate of TSA-NaCl. After an overnight incubation at 30°C, overlays were examined for the presence of plaques. Plaques were picked with a sterile tip and suspended in a small volume of SM buffer (100 mM NaCl, 8 mM MgSO4, 50 mM Tris–HCl pH 7.5, 0.01% gelatin) and treated with chloroform. Each phage was further purified by 3–4 rounds of plaquing and re-isolation of single plaques from overlays. In order to decrease the possibility of isolating sibling phages, a maximum of three phenotypically different plaques were picked per water sample.
Characterization of phage infectivity range
Isolated phages were tested for plaquing with the 29 V. parahaemolyticus isolates and with E. coli, B. subtilis, P. fluorescens, P. aeruginosa, E. piscicida, V. cholerae, and A. hydrophila (to demonstrate bacteriophage species specificity). Bacterial overlays in soft agar were prepared as follows. Bacteria were grown overnight at 30°C 200 rpm. After overnight growth, bacteria were diluted and grown again in broth to exponential phase. 135 μl of a bacterial isolate grown in broth to exponential phase was mixed with 3.2 ml of melted TSSA +2% NaCl at 55°C. Serial dilutions of phages were spotted on the solidified agar (2 μl per spot) and incubated overnight at 30°C. Overlays were examined for the formation of individual plaques.
CsCl phage purification
Vibrio parahaemolyticus FSL Y1-078 was cultured in 500 ml of TSB-NaCl supplemented with 10 mM MgSO4 to an OD600 of 0.2 and infected with a specific phage (27Ua.3, 29Fa.3, 31Fb.4, or 33Fb.4) at a MOI of ≈10. After an overnight incubation at 30°C 200 rpm, 5 ml of chloroform were added, and the incubation was continued for 10 min. RNAse and DNAse were added to a concentration of 1 μg/ml. NaCl was added to reach a final concentration of 1 M, followed by a 1-h incubation on ice. Particulates were pelleted at 15,000 g for 20 min at 4°C and the supernatant was decanted. Phages were precipitated from the supernatant by the gradual addition of 50 g of PEG8000 and incubated for a minimum of 2 h on ice water. Phages were pelleted at 11,000g for 15 min at 4°C and resuspended in 8 ml of SM Buffer. An equal volume of chloroform was added to extract PEG and the suspension was centrifuged at 3,000g for 15 min at 4°C. The aqueous layer was recovered, and the chloroform extraction was repeated until the PEG was all extracted. Phage volume was brought up to 10 ml with SM buffer and mixed with CsCl to a final concentration of 0.78 g/ml The suspension was centrifuged at 225,000g for 24 h at 4°C using a swinging bucket rotor. The band of purified phage was recovered, and the phage suspension was dialyzed against SM at 4°C. Purified phage was stored at 4°C. Phage titer was determined by spotting serial dilutions on an overlay of V. parahaemolyticus FSL Y1-078.
Phage sequencing
Genomic DNA was purified from CsCl-purified phages using Zymo Research Viral DNA kit. The samples were submitted for whole genome sequencing to the Molecular Diagnostics Laboratory of the Animal Health Diagnostic Center of Cornell University. The libraries were constructed with Nextera XT DNA Library Prep with custom barcodes, random PCR (GenBank accession numbers in Supplementary Table S3).
Genomic and phylogenetic analysis
Open reading frame (ORF) calling and preliminary annotation for each assembled phage genome was performed using pharokka (v1.0.0) with default parameters (Laslett and Canback, 2004; Chen et al., 2005; Bland et al., 2007; Steinegger and Soding, 2017; McNair et al., 2019; Alcock et al., 2020; Chan et al., 2021; Terzian et al., 2021). For phylogenetic comparisons of the major capsid and major tail proteins, seven publicly available V. parahaemolyticus phage sequences were downloaded from NCBI (Supplementary Table S3; Seguritan et al., 2003; Alanis Villa et al., 2012; Kim et al., 2012; Yuan et al., 2014; Lal and Ransangan, 2015; Pan et al., 2020). For consistent annotations, ORFs for major capsid and major tail genes were predicted using PhANNs with default parameters (Cantu et al., 2020). Major capsid and major tail sequences were identified as the highest scoring ORF, with median (±SE) PhANNs scores of 7.7 ± 0.2 and 5.9 ± 0.1, respectively, which correspond to reported confidence levels of ~95% and 85%, respectively. One previously published phage, VP16T, did not have an ORF score above 2.5 (~80% confidence) for the major tail protein and was dropped from that analysis. Phylogenetic analyses were performed using MEGA11 (version 11.0.11; Tamura et al., 2021): amino acid alignments for the PhANNs-identified ORFs for each protein were generated using MUSCLE, and then used to construct neighbor-joining trees with 1,000 bootstrap replicates.
Electron microscopy
CsCl-purified phages were visualized by transmission electron microscopy (TEM). Samples were deposited onto a copper, 200 mesh, formvar and carbon coated grid and stained with 2% aqueous uranyl acetate. Samples were viewed on an FEI Tecnai 12 Biotwin transmission electron microscope. Images were taken with a high-resolution, high-contrast, thermoelectrically (TE) cooled Gatan Orius® 1000 dual-scan CCD camera. It acquires 4,008 × 2,672 digital images, using Digital Micrograph (DM) software.
Results
Isolation of phages from coastal waters
Phages that were infectious for V. parahaemolyticus were isolated from water samples collected on July 31, 2017, at seven GPS locations along the North and South shores of Long Island, New York (Supplementary Table S1). Water parameters were as follows: temperature of 24°C–28°C, salinity of 21.30–24.20 ppt, and pH 7.5 to 8.3. Phages were identified by the formation of plaques in V. parahaemolyticus soft agar. The purified phages were stored in SM buffer at 4°C and amplified as needed in broth cultures of V. parahaemolyticus.
Determination of phage infectivity range
The purified phages were initially tested against a collection of 29 V. parahaemolyticus isolates. Four phages, each one from a different water sample, were retained for their unique pattern of infectivity: 27Ua.3, 29Fa.3, 31Fb.4, and 33Fb.4 (Table 2). These four selected phages were infectious for subsets of seven to 12 V. parahaemolyticus isolates as determined by the formation of plaques on V. parahaemolyticus soft agar. A total of 17 V. parahaemolyticus strains were susceptible to at least one of the phages, whereas 12 V. parahaemolyticus strains were resistant to the four selected phages. The other phages not selected for further analyses either showed the same infectivity pattern as one of the four selected phages or inconsistency in infection. None of the other bacterial species tested (E. coli, B. subtilis, P. fluorescens, P. aeruginosa, E. piscicida, V. cholerae, and A. hydrophila) were susceptible to the four selected phages.
To gain a better understanding of the infectivity of 27Ua.3, 29Fa.3, 31Fb.4, and 33Fb.4, the ST of each V. parahaemolyticus strain used in this study was determined (Table 1; Supplementary Table S2). The 29 V. parahaemolyticus strains represented 18 different STs that segregated into eight distinct patterns of susceptibility (PoS A-H; Table 3). Strains belonging to ST3 (CC3), ST1464 (CC3), ST8 (CC8), and ST87 were resistant to all four phages (PoS A), whereas strains belonging to ST36 (CC36) and ST46 were susceptible to all four phages (PoS H). Strains belonging to ST26 (CC24), ST88 (CC345), ST326, ST676, ST154, and ST1748 were susceptible to a single phage: 29Fa.3 (PoS B) or 33Fb.4 (PoS C). ST2666 and ST34 strains were the only representative of PoS D and PoS G, respectively. The two ST54 isolates were susceptible to different sets of phages (PoS E and PoS F): both isolates were susceptible to 31Fb.4 and 33Fb.4, but the second isolate was also susceptible to 27Ua.3 (PoS F). In addition, PoS F included the ST631 strain.
Phage characterization by electron microscopy
All four purified phages have icosahedral heads with long flexible non-contractile tails, characteristic of double-stranded DNA viruses of the Siphoviridae family (Figure 1). Head and tail measurements are reported in Table 4. Three of the phages, (27Ua.3, 31Fb.4, and 33Fb.4) have elongated prolate heads of 86–95 nm in length × 49–55 nm in width, with tails of 152–157 nm in length, whereas 29Fa.3 has a round head of 70 nm in diameter and a tail of 233 nm in length.
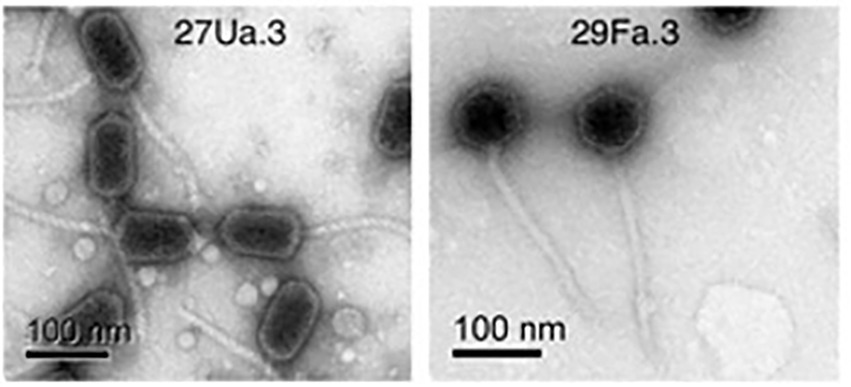
Figure 1. Representative purified phages were visualized by transmission electron microscopy. Left panel: 27Ua.3 showing elongated prolate heads with long tails (31Fb.4, and 33Fb.4 not shown had similar morphology). Right panel: 29Fa.3 showing icosahedral heads and long tails.
Phage sequences and phylogenetic analysis
The sequenced and assembled phages 27Ua.3, 29Fa.3, 31Fb.4, and 33Fb.4 each produced a single contig with high read coverage: genome sizes were 76.890, 79.348, 77.620, and 77.632 kb, with calculated GC contents of 48.8%, 46.8%, 48.9%, and 48.9%, respectively. Putative ORFs and preliminary annotations were predicted using pharokka (v1.0.0) with default parameters (Laslett and Canback, 2004; Chen et al., 2005; Bland et al., 2007; Steinegger and Soding, 2017; McNair et al., 2019; Alcock et al., 2020; Chan et al., 2021; Terzian et al., 2021).
To determine whether phylogenetic relationships between the isolated novel phage mirrored the infectivity patterns, we generated protein alignments of the predicted major capsid and major tail proteins of the four purified phages (27Ua.3, 29Fa.3, 31Fb.4, and 33Fb), as well as seven previously published V. parahaemolyticus phages (Supplementary Table S3). Because the novel and published phage genomes lacked annotation for many structural proteins, we first predicted structural protein ORFs for each using PhANNs (Cantu et al., 2020). Phylogenetic analysis of the PhANNs-predicted major capsid and major tail sequences reveal that phages 31Fb.4, 33Fb.4 and 27Ua.3 are closely related, and share major capsid gene similarity with phage MAR10 (Villa et al., 2012; Figure 2). Phage 29Fa.3 was more closely related to other phage included in the comparison, though its closest relative varied depending on the protein used for tree construction. These phylogenetic patterns parallel in part the phage infectivity profiles (Table 3), with 31Fb.4 and 33Fb.4 infecting nearly the same STs, and 29Fa.3 having the most distinct profile.
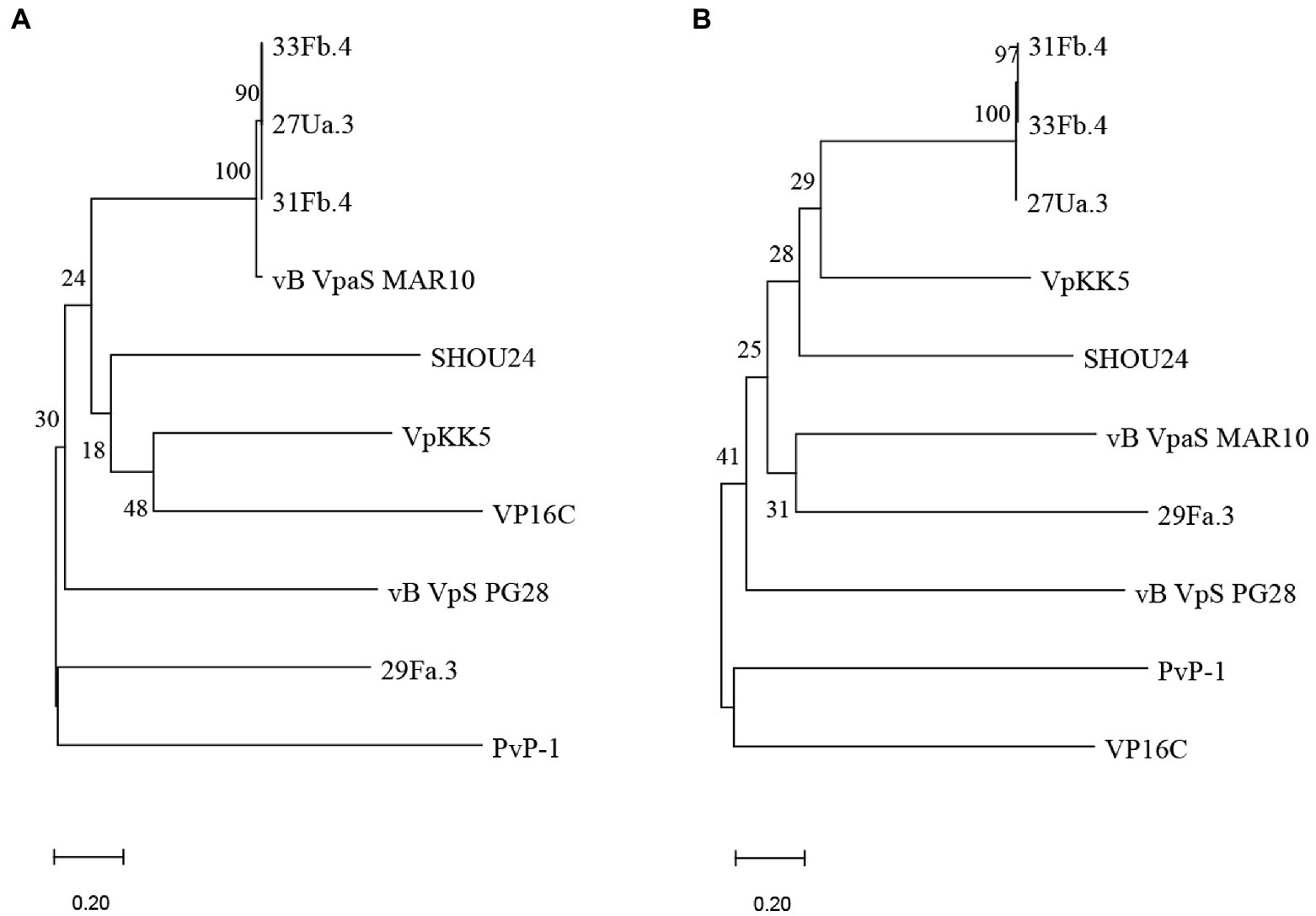
Figure 2. Phylogenetic trees based on the predicted major capsid (A) and major tail (B) proteins of the phages isolated in this study (27Ua.3, 29Fa.3, 31Fb.4, and 33Fb.4) and other publicly available Vibrio parahaemolyticus phages. Protein identities were predicted from ORFs or coding sequences using PhANNs, and analyzed using MEGA11: all putative amino acid sequences were aligned using MUSCLE and phylogenetic trees were constructed by neighbor-joining with 1,000 bootstrap replicates. Nodes are marked with the bootstrap value as percent.
Discussion
In this study, we explored the possibility that monitoring coastal waters for bacteriophages could facilitate early detection of V. parahaemolyticus STs potentially associated with seafood-borne illness. Using a selection of four bacteriophages enriched and isolated from New York Atlantic coastal waters and 29 strains of V. parahaemolyticus representing 18 STs, we observed that different STs were susceptible to different sets of phages. First, the nine isolates representing ST3 and the single ST1464 isolate were resistant to infection by all four phages. ST3 and ST1464 are part of CC3, which predominated seafood outbreaks in 1997–1998 (Nair et al., 2007), but have now been replaced by ST36 and ST631 in the United States (Haendiges et al., 2014; Xu et al., 2015, 2017a,b). This result suggests that V. parahaemolyticus belonging to CC3 were absent from coastal waters at the time of this study, as phage propagation is dependent on the presence of its specific bacterial host. On the other hand, ST36 was susceptible to all four phages isolated from the coastal waters, suggesting that ST36 likely populated the coastal waters at the time of collection. However, this susceptibility pattern was not restricted to ST36, as two ST46 isolates of unknown origin were also susceptible to all four phages. ST631 was susceptible to the same set of phages as one of the ST54 strains, which originated from a clinical case. Also noteworthy were patterns of susceptibility C and D, which included isolates of three different STs from sporadic clinical cases. In addition, an isolate from oysters (ST34 (NH/ME)) had a unique pattern of susceptibility (PoS G); ST34 has previously been associated with clinical cases (Miller et al., 2021).
A previous study showed that phages enriched from oysters out of Delaware Bay did not infect V. parahaemolyticus serotype O3:K6 (during specific collection periods), the serotype representing a previous pandemic strain (Richards et al., 2019). The present study included eight V. parahaemolyticus strains representing serotype O3:K6 (including FSL Y1-016, 017, 021, 023, 024, 025, 026, and 046; Yeung and Boor, 2004). These strains, which are part of CC3 with the exception of FSL Y1-021 (ST87), were resistant to infection by all four phages. Together, these results suggest that V. parahaemolyticus strains belonging to CC3 were absent from coastal waters at the time of these two independent studies, supporting the possibility that phages could be used to monitor the populations of V. parahaemolyticus STs that prevail around oyster farms at various times of the year. However, expanding the number of phage isolates and V. parahaemolyticus strains to identify greater inclusivity and specificity would be necessary for this approach to be useful as a prevention measure for potential food outbreaks.
The four phages isolated in this study (27Ua.3, 29Fa.3, 31Fb.4, and 33Fb.4) were sequenced and genome lengths and percent GC content values were compared to other vibriophage data available in the NCBI database. The previously published phages demonstrated characteristics of Siphoviruses with the exception of vBVpSPG28 showing tail morphology similar to that of Myoviruses (Tian et al., 2022). Phage genome lengths and percent GC content were similar to vB VpaS MAR10 (isolated off the coast of Mexico) and SHOU24 (isolated from aquatic market sewage in Shanghai, China; Villa et al., 2012; Yuan et al., 2014). Of the previously published phages, only vB VpaS MAR10 has an elongated head similar to those of 27Ua.3, 31Fb.4, and 33Fb.4 (Villa et al., 2012); the phylogenetic analysis of the major capsid protein of these four phages indicate that they are closely related. However, there is no information related to the infectivity of these other phages for the various STs representing V. parahaemolyticus strains.
Vibrio parahaemolyticus is a very diverse bacterial species. Some strains are human pathogens, others are pathogenic for aquatic animals, whereas many have no known pathogenic characteristics. Current diagnostic methods of V. parahaemolyticus, which require bacterial culture and biochemical, phenotypic, or molecular analyses can be time consuming and labor intensive. Newer molecular approaches for identification that are growing in use, including the 4 amplicon multiplex PCR detection assay designed to specifically identify ST36 isolates, have reduced the time to a result but are still a resource burden (Letchumanan et al., 2014; Whistler et al., 2015). To reduce costs and time to a result, the LAMP (loop-mediated isothermal amplification) test that is widely used to detect SARS-CoV2, was modified to detect V. parahaemolyticus in clinical samples (Zhou et al., 2021). Unfortunately, this test has limited accuracy. Thus, there is a need to develop additional methods that are cost efficient and specific for foodborne-related pathogenic strains of V. parahaemolyticus.
The present study informs future applications for use of bacteriophages in differentiating V. parahaemolyticus ST and screening for the presence of STs associated with foodborne illnesses.
Phages could be used to help monitor coastal waters in which shellfish are cultured. As phages are relatively easy to isolate and multiply exponentially when their specific hosts are abundant, newly isolated phages could be used as an initial proxy for detection of V. parahaemolyticus STs populating the waters. Considering that bacteria can acquire resistance to phages they encounter (Bondy-Denomy and Davidson, 2014), the battery of phages used to monitor waters will have to be constantly updated using V. parahaemolyticus isolates from recent infections. Moreover, large scale studies will be needed to assess statistical significance and correlation.
In conclusion, this study identified and sequenced four novel phages infectious for 18 distinct STs of V. parahaemolyticus. Phylogenetic analyses suggest that three of these novel phages form a new clade distinct from previously sequenced and published V. parahaemolyticus phage. The distinct phage susceptibility patterns of the various V. parahaemolyticus STs support the potential use of phages as a means for monitoring contamination of waters and shellfish. Pre-harvest detection of V. parahaemolyticus STs of interest would contribute to decreased morbidity and mortality due to foodborne infections, increase consumer confidence in the safety of shellfish, and consequently increase the profitability and sustainability of the industry.
Data availability statement
The data presented in the study are deposited in GenBank with accession numbers OP547477, OP547478, OP595601, OP595602.
Author contributions
KBS: study design, sample collection, data acquisition, analyses, interpretation, manuscript writing, manuscript revision. JR: sample collection, data acquisition, analyses, manuscript writing, manuscript revision. RS-C: data acquisition, analyses, interpretation, manuscript writing, manuscript revision. KLS: sample collection, data acquisition, analyses, manuscript revision. SC: data acquisition, analyses, manuscript writing, manuscript revision. MH: data acquisition, manuscript revision. GR: study review, sample collection, manuscript revision, CW and MW: study review, strain supply, manuscript revision. SJ: study review, manuscript revision. JD: strain supply, manuscript revision. RG: study review, laboratory consultation, manuscript revision. HM: study conception, study design, sample collection, analyses, interpretation, manuscript writing, manuscript revision. All authors contributed to the article and approved the submitted version.
Funding
This project was funded by the Northeastern Regional Aquaculture Center Award No. 2016-38500-25754 and Cornell Center for Materials Research Shared Facilities which are supported through the NSF MRSEC program (DMR-1719875).
Acknowledgments
Sequencing was performed by the Molecular Diagnostics Laboratory of the Animal Health Diagnostic Center. We thank Patrick Mitchell of the Molecular Diagnostics Laboratory and Steven Bogdanowicz from the Department of Ecology and Evolutionary Biology for technical support with sequence analysis, and Randi Foxall from the University of New Hampshire for critical review of the manuscript. We are grateful to Dave Relyea from FM Flower and Sons and Charles Westfall from Thatch Island and Blue Island Oysters for their assistance in collecting water samples.
Conflict of interest
The authors declare that the research was conducted in the absence of any commercial or financial relationships that could be construed as a potential conflict of interest.
Publisher’s note
All claims expressed in this article are solely those of the authors and do not necessarily represent those of their affiliated organizations, or those of the publisher, the editors and the reviewers. Any product that may be evaluated in this article, or claim that may be made by its manufacturer, is not guaranteed or endorsed by the publisher.
Supplementary material
The Supplementary material for this article can be found online at: https://www.frontiersin.org/articles/10.3389/fmicb.2022.1041942/full#supplementary-material
Footnotes
1. ^https://wwwn.cdc.gov/norsdashboard/
2. ^https://www.fda.gov/food/federalstate-food-programs/national-shellfish-sanitation-program-nssp
References
Abanto, M., Gavilan, R. G., Baker-Austin, C., Gonzalez-Escalona, N., and Martinez-Urtaza, J. (2020). Global expansion of Pacific northwest Vibrio parahaemolyticus sequence type 36. Emerg. Infect. Dis. 26, 323–326. doi: 10.3201/eid2602.190362
Alanis Villa, A., Kropinski, A. M., Abbasifar, R., and Griffiths, M. W. (2012). Complete genome sequence of Vibrio parahaemolyticus bacteriophage vB_VpaM_MAR. J. Virol. 86, 13138–13139. doi: 10.1128/JVI.02518-12
Alcock, B. P., Raphenya, A. R., Lau, T. T. Y., Tsang, K. K., Bouchard, M., Edalatmand, A., et al. (2020). CARD 2020: antibiotic resistome surveillance with the comprehensive antibiotic resistance database. Nucleic Acids Res. 48, D517–D525. doi: 10.1093/nar/gkz935
Baker-Austin, C., Trinanes, J. A., Salmenlinna, S., Lofdahl, M., Siitonen, A., Taylor, N. G., et al. (2016). Heat wave-associated vibriosis, Sweden and Finland, 2014. Emerg. Infect. Dis. 22, 1216–1220. doi: 10.3201/eid2207.151996
Bland, C., Ramsey, T. L., Sabree, F., Lowe, M., Brown, K., Kyrpides, N. C., et al. (2007). CRISPR recognition tool (CRT): a tool for automatic detection of clustered regularly interspaced palindromic repeats. BMC Bioinf. 8:209. doi: 10.1186/1471-2105-8-209
Bondy-Denomy, J., and Davidson, A. R. (2014). To acquire or resist: the complex biological effects of CRISPR-Cas systems. Trends Microbiol. 22, 218–225. doi: 10.1016/j.tim.2014.01.007
Cantu, V. A., Salamon, P., Seguritan, V., Redfield, J., Salamon, D., Edwards, R. A., et al. (2020). PhANNs, a fast and accurate tool and web server to classify phage structural proteins. PLoS Comput. Biol. 16:e1007845. doi: 10.1371/journal.pcbi.1007845
Chan, P. P., Lin, B. Y., Mak, A. J., and Lowe, T. M. (2021). tRNAscan-SE 2.0: improved detection and functional classification of transfer RNA genes. Nucleic Acids Res. 49, 9077–9096. doi: 10.1093/nar/gkab688
Chen, L., Fan, J., Yan, T., Liu, Q., Yuan, S., Zhang, H., et al. (2019). Isolation and characterization of specific phages to prepare a cocktail preventing vibrio sp. Va-F3 infections in shrimp (Litopenaeus vannamei). Front. Microbiol. 10:10. doi: 10.3389/fmicb.2019.02337
Chen, L., Yang, J., Yu, J., Yao, Z., Sun, L., Shen, Y., et al. (2005). VFDB: a reference database for bacterial virulence factors. Nucleic Acids Res. 33, D325–D328. doi: 10.1093/nar/gki008
Collier, S. A., Deng, L., Adam, E. A., Benedict, K. M., Beshearse, E. M., Blackstock, A. J., et al. (2021). Estimate of burden and direct healthcare cost of infectious waterborne disease in the United States. Emerg. Infect. Dis. 27, 140–149. doi: 10.3201/eid2701.190676
Ellis, C. N., Schuster, B. M., Striplin, M. J., Jones, S. H., Whistler, C. A., and Cooper, V. S. (2012). Influence of seasonality on the genetic diversity of Vibrio parahaemolyticus in New Hampshire shellfish waters as determined by multilocus sequence analysis. Appl. Environ. Microbiol. 78, 3778–3782. doi: 10.1128/AEM.07794-11
Haendiges, J., Rock, M., Myers, R. A., Brown, E. W., Evans, P., and Gonzalez-Escalona, N. (2014). Pandemic Vibrio parahaemolyticus, Maryland, USA, 2012. Emerg. Infect. Dis. 20, 718–720. doi: 10.3201/eid2004.130818
Jolley, K. A., and Maiden, M. C. (2010). BIGSdb: scalable analysis of bacterial genome variation at the population level. BMC Bioinf. 11:595. doi: 10.1186/1471-2105-11-595
Kim, J. H., Jun, J. W., Choresca, C. H., Shin, S. P., Han, J. E., and Park, S. C. (2012). Complete genome sequence of a novel marine siphovirus, pVp-1, infecting Vibrio parahaemolyticus. J. Virol. 86, 7013–7014. doi: 10.1128/JVI.00742-12
Lal, T. M., and Ransangan, J. (2015). Complete genome sequence of VpKK5, a novel Vibrio parahaemolyticus lytic siphophage. Genome Announc. 3, e01381–14. doi: 10.1128/genomeA.01381-14
Laslett, D., and Canback, B. (2004). ARAGORN, a program to detect tRNA genes and tmRNA genes in nucleotide sequences. Nucleic Acids Res. 32, 11–16. doi: 10.1093/nar/gkh152
Le, T. S., Southgate, P. C., O'Connor, W., Vu, S. V., and Kurtboke, D. I. (2020). Application of bacteriophages to control vibrio alginolyticus contamination in oyster (Saccostrea glomerata) larvae. Antibiotics (Basel). 9:415. doi: 10.3390/antibiotics9070415
Letchumanan, V., Chan, K. G., and Lee, L. H. (2014). Vibrio parahaemolyticus: a review on the pathogenesis, prevalence, and advance molecular identification techniques. Front. Microbiol. 5:705. doi: 10.3389/fmicb.2014.00705
Mahoney, J. C., Gerding, M. J., Jones, S. H., and Whistler, C. A. (2010). Comparison of the pathogenic potentials of environmental and clinical Vibrio parahaemolyticus strains indicates a role for temperature regulation in virulence. Appl. Environ. Microbiol. 76, 7459–7465. doi: 10.1128/AEM.01450-10
McNair, K., Zhou, C., Dinsdale, E. A., Souza, B., and Edwards, R. A. (2019). PHANOTATE: a novel approach to gene identification in phage genomes. Bioinformatics 35, 4537–4542. doi: 10.1093/bioinformatics/btz265
Miller, J. J., Weimer, B. C., Timme, R., Ludeke, C. H. M., Pettengill, J. B., Bandoy, D. J. D., et al. (2021). Phylogenetic and biogeographic patterns of Vibrio parahaemolyticus strains from North America inferred from whole-genome sequence data. Appl. Environ. Microbiol. 87, e01403–20. doi: 10.1128/AEM.01403-20
Nair, G. B., Ramamurthy, T., Bhattacharya, S. K., Dutta, B., Takeda, Y., and Sack, D. A. (2007). Global dissemination of Vibrio parahaemolyticus serotype O3:K6 and its serovariants. Clin. Microbiol. Rev. 20, 39–48. doi: 10.1128/CMR.00025-06
Newton, A. E., Garrett, N., Stroika, S. G., Halpin, J. L., Turnsek, M., Mody, R. K., et al. (2014). Increase in Vibrio parahaemolyticus infections associated with consumption of Atlantic coast shellfish--2013. MMWR Morb. Mortal. Wkly Rep. 63, 335–336.
Pan, Q., Ren, H., Sun, H., Tong, Y., Yan, Y., and Zhao, F.. (2020). Vibrio phage vB_VpS_PG28, complete genome [GenBank:MT735630.2]. NCBI.
Richards, G. P., Chintapenta, L. K., Watson, M. A., Abbott, A. G., Ozbay, G., Uknalis, J., et al. (2019). Bacteriophages against pathogenic vibrios in Delaware Bay oysters (Crassostrea virginica) during a period of high levels of pathogenic Vibrio parahaemolyticus. Food Environ. Virol. 11, 101–112. doi: 10.1007/s12560-019-09365-5
Rodgers, C., Parveen, S., Chigbu, P., Jacobs, J., Rhodes, M., and Harter-Dennis, J. (2014). Prevalence of Vibrio parahaemolyticus, and Vibrio vulnificus in blue crabs (Callinectes sapidus), seawater and sediments of the Maryland coastal bays. J. Appl. Microbiol. 117, 1198–1209. doi: 10.1111/jam.12608
Ronholm, J., Petronella, N., Chew Leung, C., Pightling, A. W., and Banerjee, S. K. (2015). Genomic features of environmental and clinical Vibrio parahaemolyticus isolates lacking recognized virulence factors are dissimilar. Appl. Environ. Microbiol. 82, 1102–1113. doi: 10.1128/AEM.03465-15
Schuster, B. M., Tyzik, A. L., Donner, R. A., Striplin, M. J., Almagro-Moreno, S., Jones, S. H., et al. (2011). Ecology and genetic structure of a northern temperate vibrio cholerae population related to toxigenic isolates. Appl. Environ. Microbiol. 77, 7568–7575. doi: 10.1128/AEM.00378-11
Seguritan, V., Feng, I. W., Rohwer, F., Swift, M., and Segall, A. M. (2003). Genome sequences of two closely related Vibrio parahaemolyticus phages, VP16T and VP16C. J. Bacteriol. 185, 6434–6447. doi: 10.1128/JB.185.21.6434-6447.2003
Steinegger, M., and Soding, J. (2017). MMseqs2 enables sensitive protein sequence searching for the analysis of massive data sets. Nat. Biotechnol. 35, 1026–1028. doi: 10.1038/nbt.3988
Tamura, K., Stecher, G., and Kumar, S. (2021). MEGA11: molecular evolutionary genetics analysis version 11. Mol. Biol. Evol. 38, 3022–3027. doi: 10.1093/molbev/msab120
Tan, C. W., Rukayadi, Y., Hasan, H., Abdul-Mutalib, N.-A., Jambari, N. N., Hara, H., et al. (2021). Isolation and characterization of six Vibrio parahaemolyticus lytic bacteriophages from seafood samples. Front. Microbiol. :12. doi: 10.3389/fmicb.2021.616548
Terzian, P., Olo Ndela, E., Galiez, C., Lossouarn, J., Perez Bucio, R. E., Mom, R., et al. (2021). PHROG: families of prokaryotic virus proteins clustered using remote homology. NAR Genom Bioinform. 3:lqab067. doi: 10.1093/nargab/lqab067
Tian, F., Li, J., Hu, Y., Zhao, F., Ren, H., Pan, Q., et al. (2022). Characterization and complete genome sequence analysis of a newly isolated phage against Vibrio parahaemolyticus from sick shrimp in Qingdao, China. PLoS One 17:e0266683. doi: 10.1371/journal.pone.0266683
Villa, A. A., Kropinski, A. M., Abbasifar, R., Abbasifar, A., and Griffiths, M. W. (2012). Genome sequence of temperate Vibrio parahaemolyticus bacteriophage vB_VpaS_MAR10. J. Virol. 86, 13851–13852. doi: 10.1128/JVI.02666-12
Wang, Y., Barton, M., Elliott, L., Li, X., Abraham, S., O'Dea, M., et al. (2017). Bacteriophage therapy for the control of Vibrio harveyi in greenlip abalone (Haliotis laevigata). Aquaculture 473, 251–258. doi: 10.1016/j.aquaculture.2017.01.003
Whistler, C. A., Hall, J. A., Xu, F., Ilyas, S., Siwakoti, P., Cooper, V. S., et al. (2015). Use of whole-genome phylogeny and comparisons for development of a multiplex PCR assay to identify sequence type 36 Vibrio parahaemolyticus. J. Clin. Microbiol. 53, 1864–1872. doi: 10.1128/JCM.00034-15
Xu, F., Gonzalez-Escalona, N., Drees, K. P., Sebra, R. P., Cooper, V. S., Jones, S. H., et al. (2017a). Parallel evolution of two clades of a major Atlantic endemic Vibrio parahaemolyticus pathogen lineage by independent acquisition of related pathogenicity islands. Appl. Environ. Microbiol. 83, e01168–e01117. doi: 10.1128/AEM.01168-17
Xu, F., Gonzalez-Escalona, N., Haendiges, J., Myers, R. A., Ferguson, J., Stiles, T., et al. (2017b). Sequence type 631 Vibrio parahaemolyticus, an emerging foodborne pathogen in North America. J. Clin. Microbiol. 55, 645–648. doi: 10.1128/JCM.02162-16
Xu, F., Ilyas, S., Hall, J. A., Jones, S. H., Cooper, V. S., and Whistler, C. A. (2015). Genetic characterization of clinical and environmental Vibrio parahaemolyticus from the Northeast USA reveals emerging resident and non-indigenous pathogen lineages. Front. Microbiol. :6. doi: 10.3389/fmicb.2015.00272
Yeung, P. S. M., and Boor, K. J. (2004). Effects of acid stress on Vibrio parahaemolyticus survival and cytotoxicity. J. Food Protect. 67, 1328–1334. doi: 10.4315/0362-028X-67.7.1328
Yeung, P. S. M., DePaola, A., Kaysner, C. A., and Boor, K. J. (2003). A PCR assay for specific detection of the pandemic Vibrio parahaemolyticus 03: K6 clone from shellfish. J. Food Sci. 68, 1459–1466. doi: 10.1111/j.1365-2621.2003.tb09667.x
Yeung, P. S. M., Hayes, M. C., DePaola, A., Kaysner, C. A., Kornstein, L., and Boor, K. J. (2002). Comparative phenotypic, molecular, and virulence characterization of Vibrio parahaemolyticus O3: K6 isolates. Appl. Environ. Microbiol. 68, 2901–2909. doi: 10.1128/AEM.68.6.2901-2909.2002
Yuan, L., Cui, Z., Wang, Y., Guo, X., and Zhao, Y. (2014). Complete genome sequence of virulent bacteriophage SHOU24, which infects foodborne pathogenic Vibrio parahaemolyticus. Arch. Virol. 159, 3089–3093. doi: 10.1007/s00705-014-2160-x
Zhou, Q. J., Lu, J. F., Su, X. R., Jin, J. L., Li, S. Y., Zhou, Y., et al. (2021). Simultaneous detection of multiple bacterial and viral aquatic pathogens using a fluorogenic loop-mediated isothermal amplification-based dual-sample microfluidic chip. J. Fish Dis. 44, 401–413. doi: 10.1111/jfd.13325
Keywords: Vibrio parahaemolyticus, vibriophage, sequence type, phage, ST36
Citation: Brossard Stoos KA, Ren J, Shields-Cutler RR, Sams KL, Caldwell S, Ho MB, Rivara G, Whistler CA, Jones SH, Wiedmann M, DeMent J, Getchell RG and Marquis H (2022) Coastal water bacteriophages infect various sets of Vibrio parahaemolyticus sequence types. Front. Microbiol. 13:1041942. doi: 10.3389/fmicb.2022.1041942
Edited by:
Alicja Wegrzyn, Institute of Biochemistry and Biophysics (PAS), PolandReviewed by:
Indrani Karunasagar, Nitte University, IndiaCarolin Charlotte Wendling, ETH Zürich, Switzerland
Copyright © 2022 Brossard Stoos, Ren, Shields-Cutler, Sams, Caldwell, Ho, Rivara, Whistler, Jones, Wiedmann, DeMent, Getchell and Marquis. This is an open-access article distributed under the terms of the Creative Commons Attribution License (CC BY). The use, distribution or reproduction in other forums is permitted, provided the original author(s) and the copyright owner(s) are credited and that the original publication in this journal is cited, in accordance with accepted academic practice. No use, distribution or reproduction is permitted which does not comply with these terms.
*Correspondence: Kari A. Brossard Stoos, a3N0b29zQGl0aGFjYS5lZHU=